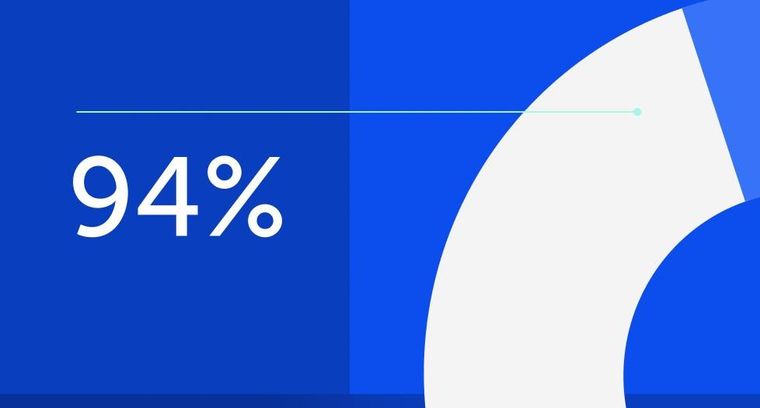
94% of researchers rate our articles as excellent or good
Learn more about the work of our research integrity team to safeguard the quality of each article we publish.
Find out more
ORIGINAL RESEARCH article
Front. Virol., 23 September 2022
Sec. Translational Virology
Volume 2 - 2022 | https://doi.org/10.3389/fviro.2022.953208
This article is part of the Research TopicWomen in Translational Virology 2022View all 5 articles
The influenza A virus (IAV) 2009 H1N1 pandemic was associated with an increased risk of maternal mortality, preterm birth, and stillbirth. The underlying mechanism for severe maternal lung disease and stillbirth is incompletely understood, but IAV infection is known to activate innate immunity triggering the release of cytokines. Elucidating the impact of progesterone (P4), a key hormone elevated in pregnancy, on the innate immune and inflammatory response to IAV infection is a critical step in understanding the pathogenesis of adverse maternal-fetal outcomes. IAV H1N1 pdm/09 was used to infect cell lines Calu-3 (lung adenoma) and ACH-3P (extravillous trophoblast) with or without P4 (100 nM) at multiplicity of infections (MOI) 0, 0.5, and 3. Cells were harvested at 24 and 48 hours post infection (hpi) and analyzed for cytopathic effects (CPE), replicating virus (TCID50), cytotoxicity (Lactate Dehydrogenase (LDH) assay), and NLRP3 inflammasome activation (caspase-1 activity, fluorometric assay). Activation of antiviral innate immunity was quantified (RT-qPCR, Luminex) by measuring biomarker gene and protein expression of innate immune activation (IFIT1, IFNB), inflammation (IL6), interferon signaling (MXA), chemokines (IL-8, IL-10). Both Calu-3 and ACH-3P were highly permissible to IAV infection at each timepoint as demonstrated by CPE and recovery of replicating virus. In Calu-3, progesterone treatment was associated with a significant increase in cytotoxicity, increased gene expression of IL6, and increased protein expression of IFN-β, IL-6, and IL-18. Conversely, in ACH-3P, progesterone treatment was associated with significantly suppressed cytotoxicity, decreased gene expression of IFNB, IL6 and IL1B, and increased protein expression of IFN-β and IL-6. In both cell lines, caspase-1 activity was significantly decreased after progesterone treatment, indicating NLRP3 inflammasome activation was not underlying the higher cell death in Calu-3. In summary, these data provide evidence that progesterone plays a dual role by ameliorating viral infection in the placenta but exacerbating influenza A virus-associated injury in the lung through nongenomic modulation of the innate immune response.
Ms. Miranda Li graduated from Columbia College at Columbia University in the City of New York with a BA in Biological Sciences in 2022. She is now a first-year medical student at the University of Washington School of Medicine in Seattle, Washington. Her research experience at the University of Washington under the mentorship of Dr. Kristina Adams Waldorf has opened her eyes to the fascinating field of translational virology, especially to the vulnerability of pregnant women to infectious diseases. She has spent several summers and part of the COVID-19 pandemic taking classes remotely while working in the Adams Waldorf laboratory.
Dr. Adams Waldorf is a Professor of Obstetrics and Gynecology and Adjunct Professor in Global Health at the University of Washington. Her research experience in virology began as an undergraduate. Although she went to medical school and trained as an obstetrician-gynecologist, a deep interest in pathogens and host response never left her. In the last 20 years, she has built a program to study the pathogenesis of infectious diseases in pregnancy, as well as the efficacy and safety of therapeutics designed to protect the mother and the fetus. Investigating how infectious diseases exploit the pregnancy-associated changes in maternal immunity that enable a fetus to grow and avoid rejection will always be central to her research program. As a mentor, she strives to meet trainees at their level, reduce feelings of “imposter syndrome” and bring them into the fascinating world of science that can be so rewarding. Her goal is to foster resilience in her trainees so that they can persevere in the face of the challenges and disappointments that occur regularly on the bench and in academia. Seeing a trainee succeed is one of the most rewarding aspects of her career.
Influenza A virus infection in pregnancy is associated with exacerbated maternal lung disease and a spectrum of adverse fetal outcomes (1). An increased risk for maternal death, hospitalization, preterm birth, perinatal mortality, and stillbirth following an IAV infection in pregnancy has been documented in many studies from both high- and middle-income countries (2–6). Maternal mortality from IAV can be significant and was estimated at 27% in the 1918 IAV pandemic (3). Approximately half of the fatal cases in reproductive-aged women during the 1957 IAV H2N2 pandemic were among pregnant individuals (7). More recently, in a case series from the 2009 H1N1 pandemic, 22% of hospitalized pregnant women with IAV infection required intensive care and 8% died (8). Stillbirth and perinatal mortality are also increased after IAV infection, as demonstrated in a United Kingdom national cohort of hospitalized pregnant women during the 2009 H1N1 pandemic (2). Mechanistically, the pathogenesis of exacerbated maternal lung disease and stillbirth are poorly understood, in part due to the complex physiologic changes in pregnancy that alter immunobiology.
The lung is the major target organ of IAV. Human lung adenocarcinoma cell line Calu-3 has been used as a well-characterized model of proximal airway epithelium to assess the innate immune response in the lung to respiratory viruses (9, 10). In IAV-infected primary lung epithelial cells, the type I IFNs activated the NRLP3 inflammasome (11). In several studies, pregnant mice were shown to have higher levels of inflammatory cytokines in the lung, more severe disease, greater mortality and lung pathology than non-pregnant mice (12–14). In considering pathogenesis of viral infections in pregnancy, it is also critical to study the placenta. The placenta is a complex and immune-rich organ that represents the gateway between the fetus and the mother. Several placental cell lines have demonstrated susceptibility and permissively to IAV infection in vitro. In the first trimester human trophoblast cell lines HTR-8/SVneo and Swan71 infected with H1N1/09, minimal viral release was noted, but IAV viral proteins and RNA were detected by immunofluorescence assay and PCR (15). H3N2 infection of the same cell lines induced rapid apoptosis and viral replication, indicating a virus-specific difference in placental cell line susceptibility to IAV-induced CPE (16). This evidence suggests that the placenta may play a critical, previously overlooked role in maternal influenza infection.
Hormones are thought to play a key role in impairing maternal adaptive and innate immunity, which helps to protect the fetus from rejection (17). Whether hormonal changes in pregnancy may impair antiviral innate immune responses to IAV in the lung and placenta are unknown. Progesterone is a key immunomodulating hormone in pregnancy and reaches levels 10-30-fold higher in the third trimester than in non-pregnant adults. Although progesterone is known to downregulate innate and adaptive immunity, the extent to which it modulates immunity after IAV infection in the maternal lung or placenta is poorly understood (17, 18). The impact of progesterone modulation on the NLRP3 inflammasome activation is an essential component of the IAV innate immune response and processes pro-caspase 1, pro-IL-1β, and pro-IL-18 into their active forms and has been implicated in IAV infection-mediated stillbirth and fetal injury (19–21). The impact of progesterone modulation on the NLRP3 inflammasome has not been studied in IAV but was observed to reduce the expression of inflammasome components in a transient focal rat model of ischemia in neurons (22). A blunted innate immune response to IAV in pregnancy could lead to delayed induction of adaptive immunity and may increase susceptibility to severe lung disease following IAV infection.
The study objective was to determine the impact of progesterone on IAV targets in pregnancy, namely the lung and placenta, which were modeled by cell lines (Calu-3, ACH-3P). Investigating the role that progesterone plays in the innate immune response to IAV infection during pregnancy is key to more completely understanding why pregnant women are more prone to developing severe lung disease and dying at higher rates than non-pregnant women from influenza infections (3, 6, 7). This study provides insight into the role of these responses in the pathogenesis of maternal disease and adverse perinatal outcomes.
Madin-Darby canine kidney (MDCK) cells (ATCC), Calu-3 cells (ATCC), and ACH-3P cells were cultured in Dulbecco’s modified Eagle’s medium (DMEM) supplemented with 10% fetal bovine serum (FBS), 25 mM HEPES, 100 U/mL penicillin, and 100 μg/mL streptomycin. All cell lines were incubated at 37°C and 5% CO2 and confirmed to be free of mycoplasma with the MycoAlert™ mycoplasma detection kit (Lonza, LT07-318).
Influenza A H1N1 virus (CA/09) was obtained from the Fuller Lab (23). Sub-confluent monolayers of MDCK cells in T150 flasks were washed with PBS and infected at MOI 0.5 (5 mL serum-free MEM) at 37°C and 5% CO2. After 1 hour, the media was removed, cells were washed with 1X PBS and media replaced. The media was harvested at 36 hours and centrifuged at room temperature to clear cell debris (1800 rpm for 5 minutes and then at 3000 rpm for 10 minutes). Virus stock was frozen at -80°C in 1 mL aliquots. Viral titer of each stock was quantified by a TCID50 assay with hemagglutination readout (described below) and converted to plaque forming units/mL. To transform TCID50/ml into PFU/ml, we multiplied TCID50/ml by 0.7. All cell culture and virus amplification procedures were performed according to Biosafety Level 2 protocols.
Calu-3 and ACH-3P cells (1 x 106 cells/well in 6-well plates) were seeded in growth culture medium and incubated overnight at 37°C and 5% CO2. The cells were washed with 1XPBS and then infected with H1N1 at MOI = 0, 0.5, or 3 for 1 hour. The virus inoculum consisted of Opti-MEM with TPCK-treated trypsin (0.5 μg/mL, Sigma). A titration curve was performed to determine the optimal TPCK-trypsin concentration for maximum virus permissibility and minimum cell monolayer lift. After inoculation, the cells washed with 1XPBS, and then replenished with serum-free medium (Opti-MEM) supplemented with TPCK-treated trypsin (0.5 μg/mL, Sigma). Samples were allowed to incubate for 0-, 24-, and 48-hours post-infection. For the progesterone treatment group, cells were treated with 100 nM P4 (24) for 24 hours pre-infection and 0-, 24-, and 48-hours post-infection. Supernatant samples were centrifuged, aliquoted, and stored at either -80°C and 4°C for future analyses. Cell pellets were suspended in either 500 μL QIAzol Lysis Reagent (cat # 79306, Qiagen, Hilden, Germany) or 500 μL 0.1% Triton-X 100 (cat # AC215682500, Fisher Scientific, Waltham, MA) and stored at -80°C.
The viral load of each supernatant sample was quantified by log10 TCID50 with hemagglutination assay read out. In brief, 75 μl of 0.33% turkey red blood cells (cat # TBA030, Hemostat, Dixon, CA), was incubated with 25 μl of sample at various dilutions for 1 hour at 4°C. A positive read out was defined by a diffuse suspension of red blood cells. TCID50 read out was calculated according to the Munch method (25). Log10 TCID50 values were then converted to PFU/ml for infection by multiplying by 0.7 (26).
Cell death by viral infection was assessed with the lactate dehydrogenase (LDH) assay (cat # MK401, Takara Bio USA, San Jose, CA) according to manufacturer protocols. Supernatant samples were stored at 4°C for no more than 24 hours after collection prior to analysis. The samples were diluted (30:70) with Opti-MEM and analyzed with a plate reader (absorbances at 492nm and 560nm). The absorbance values of the sample were normalized to absorbance values of a standard of complete cell death, yielding values that represent cell death percentage in each well.
The LDH assay used in this study quantified cell death by comparing total LDH released from dead cells due to infection (MOIs 0.5 and 3) compared to total LDH released spontaneously from uninfected, normal cells (MOI 0) in the culture of serum-free conditions. The formula used to calculate cytotoxicity was as follows: cytotoxicity (%) = (experimental value - low control)/(high control - low control) * 100. A low control was defined as cells with MOI 0, high control was defined as maximum LDH release from the cells (a well of cells was treated with 2% Triton-X in cell media to lyse all the cells). The experimental value was the LDH released by infected cells (MOIs 0.5 and 3).
The above formula was used to calculate cytotoxicity in treated (progesterone) and uncreated (control) experiments. These numbers were analyzed in comparison to each other with the above formula, and reported in the figures. By the nature of the calculation, LDH release of untreated, uninfected cells was used to calculate the cytotoxicity of H1N1 infection in untreated (control) conditions as the “low control” variable. Similarly, LDH release of treated (progesterone), uninfected cells were used to calculate the cytotoxicity of H1N1 infection in treated conditions as the “low control” variable.
Cell pellets were lysed with Qiazol and stored at -80°C prior to extraction. Total mRNA was extracted from each sample with the Qiagen miRNeasy Micro Kit (cat # 217084, Fisher Scientific, Waltham, MA) according to the manufacturer’s total RNA isolation protocol. cDNA was synthesized with random primers using the iScript™ Select cDNA Synthesis Kit (cat # 1708897BUN, Bio-Rad, Hercules, CA) according to the manufacturer’s protocol. Gene expression of IFIT1, MXA, and IFNB was measured with SYBR Green chemistry (for primer sequences, Table 1). Gene expression of IL6 (cat#: Hs00174131_m1, Thermo Fisher Scientific, Waltham, MA), IL1B (cat#: Hs01555410_m1, Thermo Fisher Scientific), and NLRP3 (cat#: Hs00918082_m1, Thermo Fisher Scientific) was measured with TaqMan® chemistry (Thermo Fisher Scientific). qPCR was run on a QuantStudio3 (Life Technologies) according to the manufacturer’s instructions. Fold-change was measured in reference to untreated samples and normalized to the Ct (cycle threshold) values for either RPL13A (Integrated DNA Technologies Assay ID: Hs.PT.58.47294843, SYBR Green, Redwood City, CA) or GAPDH (TaqMan® Gene Expression Assay Hs02758991_g1, Thermo Fisher Scientific).
Cell lysates were suspended 500 μL 0.1% Triton-X 100 and stored at -80°C. The activity of Caspase-1 in each sample was measured with the Caspase-1/ICE Fluorometric Assay Kit (cat # K110-25, BioVision, Milpitas, CA) according to the manufacturer’s instructions.
Supernatant samples were collected as described above and assessed with a customized Human ProcartaPlex Mix&Match 6-plex kit (cat # PPX-06-MX2W9XZ, Thermo Fisher Scientific) measure levels of 6 cytokines (IFN-β, IL-6, IL-8, IL-10, IL-1β, IL-18). The samples were analyzed according to the manufacturer’s protocols, using a MAGPIX. Total protein content in each sample was determined with the Pierce® BCA Protein Assay Kit (cat # 23250, Thermo Scientific) and colorimetric detection (absorbance wavelength at 562nm) with a plate reader.
The Kruskal-Wallis test was used to compare influenza-infected progesterone treated and untreated groups. A p value <0.05 was considered to be statistically significant.
To confirm whether Calu-3 was permissive to viral replication, the viral load of supernatant samples collected at 24- and 48-hours post infection was titered using the TCID50 assay with hemagglutination (HA) readout, and values were converted to PFU/ml. Live virus was recovered from the supernatant of each infected sample of Calu-3, confirming the permissibility and infectability of the lung cell lines to H1N1 pdm/09 (Figure 1A). Pre-treatment with progesterone did not impact viral load at any timepoint or with any MOI measured. However, a significant increase in LDH release (measure of cytotoxicity) was observed in Calu-3 treated with progesterone by 24 hours at MOIs of 0.5 and 3 compared to uninfected cells at the same timepoints (p<0.05, all). Progesterone exposure was associated with a significant increase in LDH release at 24 hours after H1N1 infection compared to untreated infected cells (Figure 1B, MOIs 0.5 and 3, p<0.05). By light microscopy, cytopathic effect (CPE) was observed in the infected cells including cell rounding, lifting and gaps in the monolayer and was subjectively more severe in these phenotypes with progesterone treatment compared to uninfected cells (Figures 1C–J).
Figure 1 Cell death and cytopathic effect in Calu-3, a lung cell line. IAV viral load is shown in Calu-3 (A). The bar plot depicts the Log10(PFU/ml) value grouped by MOI and time point. Cell death was quantified by LDH release in a subset of the experiments shown in panel A (B). Microscope images (40X objective lens) captured representative cellular pathology in control (C–F) and progesterone-treated Calu-3 cells (G–J) at 24 hours post-infection, the timepoint at which there was a significant increase in cell death due to progesterone treatment. The cells at 0 hours post-infection were infected with MOI 0 (C, G). At 24 hours post-infection, cells are shown infected with MOI 0 (D, H), MOI 0.5 (E, I), and MOI 3 (F, J). Blue indicates control cell lines and red indicates progesterone-treated cell lines. *p-value was less than 0.05, as determined by the statistical analysis detailed in the Methods section.
The gene and protein expression of a panel of biomarkers of interferon (IFN; e.g., interferon-beta, IFN-β), IFN-stimulated genes (ISGs; e.g., IFIT1, MxA) and cytokines (e.g., IL-6) were evaluated by RT-qPCR (cell pellets) and Luminex cytokine assays (supernatant) to determine the impact of progesterone on the antiviral innate immune response. Progesterone treatment of Calu-3 cells infected with H1N1 pdm/09 was associated with a significant upregulation of IL6, but not IFNB, IFIT1 or MXA (Figures 2A–D, MOIs 0.5 and 3, p<0.05). In contrast to the gene expression results, progesterone treatment was associated with a significant increase in protein expression of IFN-β (Figure 2E; MOIs 0.5 and 3, p<0.05). Increased IL-6 protein expression (Figure 2G, MOI 3, p<0.05) is consistent with increased cell death (Figure 1B) at 48 hours post-infection. Interestingly, the protein expression of IL-8 was significantly decreased after progesterone treatment at 48 hours post-infection (Figure 2F, MOI 3, p<0.05). In summary, progesterone treatment of lung cells was associated with an increase in IFN-β and IL-6 protein expression after H1N1 pdm/09 infection, which was not reflected by changes in gene expression specifically for IFNB.
Figure 2 Innate immune response in Calu-3, a lung cell line. Innate immune gene expression was measured by RT-qPCR. The gene expression log2 fold change is reported for IFNB (A), IFIT1 (B), MXA (C), and IL6 (D). The protein concentration of cytokines in the supernatant were measured with Luminex and are reported for IFN-β (E), IL-8 (F), IL-6 (G). *p-value was less than 0.05, as determined by the statistical analysis detailed in the Methods section.
Gene expression, protein expression, and enzymatic activity of a panel of biomarkers for the NLRP3 inflammasome activation pathway were measured by RT-qPCR, Luminex cytokine assay, and caspase-1 activity assay in the Calu-3 cell lines (Figure 3). Gene expression of NLRP3 and IL1B were not significantly altered by progesterone treatment of Calu-3 cells infected with H1N1 (Figures 3A, B, p<0.05, all). Although progesterone treatment did not alter gene expression of NLRP3, there was a temporal change in caspase-1 activity, the product of NLRP3 inflammasome activation, associated with progesterone treatment. Progesterone treatment was associated with a significant decrease in caspase-1 activity at 24 hours post-infection (MOI 0.5), and a significant increase in caspase-1 activity at 48h p.i. (MOIs 0.5 and 3) (Figure 3C, p<0.05, all). Caspase-1 cleaves pro-IL-1β and pro-IL-18 to their active forms. However, at 48 h.p.i. the change in caspase-1 activity was not completely reflected in the protein levels of IL-1β and IL-18. IL-1β levels were not significantly impacted by progesterone treatment at either timepoint, but IL-18 was significantly increased after progesterone treatment at both timepoints and MOIs measured (Figures 3D, E, p<0.05 all). Overall, progesterone treatment was associated with a time-dependent difference in caspase-1 activity in Calu-3 cells that was reflected in an increase in IL-18, but not IL-1β, protein expression at 48 h.p.i.
Figure 3 NLRP3 inflammasome activation in Calu-3, a lung cell line. Various markers of the NLRP3 inflammasome pathway were measured in Calu-3. Gene expression of IL1B (A) and NLRP3 (B) were measured by RT-qPCR. As a marker for NLRP3 inflammasome activation, caspase-1 activity was measured (C). Luminex multiplex assays were used to measure the change in concentration of IL-1β (D) and IL-18 (E) over time. *p-value was less than 0.05, as determined by the statistical analysis detailed in the Methods section.
To determine whether the placental trophoblast cell line, ACH-3P, was permissive to viral replication, the viral load of supernatant samples collected at 24 and 48 h.p.i. was measured by the TCID50 assay with HA readout and converted to PFU/ml. Infectious virus was recovered from the supernatant of each infected sample, confirming the permissibility and infectivity of the placental cell line to H1N1 pdm/09 (Figure 4A). As observed in Calu-3, progesterone treatment was not associated with a significant difference in viral load at any timepoint or MOI measured. However, infectious viral load in ACH-3P was significantly lower than that of Calu-3 at multiple timepoints and MOIs in both progesterone and controls (MOI 0.5 at 24 h.p.i. and MOI 3 at 24 h.p.i., p<0.05). Progesterone treatment of the H1N1 pdm/09 infected ACH-3P cells at both MOIs 0.5 and 3 was associated with a significantly decreased LDH release at 48 h.p.i. (Figure 4B, p<0.05, all). Like Calu-3, the CPE in ACH-3P was observed by light microscopy as early as 24 h.p.i. at both MOIs. In contrast to observations in Calu-3, progesterone treatment was associated with a less severe IAV-associated cell injury profile compared to untreated infected cells (Figures 4C–J).
Figure 4 Cell death and cytopathic effect in ACH-3P, a placental cell line. IAV viral load in ACH-3P (A). The bar plot depicts the Log10(PFU/ml) value grouped by MOI and time point. Cell death was quantified by LDH release in a subset of the experiments shown in panel A (B). Microscope images (40X objective lens) capture representative cellular pathology in the control cells (C–F) versus the progesterone-treated cells (G–J) at 24 hours post-infection, the timepoint at which there was a significant increase in cell death due to progesterone treatment. The ACH-3P cells at 0 hours post-infection were infected with MOI 0 (C, G). At 24 hours post-infection, cells are shown infected with MOI 0 (D, H), MOI 0.5 (E, I), and MOI 3 (F, J). Blue indicates control-treated cell lines and red indicates progesterone-treated cell lines. *p-value was less than 0.05, as determined by the statistical analysis detailed in the Methods section.
To determine the impact of progesterone on the antiviral innate immune responses in ACH-3P, we measured the gene and protein expression of a panel of innate immune biomarkers. Progesterone treatment was associated with a significant decrease in gene expression of IFNB at 48 h.p.i. (Figure 5A, p<0.05, all) and a significant increase in protein expression of IFN-β at both 24 and 48 h.p.i. (Figure 5E, p<0.05, all). Progesterone treatment was also associated with a significant decrease in gene expression of IL6 at 48 h.p.i. (Figure 5D, p<0.05, all) and a significant increase in protein expression of IL-6 at both 24h and 48 h.p.i. (Figure 5G, p<0.05, all). Gene expression of ISGs, IFIT1 and MXA were not significantly impacted by progesterone treatment (Figures 5B, C). Additionally, protein expression of IL-8 at both 24 and 48 h.p.i. was significantly decreased after progesterone treatment (Figure 5F, p<0.05, all). In summary, progesterone treatment in ACH-3P cells infected with H1N1 was associated with a significant increase in protein expression of IFN-β and IL-6 that was not reflected by changes in gene expression of IFNB and IL6.
Figure 5 Innate immune response in ACH-3P, a placental cell line. Innate immune gene expression was measured by RT-qPCR. Log2 fold change values are reported for IFNB (A), IFIT1 (B), MXA (C), and IL6 (D). The cytokine levels in the supernatant of ACH-3P cells were measured with Luminex. Concentrations are reported for IFN-β (E), IL-8 (F), IL-6 (G). *p-value was less than 0.05, as determined by the statistical analysis detailed in the Methods section.
Gene expression, protein expression, and protein activity were measured for a panel of biomarkers of NLRP3 inflammasome activity as described above. Progesterone treatment was not associated with a significant change in gene expression of NLRP3 (Figure 6B). However, progesterone treatment was associated with a significant decrease in gene expression of IL1B (Figure 6A, p<0.05), but a significant increase in protein expression of IL-1β at 48 h.p.i. (Figure 6D, p<0.05). Progesterone treatment was also associated with a significant increase in protein expression of IL-18 (Figure 6E, p<0.05). Furthermore, progesterone treatment was associated with a significant decrease in caspase-1 activity 24 h.p.i., but a significant increase in caspase-1 activity by 48 h.p.i. (Figure 6C, p<0.05; MOI 0.5). In summary, progesterone treatment was associated with a significant upregulation of IL-1β and IL-18 protein expression that was not reflected by IL1B gene expression. Progesterone also had a time-dependent impact on caspase-1 activity in ACH-3P that mirrored observations in Calu-3, but only at a lower MOI.
Figure 6 NLRP3 inflammasome in ACH-3P, a placental cell line. Various markers of the NLRP3 inflammasome pathway were measured in ACH-3P. Gene expression of IL1B (A) and NLRP3 (B) were measured by RT-qPCR. As a marker for NLRP3 inflammasome activation, caspase-1 activity was measured (C). The change in concentration of IL-1β (D) and IL-18 (E) over time was measured by Luminex. *p-value was less than 0.05, as determined by the statistical analysis detailed in the Methods section.
Progesterone is a master regulator of reproductive biology and has a critical role in the maintenance of human pregnancy (17, 24, 27). The impact of progesterone on innate immunity and cellular injury in the lung and placenta during an IAV infection is of particular interest given the enhanced lung disease and higher risk for stillbirth observed in infected pregnant women. Whether higher levels of progesterone may impair or enhance antiviral innate immune responses to IAV in the pregnant lung or placenta are unknown. This study investigated the impact of progesterone on cell death, innate immunity and NLRP3 inflammasome activity in Calu-3, a lung cell line, and ACH-3P, a placental cell line. Although both cell lines were permissible to IAV H1N1 pdm/2009, progesterone exposure had a differential impact on cell death in the lung and placental cell lines. Progesterone exposure was associated with significantly increased cell death in Calu-3 but had the opposite effect of reducing cell death in ACH-3P. Analysis of the antiviral innate immune response in both cell lines often revealed divergent expression of genes and their proteins indicating that progesterone signaling induced non-genomic and cell-specific responses. For example, progesterone treatment was associated with a decrease in IL6 gene expression but an increase in IL-6 protein concentrations in ACH-3P. This observation could be due to progesterone acting post-translationally on cytokines like IL-6, possibly to increase cytokine expression perdurance. Overall, progesterone modulated the effects of an IAV H1N1 pdm/2009 infection differently in lung and placental cell lines to impart genomic, non-genomic and cell-specific effects on gene expression.
Sex steroids can exert effects on non-reproductive tissues by binding to sex hormone binding globulins (SHBG) (28). Progesterone levels are high in the luteal phase of the menstrual cycle, which in several studies has been linked to the worsening of lung function in the latter half of the menstrual cycle. For example, progesterone treatment was associated with an exacerbation of lung injury in a murine model of systemic sclerosis induced by bleomycin (29). Additionally, in a cohort that spanned 22 U.S. states and two Canadian provinces, emergency room visits for acute asthma peaked during the luteal phase of the menstrual cycle (30). Further, several studies that measured the impact of sex hormones on exhaled nitric oxide concentration in pregnant women suggest that progesterone may amplify airway inflammation (31). Taken together, there is evidence to suggest that sex steroids like progesterone may exacerbate lung injury through proinflammatory effects.
Differences in progesterone concentrations among pregnant individuals and across the influenza infection time course may impact how well the lung and placenta can mitigate viral injury. The impact of progesterone on IAV H1N1 pdm/2009 viral load and innate immunity has been previously evaluated in several pregnant murine models (18, 32–34). Two murine models have indicated an inverse correlation between serum progesterone levels and lung viral load, indicating that higher systemic progesterone was associated with better viral control in the lung. Although we only tested a single progesterone dose, the higher cell death observed in progesterone-treated lung cells could act to eliminate infected cells and restrict viral replication. This would likely increase inflammation as well. Natural concentrations of progesterone have been observed to increase 4-fold from the first to the third trimester (35). Even among pregnant individuals at the same time point in pregnancy, progesterone concentrations can vary widely. In women pregnant for the first time, progesterone levels were 30-100% higher than pregnant women with a prior history of pregnancies (35). Further, the IAV infection itself can alter progesterone levels with a 5-fold reduction of serum progesterone in pregnant mice by 4 d.p.i (33). Lower levels of progesterone may be detrimental for the pregnant host. An IAV-induced reduction in systemic progesterone would be anticipated to have a pro-abortive effect on murine pregnancy as progesterone supports pregnancy maintenance and uterine quiescence. Although progesterone may acutely protect placental cells from IAV-associated injury, as shown by our findings, trophoblast cells may become more vulnerable to IAV over time, if local progesterone concentrations decrease. Varying concentrations of progesterone across pregnancy, individual pregnancies and an infection time course may impact the influenza disease course.
Progesterone is a key immunomodulating hormone during pregnancy and is likely to have nongenomic effects on signaling pathways important for innate immune defense against pathogens like IAV. In the reproductive tract, progesterone triggers posttranslational modifications of cellular receptors, transcription factors, and cofactors that results in long lasting, downstream consequences that are specific to cell types. Our findings of discordant gene and protein expression for IFN-β in both Calu-3 and ACH-3P and IL-6 in ACH-3P with progesterone treatment are consistent with nongenomic effects typical of sex steroids. The impact of these nongenomic effects has been understudied in the context of viral infections and innate immunity. In our study, progesterone treatment of both lung and trophoblast cell lines was associated with a significant increase in protein expression of IFN-β and IL-6 at 24 and 48 h.p.i. of IAV H1N1 pdm/2009. This was unexpected as progesterone typically inhibits innate immune responses and can suppress activation of macrophages and dendritic cells (36–40). Although both IFN-β and IL-6 can trigger apoptosis cellular programs, increased cell death was only observed in the lung cell line (41, 42). The impact of progesterone on innate immunity, cell death and IAV infection in Calu-3 and ACH-3P is cell-specific and contrary to expectations from other model systems.
The strength of this study is that it is the first direct assessment of the impact of progesterone on the susceptibility of human lung and trophoblast cell lines to IAV-induced innate immune activation and cell death. ACH-3P is an especially applicable cell line, because it expresses trophoblast markers and a transcriptome that closely resembles primary trophoblast (43). Nevertheless, it is important to note that cell lines are not exact models of organs and may have different expression levels of virus entry receptors or other cellular receptors that are important in innate immune activation. There are also important methodological pitfalls when studying rapid biological events that reflect genomic and non-genomic effects induced by sex steroids. Notably, our experiments were performed without fetal bovine serum, which can inhibit influenza virus replication (44) and vary in concentration of various growth hormones, introducing variability in the cell culture experimental environment (45).
The outcome of IAV infection is complex and affected by a person’s age, sex, pregnancy status and other co-morbid illnesses that create a complex signaling milieu that can be altered by sex steroids, like progesterone (17, 46–48). Our data indicate that progesterone imparts complex genomic and non-genomic effects on innate immunity in a lung and trophoblast cell line. Overall, results in these two cell lines were similar, but with a striking difference in the impact of progesterone treatment on cell death. In Calu-3, cell death was exacerbated by progesterone treatment, compared to an attenuated effect by progesterone treatment in ACH-3P. Future studies are needed to investigate cellular signaling downstream of progesterone to better elucidate pathways critical to the innate immune response to IAV and other viral infections during pregnancy.
The original contributions presented in the study are included in the article/supplementary files, further inquiries can be directed to the corresponding author.
The study design was conceived by KAW, ML, and HH. Grant funding was procured by KAW. The manuscript was written by ML, AL, and KAW. Experiments were performed by ML, HH, and AL. Materials were provided by DF and AO. Statistical analysis was performed by JM. All authors contributed to the article and approved the submitted version.
This work was supported by funding from the National Institutes of Health grants R01AI164588 to KAW.
We thank Jessie Brown and Mindi Raker for their assistance with graphic design of the figures. We thank Michael Gale Jr, PhD for the Calu-3 cell line.
Author KAW was employed by the company, GlaxoSmithKline as a consultant.
The remaining authors declare that the research was conducted in the absence of any commercial or financial relationships that could be construed as a potential conflict of interest.
All claims expressed in this article are solely those of the authors and do not necessarily represent those of their affiliated organizations, or those of the publisher, the editors and the reviewers. Any product that may be evaluated in this article, or claim that may be made by its manufacturer, is not guaranteed or endorsed by the publisher.
The content is solely the responsibility of the authors and does not necessarily represent the official views of the National Institutes of Health.
1. Vaccines against influenza: WHO position paper. Weekly Epidemiological Record (2022) 97:19. (Geneva, Switzerland: World Health Organization)
2. Pierce M, Kurinczuk JJ, Spark P, Brocklehurst P, Knight M, UKOSS. Perinatal outcomes after maternal 2009/H1N1 infection: National cohort study. BMJ 342 (2011), d3214. doi: 10.1136/bmj.d3214
3. Harris JW. Influenza occurring in pregnant women: A statistical study of thirteen hundred and fifty cases. JAMA (1919) 72:978–80. doi: 10.1001/jama.1919.02610140008002
4. Nishiura H. Excess risk of stillbirth during the 1918-1920 influenza pandemic in Japan. Eur J Obstet Gynecol Reprod Biol (2009) 147:115. doi: 10.1016/j.ejogrb.2009.07.009
5. Martin A, Cox S, Jamieson DJ, Whiteman MK, Kulkarni A, Tepper NK. Respiratory illness hospitalizations among pregnant women during influenza season, 1998-2008. Matern Child Health J (2013) 17:1325–31. doi: 10.1007/s10995-012-1135-3
6. Ribeiro AF, Pellini ACG, Kitagawa BY, Marques D, Madalosso G, Fred J, et al. Severe influenza A(H1N1)pdm09 in pregnant women and neonatal outcomes, state of sao paulo, Brazil, 2009. PloS One (2018) 13:e0194392. doi: 10.1371/journal.pone.0194392
7. Freeman DW, Barno A. Deaths from Asian influenza associated with pregnancy. Am J Obstet Gynecol (1959) 78:1172–5. doi: 10.1016/0002-9378(59)90570-8
8. Louie JK, Acosta M, Jamieson DJ, Honein MA, California Pandemic Working G. Severe 2009 H1N1 influenza in pregnant and postpartum women in California. N Engl J Med (2010) 362:27–35. doi: 10.1056/NEJMoa0910444
9. Hsu AC, Parsons K, Barr I, Lowther S, Middleton D, Hansbro PM, et al. Critical role of constitutive type I interferon response in bronchial epithelial cell to influenza infection. PloS One (2012) 7:e32947. doi: 10.1371/journal.pone.0032947
10. Harcourt JL, Caidi H, Anderson LJ, Haynes LM. Evaluation of the calu-3 cell line as a model of in vitro respiratory syncytial virus infection. J Virol Methods (2011) 174:144–9. doi: 10.1016/j.jviromet.2011.03.027
11. Pothlichet J, Meunier I, Davis BK, Ting JP, Skamene E, von Messling V, et al. Type I IFN triggers RIG-I/TLR3/NLRP3-dependent inflammasome activation in influenza a virus infected cells. PloS Pathog (2013) 9:e1003256. doi: 10.1371/journal.ppat.1003256
12. van Riel D, Mittrucker HW, Engels G, Klingel K, Markert UR, Gabriel G. Influenza pathogenicity during pregnancy in women and animal models. Semin Immunopathol (2016) 38:719–26. doi: 10.1007/s00281-016-0580-2
13. Chan KH, Zhang AJ, To KK, Chan CC, Poon VK, Guo K, et al. Wild type and mutant 2009 pandemic influenza a (H1N1) viruses cause more severe disease and higher mortality in pregnant BALB/c mice. PloS One (2010) 5:e13757. doi: 10.1371/journal.pone.0013757
14. Marcelin G, Aldridge JR, Duan S, Ghoneim HE, Rehg J, Marjuki H, et al. Fatal outcome of pandemic H1N1 2009 influenza virus infection is associated with immunopathology and impaired lung repair, not enhanced viral burden, in pregnant mice. J Virol (2011) 85:11208–19. doi: 10.1128/JVI.00654-11
15. Komine-Aizawa S, Suzaki A, Trinh QD, Izumi Y, Shibata T, Kuroda K, et al. H1N1/09 influenza a virus infection of immortalized first trimester human trophoblast cell lines. Am J Reprod Immunol (2012) 68:226–32. doi: 10.1111/j.1600-0897.2012.01172.x
16. Trinh QD, Izumi Y, Komine-Aizawa S, Shibata T, Shimotai Y, Kuroda K, et al. H3N2 influenza a virus replicates in immortalized human first trimester trophoblast cell lines and induces their rapid apoptosis. Am J Reprod Immunol (2009) 62:139–46. doi: 10.1111/j.1600-0897.2009.00723.x
17. Cervantes O, Cruz Talavera I, Every E, Coler B, Li M, Li A, et al. Role of hormones in the pregnancy and sex-specific outcomes to infections with respiratory viruses. Immunol Rev (2022) 308. doi: 10.1111/imr.13078
18. Hall OJ, Limjunyawong N, Vermillion MS, Robinson DP, Wohlgemuth N, Pekosz A, et al. Progesterone-based therapy protects against influenza by promoting lung repair and recovery in females. PloS Pathog (2016) 12:e1005840. doi: 10.1371/journal.ppat.1005840
19. Allen IC, Scull MA, Moore CB, Holl EK, McElvania-TeKippe E, Taxman DJ, et al. The NLRP3 inflammasome mediates in vivo innate immunity to influenza a virus through recognition of viral RNA. Immunity (2009) 30:556–65. doi: 10.1016/j.immuni.2009.02.005
20. Thomas PG, Dash P, Aldridge JR Jr., Ellebedy AH, Reynolds C, Funk AJ, et al. The intracellular sensor NLRP3 mediates key innate and healing responses to influenza a virus via the regulation of caspase-1. Immunity (2009) 30:566–75. doi: 10.1016/j.immuni.2009.02.006
21. Li W, Chang Y, Liang S, Zhong Z, Li X, Wen J, et al. NLRP3 inflammasome activation contributes to listeria monocytogenes-induced animal pregnancy failure. BMC Vet Res (2016) 12:36. doi: 10.1186/s12917-016-0655-2
22. Zhou K, Shi L, Wang Y, Chen S, Zhang J. Recent advances of the NLRP3 inflammasome in central nervous system disorders. J Immunol Res (2016) 2016:9238290. doi: 10.1155/2016/9238290
23. Koday MT, Leonard JA, Munson P, Forero A, Koday M, Bratt DL, et al. Multigenic DNA vaccine induces protective cross-reactive T cell responses against heterologous influenza virus in nonhuman primates. PloS One (2017) 12:e0189780. doi: 10.1371/journal.pone.0189780
24. Nadeem L, Shynlova O, Matysiak-Zablocki E, Mesiano S, Dong X, Lye S. Molecular evidence of functional progesterone withdrawal in human myometrium. Nat Commun (2016) 7:11565. doi: 10.1038/ncomms11565
25. Reed LJ MH. A simple method of estimating fifty per cent endpoints. Am J Hygiene (1938) 27:493–7. doi: 10.1093/oxfordjournals.aje.a118408
27. Mesiano S. Myometrial progesterone responsiveness. Semin Reprod Med (2007) 25:5–13. doi: 10.1055/s-2006-956771
28. Williams AE, Maskarinec G, Franke AA, Stanczyk FZ. The temporal reliability of serum estrogens, progesterone, gonadotropins, SHBG and urinary estrogen and progesterone metabolites in premenopausal women. BMC Womens Health (2002) 2:13. doi: 10.1186/1472-6874-2-13
29. Vafashoar F, Mousavizadeh K, Poormoghim H, Haghighi A, Pashangzadeh S, Mojtabavi N. Progesterone aggravates lung fibrosis in a mouse model of systemic sclerosis. Front Immunol 12 (2021). doi: 10.3389/fimmu.2021.742227
30. Zimmerman JL, Woodruff PG, Clark S, Camargo CA. Relation between phase of menstrual cycle and emergency department visits for acute asthma. Am J Respir Crit Care Med (2000) 162:512–5. doi: 10.1164/ajrccm.162.2.9910105
31. Tam A, Morrish D, Wadsworth S, Dorscheid D, Man SF, Sin DD. The role of female hormones on lung function in chronic lung diseases. BMC Womens Health (2011) 11:24. doi: 10.1186/1472-6874-11-24
32. Davis SM, Sweet LM, Oppenheimer KH, Suratt BT, Phillippe M. Estradiol and progesterone influence on influenza infection and immune response in a mouse model. Am J Reprod Immunol (New York NY (2017) 1989):78. doi: 10.1111/aji.12695
33. Littauer EQ, Esser ES, Antao OQ, Vassilieva EV, Compans RW, Skountzou I. H1N1 influenza virus infection results in adverse pregnancy outcomes by disrupting tissue-specific hormonal regulation. PloS Pathog (2017) 13:e1006757. doi: 10.1371/journal.ppat.1006757
34. Kim JC, Kim HM, Kang YM, Ku KB, Park EH, Yum J, et al. Severe pathogenesis of influenza b virus in pregnant mice. Virology (2014) 448:74–81. doi: 10.1016/j.virol.2013.10.001
35. Schock H, Zeleniuch-Jacquotte A, Lundin E, Grankvist K, Lakso H.-Å., Idahl A, et al. Hormone concentrations throughout uncomplicated pregnancies: A longitudinal study. BMC pregnancy childbirth (2016) 16:146. doi: 10.1186/s12884-016-0937-5
36. Hardy DB, Janowski BA, Corey DR, Mendelson CR. Progesterone receptor plays a major antiinflammatory role in human myometrial cells by antagonism of nuclear factor-kappaB activation of cyclooxygenase 2 expression. Mol Endocrinol (2006) 20:2724–33. doi: 10.1210/me.2006-0112
37. De Nicola AF, Garay LI, Meyer M, Guennoun R, Sitruk-Ware R, Schumacher M, et al. Neurosteroidogenesis and progesterone anti-inflammatory/neuroprotective effects. J Neuroendocrinol 30 (2018) e12502. doi: 10.1111/jne.12502
38. Gutzeit O, Segal L, Korin B, Iluz R, Khatib N, Dabbah-Assadi F, et al. Progesterone attenuates brain inflammatory response and inflammation-induced increase in immature myeloid cells in a mouse model. Inflammation (2021) 44:956–64. doi: 10.1007/s10753-020-01390-y
39. Jones LA, Anthony JP, Henriquez FL, Lyons RE, Nickdel MB, Carter KC, et al. Toll-like receptor-4-mediated macrophage activation is differentially regulated by progesterone via the glucocorticoid and progesterone receptors. Immunology (2008) 125:59–69. doi: 10.1111/j.1365-2567.2008.02820.x
40. Chernykh ER, Leplina OY, Tyrinova TV, Tikhonova MA, Sakhno LV, Ostanin AA. Contribution of dehydroepiandrosterone sulfate and progesterone to in vitro regulation of tolerogenic activity of IFN-alpha-induced dendritic cells. Bull Exp Biol Med (2011) 151:205–9. doi: 10.1007/s10517-011-1290-3
41. Jin YH, Hou W, Kang HS, Koh CS, Kim BS. The role of interleukin-6 in the expression of PD-1 and PDL-1 on central nervous system cells following infection with theiler’s murine encephalomyelitis virus. J Virol (2013) 87:11538–51. doi: 10.1128/JVI.01967-13
42. Kotredes KP, Gamero AM. Interferons as inducers of apoptosis in malignant cells. J Interferon Cytokine Res (2013) 33:162–70. doi: 10.1089/jir.2012.0110
43. Hiden U, Wadsack C, Prutsch N, Gauster M, Weiss U, Frank HG, et al. The first trimester human trophoblast cell line ACH-3P: A novel tool to study autocrine/paracrine regulatory loops of human trophoblast subpopulations–TNF-alpha stimulates MMP15 expression. BMC Dev Biol (2007) 7:137. doi: 10.1186/1471-213X-7-137
44. Hossain MJ, Mori I, Dong L, Liu B, Kimura Y. Fetal calf serum inhibits virus genome expression in madin-Darby canine kidney cells persistently infected with influenza a virus. Med Microbiol Immunol (2008) 197:21–7. doi: 10.1007/s00430-007-0054-1
46. Klein SL, Passaretti C, Anker M, Olukoya P, Pekosz A. The impact of sex, gender and pregnancy on 2009 H1N1 disease. Biol Sex Dif (2010) 1:5. doi: 10.1186/2042-6410-1-5
47. Morgan R, Klein SL. The intersection of sex and gender in the treatment of influenza. Curr Opin Virol (2019) 35:35–41. doi: 10.1016/j.coviro.2019.02.009
Keywords: H1N1 influenza virus, ACH-3P, Calu-3, progesterone, cell line
Citation: Li M, Li A, Huang H, Munson J, Obadan A, Fuller DH and Adams Waldorf KM (2022) Impact of progesterone on innate immunity and cell death after influenza A virus H1N1 2009 infection of lung and placental cells in vitro. Front. Virol. 2:953208. doi: 10.3389/fviro.2022.953208
Received: 25 May 2022; Accepted: 30 August 2022;
Published: 23 September 2022.
Edited by:
Siddappa N. Byrareddy, University of Nebraska Medical Center, United StatesReviewed by:
Craig John Bierle, University of Minnesota Twin Cities, United StatesCopyright © 2022 Li, Li, Huang, Munson, Obadan, Fuller and Adams Waldorf. This is an open-access article distributed under the terms of the Creative Commons Attribution License (CC BY). The use, distribution or reproduction in other forums is permitted, provided the original author(s) and the copyright owner(s) are credited and that the original publication in this journal is cited, in accordance with accepted academic practice. No use, distribution or reproduction is permitted which does not comply with these terms.
*Correspondence: Kristina M. Adams Waldorf, YWRhbXNrQHV3LmVkdQ==
Disclaimer: All claims expressed in this article are solely those of the authors and do not necessarily represent those of their affiliated organizations, or those of the publisher, the editors and the reviewers. Any product that may be evaluated in this article or claim that may be made by its manufacturer is not guaranteed or endorsed by the publisher.
Research integrity at Frontiers
Learn more about the work of our research integrity team to safeguard the quality of each article we publish.