The neural mechanisms mediating the subjective perception of time are currently debated (
Eagleman et al., 2005
;
Wittmann and van Wassenhove, 2009
). Two key issues are (i) whether time perception functions via a dedicated system or by more general subsystems (
Karmarkar and Buonomano, 2007
;
Ivry and Schlerf, 2008
;
van Wassenhove, 2009
) and (ii) whether the duration of events is computed over distributed or localized brain structures (
Rubia and Smith, 2004
;
Buhusi and Meck, 2005
;
Craig, 2009a
;
Wittmann, 2009
). Perceptually, the estimation of duration is susceptible to contextual effects including, but not restricted to, the physical characteristics of the stimuli (
Fraisse, 1984
), their emotional valence (
Noulhiane et al., 2007
;
Droit-Volet and Gil, 2009
), and the attentional load with respect to task requirements (
Block and Zakay, 1997
).
Recent psychophysical studies have shown that the experienced duration of an event can be systematically altered using a number of different experimental techniques. Specifically, the duration of a deviant stimulus within a stream of standard events tends to be systematically overestimated (
Tse et al., 2004
;
van Wassenhove et al., 2008
). Three explanations have currently been put forth to account for this temporal illusion. First, the increase in subjective duration may directly result from an increase of attention directed to the deviant stimulus (
Tse et al., 2004
;
New and Scholl, 2009
). The second alternative is a “coding efficiency” view in which the greater amount of energy expenditure for the encoding of a deviant stimulus dilates experienced duration (
Eagleman and Pariyadath, 2009
). A third explanation suggests that temporal effects are mostly affected by the intrinsic dynamics of the stimulus (
Kanai et al., 2006
;
New and Scholl, 2009
). Of particular relevance here, looming signals (expanding discs) are associated with subjective time dilation whereas receding signals (shrinking discs) do not result in an altered experience of time (
van Wassenhove et al., 2008
). These findings converge with the observations that looming signals are particularly salient events (
Yantis and Egeth, 1999
) and are more powerful in drawing attention compared to other stimuli (
Franconeri et al., 2005
). Looming signals are an intrinsic threat cue for the organism (
Schiff et al., 1962
) and as such, may constitute a natural self-referential stimulus (
Northoff et al., 2006
). In the context of recent proposals suggesting that the experience of time is a self-referential process (
Craig, 2009b
;
Wittmann, 2009
) we implemented the looming disc task. If self-referential processing is related to time perception, the looming signals should provoke relative overestimation of duration.
Here, we exploited the fact that a difference of time experience is elicited by a looming and a receding stimulus and tested (i) which brain structures are involved in the temporal dilation effect and (ii) whether clear differential brain activation can be observed in these structures when experiencing (with looming stimuli) or not (with receding stimuli) a temporal dilation illusion. For this, we used a previously validated psychophysical paradigm (
van Wassenhove et al., 2008
) as the basis for an event-related functional magnetic resonance imaging (fMRI) study.
Participants
Twenty right-handed young adults (7 female/13 male); mean age: 26.1 (age range 18–42) participated in the timing task while undergoing fMRI. Subjects had corrected-to-normal vision, normal structural MRI scans, gave written consent and were compensated with $50 for participation in the study that lasted approximately 90 min. Five subjects were not included in the fMRI analysis. Four subjects did not perform the task correctly (inspecting the psychophysical response curves, these participants either responded stereotypically or had no systematic, reliable responses to the different targets) and one session was cancelled due to technical problems during recording. Hence, 15 subjects were effectively considered in the psychophysical and fMRI analyses. The experimental protocol was approved by the University of California, San Diego Human Research Protections Program (UCSD HRPP) and written informed consent was obtained from all participants.
Stimuli and Procedure
Prior to scanning, participants underwent psychophysical testing in which they were presented with a stream of five visual events consisting of three consecutive standard discs (STANDARD), one target (LOOMING, RECEDING or STEADY) and one last STANDARD disc (Figure
1
). The target was
always the fourth event in the stream of 500 ms standards and its duration was systematically varied in order to establish a precise psychophysical threshold based on psychometric discrimination curves. Participants had to judge the duration of the target in comparison to all STANDARD events in the trial by answering the question, “Is the target shorter or longer than the other events?”
Figure 1. Experimental Design. The pre-fMRI training session consisted of three conditions (LOOM, RECEDE, STEADY) whereas the fMRI session tested the two main conditions (LOOM, RECEDE). One trial consisted of a stream of five visual events, four standards and one target. All standards were static discs of ∼500 ms duration; all targets were presented at the fourth position in the stream and varied in duration. In the pre-fMRI session, a target could be a static (steady), a looming or a receding disc. In the fMRI session, a target was either looming or receding. The inter-stimulus intervals (ISI) and the inter-trial intervals (ITI) were all variable.
The stimuli consisted of gray disks centered on the monitor screen displayed on a black background. In the training session, the stimuli were displayed on laptop with an LCD screen (60 Hz refresh rate). In the fMRI experiment, the stimuli were delivered on a 19” Cathode Ray Tube monitor with a refresh rate of 85 Hz and projected onto a large screen placed outside the scanner. The screen was visible through a mirror placed in front of the participants’ eyes. From the subjects’ perspective, the standard (STANDARD) stimulus subtended 6.36 degrees square of visual angle; the looming and the receding visual stimuli consisted of the same gray disk changing in size from 6.36 to 16.85 degrees square of visual angle and from 16.85° to 6.36° of visual angle, respectively. The change in stimulus size was constant irrespective of duration. All stimuli were created using MatlabTM 7.1 (TheMathworks, Inc., Natick, MA, USA) and presented in conjunction with the Psychophysics Toolbox extensions (
Brainard, 1997
) for PC. Each trial consisted of a train of five visual stimuli (see Figure
1
). The fourth stimulus or target could be a looming, a receding or a steady disc in the training session and a looming or a receding disc in the fMRI session. The training was done to insure that the stimuli did in fact lead to the hypothesized perceptual effects and to insure that the receding signal lead to similar temporal illusion as a steady signal. Because it has the same dynamics as the looming signal the receding signal is the better control condition than the steady signal. Thus, we took the receding signal as the control condition in the fMRI study. The inter-stimulus intervals (ISI, between stimuli within a trial) were pseudo-randomly chosen from 500 to 1000 ms. Randomization of the ISI was done to prevent participants from using rhythmic cues in their duration judgments. The inter-trial intervals were pseudo-randomly chosen from 2 to 4 s in the training session and from 12 to 14 s in the fMRI session.
The training session took place just prior to the fMRI session: participants were given practice trials on three experimental conditions (LOOM, RECEDE, STEADY). In both the training and the fMRI sessions, a two-alternative forced choice paradigm was used and participants had to judge whether the target (fourth stimulus) was “shorter” or “longer” than all other stimuli in the trial (the first, second, third and fifth). Subjects were instructed to press the button after the last stimulus of the trial had appeared. Due to hardware constraints (refresh rate) slight differences in standard and target durations were chosen in the training and the fMRI sessions: in the training session, standard stimuli were 500 ms and the targets were ±23.3%, ±10% or ±3.3% of this standard duration; in the fMRI session, standard stimuli were 494 ms and the targets were ±23.8%, ±7.1% or ±2.4% of the standard duration (Table
1
). All durations were consistently checked with an oscilloscope and a photodiode directly applied onto the monitor (training session) or screen (fMRI session) as well as recorded on a trial-basis for each session. No significant jitters or variations in display duration were observed over the course of the study.
Table 1. Stimulus characteristics. Stimulus duration for the pre-fMRI and the fMRI session for the standard stimulus and the target stimulus, which varied with the presentation of six different durations.
In the training session, the LOOM and RECEDE data were used in comparison to the STEADY data to confirm the existence of illusory time distortions for LOOM. In the fMRI session, only the LOOM and RECEDE conditions were tested using a similar design. In the fMRI session, the extreme target durations (380 and 620 ms) were presented twice per run and all other target durations were presented three times per run. There was a total of four fMRI runs, hence participants were tested for a total of 128 trials (eight trials per extreme target duration per condition (LOOM, RECEDE) and 12 trials for all other durations per condition).
Psychophysical Data Analysis
Percentage of “longer” responses and reaction times were averaged for each condition and target duration resulting in individual psychometric curves. The grand average performance data are provided in Figures
2
A,B and the grand average reaction times in Figures
2
C,D for the training and fMRI sessions, respectively. Each individual psychometric function was fitted to a normal cumulative distribution function using a non-linear least-square data fitting procedure. Individual point-of-subjective-equality (PSE; the duration at which subjects judge the target stimulus to equal the duration of the standards) was determined at the 50% crossing for each condition. Figures
2
E,F reports the grand average PSE for each condition in the training and fMRI sessions, respectively. Additionally, individuals’ measures of d′ (sensitivity) and β (bias) were computed based on classic signal detection theory for 2AFC paradigms for each condition of the fMRI session:
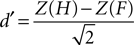
and
where H is the hit rate (probability of answering “longer” when the target is longer than the standards) and F is the false alarm rate (probability of answering “longer” when the target is shorter than the standards); hit rates and false alarm rates were converted into their respective Z-scores. Statistical analyses on thresholds, sensitivity and bias measures consisted in repeated measures ANOVA and paired t-tests performed using SPSS 14.0 (SPSS Inc., Chicago, IL, USA) unless otherwise specified. All statistical measures were Greenhouse–Geisser corrected based on a Mauchly’s test of sphericity.
Figure 2. Psychophysical results of pre-fMRI training session (left column) and of the fMRI session (right column): Percent longer responses (pre-fMRI = (A), fMRI = (B)), reaction time (pre-fMRI = (C), fMRI = (D)), point of subjective equality (pre-fMRI = (E), fMRI = (F)).
fMRI Acquisition and Analysis
Participants were scanned in a 3T GE Sigma scanner using an 8-channel head array coil. Each scanning session consisted of a three-plane scout scan, a sagittally acquired spoiled gradient recalled (SPGR) sequence for acquiring T1-weighted images (FOV 25 cm; matrix: 192 × 256; 172 slices; thickness: 1 mm; TR: 8 ms; TE: 3 ms; flip angle: 12°) and four T2*-weighted axially acquired echo-planar imaging (EPI) scans to measure BOLD functional activity. The parameters for the EPI scans were 3.43 × 3.43 × 2.6 mm with a 1.4-mm gap, TR = 2 s, TE = 32 ms, flip angle of 90°, and 30 slices (whole brain). Cushions were arranged around the head and neck to maximize comfort and minimize motion. The data were preprocessed, normalized to Talairach coordinates and analyzed with the Analysis of Functional NeuroImages (AFNI) software package. For preprocessing, voxel time series data were interpolated to correct for non-simultaneous slice acquisition within each volume and corrected for 3-D motion. Motion-corrected voxel time series data were visually inspected to remove large movement artifacts. Preprocessed time series data for each individual were analyzed using a multiple regression model containing nine regressors. Specifically, three response regressors were generated for each of the three stimuli (two for the targets, LOOM, RECEDE and one for the STANDARDs) in the timing task, that is, the boxcar regressors covered the presentation times (around 500 ms) of the stimuli. For the standard condition it covered the four presentation times of the standard stimulus and for the looming and receding condition it covered these respective stimuli. It has to be noted that stimuli presented with an ISI of less than 2 s are assumed to have activations that add in a non-linear way (especially for the activations representing the standard stimuli), and thus may be poorly estimated by a linear model. However, although there may be some non-linear influences, temporal events as short as several hundred milliseconds can be resolved using jittered event timing and slice timing alignment (especially for the decisive contrast between looming and receding). Three regressors were used to model residual motion (in the roll, pitch, and yaw directions). Additional three nuisance regressors were used: The baseline and linear drift were added to account for overall signal intensity changes. The white matter regressor was generated in the following manner: (1) a grey-white matter mask was generated based on the associated high level anatomical scan, (2) this mask was down-sampled to the resolution of the echoplanar image, (3) the mask was used to obtain an overall average across all white matter voxels for each time point, (4) the white matter regressor was normalized, i.e. divided by the range of the values. The goal of this approach was to eliminate signal fluctuations that are not due to BOLD-signal changes but are due to undulating echoplanar signal variation. Each of the three stimulus regressors was convolved with a modified gamma variate function modeling a prototypical hemodynamic response. The AFNI program 3dDeconvolve was used to calculate the estimated voxel-wise response amplitude and function for each regressor and to generate the contrasts: LOOM – STANDARD, RECEDE – STANDARD, LOOM – RECEDE. All first-level analyses were carried out using a multiple regressor approach, which does not consider the inherent autocorrelation of the time series data. Thus, individual level statistics were not used and have to be considered with care as they overestimate the significance of the model. A Gaussian filter with full-width half-maximum (FWHM) of 6 mm was applied to the voxel-wise percent signal change data to account for individual variations of the anatomical landmarks. Both structural and echoplanar images from each subject were normalized to Talairach space. Voxel-wise
t-tests where applied to test for activation differences between the stimulus contrasts LOOM > STANDARD, RECEDE > STANDARD, LOOM > RECEDE. Activations are coded by yellow to red voxels (activation) and blue (deactivation) and superimposed on the average of anatomical images of the 15 included subjects. A threshold adjustment method based on Monte-Carlo simulations was used in a whole-brain cluster analysis (
Forman et al., 1995
). Based on these simulations, a voxel-wise
a priori probability of 0.001 (0.05) results in a corrected cluster-wise activation probability of 0.001 (0.05) and a voxel-wise
a posteriori probability of 0.00000085 (0.00008491) if a minimum volume of 512 μl (1440 μl) and a connectivity radius of 4.0 mm is considered [corresponding to 13 (30) connected voxels].
Pre-fMRI Session – Behavioral
The psychophysical results replicated prior findings (
van Wassenhove et al., 2008
): a temporal dilation effect was observed only for LOOM targets. The evaluation of duration for RECEDE targets did not significantly differ from that of STEADY targets. Grand average data are provided in Figure
2
A (performance: % detection of target stimulus as longer), Figure
2
C (reaction times) and Figure
2
E (PSE). Statistical comparisons of the PSE revealed significant differences when comparing LOOM with STEADY [
t(1,10) = −3.846,
P < 0.003), LOOM with RECEDE [
t(1,11) = −5.269,
P < 0.0001] but not RECEDE with STEADY [
t(1,12) = −1.291,
P = 0.221]. Thus, on average, a steady target of 527 ms, a looming target of 419 ms and a receding target of 502 ms were all perceived as having the same duration as a standard event of 500 ms (the PSE, Figure
2
E). During this training session participants showed significant differences in reaction times (RT): repeated measures ANOVA with factors of condition (LOOM, RECEDE and STEADY) and duration showed significant main effects of condition [
F(2,30) = 28.568,
P < 0.0001], duration [
F(5,75) = 0.288,
P < 0.001], and an interaction between condition and duration [
F(10,150) = 5.941,
P < 0.0001]. Figure
2
C illustrates that the difference is mainly due to slower reaction times for target durations longer than the standard duration in the STEADY condition. Additional paired
t-tests indeed showed a significant effect of condition in the longer duration range when comparing LOOM and STEADY [
t(1,15) = −7.948,
P < 0.0001] and RECEDE and STEADY [
t(1,15) = −5.550,
P < 0.0001]. There were no differences in RT between the LOOM and RECEDE conditions across durations, suggesting that the STEADY task was the most difficult condition and conversely, that task difficulty is comparable for the LOOM and RECEDE conditions; thus, these two conditions were selected for the fMRI session. On the basis of these results, the same paradigm was used to contrast LOOM (illusory duration) with RECEDE (no illusory duration) during fMRI to preserve equivalent dynamic properties in early sensory representations.
fMRI Session – Behavioral
In the fMRI session, the PSE obtained in LOOM and RECEDE conditions revealed a significant effect of condition [
t(1,13) = −7.365,
P < 0.0001] (Figures
2
B,F). As in the pre-fMRI training session (Figure
2
A) a time dilation effect was observed for the LOOM but not for the RECEDE condition. On average, a 406-ms looming and a 511-ms receding events were subjectively equivalent to a 490 ms STANDARD (Figure
2
F). Participants’ reaction times in the fMRI did not significantly differ (Figure
2
D) across conditions [
F(1,15) = 0.981,
P = 0.338] or durations [
F(5,75) = 1.493,
P = 0.202] nor with their interaction [
F(2.056,30.84) = 2.360,
P = 0.110].
Further analyses, based on classic signal detection theory, were performed on individual data to assess participants’ sensitivity (d′) and bias (β) in each experimental condition. The condition (LOOM versus RECEDE) marginally affected the d′ [t(1,15) = −2.271, P < 0.038]: participants’ sensitivity was on average slightly smaller in the LOOM than in the RECEDE condition. Conversely, the condition greatly affected participants’ β values [t(1,15) = −7.772, P < 0.0001] with a much larger bias in the LOOM than in the RECEDE condition. These results show that the evaluation of duration was more variable in the LOOM than in the RECEDE condition: this result suggests that additional mechanisms not specifically involved in time estimation may be involved in the illusion – e.g. general mechanisms of attention or affect (see Discussion).
fMRI Session – Brain Activation
We contrasted the LOOM versus STANDARD and RECEDE versus STANDARD discs of the fMRI task in order to detect areas of activation that are generally involved in the timing of events. For both contrasts, similar brain activations were detected (
P < 0.001, corrected; see Figure
3
), namely a stronger activation was observed for the dynamic targets (LOOM, RECEDE) in (i) the left insula and the adjacent claustrum and lentiform nucleus, (ii) the anterior cingulate cortex, (iii) the right middle frontal cortex, and (iv) left and right superior frontal regions (Tables
2 and
3
). Additionally, activation was visible for the RECEDE stimulus as compared to the STANDARD in the right insula and the cingulate cortex bilaterally. These areas, especially the anterior insula, the basal ganglia, and the frontal gyri have repeatedly been identified as involved in the processing of duration (
Rubia and Smith, 2004
;
Wittmann, 2009
) and are thus presumably involved in the temporal estimation of the target stimuli.
Figure 3. fMRI: LOOM > STANDARD, RECEDE > STANDARD. Brain regions with significantly greater activation (yellow to red voxels) for the LOOM > STANDARD (left) and the RECEDE > STANDARD (right) contrasts. Activation is depicted in axial (row 1 and 2 of brain slices) and sagittal views (row 3 and 4); percent signal change is plotted (mean; SEM) as obtained through t-tests (P < 0.001, corrected). Numbers in slices correspond to numbers in bar charts representing average percent signal change in specific regions of interest.
Table 2. LOOM > STANDARD. Regions of significant activation (P < 0.001,corrected) for the contrast looming > standard signal.
Table 3. RECEDE > STANDARD. Regions of signifi cant activation (P < 0.001, corrected) for the contrast receding > standard signal.
Brain activation differences between the LOOM and the RECEDE conditions should reveal neural correlates for the time dilation effect. The goal of our fMRI study was to specifically test illusory temporal perception while minimizing physical differences of the stimuli. Hence, we chose two dynamic stimuli, looming and receding, which lead to illusion and no illusion respectively. The factors of size, motion, integrated luminance, etc. are identical in the two conditions. The only difference between the conditions is that the stimulus is moving towards (producing subjective time dilation) versus moving away from the viewer (not leading to a temporal illusion). In Figure
2
B, the large area between the curves for the LOOM and the RECEDE condition reflects the magnitude of the subjective looming effect, indicating a large subjective difference between these two conditions. Accordingly, a contrast between fMRI signals in the LOOM and the RECEDE conditions was obtained (Figure
4
,
P < 0.05, corrected; see also Table
4
; Figure
5
). Significantly stronger activity for LOOM versus RECEDE (yellow) is present in the left medial frontal (including the left mid-cingulate) and the posterior cingulate and pre-cuneus regions (
z = 43,
x = −9), areas which have been associated with self-referential episodic memory and the so-called “default network” (
Raichle et al., 2001
;
Johnson et al., 2005
;
Seeley et al., 2009
), together with small portions of the left superior frontal and middle frontal areas (
z = 11). By contrast, stronger activity for RECEDE versus LOOM (in blue) was observed in the left anterior insula, the left dorsal thalamus (
z = 11) and the anterior cerebellum (
z = −5).
Figure 4. fMRI: LOOM > RECEDE. Brain regions with significantly greater activation for the LOOM > RECEDE (yellow to red voxels) and the RECEDE > LOOM (blue voxels) contrasts. Activation is depicted in axial (upper row) and sagittal views (lower rows); percent signal change is plotted (mean; SEM) as obtained through t-tests (P < 0.05, corrected). Numbers in slices correspond to numbers in bar charts representing average percent signal change in specific regions of interest.
Table 4. LOOM > RECEDE, RECEDE > LOOM. Regions of signifi cant activation (P = 0.05, corrected) for the contrast looming > receding signal and receding > looming signal.
Figure 5. fMRI: LOOM > RECEDE. Percent signal change (mean; SEM) in brain regions with significantly greater activation for the LOOM > RECEDE contrast (upper row: L medial, L superior frontal) and the RECEDE > LOOM contrast (lower row: R cerebellum, L anterior insula, R thalamus) for the three stimulus conditions (LOOM, RECEDE, STANDARD).
This study is a first attempt, using neuroimaging techniques, at characterizing brain regions implicated in a temporal illusion. The psychophysical task that was used evoked a robust temporal dilation effect, replicating prior findings (
van Wassenhove et al., 2008
). Since a significant time dilation effect was only elicited in the LOOM condition and not in the RECEDE condition, we suggest that it is not the occurrence of a differing or oddball target stimulus
per se that evokes an overestimation of duration but the specific occurrence of a looming signal that is virtually moving towards the viewer. The looming disc elicits self-referential processes that potentially signal a threat (
Northoff et al., 2006
). In contrast to receding stimuli, looming signals are a class of particularly salient stimuli (
Yantis and Egeth, 1999
) and receding signals do not signal a threat (moving away). Thus, the intrinsic features of the stimulus critically affect subjective time distortions (
van Wassenhove et al., 2008
). Concerning the analysis of the sensitivity and bias parameters (d′ and β, respectively), the evaluation of duration was clearly more variable in the LOOM (smaller sensitivity and larger bias) as compared to the RECEDE condition. These findings indicate that additional mechanisms not specifically involved in duration discrimination may be involved in the temporal illusion. These could be mechanisms of affect that are related to the “threatening” nature of the looming disc (see below).
There is yet no conclusive answer as to what kind of time keeping mechanism is implemented in the brain (
van Wassenhove, 2009
;
Wittmann, 2009
). Cognitive models, however, which assume that an internal clock with a pacemaker produces subjective time units have been influential in interpreting human time perception and animal timing behavior (
Gibbon et al., 1984
;
Zakay and Block, 1997
). In these models, duration is defined as a function of accumulated units over a time span. Two mechanisms can increase the number of pulses in an assumed accumulator and thus expand subjective duration: (1) increased attention to time leads to an accumulation of more pulses over a time span, (2) an arousal-related increase in pulse rate (a faster clock rate) leads to a greater accumulation of temporal units (
Burle and Casini, 2001
;
Wittmann and Paulus, 2008
). High-arousing acoustic or visual stimuli with emotional content, for example, lead to an overestimation of duration which is interpreted as resulting from an increased pacemaker rate (
Noulhiane et al., 2007
;
Droit-Volet and Gil, 2009
). In line with this notion one could also interpret temporal dilation effects in the context of an increased pacemaker rate due to the occurrence of the looming signal that, through its movement towards the perceiver, has an inherently emotional component to it.
The fMRI findings indicate that similar regions of the brain were activated in the LOOM and the RECEDE conditions as contrasted with the STANDARD (insula, anterior cingulate cortex, basal ganglia, dorsolateral pre-frontal cortex) and the activated regions are consistent with the results of prior studies of subjective time perception (
Lewis and Miall, 2003
;
Rubia and Smith, 2004
;
Wittmann, 2009
). In the decisive contrast addressing the temporal dilation effect (LOOM versus RECEDE), positive activation for the LOOM condition was detected in left-sided midline structures (including mid- and posterior cingulate cortex) as well as areas of the left middle and superior frontal cortex. Positive activation for the RECEDE condition (deactivation in the mentioned contrast) was shown for the left anterior insula, the right thalamus and the right cerebellum.
Our considerations concerning self-related processes due to an approaching object during the LOOM condition are relevant to the observed activation in the mid- and posterior cingulate regions of the left hemisphere for the decisive LOOM versus RECEDE contrast (the contrast that represents the time dilation effect). Several studies have shown that cortical mid and posterior midline structures are associated with the default mode of brain function (the “default network”;
Raichle et al., 2001
) and, importantly, with the processing of self-related stimuli (
Kelley et al., 2002
). The medial prefrontal cortex is often found during resting conditions when individuals presumably engage in self-relevant thoughts and beliefs (
Wicker et al., 2003
). Moreover, direct appraisals as compared to reflected appraisals recruit regions associated with a first-person perspective, namely the mid and posterior cingulate cortex (
Ochsner et al., 2005
). The involvement of midline structures in the temporal perception of looming (but not receding) signals could be related to the “potential threat” carried by these signals to the perceiver (
Schiff et al., 1962
) i.e. increased self-referential processing of looming compared to receding stimuli. Of course, to date, this interpretation is speculative. Further empirical data (e.g., using physiological recordings) will have to show that the looming stimuli indeed are experienced as posing a “threat”.
One finding is the asymmetry of activation in the “core control network”, namely the left sided activation of the anterior insula in the RECEDE versus LOOM condition. This fits with a proposal that subjective time perception involves an inherently asymmetric representation of emotional feelings in the anterior insular cortex (
Craig, 2008
). The insular cortex of primates has been identified as the primary receptive area for sensory activity representing the physiological condition of the body, and re-representations of this homeostatic afferent activity has been proposed to provide the basis for subjective awareness of emotional states (
Craig, 2009b
). In this model, the anterior insula and the anterior cingulate are conjointly engaged during task performance as complementary limbic sensory and motor regions that work together, and recent evidence suggesting that these two regions together form a “core control network” supports this hypothesis (
Raichle et al., 2001
;
Johnson et al., 2005
;
Seeley et al., 2009
). The core of this model is a cinemascopic representation of the “sentient self” indexed by an endogenous time base that is sensitive to emotional salience. This model suggests a neuroanatomical explanation for forebrain emotional asymmetry (
Craig, 2008
), in which the left forebrain is associated predominantly with parasympathetic activity (with approach, safety, positive affect), and the right forebrain is associated predominantly with sympathetic activity (with arousal, danger, negative affect). Thus, the activation in the left anterior insula for the RECEDE condition could be related to the different emotional feelings associated with the receding (non-threatening) stimuli which are virtually moving away from the perceiver. The strong relationship between affect and time is well documented in many empirical studies. Notably, strong emotional stimuli are overestimated in relation to more neutral stimuli (
Noulhiane et al., 2007
;
Droit-Volet and Gil, 2009
). Our interpretation, however, is weakened by the fact that no right-sided anterior insula activation (related to negative affect) was found in the LOOM condition, which would have been predicted by this model.
The underlying neural mechanisms of temporal distortions are incompletely understood and this fMRI study is but a first attempt to capture the underlying neural mechanisms of a duration illusion. Although we used a robust psycho-physical paradigm that has been probed and tested previously and that elicits a reliable illusion, other procedures may lead to more pronounced neuroimaging results. Future experimental designs will likely use 3-D objects for more realistic stimulus rendering or will probe for auditory and cross-modal effects. A more realistic stimulation (i.e. a 3-D object moving towards the observer) would be more emotionally salient and could lead to stronger brain activation differences between the LOOM and RECEDE conditions. Despite these shortcomings, our first findings are noteworthy in relating self-related processes, emotion and time perception (
Craig, 2009a
;
Wittmann, 2009
).
Conflict of Interest Statement
The authors declare that the research was conducted in the absence of any commercial or financial relationships that could be construed as a potential conflict of interest.
Marc Wittmann and Martin Paulus are supported by a grant from the National Institutes of Health (NIH), National Institute of Drug Abuse (NIDA) (1R03DA020687-01A1) and by a grant from the Kavli Institute for Brain and Mind (KIBM 07-33).
Block, R. A., and Zakay, D. (1997). Prospective and retrospective duration judgments. A meta-analytic review.
Psychon. Bull. Rev. 4, 184–197.
Brainard, D. H. (1997). The psychophysics toolbox.
Spat. Vis. 10, 433–436.
Buhusi, C. V., and Meck, W. H. (2005). What makes us tick? Functional and neural mechanisms of interval timing.
Nat. Rev. Neurosci. 6, 755–765.
Burle, B., and Casini, L. (2001). Dissociation between activation and attention effects in time estimation: implications for internal clock models.
J. Exp. Psychol. Hum. Percept. Perform. 27, 195–205.
Craig, A. D. (2008). Interoception and emotion: a neuroanatomical perspective. In Handbook of Emotion, M. Lewis, J. M. Haviland-Jones and L. F. Barrett, eds (New York, Guilford), pp. 272–288.
Craig, A. D. (2009a). Emotional moments across time: a possible neural basis for time perception in the anterior insula.
Philos. Trans. R. Soc. Lond., B, Biol. Sci. 364, 1933–1942.
Craig, A. D. (2009b). How do you feel – now? The anterior insula and human awareness.
Nat. Rev. Neurosci. 10, 59–70.
Droit-Volet, S., and Gil, S. (2009). The time-emotion paradox.
Philos. Trans. R. Soc. Lond., B, Biol. Sci. 364, 1943–1953.
Eagleman, D., and Pariyadath, V. (2009). Is subjective duration a signature for coding efficiency?
Philos. Trans. R. Soc. Lond., B, Biol. Sci. 364, 1841–1851.
Eagleman, D. M., Tse, P. U., Buonomano, D., Janssen, P., Nobre, A. C., and Holcombe, A. O. (2005). Time and the brain: how subjective time relates to neural time.
J. Neurosci. 25, 10369–10371.
Forman, S. D., Cohen, J. D., Fitzgerald, M., Eddy, W. F., Mintun, M. A., and Noll, D. C. (1995). Improved assessment of significant activation in functional magnetic resonance imaging (fMRI): use of a cluster-size threshold.
Magnet. Reson. Med. 33, 636–647.
Fraisse, P. (1984). Perception and estimation of time.
Annu. Rev. Psychol. 35, 1–36.
Franconeri, S. L., Hollingworth, A., and Simons, D. J. (2005). Do new objects capture attention?
Psychol. Sci. 16, 275–281.
Gibbon, J., Church, R. M., and Meek, W. H. (1984). Scalar timing in memory. In Annals of the New York Academy of Sciences, Vol. 423, Timing and Time Perception, J. Gibbon and L. Allan, eds (New York, New York Academy of Sciences), pp. 52–77.
Ivry, R. B., and Schlerf, J. E. (2008). Dedicated and intrinsic models of time perception.
Trends Cogn. Sci. 12, 273–280.
Johnson, S. C., Schmitz, T. W., Kawahara-Baccus, T. N., Rowley, H. A., Alexander, A. L., Lee, J., and Davidson, R. J. (2005). The cerebral response during subjective choice with and without self-reference.
J. Cogn. Neurosci. 17, 1897–1906.
Kanai, R., Paffen, C. L. E., Hogendoorn, H., and Verstraten, F. A. J. (2006). Time dilation in dynamic visual display.
J. Vision 6, 1421–1430.
Karmarkar, U. R., and Buonomano, D. V. (2007). Timing in the absence of clocks: encoding time in neural network states.
Neuron 53, 427–438.
Kelley, W. M., Macrae, C. N., Wyland, C. L., Caglar, S., Inati, S., and Heatherton, T. F. (2002). Finding the self? An event-related fMRI study.
J. Cogn. Neurosci. 14, 785–794.
Lewis, P. A., and Miall, R. C. (2003). Distinct systems for automatic and cognitively controlled time measurement: evidence from neuroimaging.
Curr. Opin. Neurobiol. 13, 250–255.
New, J. J., and Scholl, B. J. (2009). Subjective time dilation: spatially local, object-based, or a global visual experience.
J. Vision 9, 1–11.
Northoff, G., Heinzel, A., de Greck, M., Bermpohl, F., Dobrowolny, H., and Panksepp, J. (2006). Self-referential processing in our brain: a meta-analysis of imaging studies on the self.
Neuroimage 31, 440–457.
Noulhiane, M., Mella, N., Samson, S., Ragot, R., and Pouthas, V. (2007). How emotional auditory stimuli modulate time perception.
Emotion 7, 697–704.
Ochsner, K. N., Beer, J. S., Robertson, E. R., Cooper, J. C., Gabrieli, J. D., Kihsltrom, J. K., and D’Esposito, M. (2005). The neural correlates of direct and reflected self-knowledge.
Neuroimage 28, 797–814.
Raichle, M. E., MacLeod, A. M., Snyder, A. Z., Powers, W. J., Gusnard, D. A., and Shulman, G. L. (2001). A default mode of brain function.
Proc. Natl. Acad. Sci. U.S.A. 98, 676–682.
Rubia, K., and Smith, A. (2004). The neural correlates of cognitive time management: a review.
Acta Neurobiol. Exp. 64, 329–340.
Schiff, W., Caviness, J. A., and Gibson, J. J. (1962). Persistent fear responses in rhesus monkeys to the optical stimulus of “looming”.
Science 136, 982–983.
Seeley, W. W., Crawford, R. K., Zhou, J., Miller, B. L., and Greicius, M. D. (2009). Neurodegenerative diseases target large-scale human brain networks.
Neuron 62, 42–52.
Tse, P. U., Intriligator, J., Rivest, J., and Cavanagh, P. (2004). Attention and the subjective expansion of time.
Percept. Psychophys. 66, 1171–1189.
van Wassenhove, V. (2009). Minding time – an amodel representational space for time perception.
Philos. Trans. R. Soc. Lond., B, Biol. Sci. 364, 1815–1830.
van Wassenhove, V., Buonomano, D. V., Shimojo, S., and Shams, L. (2008). Distortions of subjective time perception within and across senses.
PLoS ONE 3, e1437. doi: 10.1371/journal.pone.0001437.
Wicker, B., Ruby, P., Royet, J. P., and Fonlupt, P. (2003). A relation between rest and the self in the brain?
Brain Res. Brain Res. Rev. 43, 224–230.
Wittmann, M. (2009). The inner experience of time.
Philos. Trans. R. Soc. Lond., B, Biol. Sci. 364, 1955–1967.
Wittmann, M., and Paulus, M. P. (2008). Decision making, impulsivity, and time perception.
Trends Cogn. Sci. 12, 7–12.
Wittmann, M., and van Wassenhove, V. (2009). The experience of time: neural mechanisms and the interplay of emotion, cognition and embodiment.
Philos. Trans. R. Soc. Lond., B, Biol. Sci. 364, 1809–1813.
Yantis, S., and Egeth, H. E. (1999). On the distinction between visual salience and stimulus-driven attentional capture.
J. Exp. Psychol. Hum. Percept. Perform. 25, 661–676.
Zakay, D., and Block, R. A. (1997). Temporal cognition.
Curr. Dir. Psychol. Sci. 6, 12–16.