- 1Case Cardiovascular Research Institute, Case Western Reserve University, Harrington Heart and Vascular Institute, University Hospitals Cleveland Medical Center, Cleveland, OH, United States
- 2Department of Pathology, Case Western Reserve University, Cleveland, OH, United States
The role of inflammation in vascular disease is well recognized, involving dysregulation of both circulating immune cells as well as the cells of the vessel wall itself. Unrestrained vascular inflammation leads to pathological remodeling that eventually contributes to atherothrombotic disease and its associated sequelae (e.g., myocardial/cerebral infarction, embolism, and critical limb ischemia). Signaling events during vascular inflammation orchestrate widespread transcriptional programs that affect the functions of vascular and circulating inflammatory cells. The Krüppel-like factors (KLFs) are a family of transcription factors central in regulating vascular biology in states of homeostasis and disease. Given their abundance and diversity of function in cells associated with vascular inflammation, understanding the transcriptional networks regulated by KLFs will further our understanding of the pathogenesis underlying several pervasive health concerns (e.g., atherosclerosis, stroke, etc.) and consequently inform the treatment of cardiovascular disease. Within this review, we will discuss the role of KLFs in coordinating protective and deleterious responses during vascular inflammation, while addressing the potential targeting of these critical transcription factors in future therapies.
Introduction
The role of inflammation in vascular disease is well recognized, involving dysregulation of both circulating immune cells as well as the cells of the vessel wall itself. Upon exposure to noxious stimuli (altered hemodynamics, circulating inflammatory factors, and oxygenation level), the vessel wall undergoes characteristic changes such as endothelial cell (EC) activation and vascular smooth muscle cell (VSMC) proliferation and migration, leading to the presentation of a “sticky” surface attractive to circulating monocytes and other immune cells. In certain contexts (e.g., acute thrombotic occlusion), this inflammation results in beneficial vascular remodeling that maintains proper perfusion to ischemic organs. During chronic insults, such as in dyslipidemia, unrestrained vascular inflammation leads to pathological remodeling that eventually contributes to atherothrombotic disease and its associated sequelae (e.g., myocardial/cerebral infarction, embolism, and critical limb ischemia).
Signaling events during vascular inflammation orchestrate widespread transcriptional programs that affect the functions of ECs, VSMCs, and circulating inflammatory cells. Central to many of these programs is the nuclear factor (NF)-κB signaling cascade. Upon stimulation by inflammatory stimuli [cytokines, oxidized low-density lipoprotein (oxLDL), glucose], regulatory cytosolic protein IκB is phosphorylated and targeted for ubiquitin–proteosome degradation. This liberates the normally sequestered cofactors p65 and p50 to translocate into the nucleus, where they mediate pro-inflammatory transcription [reviewed in Ref. (1)]. In addition, the coactivator p300 can complex with p65/p50 to stabilize the chromatin structure for effective transcription (2). Outside of NF-κB signaling, regulation of vascular inflammation can also occur through microRNAs (miRs). miRs are non-coding RNAs that regulate post-transcriptional gene expression by inhibiting mRNA translation. Within the scope of vascular inflammation, miRs can either enhance or diminish pathological inflammation, depending on the target genes [reviewed in Ref. (3)]. Multiple facets of vascular cell biology, including NF-κB and miR signaling, are regulated by Krüppel-like factors (KLFs). Within this review, we will discuss the role of KLFs in coordinating protective and deleterious responses during vascular inflammation, while addressing the potential targeting of these critical transcription factors in future therapies.
KLFs Background
Originally discovered as homologs to the Drosophila melanogaster gene, Krüppel (4), KLFs belong to a family of zinc-finger containing transcription factors with roles in cellular development, differentiation, metabolism, and activation. There are 18 currently predicted mammalian KLFs expressed in various tissues and during periods of development. KLFs share within their C-terminal regions three highly conserved zinc-fingers recognizing a 5′-C(A/T)CCC-3′ consensus sequence often near target genes, though the sequence can occur in distant regions as well such as in enhancers. The amino-terminus functions in transactivation or repression and participates also in protein–protein interactions (5). For many KLFs, there is considerable overlap in gene targets within a single cell type. However, despite the homology of structure, binding sequences, and protein interaction targets, there are also substantial differences in downstream transcriptional effects between KLFs. Several excellent reviews are available discussing sequence homology, chromosomal location, and expression pattern of the KLFs (5, 6). Given their abundance and diversity of function in cells associated with vascular inflammation, understanding the transcriptional networks regulated by KLFs will further our understanding of the pathogenesis underlying several pervasive health concerns (e.g., atherosclerosis, stroke, etc.) and consequently inform the treatment of cardiovascular disease.
EC KLFs
The vascular endothelium acts as an initial sensor and transducer of inflammatory stimuli such as disturbed blood flow, cytokines, oxLDL, and advanced glycation end products, often responding with activation of classical inflammatory cascades which have been elegantly dissected over the past few decades. While brief bouts of inflammation, particular during wound healing, are an appropriate physiologic response, endothelial dysfunction resulting in sustained, chronic inflammation is central to a diverse array of cardiovascular diseases. The transcriptional regulation of endothelial inflammation therefore remains of critical interest. An accumulating body of evidence now exists defining key roles for several KLF transcription factors in the control of vascular inflammation, which we review below (Figure 1).
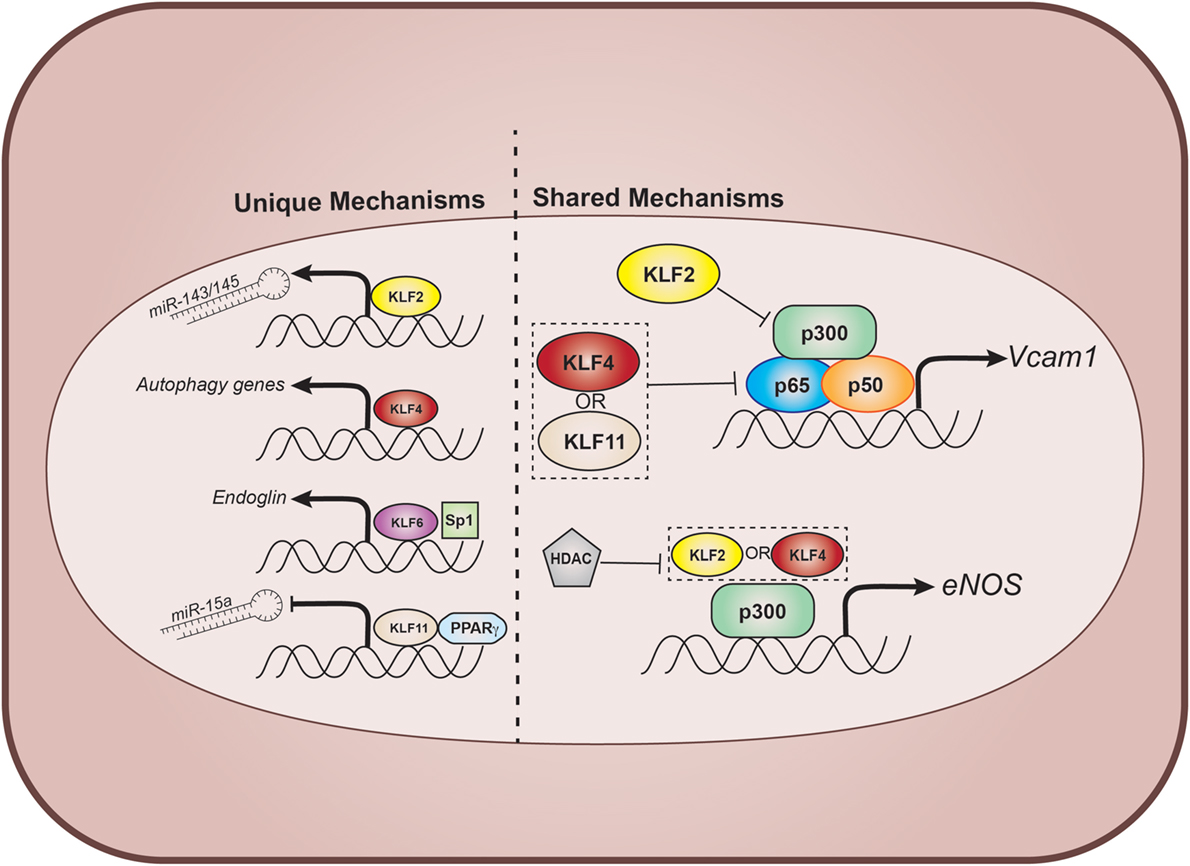
Figure 1. Select effector functions of endothelial KLFs. Within ECs, there are both unique and shared gene targets and binding partners for various KLFs. In general, KLF2, 4, and 11 resist endothelial adhesive transcription by binding to, and inhibiting, multiple cofactors of nuclear factor-κB signaling. Additionally, KLF2 and 4 promote transcription of the vasoprotective factor, eNOS, via cooperation with p300. This process is inhibited by recruitment of HDACs to the eNOS promoter. KLFs also affect endothelial function during vascular inflammation through unique transcriptional events that include modulation of miRs and stimulating protective cellular component recycling through autophagy. KLF, Krüppel-like factor; EC, endothelial cell; HDAC, histone deacetylase; VCAM-1, vascular cell adhesion molecule-1; eNOS, endothelial nitric oxide synthase; miR, microRNA; Sp1, specificity protein 1; PPARγ, peroxisome proliferator-activated receptor gamma.
Krüppel-Like Factor 2
Endothelial KLF2 has primarily anti-inflammatory, anti-thrombotic, and anti-migratory functions. As a regulator of inflammation, KLF2 inhibits both the expression of inflammatory cytokines and the production of adhesion molecules that are critical for leukocyte recruitment and extravasation (7–10). Through its binding to the transcriptional coactivator p300/CBP, KLF2 is capable of preventing NF-κB/p300 interaction and subsequent activation of the vascular cell adhesion molecule-1 (VCAM-1) promoter (11) Furthermore, the KLF2-p300 interaction permits KLF2 binding to the endothelial nitric oxide synthase (eNOS) promoter to induce transcription of this vasoprotective enzyme (11). These early studies demonstrated KLF2’s ability to influence transcription through direct DNA-binding and or indirect cofactor sequestration mechanisms. In addition to affecting the NF-κB pathway, studies have shown that KLF2’s anti-inflammatory effects are also produced through preventing the nuclear translocation of the inflammatory transcription factor ATF2 (9). In response to various stressors, JNK signaling leads to ATF2 nuclear translocation and successive inflammatory transcription. During shear stress, however, KLF2 induces cytoskeletal remodeling that eventually prevents JNK activation, and thus ATF2 translocation (12). Additionally, KLF2 provides protection against oxidative damage in ECs via the induction of hemeoxygenase-1 (13). Furthermore, ECs overexpressing KLF2 secrete atheroprotective miRs-143/145 in microvesicles that reduce atherosclerosis by targeting genes critical for VSMC dedifferentiation (Mmp3, Elk1, Camk2d) (14).
Thrombosis associated with atherosclerotic lesions contributes to many of the complications associated with atherosclerosis. Similar to its ability to repress endothelial inflammation, KLF2 modulates anti-thrombotic transcription. KLF2 binds directly to the promoter of thombomodulin-1, thereby increasing transcription of this potent anti-thrombotic and anti-inflammatory factor (8, 15, 16). Additionally, KLF2 inhibits the effects of thrombin-mediated endothelial activation by preventing transcription of thrombin’s receptor, PAR-1 (17). In vivo, there is a clear association between KLF2 levels and vascular inflammatory disease. While complete knockout of KLF2 is embryonically lethal (18, 19), mice with hemizygous deletion of KLF2 (KLF2±) are viable. KLF2± mice crossed with ApoE−/− mice are more susceptible to atherosclerotic disease compared with KLF2+/+ApoE−/− mice (20). Additionally, post-natal deletion of KLF2 leads to a thrombotic phenotype, while globally overexpressing KLF2 protects mice from thrombus formation in part through the decreased expression of endothelial thrombotic genes (21).
Vascular inflammation is also a major player in the pathogenesis of diabetic vascular disease. Interestingly, hyperglycemia decreases endothelial KLF2 expression via FOXO1-dependent transcriptional silencing (22). Moreover, insulin induces KLF2 expression in ECs and KLF2 expression is reduced in the glomerulus of diabetic rats (23). Endothelial KLF2 is also implicated in vascular inflammation seen in neurodegenerative diseases such as Alzheimer’s disease. Amyloid beta plaques, a hallmark of Alzheimer’s, decrease KLF2 levels in cerebral ECs; and overexpression of KLF2 protects against amyloid-induced oxidative stress (24). These studies further demonstrate the protective nature of KLF2 during states of vascular inflammation, expanding the diversity of disease states that would potentially benefit from pharmacological targeting of KLF2.
Vessel hemodynamics strongly influence vascular inflammation and KLF2 is exquisitely sensitive to the biomechanical forces exerted by laminar versus turbulent shear stress. Under conditions of laminar shear stress (LSS), KLF2 is robustly expressed in ECs in vitro and in vivo (7, 11). Indeed, as recently confirmed by Dekker et al. in humans, KLF2 expression is focally lowered in areas of low LSS such as the bifurcation of the aorta to the iliac and carotid arteries, and this may be downstream of a dual specificity mitogen-activated protein kinase kinase 5(MEK5)/extracellular-signal-regulated kinase 5 (ERK5)/myocyte enhancer factor 2 (MEF2) pathway (7, 25, 26). It has long been known that atherosclerotic lesions have a predilection to form at regions experiencing low LSS, such as bifurcations of the vasculature. Within these so-called “atheroprone” regions, ECs are more likely to become activated and increase production of pro-inflammatory mediators (27, 28).
The extent of KLF2 expression and activity is highly regulated in ECs. As previously mentioned, LSS induces KLF2 expression. The signaling cascade behind this induction has been extensively characterized: In response to LSS, MEK5 is activated, which then phosphorylates ERK5. ERK5 subsequently phosphorylates MEF2 at the KLF2 promoter, leading to KLF2 gene transcription (7, 29). Conversely, KLF2 transcription can be inhibited by tumor necrosis factor alpha (TNF-α) signaling via p65 and histone deacetylase (HDAC) 4/5 inhibition of MEF2 (26). p53 also utilizes HDAC5-mediated KLF2 suppression to induce endothelial dysfunction (30). Interestingly, HDAC5 also regulates KLF2’s ability to induce transcription of eNOS, implicating HDACs as regulators of KLF2 function at multiple points (31, 32). Post-transcriptionally, endothelial KLF2 is targeted by microRNA-92a (miR-92a). Low-shear stress and oxidized LDL, factors both associated with atherogenesis, induce miR-92a expression (33, 34). miR-92a is then capable of binding to the 3′-UTR of KLF2, leading to its degradation (33, 35). In fact, targeting miR-92a in vivo using an antagomir leads to protection from atherosclerosis, providing a method to indirectly target KLF2 (33). Additional post-transcriptional regulation of KLF2 occurs through PI3K-dependent mRNA stabilization in response to LSS (36).
Krüppel-like factor 2 serves as a prototypical vasoprotective factor as it (1) is induced by EC activating stimuli, (2) resists harmful pro-inflammatory and pro-thrombotic gene transcription, and (3) is associated with protection against vascular inflammatory disease.
Krüppel-Like Factor 4
Krüppel-like factor 4 shares many transcriptional targets and protective functions with KLF2 in ECs. Like KLF2, KLF4 is induced during LSS (37, 38). Moreover, KLF4 expression is repressed under turbulent flow as a result of DNA methyltransferase-mediated methylation within the KLF4 promoter (39). The same mechanism also silences endothelial KLF3 under turbulent flow, an anti-inflammatory KLF that is less well characterized in ECs (40). Downstream transcriptional effects of KLF4 are similar to those seen in KLF2. For instance, a KLF4–p65 interaction inhibits VCAM-1 induction, reducing leukocyte homing (38, 41). KLF4 also regulates expression of eNOS. In multiple studies, overexpression or knockdown of EC KLF4 leads to increased or decreased eNOS production, respectively (38, 42, 43). KLF4 overexpression also increases transcription of anti-thrombotic factor thrombomodulin as well via a physical association with its cofactor, p. 300 (38). A novel and fascinating role of endothelial KLF4 was recently discovered in the context of endothelial inflammation and cholesterol flux. KLF4 induces the expression of cholesterol-25-hydroxylase (Ch25h) and liver X receptor (LXR) in ECs, which contribute to reverse cholesterol transport out of the vascular wall and inhibition of endothelial inflammasome activation, both protective against atherosclerosis (44).
In addition to its role in maintaining an anti-adhesive and anti-thrombotic endothelium, KLF4 also modulates intrinsic EC health. Autophagy is a conserved process by which cells recycle damaged organelles and misfolded proteins. Disrupted autophagy has been associated with multiple age-related phenotypes such as metabolic dysfunction, neurodegeneration, and cardiovascular disease [reviewed in Ref. (45)]. A recent study identified a role for endothelial KLF4 in regulating autophagic genes (46). Endothelial overexpression of Klf4 protected vessels from vascular aging, an effect that is likely largely due to enhanced autophagy (46). Interestingly, this study also demonstrated an inverse correlation between the age of vessels and KLF4 expression in humans.
The essential role of endothelial KLF4 in vascular health has been demonstrated in multiple in vivo models. Endothelial-specific knockout of Klf4 using a VE-Cadherin driven Cre-Lox system results in significantly enhanced atherosclerotic lesions when backcrossed onto the Apoe−/− mouse line (38). Additionally, EC-Klf4 KO exhibited increased thrombotic capacity. Conversely, endothelial-driven overexpression of Klf4 is protective against atherosclerosis. Conversely, endothelial-driven overexpression of Klf4 is protective against atherosclerosis and thrombosis (38). Outside of atherothrombotic disease, vascular inflammation can also negatively affect renal arteries during instances of ischemia. Endothelial KLF4 is vasoprotective in this context as demonstrated in a model of hematopoietic deletion of Klf4 during ischemia–reperfusion injury (47). Mice lacking KLF4 demonstrated exacerbated renal injury as a result of increased adhesion molecule expression on ECs with consequent immune cell invasion (47). This mechanism was also at play in a model of carotid artery injury. Interestingly, loss of endothelial KLF4 resulted in enhanced proliferation of both EC and neointimal VSMCs, as mediated by increased immune cell presence (41). In another disease model of pathological vascular remodeling, KLF4 levels are decreased in the lungs of patients with pulmonary artery hypertension (PAH) (42). Loss of endothelial KLF4 is associated with increased hypertension and pulmonary artery vascularization, in part through enhanced expression of endothelin-1 (ET-1) and decreased eNOS expression (42).
Like KLF2, KLF4 is post-transcriptionally regulated by miRs. Specifically, both KLF2 and KLF4 are inhibited by the “atheromiR,” miR-92a (33, 35). Additionally, however, KLF4 is negatively regulated by miR-103 (48). In mice with endothelial-specific deletion of miR processing machinery, Dicer, there is a decrease in miR-103-mediated KLF4 suppression; this increase in KLF4 subsequently restrains NF-κB-driven CXCL1 and macrophage infiltration in atherosclerotic lesions (48).
Endothelial KLF2 and KLF4 have remarkably similar functions; they respond to many of the same stimuli, share gene targets and have a high degree of similarity in amino acid sequence. Early studies on endothelial KLF4 function noted overlapping functions between the two phylogenetically close factors (37, 49). Indeed, loss of one allele of Klf2 leads to a compensatory increase in Klf4, while a single allele of either Klf2 or Klf4 is sufficient to rescue lethality in a double Klf2/Klf4 knockout mouse, suggesting genetic redundancy of functions central to endothelial function and identity (20, 50). Indeed, the double Klf2/Klf4 knockout mouse demonstrates loss of endothelial integrity and hemostatic dysfunction, as well as the loss of an endothelial-like transcriptome.
Krüppel-Like Factors 5 and 6
Unlike KLF2 and 4, endothelial KLF5 and 6 are associated with vascular inflammation and remodeling that is largely deleterious. While KLF5 is largely considered to be a major effector of VSMC function (see below), there is evidence that it mediates endothelial chemotactic function. Specifically, knockdown of endothelial KLF5 in vitro reduces TNF-α-induced expression of key monocyte chemoattractant protein, MCP-1 (51). While the in vivo implications of this phenomenon are unclear, there is ample evidence implicating MCP-1 in many forms of vascular inflammation including atherogenesis, diabetic vascular disease, and vascular occlusion (52–54).
Largely implicated in cancer biology, KLF6 also has documented roles in ECs. KLF6 is an early response factor to vascular injury that induces transcription and processing of the pro-angiogenic factor endoglin, a member of the TGF-β receptor superfamily member (55). Mechanistically, KLF6 interacts with related transcription factor specificity protein 1 (Sp1) to bind to the endoglin promoter (56). While endoglin’s role in angiogenesis has been extensively characterized, it is also implicated in leukocyte trafficking during vascular inflammation (57). Endothelial KLF6, therefore, may promote immune cell infiltration during vascular injury. In addition to regulating endoglin, KLF6 also induces expression of activin receptor-like kinase 1 (ALK1), another member of the TGF-β receptor family (58). KLF6–Sp1 interactions mediate Alk1 transcription during endothelial denudation, and KLF6 heterozygotes exhibit reduced neointimal formation in response to vascular injury, a mechanism that is proposed to be through reduced ALK1 levels (58). While additional studies regarding endothelial KLF5 and 6 need to be completed, both factors seem to promote vascular inflammation and remodeling in response to injury.
Krüppel-Like Factor 11
Krüppel-like factor 11 is also highly expressed in ECs and is involved in regulating vascular inflammation. While Klf2 expression is ultimately inhibited by TNF-α, KLF11 is induced as a result of inflammation, and similar to interactions seen with endothelial KLF4, KLF11 binds to p65 to inhibit transcription of NF-κB target genes such as VCAM-1 and E-selectin resulting in less leukocyte adhesion to ECs during vascular inflammation (59). This allows KLF11 to serve as an inflammatory-responsive factor to reduce excessive endothelial activation. In fact, loss of KLF11 in a model of cerebral ischemia results in enhanced inflammation and worse outcome (60). Endothelial KLF11 is regulated, in part, by the peroxisome proliferator-activated receptor (PPAR) family of nuclear receptor proteins, which includes three mammalian isoforms: α, β, and γ. Administration of a PPARγ agoinst leads to increased KLF11 expression in cerebral vascular ECs (61). Interestingly, KLF11 also serves as a coregulator of PPARγ target genes via physical interaction of the two proteins at PPAR responsive elements (61). This interaction results in repression of pro-apoptotic miR-15a, increasing EC survival and conferring protection against cerebrovascular ischemia. These two studies demonstrate a role of endothelial KLF11 in regulating vascular inflammation during ischemic events. In addition to being responsive to PPARγ, Klf11 transcription is also induced by PPARα (62). Administration of PPARα agonist causes KLF11 induction and its subsequent binding and inhibition of the ET-1 promoter. This phenomenon further bolsters KLF11’s role as a vasoprotective factor through its ability to inhibit endothelial inflammation and vasoconstriction.
VSMC KLFs
Vascular smooth muscle cells, along with collagen and elastin, form the medial layer of blood vessels and regulate vasomotor tone to maintain proper hemodynamic pressure throughout the vascular system. In the absence of noxious stimuli, VSMCs express numerous mature markers including smooth muscle α-actin, SM22α, and smoothelin (63). When challenged by growth factors, inflammation, or injury, VSMCs undergo phenotypic switching: cells dedifferentiate, losing mature VSMC markers and regaining the ability to proliferate, migrate, and synthesize extracellular matrix proteins. This can lead to pathological vessel remodeling, ultimately resulting in obstruction of proper blood flow. Multiple KLFs modulate VSMC phenotype switching in the face of vascular injury and inflammation (Figure 2).
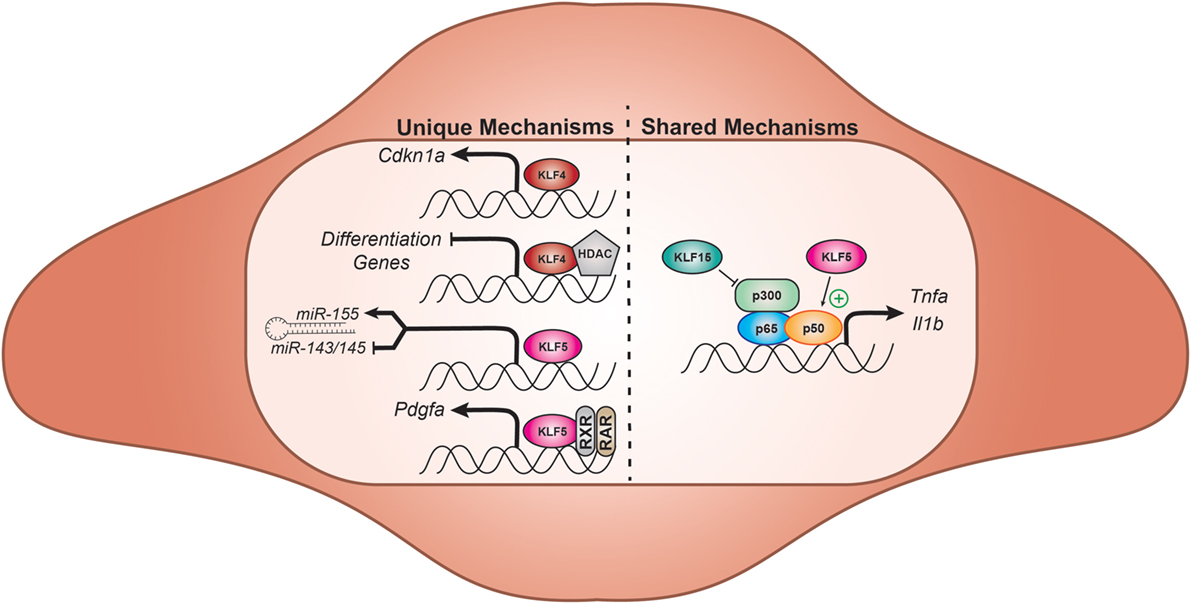
Figure 2. Select effector functions of vascular smooth muscle KLFs. KLFs differentially affect VSMC differentiation, proliferation, and inflammation through a multitude of mechanisms. KLF5 and 15 exhibit reciprocal regulation of nuclear factor-κB signaling within VSMCs by binding to cofactors p50 and p300, respectively. KLF5 also promotes proliferation and dedifferentiation by modulating important driver of VSMC dedifferentiation, platelet-derived growth factor-A (PDGF-A), and differentially regulating miR transcription. While KLF4 also promotes dedifferentiation, it resists VSMC proliferation through the transcription of CDKN1A. KLF, Krüppel-like factor; VSMC, vascular smooth muscle cell; HDAC, histone deacetylase; miR, microRNA; TNF-α, tumor necrosis factor alpha; IL-1β, interleukin-1 beta; RXR, retinoid X receptor; RAR, retinoid acid receptor; Cdkn1a, cyclin-dependent kinase inhibitor 1a.
Krüppel-Like Factor 4
Vascular smooth muscle cell KLF4 maintains cells in a dormant state by binding to and recruiting the potent anti-proliferative protein p53 to the p21WAF1/Cip1 (Cdkn1a) promoter/enhancer, consequently increasing transcription of Cdkn1a, a cell cycle inhibitor (64, 65). Further, viral overexpression of KLF4 in both VSMC and balloon-injured rat carotid arteries leads to increased expression of a panel of anti-proliferative genes such as p57 and GADD45β (66). In vivo implications of KLF4’s anti-proliferative effects are seen in a carotid artery ligation model using conditional KLF4 knockout: KLF4 KO mice demonstrate enhanced neointimal formation as a result of dysregulated VSMC proliferation (64).
Given VSMC KLF4’s role in suppressing proliferation, KLF4 also plays a somewhat counterintuitive role in promoting dedifferentiation of VSMCs. KLF4 coordinates multiple molecular events to repress markers of VSMC maturity in the context of pathological vessel remodeling. KLF4 binds directly to the TGF-β control element (TCE) to inhibit transcription of smooth muscle α-actin and SM22α (64, 67). Moreover, KLF4 recruits inhibitors of mature VSMC marker transcription such as ELK-1 and HDACs to TCEs (68). Recent evidence indicates that VSMC dedifferentiation during vascular injury not only decreases markers of mature VSMCs, but also causes VSMCs to express markers associated with macrophages, myofibroblasts, and mesenchymal stem cells. Utilizing elegant lineage tracing experiments, Shankman et al. showed that KLF4 is necessary for VSMCs to gain genetic characteristics of other cell types within atherosclerotic lesions (69). Evidence for this transition from VSMC to a “macrophage-like” cell type was recapitulated in vitro using cholesterol loaded Klf4-sufficient VSMCs, an effect that was lost in Klf4 mutant cells (69). These studies demonstrate KLF4’s importance in VSMC phenotype switching during pathological remodeling. Modulating KLF4 expression may provide an important therapeutic avenue to adjust VSMC phenotype and disease progression.
Mature, uninjured VSMCs express very low levels of KLF4 both in vitro and in vivo (67, 70); however, KLF4 expression is significantly increased in injured VSMCs, contributing to subsequent dedifferentiation. Oxidized phospholipids, which are associated with atherosclerotic burden, induce both KLF4 mRNA transcription and KLF4 nuclear translocation in VSMCs (71). Additionally, carotid artery ligation-induced vascular injury leads to a swift increase in VSMC KLF4 expression that is associated with repression of smooth muscle α-actin (64). Mechanistically, this occurs through the reduction of miR-143/145, which normally inhibit KLF4 expression to promote VSMC maturation (72). Cigarette smoke is another well-characterized stimulant of vascular inflammation. Cigarette smoke extract has been shown to induce Klf4 transcription, enhance KLF4 binding at the promoters of VSMC differentiation genes, and increase KLF4-driven epigenetic changes that are associated with transcriptional repression (73).
Krüppel-Like Factor 5
Krüppel-like factor 5 modulates processes that engender pathological remodeling of the vasculature: like KLF4, KLF5 promotes VSMC dedifferentiation; however, dissimilarly, KLF5 also promotes cellular proliferation. KLF5’s importance in phenotype switching in the face of vascular injury has been shown both in vitro and in vivo. Overexpression of KLF5 leads to reduced expression of VSMC maturity markers myocardin and smooth muscle α-actin while the converse occurs with KLF5 knockdown (74). Concordantly, in vivo experiments show that in wild-type mice, vascular injury causes a decrease in smooth muscle α-actin and smooth muscle myosin heavy chain (MHC), whereas this effect is not seen in mice heterozygous for KLF5 (75).
Krüppel-like factor 5’s exerts its pro-inflammatory effects through two main mechanisms: complex formation with unliganded retinoid acid receptor (RAR)/retinoid X receptor (RXR) and recruitment of NF-κB subunit p50. KLF5-RAR/RXR complex binds to the promoter of platelet-derived growth factor-A (PDGF-A), a potent inducer of VSMC proliferation and dedifferentiation (75, 76). Administration of a synthetic retinoid abolishes KLF5-RAR/RXR interaction, resulting in decreased KLF5 transcriptional activity; whereas, administration of an RAR antagonist stabilizes KLF5 transcriptional effects (75, 77). Furthermore, this mechanism has been shown in vivo to govern VSMC proliferation in times of injury. Both pharmacological KLF5 inhibition via the administration of an RAR agonist and genetic loss of one Klf5 allele diminishes neointimal formation and vascular remodeling in models of AngII infusion or femoral artery cuff injury, indicating a critical role of KLF5 in VSMC proliferation in response to injury (77). In addition to KLF5-RAR/RXR complex formation, KLF5 recruits p50 to influence VSMC phenotype toward proliferation and dedifferentiation, as well as augment VSMC inflammatory transcription (78, 79). In VSMCs from diabetic patients, increased KLF5 and inducible nitric oxide synthase expression leads to augmented nitrated-KLF5, which possesses a heightened ability to interact with p50 and subsequently enhance TNF-α and interleukin-1 beta (IL-1β) expression (79). Interestingly, estradiol competes with KLF5 for p50 binding and can inhibit KLF5-p50-mediated transcription of inflammatory genes (79).
Krüppel-like factor 5 has been found to be upregulated in both human lung biopsies and isolated human pulmonary artery smooth muscle cells from patients with PAH, a vascular remodeling disease process (80). Using a hypoxic PAH model, Li et al. demonstrated that KLF5 serves as an upstream regulator of hypoxia inducible factor 1-alpha activity (81). Loss of KLF5 abrogated hypoxia-induced vascular remodeling partly through upregulating proliferation factors (e.g., cyclin B1 and D1) and downregulating apoptosis factors (e.g., bax, bcl-2, cleaved caspase-3, and cleaved caspase-9) (80, 81). A similar effect is seen in cardiomyocytes in response to ischemia/reperfusion injury, further emphasizing a conserved role of KLF5 in promoting proliferation and cell survival (82).
Similar to other KLFs, VSMC KLF5 both regulates and is regulated by the transcription of miRs. miR-145 is highly expressed in differentiated VSMCs and is important in maintaining cellular differentiation: expression of miR-145 is associated with upregulation of smooth muscle α-actin, calponin, and smooth muscle-MHC and reciprocal downregulation of KLF5 (72, 74, 83). In injured vessels such as those seen in atherosclerotic lesions, however, expression of miR-145 is downregulated within VSMCs (74, 84). In the absence of vascular injury, miR-145 directly targets the 3′-UTR of KLF5 to inhibit it. Following injury, PDGF inhibits miR-145 expression, thus attenuating KLF5 degradation and consequently suppressing transcription of differentiation markers (see above) and myocardin, a modulator of differentiation genes (74). In addition to being regulated by miRs, KLF5 also controls the expression of the pro-inflammatory miR-155. When VSMCs are exposed to oxLDL, KLF5 is induced, resulting in decreased anti-inflammatory miRs-143/145 and increased miR-155 (85). Interestingly, KLF5-mediated miR-155 production leads to secreted miR-155 in exosomes that are capable of destroying endothelial tight junctions and enhancing atherosclerotic progression (85).
Krüppel-Like Factor 15
Krüppel-like factor 15 serves largely as a protective factor in many aspects of cardiovascular biology including inhibiting cardiomyocyte hypertrophy, regulating cardiac lipid metabolism, and establishing circadian control of ventricular rhythm (86–88).
Vascular smooth muscle cell KLF15 primarily acts as a protective factor against vascular inflammation and disease by resisting VSMC proliferation and inflammation. Similar to other KLFs, VSMC KLF15 interacts with the histone acetylase, p300. Direct binding to p300 prevents acetylation of NF-κB member p65, thus limiting transcription of NF-κB target genes and inflammation. Both rat aortic VSMCs exposed to oxidized phospholipids and human atherosclerotic tissue demonstrate markedly decreased KLF15 expression, suggesting that KLF15 plays an important role in atherogenesis (89). Orthotopic carotid artery transplantation from Klf15−/− mice into Apoe−/− mice results in significantly enhanced intimal hyperplasia and inflammatory cell infiltrate. Additionally, these VSMCs express higher levels of inflammatory proteins such as VCAM-1, MCP-1, and MMP3. These results have been recapitulated in smooth muscle-specific deletion of Klf15 on the Apoe−/− background. When rat aortic smooth muscle cells are exposed to PDGF-BB, a stimulator of VSMC proliferation and migration, Klf15 mRNA expression is reduced (90). Interestingly, in KLF15-deficient VSMCs, Pdgf transcription is enhanced, demonstrating a feed-forward loop that permits VSMC proliferation and inflammation by decreasing KLF15 levels (89). In humans and mice, KLF15 deficiency is associated with cardiomyopathy and aortic aneurysm (91). Additionally, humans with ruptured intracranial aneurysms exhibit diminished KLF15 expression while expressing elevated levels of inflammatory genes (92).
Monocyte/Macrophage KLFs
In addition to cells of the vessels themselves, circulating immune cells and their infiltration into the vascular wall are paramount to the initiation and propagation of vascular inflammation. There is ample research implicating both innate and adaptive immune cells in the progression of atherosclerosis [reviewed in Ref. (93, 94), respectively]. Comparable with their role in vascular cells, KLFs have divergent functions in myeloid cell-derived inflammation, capable of either repressing or promoting inflammatory processes (Figure 3).
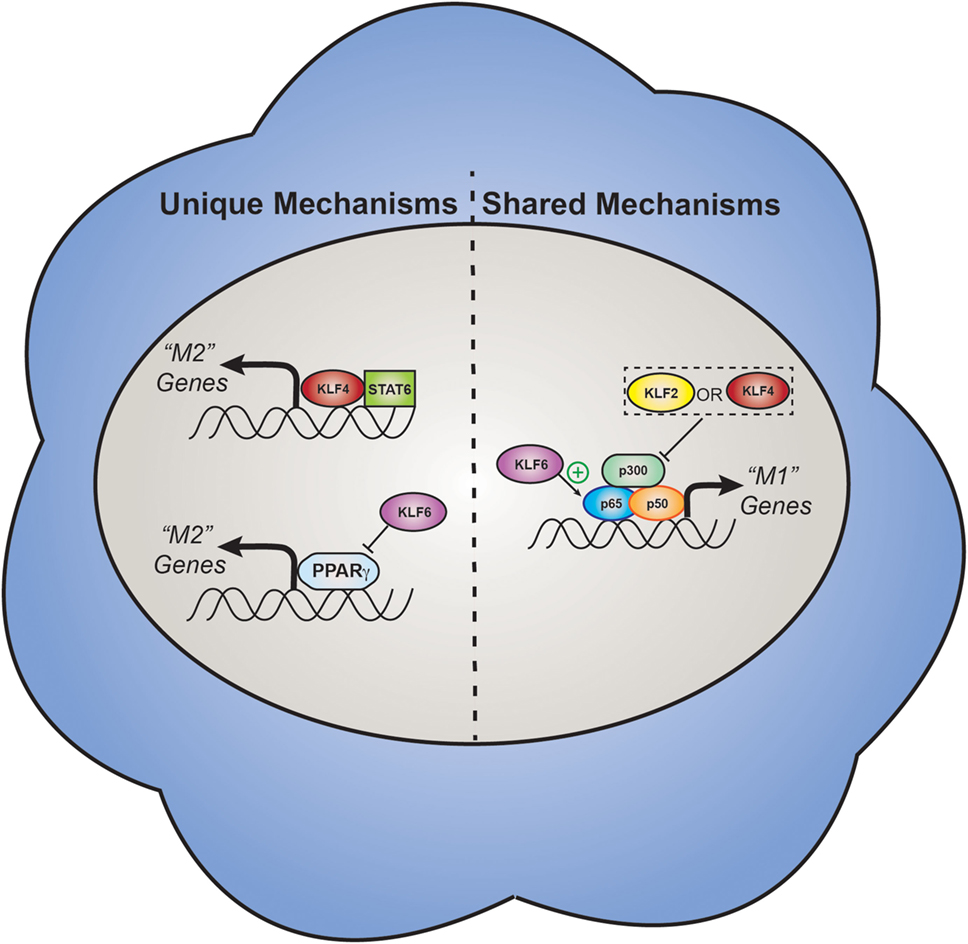
Figure 3. Select effector functions of myeloid KLFs. Myeloid KLFs affect polarization of cells to a pro-inflammatory (“M1”) or anti-inflammatory (“M2”) state. KLF2 and 4 inhibit M1 polarization by negatively regulating nuclear factor (NF)-κB-mediated transcription. Additionally, KLF4 promotes M2 polarization through its interactions with STAT6, a prominent transcription factor in M2 skewing. KLF6 promotes M1 polarization through cooperation with NF-κB cofactor p65 and through its inhibition of PPARγ-mediated M2 transcription. KLF, Krüppel-like factor; miR, microRNA; PPARγ, peroxisome proliferator-activated receptor gamma; M1, pro-inflammatory classical macrophage activation; M2, anti-inflammatory alternative macrophage activation; STAT6, signal transducer and activator of transcription 6.
Krüppel-Like Factor 2
Originally studied in the context of acute inflammation and bacterial sepsis, KLF2 is a central regulator of monocyte inflammatory activation (95, 96). KLF2 resists inflammation within macrophages via recruitment of NF-κB cofactors away from the promoters of inflammatory genes. Among these factors bound by KLF2 are p300 and p300/CBP-associated factor (PCAF) (95, 97, 98). Given the inflammatory potential of KLF2 knockout macrophages, one would predict that loss of myeloid KLF2 would be associated with increased vascular inflammation and atherosclerosis. This is, indeed, the case as mice with myeloid-specific KLF2 deletion on the Apoe−/− background exhibit increased atherosclerosis with increased vascular oxidative stress (99). This is the result of increased adhesive potential of KLF2 knockout neutrophils and macrophages. Interestingly, a similar effect is seen with dendritic cell (DC)-specific KLF2 knockout. Loss of KLF2 in DCs aggravates atherosclerosis as a result of enhanced T-cell activation and heightened inflammatory cytokine production (100). Together, these studies demonstrate a central role of KLF2 in maintaining quiescence in circulating myeloid cells; a role it serves in ECs as well.
Krüppel-like factor 2 is itself controlled by atherogenic stimuli. When anti-inflammatory macrophages are challenged with oxLDL, they shift to a pro-inflammatory state via the downregulation of KLF2 (101). There is also a link between low-immune cell KLF2 levels with increased risk of cardiovascular disease in humans. Monocytes from patients with atherosclerosis exhibit less Klf2 expression than healthy controls, indicating that the inflammatory state associated with low-KLF2 translates to atherosclerotic disease (98).
Krüppel-Like Factor 4
Just as KLF2 and KLF4 have overlapping functions in ECs, this is also the case in monocytes/macrophages. While KLF2 regulates inflammatory activation of monocytes, KLF4 regulates macrophage polarization from the pro-inflammatory (“M1”) state to the anti-inflammatory (“M2”) state (102). Like KLF2, KLF4 recruits p300 and PCAF away from the promoter of inflammatory genes, resisting the M1 polarization state. Complementarily, KLF4 promotes the M2 state by cooperating with critical M2 transcription factor STAT6 to induce transcription of traditional M2 genes through induction of MCP-1-induced protein (102, 103). While STAT6 is largely responsible for anti-inflammatory polarization in macrophages, CREB is another transcription factor that limits resists inflammation (104). In addition to interacting with STAT6 to modulate anti-inflammatory transcription, KLF4 also interacts with CREB to increase transcription at the apoE promoter in macrophages, ultimately resulting in atheroprotection (105–107). Myeloid KLF4, therefore, resists inflammation and is largely a protective factor against vascular inflammation. Moreover, loss of myeloid KLF4 is associated with augmented atherosclerosis, and macrophages deficient in KLF4 display increase inflammation in response to oxidized phospholipids (108). While KLF4-mediated Ch25h and LXR expression drives reverse cholesterol transport in ECs and macrophages, there is also evidence implicating Ch25h and LXR in KLF4-mediated M2 polarization (44). It is evident that KLF4 regulation of macrophage polarization and its role in preventing vascular inflammation is exceedingly complex and likely involves multiple downstream regulators.
Krüppel-Like Factors 5 and 6
The increased inflammatory drive associated with endothelial KLF5 and 6 is paralleled by that of macrophage KLF5 and 6. Overexpression of KLF5 increases the ability of macrophages to migrate and proliferate (109). This contributes to worsened intimal hyperplasia following carotid ligation in KLF5 overexpressing mice. This is in contrast to the protective effect afforded by myeloid-specific KLF5 deletion. Interestingly, pro-inflammatory stimuli stabilize KLF5 protein via various post-translational modifications. TNF-α increases KLF5 sumoylation and decreases ubiquitination to stabilize the protein and prevent degradation (109, 110). KLF5’s responsiveness to inflammatory stimuli, along with its ability to propagate macrophage-mediated inflammation, contributes to its deleterious role in vascular inflammation.
Krüppel-like factor 6 expression is also responsive to pro-inflammatory stimuli. KLF6 increases when macrophages are stimulated with M1-driving stimuli and decreases with M2-driving stimuli (111). Additionally, KLF6 impacts both M1 and M2 gene transcription. KLF6 is required for optimal binding of p65 binding to its promoters, and importantly, through its interaction with p65, KLF6 promotes transcription of NF-κB targets (112). Additionally, KLF6 suppresses B cell lymphoma 6 expression, which leads to increased pro-inflammatory gene expression and increased macrophage motility (113). Conversely, KLF6 binds to PPARγ and prevents it from inducing M2 gene transcription (111). It is evident that KLF6 is a dynamic regulator of macrophage polarization.
Krüppel-Like Factor 14
Within the past 5 years, KLF14 has been extensively studied in its role in lipid and cholesterol metabolism. Given that aberrant nutrient handling, obesity, and type 2 diabetes are risk factors for atherosclerosis, it is unsurprising that multiple genetic variants involving the KLF14 gene have been implicated in the development of atherosclerotic disease. While the genetic associations of KLF14 variants on metabolic disease have been extensively studied (114), the role of KLF14 in macrophages is less well characterized. Recent work by Wei and colleagues has begun to parse out details on how KLF14 contributes to atherogenesis. They found that Apoe−/− mice aortas had elevated levels of Klf14 on either high-fat diet or standard chow (115). This increase in Klf14 expression was associated with elevated pro-inflammatory cytokines in circulation: Klf14 adenoviral knockdown ameliorated this effect. Importantly, overexpression of Klf14 in a macrophage cell line led to increased inflammatory cytokine production as well as total cholesterol and cholesteryl ester content, a classic signature of atherogenic foam cells (116). Mechanistically, KLF14-mediated inflammation seems to be dependent on p38 MAPK and ERK1/2 signaling leading to increased cytokine release (115). Together, this work provides evidence that KLF14 may play a causal role in modulating inflammation associated with atherosclerosis, further implicating it in metabolic disease.
KLFs in Other Circulating Immune Cells
In addition to regulating differentiation, activation, and polarization of monocytes, KLFs also shape lymphocyte and DC function. While there is a paucity of studies investigating KLF-driven lymphocyte processes in vascular inflammation, there is extensive evidence demonstrating the importance of KLFs in lymphocyte biology that can be extrapolated to the context of vascular disease (Figure 4).
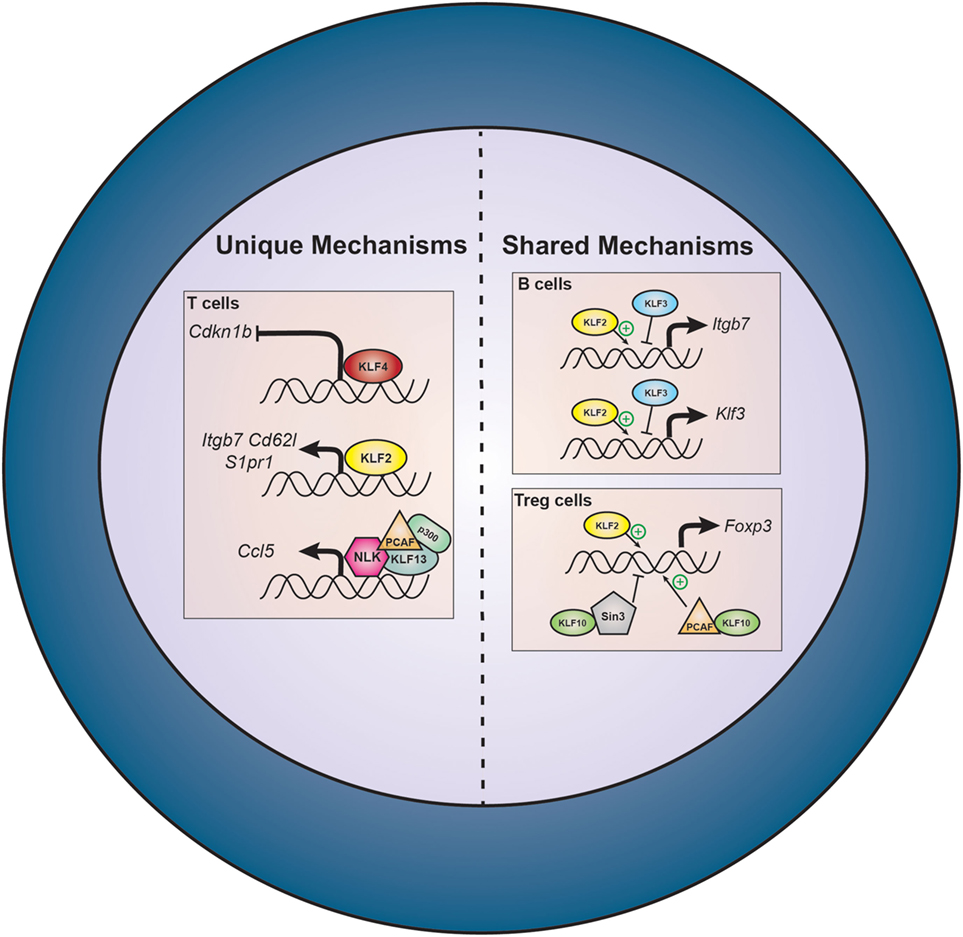
Figure 4. Select effector functions of lymphocyte KLFs. Lymphocyte KLFs largely dictate cellular identity, proliferation, and survival. KLF2 and KLF3 oppose each other’s function at the Itgb7 and Klf3 promoters. In regulatory T cells (Treg), corepressor/coactivator interactions determine the transcriptional effects of KLF10. KLF, Krüppel-like factor; Itgb7, β7 integrin; Foxp3, forkhead box P3; Sin3, represents Sin3-histone deacetylase complex (Sin3-HDAC); PCAF, P300/CBP-associated factor; Cdkn1b, cyclin-dependent kinase inhibitor 1b (p27Kip1); Cd62l, cluster of differentiation 62 ligand (L-selectin); S1pr1, sphingosine-1-phosphate receptor 1 (S1P1); Ccl5, chemokine ligand 5 (RANTES); NLK, Nemo-like kinase.
Krüppel-Like Factor 2
Like in monocytes, KLF2 expression maintains T cells in a quiescent state. KLF2 is expressed in naïve, effector, and memory T cells (117, 118) and its loss causes single-positive, resting T cells to spontaneously activate and apoptose in the spleen and lymph nodes (18, 19). In CD8+ T lymphocytes, KLF2 levels decrease upon stimulation of T-cell receptors and its expression is reestablished after treatment with IL-2 or IL-7 (118). In contrast, CD4+ T lymphocytes demonstrate a transient increase in KLF2 expression upon stimulation that is associated with increased IL-2 production (119). KLF2 is essential in the expression of T-cell migration factors such as S1P1, cluster of differentiation 62 ligand (L-selectin) (CD62L), and β7 integrin (Itgb7), allowing T cells to traffic to sites of vascular inflammation or draining lymph nodes (120, 121). Furthermore, statin-induced KLF2 expression in effector T cells reduces inflammation in a myocarditis model, an effect that is likely related to diminished interferon-γ production (122).
In addition to the proatherogenic functions of effector T cells, regulatory T cells (Tregs) play an important role in suppressing vascular inflammation (123). In the presence of oxLDL, Tregs restore endothelial KLF2 to protect the vasculature from inflammation (124). Within Tregs, forkhead box P3 (FoxP3), a lineage-specific transcription factor, is under direct control of KLF2 (125). Loss of KLF2 prior to FoxP3 induction results in impaired Tregs differentiation, while loss of KLF2 after FoxP3 induction does not affect this process. Pabbisetty et al. also demonstrated that stabilization of KLF2 protein through statin administration or by genetic deficiency of E3 ubiquitin ligase SMURF1 results in enhanced Treg production.
Within B lymphocytes, KLF2 appears to be important in determining cellular identity. Higher KLF2 expression is associated with B1 B cells in the periphery versus follicular or marginal zone B cells. Concordantly, inactivation of KLF2 in B cells leads to a decrease in B1 B cells with a concurrent increase in marginal zone B cells (126, 127). Similar to T cells, loss of KLF2 in B cells is also associated with less CD62L and Itgb7 expression, resulting in impaired B-cell trafficking (126, 128). Finally, KLF2 also plays a role in regulating the DC response during vascular inflammation. As is seen with monocytes and neutrophils (99), loss of KLF2 in DCs increases inflammatory cytokine production, DC tissue infiltration, and T-cell activation in atherogenic Ldlr−/− mice (100). Together, these studies further demonstrate that KLF2 largely opposes inflammatory activation in circulating immune cells.
Krüppel-Like Factor 3
Within B cells, KLF2 and KLF3 have opposing effects and compete for the same gene targets. While KLF2 is associated with a B1 B-cell differentiation pattern (with lower levels associated with follicular and marginal zone cells), KLF3 expression favors marginal zone B-cell development (129). Additionally, KLF2 and KLF3 compete for occupancy of the Itgb7 promoter: while KLF2 promotes expression of Itgb7 and, thus, migration, KLF3 leads to downregulation of Itgb7 and impaired homing ability of lymphocytes (130). Interestingly, KLF2 and 3 differentially regulate KLF3 expression itself. KLF3 negatively regulates its own expression through direct binding to the KLF3 promoter (130). Conversely, loss of KLF2 in B cells results in decreased expression of KLF3 (i.e., KLF2 increases KLF3 expression) (128). The interplay between these two factors is critical for B-cell differentiation and function.
Krüppel-Like Factor 4
Given its well-defined role in maintaining self-renewing capabilities of stem cells, it is unsurprising that loss of KLF4 expression is necessary for proper T-cell development. Remarkably, KLF4 is the only Yamanaka factor that is downregulated throughout each step of T-cell differentiation (131). This attenuation is required for the transition from double negative (DN)2 to DN3 as evidenced by diminished T-cell differentiation at this stage during forced KLF4 overexpression (131). While loss of KLF4 is critical to T-cell differentiation, DN T-cell population proliferation is maintained through KLF4 activity: KLF4 binds to and inhibits the promoter of cyclin-dependent kinase inhibitor 1b/p27Kip1, releasing inhibition of CDK-mediated proliferation (132). Interestingly, this is contrary to how KLF4 interacts with p27Kip1 in VSMCs [(66); see below], demonstrating cell-type specific functions of KLF4 in regulating proliferation. Within B cells, KLF4 is lowly expressed in the most immature stages but is increased throughout B-cell maturation (133). Upon activation, however, mature B cells decrease KLF4 levels. Additionally, KLF4 appears to be important in promoting B-cell proliferation through the activation of cyclin D2 (133). KLF4’s role in DC biology closely mirrors that seen in monocyte/macrophages. IRF4-expressing DCs are important in promoting type 2 helper T-cell (Th2) response, and KLF4 is required for this interaction (134). Additionally, loss of KLF4 in pre-DCs leads to fewer IRF4-expressing DCs. Together, the DC and monocyte data suggests that KLF4 strongly favors the “anti-inflammatory” polarization of immune cells and its expression may be a potential target to reduce deleterious vascular inflammation.
Krüppel-Like Factor 10
Krüppel-like factor 10 plays an important role in establishing Treg identity through FoxP3 expression while also directly promoting Treg function through TGFβ1 production. Indeed, forced overexpression of KLF10 in CD4+ CD25− (non-Treg) T cells induces both Foxp3 and Tgfb1 expression while downregulating markers of Th1 and Th2 cells (Tbet and Gata3, respectively) (135). Conversely, loss of KLF10 in CD4+ CD25− cells enhances Th1 and Th2 differentiation. In response to Treg stimulating factor TGFβ1, KLF10 transactivates both FoxP3 and TGFβ1 promoters, representing a positive feedback loop of Treg function (135). Important in vascular inflammation, the addition of KLF10 knockout CD4+ CD25− T cells promoted atherosclerosis in ApoE−/−/scid/scid mice via increased leukocyte accumulation and inflammatory cytokine production (135). Recent studies have provided mechanistic insight on how KLF10 regulates FoxP3 transcription. Within Tregs, KLF10 recruits PCAF to the FoxP3 promoter, leading to acetylation and subsequent activation of the FoxP3 promoter (136). Remarkably, KLF10 also associates with the corepressor Sin3-HDAC to repress FoxP3 transcription. A study by Xiong et al. demonstrated that PCAF disrupts KLF10/Sin3 interactions to allow PCAF-mediated FoxP3 acetylation through its interaction with KLF10 (137). The authors of this study posit that KLF10 interacts with Sin3-HDAC in the dominant state while post-translational modifications in KLF10 downstream of lymphocyte signaling is required to favor PCAF/KLF10 interactions. Along these lines, KLF10 interaction with the FoxP3 promoter appear to be dependent on Itch-mediated ubiquitination of KLF10 in a degradation-independent manner (138). While this study did not investigate how ubiquitination of KLF10 affects PCAF or Sin3 interactions, ubiquitination of KLF10 promoted FoxP3 expression suggesting that this mechanism may contribute to KLF10’s interaction with PCAF.
Krüppel-Like Factor 13
Krüppel-like factor 13 also demonstrates complex interactions with acetyl transferases/deacetylases to regulate activation of T cells. RANTES (or chemokine ligand 5) is a classically expressed gene late in T-cell activation whose blockade is associated with diminished atherosclerosis (139). KLF13 promotes RANTES expression through the recruitment of a “enhancesome” that consists of various kinases and acetyltransferases. Specifically, Nemo-like kinase is recruited to phosphorylate the H3 histone on the RANTES promoter. Following this, PCAF and CBP/p300 are recruited to acetylate H3 and allow for ATP-dependent chromatin remodeling and RNA Polymerase II binding (140, 141). In addition to promoting RANTES expression in T cells, KLF13 also promotes apoptosis by binding to the promoter of anti-apoptotic factor BCL-XL and reducing its expression (142). The authors of this study suggest that KLF13-mediated repression of BCL-XL occurs through the recruitment of Sin3-HDAC to the promoter, as is seen in the context of other genes inhibited by KLF13 (143).
Pharmacological Modulation of KLFs
Current therapies for atherosclerosis largely target mechanisms known to activate vascular inflammatory cascades such as dyslipidemia (statins), disturbed flow (anti-hypertensives), and activated circulating inflammatory cells (aspirin). Given the importance of these stimuli in the pathogenesis of atherosclerosis and thrombosis, understanding molecular mediators of vascular inflammation is imperative in developing novel agents against cardiovascular disease.
While accomplishing specificity in targeting KLFs will likely be difficult, multiple compounds act upstream of KLFs, thereby modulating their expression and function (Figure 5). Below, we summarize a few modulators of KLF biology, with special emphasis on those that affect multiple different KLFs important in vascular inflammation.
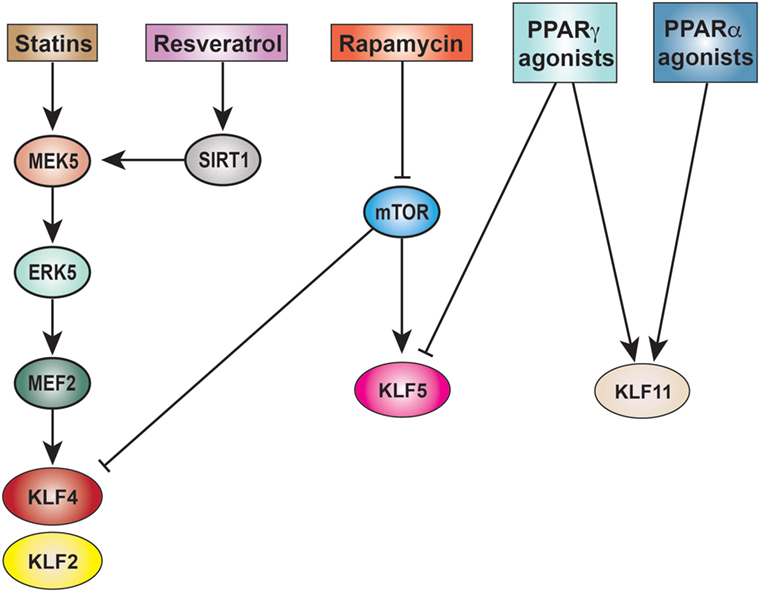
Figure 5. Pharmacological targeting of KLFs in vascular inflammation. There is substantial crossover in the mechanisms of drugs targeting the induction/inhibition of KLFs in vascular inflammation. Both statins and resveratrol augment KLF2 through MEF2-mediated transcription, the same pathway used in statin-mediated KLF4 induction. Rapamycin has differential effects on KLF4 and KLF5 expression, eventually stimulating KLF4 production while inhibiting KLF5. Inhibition of KLF5 is also achieved through use of PPARγ agonists (e.g., rosiglitazone). KLF11 is induced through PPAR signaling, exhibiting increased expression in response to agonists to both PPARγ and PPARα (e.g., fenofibrate). KLF, Krüppel-like factor; MEK5, dual specificity mitogen-activated protein kinase5; ERK5, extracellular-signal-regulated kinase 5; MEF2, myocyte enhancer factor 2; SIRT1, sirtuin 1; mTOR, mammalian target of rapamycin; PPAR, peroxisome proliferator-activated receptor.
Krüppel-Like Factor 2
Numerous pharmacological agents induce KLF2. Notably, the prominent lipid-lowering statins are potent inducers of KLF2 expression in ECs and circulating immune cells via MEF2 (144–146). Studies in mouse have demonstrated a potential role for statin-induced KLF2 expression in protecting against diabetic vascular reactivity and inflammation, as well as myocarditis (122, 147). These studies indicate the widespread anti-inflammatory properties of statins through the modulation of KLF2. In addition to statins, phenol compounds such as tannic acid and resveratrol, are capable of inducing endothelial KLF2 and preventing inflammation (148, 149). Acting via sirtuin 1 and MEK5/MEF2-dependent mechanisms, resveratrol induces the expression of KLF2-dependent atheroprotective genes (149). While the benefits of chronic resveratrol therapy in humans are still under investigation, it has been attributed with increasing lifespan and the prevention of multiple age-related diseases in small mammals [(150, 151)]. Additional work needs to be done, however, to determine the relative contribution of KLF modulation in resveratrol’s protective qualities.
Therapeutic proteasome inhibitor Bortezomib has also been demonstrated to induce KLF2 in multiple cell types (21). Normally prescribed to combat multiple myeloma, Bortezomib treatment at non-myelosuppresive doses is actually thromboprotective, in part, through KLF2 induction (21).
Krüppel-Like Factor 4
As with KLF2, KLF4 expression is induced by statin use. Utilizing a MEK5/ERK5 axis, statin-induced KLF4 expression leads to increased transcription of genes associated with anti-thrombosis, vasodilation, and hemostasis while increasing apoptosis resistance and decreasing inflammatory potential in ECs (43). Additionally, in a model of renal ischemia–reperfusion injury, statins protected against injury in a KLF4-dependent manner (47). Given their widespread use in patients at risk for cardiovascular disease, statins represent a tool to further understand the importance of KLFs in regulating vascular inflammation in humans.
Vascular smooth muscle cell KLF4 is also induced by multiple pharmacological agents including rapamycin and cyclosporine A (CSA). Rapamycin is a known inhibitor of cell proliferation via induction of p27kip1 (152) and has long been used in drug-eluting stents to prevent restenosis via VSMC proliferation (153). Within VSMCs, rapamycin inhibits mammalian target of rapamycin (mTOR), which subsequently increases KLF4 production (66). Interestingly, overexpression of KLF4 results in increased p27kip1 production and inhibition of VSMC proliferation. These results suggest that rapamycin and VSMC KLF4 enhance each other’s activities in the regulation of VSMC proliferation. CSA is an immunosuppressant used in inhibiting lymphocyte proliferation that upregulates VSMC KLF4 production, resulting in anti-proliferative and phenotype switching effects (154).
Krüppel-Like Factor 5
Contrary to its inductive effect on endothelial KLF2, resveratrol has been shown to decrease TGF-β-mediated KLF5 transcription (155). Through its inhibition of the Akt-mTOR pathway, resveratrol is capable of blocking KLF5-driven VSMC dedifferentiation, thereby preventing intimal hyperplasia. Additionally, targeting this TGF-β/phospho-Akt/phospho-mTOR/KLF5 axis with Akt inhibitor LY249004 or mTOR inhibitor rapamycin also decreases KLF5 levels. As previously mentioned, retinoid agonists and antagonists can also diminish and augment KLF5 activity, respectively, by targeting processes downstream of KLF5-mediated transcription. A recent study demonstrated that the PPARγ agonist, rosiglitazone, is capable of reducing VSMC proliferation by suppressing KLF5 expression (156). While PPAR agonists have differential effects on KLF expression (see KLF11 below), their importance in modulating KLF activity cannot be understated as they represent critical modulators of vascular inflammation. Interestingly, there is also evidence that the traditional Chinese medicine Tongxinluo inhibits macrophage KLF5 transcription and blocks PI3K/Akt signaling to prevent KLF5 sumoylation (109).
Krüppel-Like Factor 10
Given its importance in Treg homeostasis, targeting KLF10 is a potential therapeutic option to combat vascular inflammation. Interestingly, a screen investigating small molecule inhibitors of KLF10 identified multiple compounds that are able to prevent conversion of CD4+ CD25− T cells to CD4+ CD25+ Tregs (157). While this was done in the context of reducing Treg effects in immunosuppression seen in cancer, it is feasible that a similar screen can be utilize to identify small molecule activators of KLF10 to be used in inflammatory conditions.
Krüppel-Like Factor 11
Krüppel-like factor 11 is under the transcriptional control of PPAR nuclear receptors and its expression and activity can be indirectly targeted through the use of PPAR agonists. The PPARα ligand fenofibrate stimulates KLF11 transcription and, therefore, inhibits ET-1 production (62). In addition, fenofibrate has demonstrable beneficial effects in preventing diabetic microvascular complications (158). Taken together, KLF11 targeting may serve as a potential mechanism of vascular protection during PPARα agonist use. Pioglitazone, a PPARγ agonist, has cytoprotective properties in cerebrovascular ECs in vitro and in vivo (61). In the absence of KLF11, however, these effects are lost, indicating a dependency of pioglitazone on KLF11.
Concluding Remarks
Vascular inflammation is central to the pathogenesis of a wide array of debilitating conditions, especially those most prominent in Western society. Inflammatory responses in the vessel wall and circulating cells are governed, in part, through the action of select transcriptional regulators with a body of evidence pointing to the KLFs as having such a role. As critical regulators of the vascular inflammatory response in multiple tissue types, future investigations of the KLFs utilizing whole transcriptome approaches will provide valuable information regarding the breadth of KLF influence as well as potential interactions among them; these promise to be complex, as the shared consensus sequence 5′-C(A/T)CCC-3′ is prevalent throughout the genome. Additionally, the KLFs may represent attractive targets for therapeutic intervention; this will require further exploration, as the targeting of zinc-finger transcription factors remains non-trivial. Ultimately, mechanistic and therapeutic insights in KLF biology will advance our understanding of the complex signaling networks at play during vascular inflammation.
Author Contributions
DS, LF, PH, and MJ contributed conception of the manuscript. DS wrote the first draft of the manuscript. DS, LF, PH, and MJ contributed to manuscript revision. All authors read and approved submitted version.
Conflict of Interest Statement
The authors declare that the research was conducted in the absence of any commercial or financial relationships that could be construed as a potential conflict of interest.
Funding
This work was supported by the National Institute of Health (NIH) grants R01DK111468, R35HL135789, and R01HL086548 (to MJ), T32GM007250 (to DS, LF, and PH), F30AG054237 (to PH), T32HL134622 (to LF), and F30HL139014 (to DS).
References
1. Xiao L, Liu Y, Wang N. New paradigms in inflammatory signaling in vascular endothelial cells. Am J Physiol Heart Circ Physiol (2014) 306(3):H317–25. doi:10.1152/ajpheart.00182.2013
2. Brasier AR. The nuclear factor-B-interleukin-6 signalling pathway mediating vascular inflammation. Cardiovasc Res (2010) 86(2):211–8. doi:10.1093/cvr/cvq076
3. Yamakuchi M. MicroRNAs in vascular biology. Int J Vasc Med (2012) 2012:794898. doi:10.1155/2012/794898
4. Preiss A, Rosenberg UB, Kienlin A, Seifert E, Jäckle H. Molecular genetics of Krüppel, a gene required for segmentation of the Drosophila embryo. Nature (1985) 313(5997):27–32. doi:10.1038/313027a0
5. McConnell BB, Yang VW. Mammalian Kruppel-like factors in health and diseases. Physiol Rev (2010) 90(4):1337–81. doi:10.1152/physrev.00058.2009
6. Swamynathan SK. Krüppel-like factors: three fingers in control. Hum Genomics (2010) 4(4):263–70. doi:10.1186/1479-7364-4-4-263
7. Parmar KM, Larman HB, Dai G, Zhang Y, Wang ET, Moorthy SN, et al. Integration of flow-dependent endothelial phenotypes by Kruppel-like factor 2. J Clin Invest (2006) 116(1):49–58. doi:10.1172/JCI24787
8. Dekker RJ, Boon RA, Rondaij MG, Kragt A, Volger OL, Elderkamp YW, et al. KLF2 provokes a gene expression pattern that establishes functional quiescent differentiation of the endothelium. Blood (2006) 107(11):4354–64. doi:10.1182/blood-2005-08-3465
9. Fledderus JO, Van Thienen JV, Boon RA, Dekker RJ, Rohlena J, Volger OL, et al. Prolonged shear stress and KLF2 suppress constitutive proinflammatory transcription through inhibition of ATF2. Blood (2007) 109(10):4249–58. doi:10.1182/blood-2006-07-036020
10. Bhattacharya R, Senbanerjee S, Lin Z, Mir S, Hamik A, Wang P, et al. Inhibition of vascular permeability factor/vascular endothelial growth factor-mediated angiogenesis by the Kruppel-like factor KLF2. J Biol Chem (2005) 280(32):28848–51. doi:10.1074/jbc.C500200200
11. Senbanerjee S, Lin Z, Atkins GB, Greif DM, Rao RM, Kumar A, et al. KLF2 is a novel transcriptional regulator of endothelial proinflammatory activation. J Exp Med (2004) 199(10):1305–15. doi:10.1084/jem.20031132
12. Boon RA, Leyen TA, Fontijn RD, Fledderus JO, Baggen JMC, Volger OL, et al. KLF2-induced actin shear fibers control both alignment to flow and JNK signaling in vascular endothelium KLF2-induced actin shear fibers control both alignment to flow and JNK signaling in vascular endothelium. Blood (2010) 115(12):2533–42. doi:10.1182/blood-2009-06-228726
13. Ali F, Hamdulay SS, Kinderlerer AR, Boyle JJ, Lidington EA, Yamaguchi T, et al. Statin-mediated cytoprotection of human vascular endothelial cells: a role for Kruppel-like factor 2-dependent induction of heme oxygenase-1. J Thromb Haemost (2007) 5(12):2537–46. doi:10.1111/j.1538-7836.2007.02787.x
14. Hergenreider E, Heydt S, Tréguer K, Boettger T, Horrevoets AJG, Zeiher AM, et al. Atheroprotective communication between endothelial cells and smooth muscle cells through miRNAs. Nat Cell Biol (2012) 14(3):249–56. doi:10.1038/ncb2441
15. Pathak R, Shao L, Chafekar SM, Feng W, Ponnappan U, Fink LM. IKK B regulates endothelial thrombomodulin in a Klf2-dependent manner. J Thromb Haemost (2014) 12(9):1533–44. doi:10.1111/jth.12664
16. Lin Z, Kumar A, Senbanerjee S, Staniszewski K, Parmar K, Vaughan DE, et al. Kruppel-like factor 2 (KLF2) regulates endothelial thrombotic function. Circ Res (2005) 96(5):e48–57. doi:10.1161/01.RES.0000159707.05637.a1
17. Lin Z, Hamik A, Jain R, Kumar A, Jain MK. Kruppel-like factor 2 inhibits protease activated receptor-1 expression and thrombin-mediated endothelial activation. Arterioscler Thromb Vasc Biol (2006) 26(5):1185–9. doi:10.1161/01.ATV.0000215638.53414.99
18. Kuo CT, Veselits ML, Barton KP, Lu MM, Clendenin C, Leiden JM. The LKLF transcription factor is required for normal tunica media formation and blood vessel stabilization during murine embryogenesis. Genes Dev (1997) 11(22):2996–3006. doi:10.1101/gad.11.22.2996
19. Kuo CT, Veselits ML, Leiden JM. LKLF: a transcriptional regulator of single-positive T cell quiescence and survival. Science (1997) 277:1986–90. doi:10.1126/science.277.5334.1986
20. Atkins GB, Wang Y, Mahabeleshwar GH, Shi H, Gao H, Kawanami D, et al. Hemizygous deficiency of Kruppel-like factor 2 augments experimental atherosclerosis. Circ Res (2008) 103(7):690–3. doi:10.1161/CIRCRESAHA.108.184663
21. Nayak L, Shi H, Atkins GB, Lin Z, Schmaier AH, Jain MK. The thromboprotective effect of bortezomib is dependent on the transcription factor Kruppel-like factor 2 (KLF2). Blood (2014) 123(24):3828–32. doi:10.1182/blood-2014-01-547448
22. Lee HY, Youn SW, H-J Cho, Kwon YW, Lee SW, Kim SJ, et al. FOXO1 impairs whereas statin protects endothelial function in diabetes through reciprocal regulation of Krüppel-like factor 2. Cardiovasc Res (2013) 97(1):143–52. doi:10.1093/cvr/cvs283
23. Zhong F, Chen H, Wei C, Zhang W, Li Z, Jain MK, et al. Ppel-like factor 2 expression may reduced Kru aggravate the endothelial injury of diabetic nephropathy. Kidney Int (2015) 87(2):382–95. doi:10.1038/ki.2014.286
24. Fang X, Zhong X, Yu G, Shao S, Yang Q. Vascular protective effects of KLF2 on Aβ-induced toxicity: implications for Alzheimer’s disease. Brain Res (2017) 1663:174–83. doi:10.1016/j.brainres.2017.01.006
25. Dekker RJ, van Thienen JV, Rohlena J, de Jager SC, Elderkamp YW, Seppen J, et al. Endothelial KLF2 links local arterial shear stress levels to the expression of vascular tone-regulating genes. Am J Pathol (2005) 167(2):609–18. doi:10.1016/S0002-9440(10)63002-7
26. Kumar A, Lin Z, Senbanerjee S, Jain MK. Tumor necrosis factor alpha-mediated reduction of KLF2 is due to inhibition of MEF2 by NF-κB and histone deacetylases tumor necrosis factor alpha-mediated reduction of KLF2 is due to inhibition of MEF2 by NF-B and histone deacetylases. Mol Cell Biol (2005) 25(14):5893–903. doi:10.1128/MCB.25.14.5893
27. Feaver RE, Gelfand BD, Blackman BR. Human haemodynamic frequency harmonics regulate the inflammatory phenotype of vascular endothelial cells. Nat Commun (2013) 4:1525. doi:10.1038/ncomms2530
28. Hajra L, Evans AI, Chen M, Hyduk SJ, Collins T, Cybulsky MI. The NF-kappa B signal transduction pathway in aortic endothelial cells is primed for activation in regions predisposed to atherosclerotic lesion formation. Proc Natl Acad Sci U S A (2000) 97(16):9052–7. doi:10.1073/PNAS.97.16.9052
29. Xu Z, Yoshida T, Wu L, Maiti D, Cebotaru L, Duh EJ. Transcription factor MEF2C suppresses endothelial cell inflammation via regulation of NF-κB and KLF2. J Cell Physiol (2015) 230(6):1310–20. doi:10.1002/jcp.24870
30. Kumar A, Kim CS, Hoffman TA, Naqvi A, Dericco J, Jung SB, et al. P53 impairs endothelial function by transcriptionally repressing Kruppel-like factor 2. Arterioscler Thromb Vasc Biol (2011) 31(1):133–41. doi:10.1161/ATVBAHA.110.215061
31. Kwon IS, Wang W, Xu S, Jin ZG. Histone deacetylase 5 interacts with Krüppel-like factor 2 and inhibits its transcriptional activity in endothelium. Cardiovasc Res (2014) 104(1):127–37. doi:10.1093/cvr/cvu183
32. Wang W, Ha CH, Jhun BS, Wong C, Jain MK, Jin ZG. Fluid shear stress stimulates phosphorylation-dependent nuclear export of HDAC5 and mediates expression of KLF2 and eNOS. Blood (2010) 115(14):2971–9. doi:10.1182/blood-2009-05-224824
33. Loyer X, Potteaux S, Vion AC, Guérin CL, Boulkroun S, Rautou PE, et al. Inhibition of microRNA-92a prevents endothelial dysfunction and atherosclerosis in mice. Circ Res (2014) 114(3):434–43. doi:10.1161/CIRCRESAHA.114.302213
34. Wu W, Xiao H, Laguna-Fernandez A, Villarreal G, Wang KC, Geary GG, et al. Flow-dependent regulation of Krüppel-like factor 2 is mediated by microRNA-92a. Circulation (2011) 124(5):633–41. doi:10.1161/CIRCULATIONAHA.110.005108
35. Fang Y, Davies PF. Site-specific microRNA-92a regulation of Kruppel-like factors 4 and 2 in atherosusceptible endothelium. Arterioscler Thromb Vasc Biol (2012) 32(4):979–87. doi:10.1161/ATVBAHA.111.244053
36. van Thienen JV, Fledderus JO, Dekker RJ, Rohlena J, van IJzendoorn GA, Kootstra NA, et al. Shear stress sustains atheroprotective endothelial KLF2 expression more potently than statins through mRNA stabilization. Cardiovasc Res (2006) 72(2):231–40. doi:10.1016/j.cardiores.2006.07.008
37. Hamik A, Lin Z, Kumar A, Balcells M, Sinha S, Katz J, et al. Kruppel-like factor 4 regulates endothelial inflammation. J Biol Chem (2007) 282(18):13769–79. doi:10.1074/jbc.M700078200
38. Zhou G, Hamik A, Nayak L, Tian H, Shi H, Lu Y, et al. Endothelial Kruppel-like factor 4 protects against atherothrombosis in mice. J Clin Invest (2012) 122(12):4727–31. doi:10.1172/JCI66056
39. Jiang YZ, Jimenez JM, Ou K, McCormick ME, Zhang LD, Davies PF. Hemodynamic disturbed flow induces differential DNA methylation of endothelial Kruppel-like factor 4 promoter in vitro and in vivo. Circ Res (2014) 115(1):32–43. doi:10.1161/CIRCRESAHA.115.303883
40. Dunn J, Qiu H, Kim S, Jjingo D, Hoffman R, Kim W, et al. Flow alters genome-wide methylation, regulating endothelial gene expression and atherosclerosis. J Clin Invest (2014) 124(7):3187–99. doi:10.1172/JCI74792.(24)
41. Yoshida T, Yamashita M, Horimai C, Hayashi M. Deletion of Krüppel-like factor 4 in endothelial and hematopoietic cells enhances neointimal formation following vascular injury. J Am Heart Assoc (2014) 3(1):e000622. doi:10.1161/JAHA.113.000622
42. Shatat MA, Tian H, Zhang R, Tandon G, Hale A, Fritz JS, et al. Endothelial Krüppel-like factor 4 modulates pulmonary arterial hypertension. Am J Respir Cell Mol Biol (2014) 50(3):647–53. doi:10.1165/rcmb.2013-0135OC
43. Ohnesorge N, Viemann D, Schmidt N, Czymai T, Spiering D, Schmolke M, et al. ERK5 activation elicits a vasoprotective endothelial phenotype via induction of Kruppel-like factor 4 (KLF4). J Biol Chem (2010) 285(34):26199–210. doi:10.1074/jbc.M110.103127
44. Li Z, Martin M, Zhang J, Huang HY, Bai L, Zhang J, et al. KLF4 regulation of Ch25h and LXR mitigates atherosclerosis susceptibility. Circulation (2017) 136(14):1315–30. doi:10.1161/CIRCULATIONAHA.117.027462
45. Rubinsztein DC, Mariño G, Kroemer G. Autophagy and aging. Cell (2011) 146(5):682–95. doi:10.1016/j.cell.2011.07.030
46. Hsieh PN, Zhou G, Yuan Y, Zhang R, Prosdocimo DA, Sangwung P, et al. A conserved KLF-autophagy pathway modulates nematode lifespan and mammalian age-associated vascular dysfunction. Nat Commun (2017) 8(1):914. doi:10.1038/s41467-017-00899-5
47. Yoshida T, Yamashita M, Iwai M, Hayashi M. Endothelial Krüppel-like factor 4 mediates the protective effect of statins against ischemic AKI. J Am Soc Nephrol (2015) 27(5):1379–88. doi:10.1681/ASN.2015040460
48. Hartmann P, Zhou Z, Natarelli L, Wei Y, Nazari-Jahantigh M, Zhu M, et al. Endothelial dicer promotes atherosclerosis and vascular inflammation by miRNA-103-mediated suppression of KLF4. Nat Commun (2016) 7:10521. doi:10.1038/ncomms10521
49. Bieker JJ. Krüppel-like factors: three fingers in many pies. J Biol Chem (2001) 276(37):34355–8. doi:10.1074/jbc.R100043200
50. Sangwung P, Zhou G, Nayak L, Chan ER, Kumar S, Kang DW, et al. KLF2 and KLF4 control endothelial identity and vascular integrity. JCI Insight (2017) 2(4):e91700. doi:10.1172/jci.insight.91700DS1
51. Kumekawa M, Fukuda G, Shimizu S, Konno K, Odawara M. Inhibition of monocyte chemoattractant protein-1 by Krüppel-like factor 5 small interfering RNA in the tumor necrosis factor-alpha-activated human umbilical vein endothelial cells. Biol Pharm Bull (2008) 31(8):1609–13. doi:10.1248/bpb.31.1609
52. Aiello RJ, Bourassa P-A, Lindsey S, Weng W, Natoli E, Rollins BJ, et al. Monocyte chemoattractant protein-1 accelerates atherosclerosis in apolipoprotein E-deficient mice. Arterioscler Thromb Vasc Biol (1999) 19(6):1518–25. doi:10.1161/01.ATV.19.6.1518
53. Haubner F, Lehle K, Münzel D, Schmid C, Birnbaum DE, Preuner JG. Hyperglycemia increases the levels of vascular cellular adhesion molecule-1 and monocyte-chemoattractant-protein-1 in the diabetic endothelial cell. Biochem Biophys Res Commun (2007) 360(3):560–5. doi:10.1016/j.bbrc.2007.06.044
54. Ito WD, Arras M, Winkler B, Scholz D, Schaper J, Schaper W. Monocyte chemotactic protein-1 increases collateral and peripheral conductance after femoral artery occlusion. Circ Res (1997) 80(6):829–37. doi:10.1161/01.RES.80.6.829
55. Gallardo-Vara E, Blanco FJ, Roqué M, Friedman SL, Suzuki T, Botella LM, et al. Transcription factor KLF6 upregulates expression of metalloprotease MMP14 and subsequent release of soluble endoglin during vascular injury. Angiogenesis (2016) 19(2):155–71. doi:10.1007/s10456-016-9495-8
56. Botella LM, Sa T, Sanz-rodriguez F, Kojima S, Shimada J, Guerrero-esteo M, et al. Transcriptional activation of endoglin and transforming growth factor-β signaling components by cooperative interaction between Sp1 and KLF6: their potential role in the response to vascular injury. Blood (2002) 100(12):4001–10. doi:10.1182/blood.V100.12.4001
57. Rossi E, Sanz-Rodriguez F, Eleno N, Düwell A, Blanco FJ, Langa C, et al. Endothelial endoglin is involved in inflammation: role in leukocyte adhesion and transmigration. Blood (2013) 121(2):403–15. doi:10.1182/blood-2012-06-435347
58. Garrido-Martin EM, Blanco FJ, Roque M, Novensa L, Tarocchi M, Lang UE, et al. Vascular injury triggers Kruppel-like factor 6 mobilization and cooperation with specificity protein 1 to promote endothelial activation through upregulation of the activin receptor-like kinase 1 gene. Circ Res (2013) 112(1):113–27. doi:10.1161/CIRCRESAHA.112.275586
59. Fan Y, Guo Y, Zhang J, Subramaniam M, Song CZ, Urrutia R, et al. Krüppel-like factor-11, a transcription factor involved in diabetes mellitus, suppresses endothelial cell activation via the nuclear factor-κB signaling pathway. Arterioscler Thromb Vasc Biol (2012) 32(12):2981–8. doi:10.1161/ATVBAHA.112.300349
60. Tang X, Liu K, Hamblin MH, Xu Y, Yin KJ. Genetic deletion of Krüppel-like factor 11 aggravates ischemic brain injury. Mol Neurobiol (2017). doi:10.1007/s12035-017-0556-9
61. Yin KJ, Fan Y, Hamblin M, Zhang J, Zhu T, Li S, et al. KLF11 mediates PPAR cerebrovascular protection in ischaemic stroke. Brain (2017) 136:1274–87. doi:10.1093/brain/awt002
62. Glineur C, Gross B, Neve B, Rommens C, Chew GT, Martin-nizard F, et al. Fenofibrate inhibits endothelin-1 expression by peroxisome proliferator-activated receptor α-dependent and independent mechanisms in human endothelial cells. Arterioscler Thromb Vasc Biol (2013) 33(3):621–8. doi:10.1161/ATVBAHA.112.300665
63. Gomez D, Owens GK. Smooth muscle cell phenotypic switching in atherosclerosis. Cardiovasc Res (2012) 95(2):156–64. doi:10.1093/cvr/cvs115
64. Yoshida T, Kaestner KH, Owens GK. Conditional deletion of Krüppel-like factor 4 delays downregulation of smooth muscle cell differentiation markers but accelerates neointimal formation following vascular injury. Circ Res (2008) 102(12):1548–57. doi:10.1161/CIRCRESAHA.108.176974
65. Wassmann S, Wassmann K, Jung A, Velten M, Knuefermann P, Petoumenos V, et al. Induction of p53 by GKLF is essential for inhibition of proliferation of vascular smooth muscle cells. J Mol Cell Cardiol (2007) 43(3):301–7. doi:10.1016/j.yjmcc.2007.06.001
66. Wang Y, Zhao B, Zhang Y, Tang Z, Shen Q, Zhang Y, et al. Krüppel-like factor 4 is induced by rapamycin and mediates the anti-proliferative effect of rapamycin in rat carotid arteries after balloon injury. Br J Pharmacol (2012) 165(7):2378–88. doi:10.1111/j.1476-5381.2011.01734.x
67. Liu Y, Sinha S, Owens G. A transforming growth factor-β control element required for SM α-actin expression in vivo also partially mediates GKLF-dependent transcriptional repression. J Biol Chem (2003) 278(48):48004–11. doi:10.1074/jbc.M301902200
68. Yoshida T, Gan Q, Owens GK. Kruppel-like factor 4, Elk-1, and histone deacetylases cooperatively suppress smooth muscle cell differentiation markers in response to oxidized phospholipids. Am J Physiol Cell Physiol (2008) 295(5):C1175–82. doi:10.1152/ajpcell.00288.2008
69. Shankman LS, Gomez D, Cherepanova OA, Salmon M, Alencar GF, Haskins RM, et al. KLF4-dependent phenotypic modulation of smooth muscle cells has a key role in atherosclerotic plaque pathogenesis. Nat Med (2015) 21(6):628–37. doi:10.1038/nm.3866
70. Adam PJ, Regan CP, Hautmann MB, Owens GK. Positive- and negative-acting Kruppel-like transcription factors bind a transforming growth factor β control element required for expression of the smooth muscle cell differentiation marker SM22α in vivo. J Biol Chem (2000) 275(48):37798–806. doi:10.1074/jbc.M006323200
71. Pidkovka NA, Cherepanova OA, Yoshida T, Alexander MR, Deaton RA, Thomas JA, et al. Oxidized phospholipids induce phenotypic switching of vascular smooth muscle cells in vivo and in vitro. Circ Res (2007) 101(8):792–801. doi:10.1161/CIRCRESAHA.107.152736
72. Cordes KR, Sheehy NT, White MP, Berry EC, Morton SU, Muth AN, et al. miR-145 and miR-143 regulate smooth muscle cell fate and plasticity. Nature (2009) 460(7256):705–10. doi:10.1038/nature08195
73. Starke RM, Ali MS, Jabbour PM, Tjoumakaris SI, Gonzalez F, Hasan DM, et al. Cigarette smoke modulates vascular smooth muscle phenotype: implications for carotid and cerebrovascular disease. PLoS One (2013) 8(8):e71954. doi:10.1371/journal.pone.0071954
74. Cheng Y, Liu X, Yang J, Lin Y, Xu DZ, Lu Q, et al. MicroRNA-145, a novel smooth muscle cell phenotypic marker and modulator, controls vascular neointimal lesion formation. Circ Res (2009) 105(2):158–66. doi:10.1161/CIRCRESAHA.109.197517
75. Fujiu K, Manabe I, Ishihara A, Oishi Y, Iwata H, Nishimura G, et al. Synthetic retinoid Am80 suppresses smooth muscle phenotypic modulation and in-stent neointima formation by inhibiting KLF5. Circ Res (2005) 97(11):1132–41. doi:10.1161/01.RES.0000190613.22565.13
76. Kaplan-Albuquerque N, Bogaert YE, Van Putten V, Weiser-Evans MC, Nemenoff RA. Patterns of gene expression differentially regulated by platelet-derived growth factor and hypertrophic stimuli in vascular smooth muscle cells: markers for phenotypic modulation and response to injury. J Biol Chem (2005) 280(20):19966–76. doi:10.1074/jbc.M500917200
77. Shindo T, Manabe I, Fukushima Y, Tobe K, Aizawa K, Miyamoto S, et al. Krüppel-like zinc-finger transcription factor KLF5/BTEB2 is a target for angiotensin II signaling and an essential regulator of cardiovascular remodeling. Nat Med (2002) 8(8):856. doi:10.1038/nm738
78. Aizawa K, Suzuki T, Kada N, Ishihara A, Kawai-Kowase K, Matsumura T, et al. Regulation of platelet-derived growth factor-A chain by Krüppel-like factor 5: new pathway of cooperative activation with nuclear factor-kappaB. J Biol Chem (2004) 279(1):70–6. doi:10.1074/jbc.M306621200
79. Zhang ML, Zheng B, Tong F, Yang Z, Wang ZB, Yang BM, et al. iNOS-derived peroxynitrite mediates high glucose-induced inflammatory gene expression in vascular smooth muscle cells through promoting KLF5 expression and nitration. Biochim Biophys Acta (2017) 1863(11):2821–34. doi:10.1016/j.bbadis.2017.07.004
80. Courboulin A, Tremblay VL, Barrier M, Meloche J, Jacob MH, Chapolard M, et al. Krüppel-like factor 5 contributes to pulmonary artery smooth muscle proliferation and resistance to apoptosis in human pulmonary arterial hypertension. Respir Res (2011) 12:128. doi:10.1186/1465-9921-12-128
81. Li X, He Y, Xu Y, Huang X, Liu J, Xie M, et al. KLF5 mediates vascular remodeling via HIF-1α in hypoxic pulmonary hypertension. Am J Physiol Lung Cell Mol Physiol (2015) 310(4):L299–310. doi:10.1152/ajplung.00189.2015
82. Li Y, Li J, Hou Z, Yu Y, Yu B. KLF5 overexpression attenuates cardiomyocyte inflammation induced by oxygen-glucose deprivation/reperfusion through the PPARg/PGC-1a/TNF-a signaling pathway. Biomed Pharmacother (2016) 84:940–6. doi:10.1016/j.biopha.2016.09.100
83. Boettger T, Beetz N, Kostin S, Schneider J, Krüger M, Hein L, et al. Acquisition of the contractile phenotype by murine arterial smooth muscle cells depends on the miR143/145 gene cluster. J Clin Invest (2009) 119(9):2634–47. doi:10.1172/JCI38864
84. Zhang YN, Xie BD, Sun L, Chen W, Jiang SL, Liu W, et al. Phenotypic switching of vascular smooth muscle cells in the ’normal region’ of aorta from atherosclerosis patients is regulated by miR-145. J Cell Mol Med (2016) 20(6):1049–61. doi:10.1111/jcmm.12825
85. Zheng B, Yin WN, Suzuki T, Zhang XH, Zhang Y, Song LL, et al. Exosome-mediated miR-155 transfer from smooth muscle cells to endothelial cells induces endothelial injury and promotes atherosclerosis. Mol Ther (2017) 25(6):1279–94. doi:10.1016/j.ymthe.2017.03.031
86. Fisch S, Gray S, Heymans S, Haldar SM, Wang B, Pfister O, et al. Kruppel-like factor 15 is a regulator of cardiomyocyte hypertrophy. Proc Natl Acad Sci U S A (2007) 104(17):7074–9. doi:10.1073/pnas.0701981104
87. Prosdocimo DA, Anand P, Liao X, Zhu H, Shelkay S, Artero-Calderon P, et al. Kruppel-like factor 15 is a critical regulator of cardiac lipid metabolism. J Biol Chem (2014) 289(9):5914–24. doi:10.1074/jbc.M113.531384
88. Jeyaraj D, Haldar SM, Wan X, McCauley MD, Ripperger JA, Hu K, et al. Circadian rhythms govern cardiac repolarization and arrhythmogenesis. Nature (2012) 483:96–9. doi:10.1038/nature10852
89. Lu Y, Zhang L, Liao X, Sangwung P, Prosdocimo DA, Zhou G, et al. Kruppel-like factor 15 is critical for vascular inflammation. J Clin Invest (2013) 123(10):4232–41. doi:10.1172/JCI68552DS1
90. Lu Y, Haldar S, Croce K, Wang Y, Sakuma M, Morooka T, et al. Kruppel-like factor 15 regulates smooth muscle response to vascular injury. Arterioscler Thromb Vasc Biol (2010) 30(8):1550–2. doi:10.1161/ATVBAHA.110.207050
91. Haldar SM, Lu Y, Jeyaraj D, Kawanami D, Cui Y, Eapen SJ, et al. KLF15 deficiency is a molecular link between heart failure and aortic aneurysm formation. Sci Transl Med (2010) 2(26):26ra26. doi:10.1126/scitranslmed.3000502
92. Nakaoka H, Tajima A, Yoneyama T, Hosomichi K, Kasuya H, Mizutani T, et al. Gene expression profiling reveals distinct molecular signatures associated with the rupture of intracranial aneurysm. Stroke (2014) 45(8):2239–45. doi:10.1161/STROKEAHA.114.005851
93. Moore KJ, Tabas I. Macrophages in the pathogenesis of atherosclerosis. Cell (2011) 145(3):341–55. doi:10.1016/j.cell.2011.04.005
94. Campbell KA, Lipinski MJ, Doran AC, Skaflen MD, Fuster V, McNamara CA. Lymphocytes and the adventitial immune response in atherosclerosis. Circ Res (2012) 110(6):889–900. doi:10.1161/CIRCRESAHA.111.263186
95. Mahabeleshwar GH, Kawanami D, Sharma N, Takami Y, Zhou G, Shi H, et al. The myeloid transcription factor KLF2 regulates the host response to polymicrobial infection and endotoxic shock. Immunity (2011) 34(5):715–28. doi:10.1016/j.immuni.2011.04.014
96. Mahabeleshwar GH, Qureshi MA, Takami Y, Sharma N, Lingrel JB, Jain MK. A myeloid hypoxia-inducible factor 1α-Krüppel-like factor 2 pathway regulates gram-positive endotoxin-mediated sepsis. J Biol Chem (2012) 287(2):1448–57. doi:10.1074/jbc.M111.312702
97. Nayak L, Goduni L, Takami Y, Sharma N, Kapil P, Jain MK, et al. Kruppel-like factor 2 is a transcriptional regulator of chronic and acute inflammation. Am J Pathol (2013) 182(5):1696–704. doi:10.1016/j.ajpath.2013.01.029
98. Das H, Kumar A, Lin Z, Patino WD, Hwang PM, Feinberg MW, et al. Kruppel-like factor 2 (KLF2) regulates proinflammatory activation of monocytes. Proc Natl Acad Sci U S A (2006) 103(17):6653–8. doi:10.1073/pnas.0508235103
99. Lingrel JB, Pilcher-Roberts R, Basford JE, Manoharan P, Neumann J, Konaniah ES, et al. Myeloid-specific Krüppel-like factor 2 inactivation increases macrophage and neutrophil adhesion and promotes atherosclerosis. Circ Res (2012) 110(10):1294–302. doi:10.1161/CIRCRESAHA.112.267310
100. Alberts-Grill N, Engelbertsen D, Bu D, Grabie N, Herter JM, Kuperwaser F, et al. Dendritic cell KLF2 expression regulates T cell activation and proatherogenic immune responses. J Immunol (2016) 197(12):4651–62. doi:10.4049/jimmunol.1600206
101. Tits LJHV, Stienstra R, Van Lent PL, Netea MG, Joosten LAB, Stalenhoef AFH. Oxidized LDL enhances pro-inflammatory responses of alternatively activated M2 macrophages: a crucial role for Krüppel-like factor 2. Atherosclerosis (2011) 214(2):345–9. doi:10.1016/j.atherosclerosis.2010.11.018
102. Liao X, Sharma N, Kapadia F, Zhou G, Lu Y, Hong H, et al. Kruppel-like factor 4 regulates macrophage polarization. J Clin Invest (2011) 121(7):2736–49. doi:10.1172/JCI45444DS1
103. Kapoor N, Niu J, Saad Y, Kumar S, Sirakova T, Becerra E, et al. Transcription factors STAT6 and KLF4 implement macrophage polarization via the dual catalytic powers of MCPIP. J Immunol (2015) 194(12):6011–23. doi:10.4049/jimmunol.1402797
104. Wen AY, Sakamoto KM, Miller LS. The role of transcription factor CREB in immune function. J Immunol (2010) 185:6413–9. doi:10.4049/jimmunol.1001829
105. Stavri S, Simionescu M, Kardassis D, Gafencu AV. Krüppel-like factor 4 synergizes with CREB to increase the activity of apolipoprotein E gene promoter in macrophages. Biochem Biophys Res Commun (2015) 468:66–72. doi:10.1016/j.bbrc.2015.10.163
106. Bellosta S, Mahley RW, Sanan DA, Murata J, Newland DL, Taylor JM, et al. Macrophage-specific expression of human apolipoprotein E reduces athersclerosis in hypercholesterolemic apolipoprotein E-null mice. J Clin Invest (1995) 96(5):2170–9. doi:10.1172/JCI118271
107. Zanotti I, Pedrelli M, Potì F, Stomeo G, Gomaraschi M, Calabresi L, et al. Macrophage, but not systemic, apolipoprotein E is necessary for macrophage reverse cholesterol transport in vivo. Arterioscler Thromb Vasc Biol (2011) 31:74–80. doi:10.1161/ATVBAHA.110.213892
108. Sharma N, Lu Y, Zhou G, Liao X, Kapil P, Anand P, et al. Myeloid Krüppel-like factor 4 deficiency augments atherogenesis in ApoE-/- mice—brief report. Arterioscler Thromb Vasc Biol (2012) 32(12):2836–8. doi:10.1161/ATVBAHA.112.300471
109. Jiang W, Zheng B, Zhang XH, Yue LY, Liu C, Ma D, et al. Tongxinluo inhibits neointimal formation by regulating the expression and post-translational modification of KLF5 in macrophages. Am J Transl Res (2016) 8(11):4778–90.
110. Chen C, Zhou Z, Guo P, Tang Dong J. Proteasomal degradation of the KLF5 transcription factor through a ubiquitin-independent pathway. FEBS Lett (2007) 581(6):1124–30. doi:10.1016/j.febslet.2007.02.018
111. Date D, Das R, Narla G, Simon DI, Jain MK, Mahabeleshwar GH. Kruppel-like transcription factor 6 regulates inflammatory macrophage polarization. J Biol Chem (2014) 289(15):10318–29. doi:10.1074/jbc.M113.526749
112. Zhang Y, Lei CQ, Hu YH, Xia T, Li M, Zhong B, et al. Krüppel-like factor 6 is a co-activator of NF-κB that mediates p65-dependent transcription of selected downstream genes. J Biol Chem (2014) 289(18):12876–85. doi:10.1074/jbc.M113.535831
113. Kim GD, Das R, Goduni L, McClellan S, Hazlett LD, Mahabeleshwar GH. Kruppel-like factor 6 promotes macrophage-mediated inflammation by suppressing B cell leukemia/lymphoma 6 expression. J Biol Chem (2016) 291(40):21271–82. doi:10.1074/jbc.M116.738617
114. Civelek M, Lusis AJ. Systems genetics approaches to understand complex traits. Nat Rev Genet (2014) 15(1):34–48. doi:10.1038/nrg3575
115. Wei X, Yang R, Wang C, Jian X, Li L, Liu H, et al. A novel role for the Krüppel-like factor 14 on macrophage inflammatory response and atherosclerosis development. Cardiovasc Pathol (2017) 27:1–8. doi:10.1016/j.carpath.2016.11.003
116. Tabas I. Consequences of cellular cholesterol accumulation: basic concepts and physiological implications. J Clin Invest (2002) 110(7):905–11. doi:10.1172/JCI16452
117. Grayson JM, Murali-Krishna K, Altman JD, Ahmed R. Gene expression in antigen-specific CD8+ T cells during viral infection. J Immunol (2001) 166:795–9. doi:10.4049/jimmunol.166.2.795
118. Schober SL, Kuo CT, Schluns KS, Lefrancois L, Leiden JM, Jameson SC. Expression of the transcription factor lung Krüppel-like factor is regulated by cytokines and correlates with survival of memory T cells in vitro and in vivo. J Immunol (1999) 163:3662–7.
119. Wu J, Lingrel JB. Krüppel-like factor 2, a novel immediate-early transcriptional factor, regulates IL-2 expression in T lymphocyte activation. J Immunol (2005) 175:3060–6. doi:10.4049/jimmunol.175.5.3060
120. Carlson CM, Endrizzi BT, Wu J, Ding X, Weinreich MA, Walsh ER, et al. Kruppel-like factor 2 regulates thymocyte and T-cell migration. Nature (2006) 442(7100):299–302. doi:10.1038/nature04882
121. Bai A, Hu H, Yeung M, Chen J. Krüppel-like factor 2 controls T cell trafficking by activating L-seclectin (CD62L) and sphingosin-1-phosphate receptor 1 transcription. J Immunol (2007) 178:7632–9. doi:10.4049/jimmunol.178.12.7632
122. Bu DX, Tarrio M, Grabie N, Zhang Y, Yamazaki H, Stavrakis G, et al. Statin-induced Kruppel-like factor 2 expression in human and mouse T cells reduces inflammatory and pathogenic responses. J Clin Invest (2010) 120(6):1961–70. doi:10.1172/JCI41384
123. Pastrana JL, Sha X, Virtue A, Mai J, Cueto R, Lee IA, et al. Regulatory T cells and atherosclerosis. J Clin Exp Cardiol (2012) 2012(Suppl 12):2. doi:10.4172/2155-9880.S12-002.f
124. Li M, Wang X, Fu W, He S, Li D, Ke Q. CD4+ CD25+ Foxp3+ regulatory T cells protect endothelial function impaired by oxidized low density lipoprotein via the KLF2 transcription factor. Cell Physiol Biochem (2011) 28:639–48. doi:10.1159/000335759
125. Pabbisetty SK, Rabacal W, Maseda D, Cendron D, Collins PL, Hoek KL, et al. KLF2 is a rate-limiting transcription factor that can be targeted to enhance regulatory T-cell production. Proc Natl Acad Sci U S A (2014) 111(26):9579–84. doi:10.1073/pnas.1323493111
126. Winkelmann R, Sandrock L, Porstner M, Roth E, Mathews M, Hobeika E, et al. B cell homeostasis and plasma cell homing controlled by Krüppel-like factor 2. Proc Natl Acad Sci U S A (2011) 108(2):710–5. doi:10.1073/pnas.1012858108
127. Hart GT, Peery SL, Hamilton SE, Jameson SC. Cutting edge: Krüppel-like factor 2 is required for phenotypic maintenance but not development of B1 B cells. J Immunol (2012) 189:3293–897. doi:10.4049/jimmunol.1201439
128. Hart GT, Wang X, Hogquist KA, Jameson SC. Krüppel-like factor 2 (KLF2) regulates B-cell reactivity, subset differentiation, and trafficking molecule expression. Proc Natl Acad Sci U S A (2011) 108(2):716–21. doi:10.1073/pnas.1013168108
129. Vu TT, Gatto D, Turner V, Funnell APW, Mak KS, Norton LJ, et al. Impaired B cell development in the absence of Krüppel-like factor 3. J Immunol (2011) 187:5032–42. doi:10.4049/jimmunol.1101450
130. Alles M, Turchinovich G, Zhang P, Schuh W, Agenès F, Kirberg J. Leukocyte B7 integrin targeted by Krüppel-like factors. J Immunol (2014) 193:1737–46. doi:10.4049/jimmunol.1302613
131. Wen X, Liu H, Xiao G, Liu X. Downregulation of the transcription factor KLF4 is required for lineage commitment of T cells. Cell Res (2011) 21(12):1701–10. doi:10.1038/cr.2011.183
132. An J, Golech S, Klaewsongkram J, Zhang Y, Subedi K, Huston GE, et al. Krüppel-like factor 4 (KLF4) directly regulates proliferation in thymocyte development and IL-17 expression during Th17 differentiation. FASEB J (2011) 25(10):3634–45. doi:10.1096/fj.11-186924
133. Klaewsongkram J, Yang Y, Golech S, Katz J, Kaestner KH, Weng NP. Krüppel-like factor 4 regulates B cell number and activation-induced B cell proliferation. J Immunol (2007) 179:4679–84. doi:10.4049/jimmunol.179.7.4679
134. Tussiwand R, Everts B, Grajales-Reyes GE, Kretzer NM, Iwata A, Bagaitkar J, et al. Klf4 expression in conventional dendritic cells is required for T helper 2 cell responses. Immunity (2015) 42:916–28. doi:10.1016/j.immuni.2015.04.017
135. Cao Z, Wara AK, Icli B, Sun X, Packard RRS, Esen F, et al. Kruppel-like factor KLF10 targets transforming growth factor-B1 to regulate CD4+CD25-T cells and T regulatory cells. J Biol Chem (2009) 284(37):24914–24. doi:10.1074/jbc.M109.000059
136. Xiong Y, Khanna S, Grzenda AL, Sarmento OF, Svingen PA, Lomberk GA, et al. Polycomb antagonizes p300/CREB-binding protein-associated factor to silence FOXP3 in a Kruppel-like factor-dependent manner. J Biol Chem (2012) 287(41):34372–85. doi:10.1074/jbc.M111.32532
137. Xiong Y, Svingen PA, Sarmento OO, Smyrk TC, Dave M, Khanna S, et al. Differential coupling of KLF10 to Sin3-HDAC and PCAF regulates the inducibility of the FOXP3 gene. Am J Physiol Regul Integr Comp Physiol (2014) 307:R608–20. doi:10.1152/ajpregu.00085.2014
138. Venuprasad K, Huang H, Harada Y, Elly C, Subramaniam M, Spelsberg T, et al. The E3 ubiquitin ligase itch regulates expression of transcription Foxp3 and airway inflammation by enhancing the function of transcription factor TIEG1. Nat Immunol (2008) 9(3):245–53. doi:10.1038/ni1564
139. Veillard NR, Kwak B, Pelli G, Mulhaupt F, James RW, Proudfoot AEI, et al. Antagonish of RANTES receptors reduces atherosclerotic plaque formation in mice. Circ Res (2004) 94:253–61. doi:10.1161/01.RES.0000109793.17591.4E
140. Ahn Y-T, Huang B, McPherson L, Clayberger C, Krensky AM. Dynamic interplay of transcriptional machinery and chromatin regulates ’late’ expression of the chemokine RANTES in T lymphocytes. Mol Cell Biol (2007) 27(1):253–66. doi:10.1128/MCB.01071-06
141. Song CZ, Keller K, Murata K, Asano H, Stamatoyannopoulos G. Functional interaction between coactivators CBP/p300, PCAF, and transcription factor FKLF2. J Biol Chem (2002) 277(9):7029–36. doi:10.1074/jbc.M108826200
142. Zhou M, McPherson L, Feng D, Song A, Dong C, Lyu SC, et al. Krüppel-like transcription factor 13 regulates T lymphocyte survival in vivo. J Immunol (2007) 178:5496–504. doi:10.4049/jimminol.178.9.5496
143. Kaczynski J, Zhang JS, Ellenrieder V, Conley A, Duenes T, Kester H, et al. The Sp1-like protein BTEB3 inhibits transcription via the basic transcription box by interacting with mSin3A and HDAC-1 co-repressors and competing with Sp1. J Biol Chem (2001) 276(39):36749–56. doi:10.1074/jbc.M105831200
144. Sen-Banerjee S, Mir S, Lin Z, Hamik A, Atkins GB, Das H, et al. Kruppel-like factor 2 as a novel mediator of statin effects in endothelial cells. Circulation (2005) 112(5):720–6. doi:10.1161/CIRCULATIONAHA.104.525774
145. Parmar KM, Nambudiri V, Dai G, Larman HB, Gimbrone MA, García-Cardeña G. Statins exert endothelial atheroprotective effects via the KLF2 transcription factor. J Biol Chem (2005) 280(29):26714–9. doi:10.1074/jbc.C500144200
146. Tuomisto TT, Lumivuori H, Kansanen E, Häkkinen SK, Turunen MP, Van Thienen JV, et al. Simvastatin has an anti-inflammatory effect on macrophages via upregulation of an atheroprotective transcription factor, Kruppel-like factor 2. Cardiovasc Res (2008) 78(1):175–84. doi:10.1093/cvr/cvn007
147. Le NT, Takei Y, Izawa-Ishizawa Y, Heo KS, Lee H, Smrcka AV, et al. Identification of activators of ERK5 transcriptional activity by high-throughput screening and the role of endothelial ERK5 in vasoprotective effects induced by statins and antimalarial agents. J Immunol (2014) 193(7):3803–15. doi:10.4049/jimmunol.1400571
148. Xu Y, Liu P, Xu S, Koroleva M, Zhang S, Si S, et al. Tannic acid as a plant-derived polyphenol exerts vasoprotection via enhancing KLF2 expression in endothelial cells. Sci Rep (2017) 7(1):6686. doi:10.1038/s41598-017-06803-x
149. Gracia-Sancho J, Villarreal G Jr, Zhang Y, Garcı G. Activation of SIRT1 by resveratrol induces KLF2 expression conferring an endothelial vasoprotective phenotype. Cardiovasc Res (2010) 85(3):514–9. doi:10.1093/cvr/cvp337
150. Petrovski G, Gurusamy N, Das DK. Resveratrol in cardiovascular health and disease. Ann N Y Acad Sci (2011) 1215:22–33. doi:10.1111/j.1749-6632.2010.05843.x
151. Marchal J, Pifferi F, Aujard F. Resveratrol in mammals: effects on aging biomarkers, age-related diseases, and life span. Ann N Y Acad Sci (2013) 1290:67–72. doi:10.1111/nyas.12214
152. Luo Y, Marx SO, Kiyokawa H, Koff A, Massagué J, Marks AR. Rapamycin resistance tied to defective regulation of p27Kip1. Mol Cell Biol (1996) 16(12):6744–51. doi:10.1128/MCB.16.12.6744
153. Gallo R, Padurean A, Jayaraman T, Marx S, Roque M, Adelman S, et al. Inhibition of intimal thickening after balloon angioplasty in porcine coronary arteries by targeting regulators of the cell cycle. Circulation (1999) 99(16):2164–70. doi:10.1161/01.CIR.99.16.2164
154. Garvey SM, Sinden DS, Schoppee Bortz PD, Wamhoff BR. Cyclosporine up-regulates Krüppel-like factor-4 (KLF4) in vascular smooth muscle cells and drives phenotypic modulation in vivo. J Pharmacol Exp Ther (2010) 333(1):34–42. doi:10.1124/jpet.109.163949
155. Zhu Y, Toshio T, Wang B, Kent A, Zhang M, Binde BYK, et al. Restenosis inhibition and re-differentiation of TGF β/Smad3-activated smooth muscle cells by resveratrol. Sci Rep (2017) 7:41916. doi:10.1038/srep41916
156. Gao D, Hao G, Meng Z, Ning N, Yang G, Liu Z, et al. Rosiglitzone suppresses angiotensin II-induced production of KLF5 and cell proliferation in rat vascular smooth muscle cells. PLoS One (2015) 10(4):e0123724. doi:10.1371/journal.pone.0123724
157. Khedkar SA, Sun X, Rigby AC, Feinberg MW. Discovery of small molecule inhibitors to Krüppel-like factor 10 (KLF10): implications for modulation of T regulatory cell differentiation. J Med Chem (2015) 58:1466–78. doi:10.1021/jm5018187
Keywords: Krüppel-like factor, Krüppel-like transcription factors, vascular inflammation, atherosclerosis, endothelial cells, vascular smooth muscle cells, macrophages
Citation: Sweet DR, Fan L, Hsieh PN and Jain MK (2018) Krüppel-Like Factors in Vascular Inflammation: Mechanistic Insights and Therapeutic Potential. Front. Cardiovasc. Med. 5:6. doi: 10.3389/fcvm.2018.00006
Received: 03 November 2017; Accepted: 17 January 2018;
Published: 05 February 2018
Edited by:
Masanori Aikawa, Harvard Medical School, United StatesReviewed by:
Ying Hu Shen, Baylor College of Medicine, United StatesXiao-feng Yang, Temple University, United States
Copyright: © 2018 Sweet, Fan, Hsieh and Jain. This is an open-access article distributed under the terms of the Creative Commons Attribution License (CC BY). The use, distribution or reproduction in other forums is permitted, provided the original author(s) and the copyright owner are credited and that the original publication in this journal is cited, in accordance with accepted academic practice. No use, distribution or reproduction is permitted which does not comply with these terms.
*Correspondence: Mukesh K. Jain, bXhqODRAY2FzZS5lZHU=