- 1CNS Disease Modeling Laboratory, Department of Microbiology, Institute of Biomedical Sciences, University of São Paulo, São Paulo, Brazil
- 2CNS Disease Modeling Laboratory, Scientific Platform Pasteur-USP, São Paulo, Brazil
Zika virus (ZIKV) is an arthropod-borne virus (arbovirus) from the Flaviviridae family, first isolated from the Rhesus monkey in 1947 in Uganda. ZIKV is transmitted by mosquito bites, but vertical and sexual transmissions have also been reported. ZIKV infection during pregnancy causes malformation in the developing fetus, especially central nervous system (CNS) damages, with a noticed microcephaly, making ZIKV be recognized as a teratogenic agent and the responsible for congenital Zika syndrome (CZS). However, it is still a short time since CZS was first reported. Consequently, ZIKV pathogenesis is not entirely elucidated, especially considering that affected children are still under neurodevelopment. Here, we will explore the current knowledge about ZIKV teratogenesis focusing on neurological clinical findings in humans, mechanisms, and experimental models used to understand ZIKV pathophysiology.
Introduction
Although ZIKV infection is asymptomatic in most cases, the common signs and symptoms are fever, rash, arthralgia, and conjunctival hyperemia (1). It is noteworthy that ZIKV infection has also been related to more severe clinical outcomes, especially considering neurological signs, both in CNS and peripheral nervous systems, such as meningoencephalitis, acute myelitis, and Guillain-Barré syndrome (2).
In 2015, Brazil had a significant increase in the number of cases of newborns with microcephaly. In 2016, key works proved that ZIKV infection during pregnancy was responsible for the malformation in the developing fetus, especially leading to structural and neurological defects (3, 4). In vivo and in vitro approaches were decisive to demonstrate that ZIKV can cross the placental barrier affecting fetal development and has a tropism for neural progenitor cells (NPCs), showing a causal relationship between ZIKV and microcephaly (5, 6). Later on, and based on clinical investigation, other symptoms were associated with ZIKV pathogenesis during fetus neurodevelopment, such as brain calcifications, hydrocephalus, ventriculomegaly, lissencephaly, holoprosencephaly, seizures, and neurosensorial deficits (7). ZIKV was first identified as a possible teratogenic agent in Brazil in 2015, calling the clinical picture of newborns as Congenital Zika Syndrome (CZS). Studies suggest that fetal abnormalities induced by ZIKV may occur in all trimesters of pregnancy. However, the manifestations with the most significant negative impact are associated with infections in the first and second trimester (8), and depending on that period, ZIKV vertical infection can cause limitations of intrauterine growth, spontaneous abortion, and microcephaly.
Considering the information above mentioned, and being established a relationship between the increased number of microcephaly cases in newborns caused by ZIKV infection, the WHO declared in 2016 ZIKV infection during pregnancy as a public health emergency. ZIKV represents a threat on a global scale since there are no drugs and vaccines available to treat or prevent the infection (9).
The pathogenesis of ZIKV is still not fully understood. Here, we will overview ZIKV teratogenesis focusing on neurological clinical findings in humans, mechanisms, and experimental models used to understand ZIKV pathophysiology.
Neurological Clinical Findings in Human
After the outbreak in Brazil, many studies have associated ZIKV with neurological diseases in newborns whose mothers contracted the virus during pregnancy. Additionally, ZIKV RNA was identified in babies with microcephaly brain tissue (4). In microscopic examinations of a fetal brain infected with ZIKV, apoptotic neurons were observed, mainly post-migratory neurons with intermediate differentiation (10).
The harmful effects of congenital viruses on pregnancy and fetal outcomes are partly because of impaired trophoblastic function, as the placenta is a kind of selective barrier due to multiple immune and cellular structures (11). Profound pathological changes were observed in placentas infected by ZIKV, like abnormal fetal capillaries, trophoblastic apoptosis, increased fetal nucleated erythrocytes, which indicates a biological malfunction (12). ZIKV can infect the placenta through blood-placenta transmission leading to microcephaly and a severe loss of intracranial volume (13, 14). A neuroimaging report showed a cranial bone collapse in babies born from mothers suspected of having ZIKV during pregnancy (15). Magnetic resonance identified a spectrum of anomalies that include marked cortical thinning with an abnormal gyratory pattern, increased fluid spaces (ventricular and extra-axial), hypoplasia or absence of corpus callosum, and hypoplasia in the cerebellar vermis (16).
Postmortem CNS analysis from newborns who died within 48 h after birth from ischemia-associated consequences showed that ZIKV infects neuroglial progenitor cells. Calcifications and destructive lesions were also found, supporting changes in the brain, delayed cerebral atrophy, and transient convulsive activities (17–20).
Besides vertical transmission, neurological symptoms were also reported in adults after ZIKV infection. In adults, ZIKV infection has been associated with conditions of transverse myelitis, peripheral neuropathy, and meningoencephalitis (2, 21). An imaging study showed a reduced volume of gray matter in specific motor cortical regions compared with controls, leading to a life-term impact on the CNS (22). Acute myelitis was described 7 days after ZIKV infection in a teenager in Guadalupe. A spinal magnetic resonance imaging showed an increase in the thoracic and cervical spinal cord. ZIKV RNA was found in serum, urine, and cerebrospinal fluid (CSF) on the second day of neurological complaints. The presence of ZIKV in the CSF reinforces that ZIKV is neurotropic (23). Besides CNS, ocular abnormalities have also been reported as part of the effects of CZS and anomalies of the optic nerve, focal pigmentary gait, and chorioretinal atrophy (24, 25).
Based on current knowledge about the pathogenesis of ZIKV and the other defects that the infection causes in fetal development, ZIKV should be considered a TORCH pathogen (Toxoplasmosis, Other (syphilis, varicella-zoster, parvovirus B19), Rubella, Cytomegalovirus, and Herpes) (26). The similarities between congenital disorders considered TORCH and ZIKV are striking and say about their neurotropism (27). Malformations induced by TORCH and ZIKV pathogen depend on the gestational age of the fetal infection, being more severe as the earlier occurs during pregnancy, like in the first trimester of gestation. As the pregnancy progresses, the risk of congenital malformations that result from virus infections decreases and becomes low during and after the second trimester.
Mechanism of Teratogenesis Caused by ZIKV Infection
Studying the SARS-CoV-2′s pathogenesis mechanisms could help understand the symptoms caused by its illness, find drugs to combat the infection, and select potential targets for vaccines.
ZIKV is composed of a single positive-sense RNA strand, with ~10 kb, protected by a capsid and an envelope of lipids and proteins. Its genome codifies three structural proteins, pre-Membrane (prM), Envelope (E), and Capsid (C), and seven non-structural proteins (NS1, NS2A, NS2B, NS3, NS4a, NS4b, NS5).
ZIKV can enter Neural Progenitor Cells (NPCs) (and other cells of CNS) using mainly AXL receptor (AXL Receptor Tyrosine Kinase) in the cell surface (6). Once ZIKV enters the cell, its RNA is rapidly translated by local ribosomes into a polyprotein that encodes structural and non-structural proteins, which become part of the virions and play a role in viral replication. The virus modifies the cellular endoplasmic reticulum (ER), forming “replication factories” where viral replication and production of viral proteins occurs, inducing ER stress and unfolded protein response (UPR), which inhibits protein synthesis and activates ER-associated degradation (ERAD). Lastly, the viral genomes assemble with the new virions particles and are secreted through the Golgi apparatus.
Besides forming the replication machinery, non-structural proteins help to inhibit the antiviral response. NS5, NS2B, and NS3 regulate type 1 IFN pathways, NS5 protein inhibits human STAT2 suppressing IFN-I production and favoring viral proliferation (28) (Figure 1). Further, NS4A and NS4B have an important function related to apoptosis and growth arrest since they act together to inhibit the AKT-mTOR pathway (29), which causes mitochondrial elongation, and extends production of ATP (Adenosine Triphosphate) by oxidative phosphorylation resulting in a rise in reactive oxygen species by glial cells, increasing mitochondrial stress (30, 31). Increased cellular stress could activate the p53 intrinsic apoptosis pathway (32). So, proapoptotic proteins such as Bcl-2-associated protein X (BAX) cause the release of cytochrome C by mitochondria, which activates caspase pathways (33). Moreover, ZIKV's NS4B protein can directly recruit BAX from the cytosol into the mitochondria activating this apoptotic pathway (34) (Figure 1).
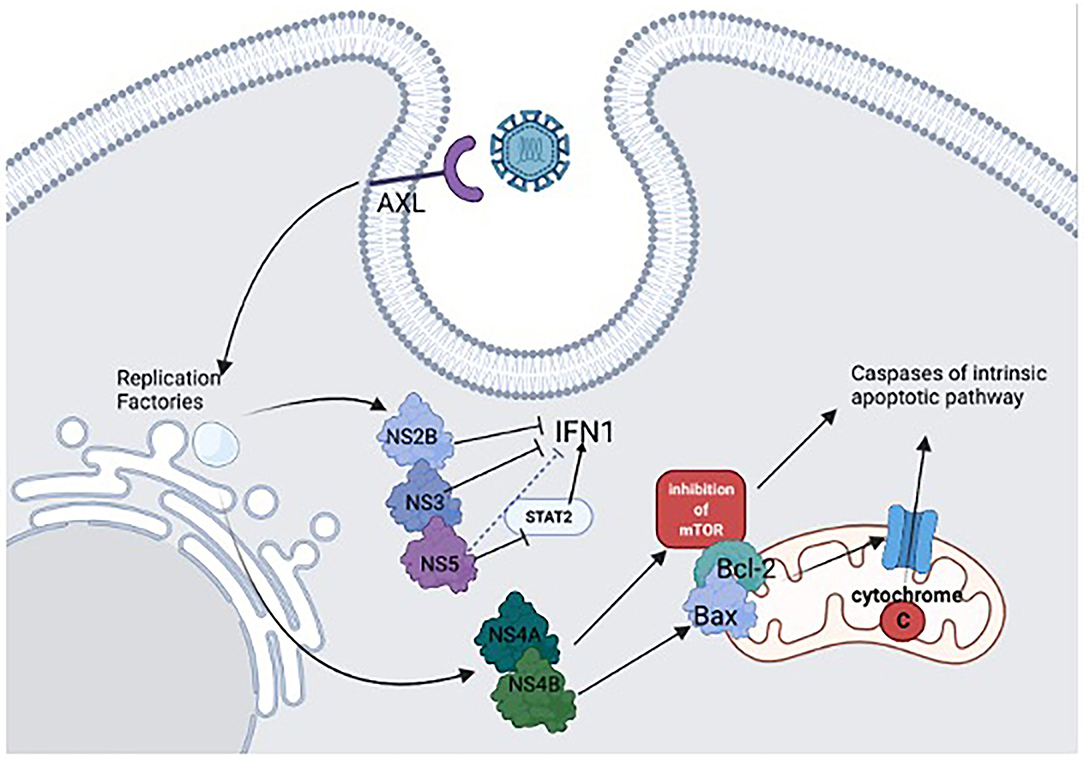
Figure 1. ZIKV adsorbs to the cell surface receptor AXL to enter CNS cells. When the viral replication machinery is active, ZIKV produces non-structural proteins (NS). The NS2B and NS3 help to inhibit antiviral response by regulating type 1 IFN pathways. The NS5 protein inhibits human STAT2, which indirectly suppresses IFN-I production, favoring viral proliferation. NS4A and NS4B act in the apoptosis pathway and growth arrest, inhibiting the AKT-mTOR pathway and increasing mitochondrial stress, activating the p53 intrinsic apoptosis pathway. Additionally, NS4B activates pro-apoptotic proteins such as Bcl-2-associated protein X (BAX), causing cytochrome C release and activating caspase pathways.
It is believed that the immune response elicited by the infection plays a role in this growth arrest. In NPCs, IFN-independent Interferon Stimulated Genes (ISG) activations, such as IRF3 (Interferon Regulatory Factor 3) or NF-?B (Nuclear Factor kappa B), were observed, while no TLR3 (toll-like receptor 3) responses were activated (35). Nevertheless, in brain organoids, in the late stage of development, TLR3 was overexpressed after ZIKV infection. TLR3 activation was correlated with 41 genes expression linked to neuronal development, suggesting a perturbation in neurogenesis. Moreover, since these genetic hubs are regulators of axon guidance processes, anti-apoptotic and cell-cycle pathways, they can mediate microcephaly phenotype as revealed in brain organoid models (36).
The mechanisms described here revealed how ZIKV causes apoptosis, cell cycle-growth arrest and induces premature differentiation, leading to microcephaly and other birth disorders. However, the link between molecular mechanisms and phenotypic clinical findings must be better understood to clarify why some fetuses are affected by ZIKV and others do not or why they have different grades of disease severity. Not only that but elucidating ZIKV pathogenesis will be beneficial for drug discovery to prevent CZS and vaccine development against ZIKV infection.
Experimental In Vitro Models: Brain Cells
Understanding associated teratogenic mechanisms and molecular pathways referred to as CZS are also related to understanding human development. Mouse models have provided important information on the subject, as most protein-coding genes are shared with humans (37, 38), but there are relevant restrictions, especially when considering eye and brain development (37) and gene expression patterns along with development (39) which present significant discrepancies when compared with humans. Furthermore, rodents need to have their antiviral defenses knocked down with dampened interferon responses to allow the viral infection (40, 41), which may raise questions about the use of this model.
Advances in producing and applying induced pluripotent stem cell (iPSC) technology have provided essential tools for disease modeling in vitro (42, 43). Through the application of different protocols of differentiation, neural progenitors, neurons, glia cells, and brain organoids derived from iPSC have been helpful for investigations on ZIKV infection (44).
Neural Progenitor Cells (NPCS)
Reports about ZIKV infection have shown that the Neural Progenitor Cells (NPCs) are sensible and permissive to the virus (45, 46). These reports, concomitant with the investigation of the association of prenatal ZIKV infection and microcephaly, and other malformations, revealed that ZIKV is a potential teratogen agent, culminating in physical or functional congenital disabilities from abnormal fetal development (47).
Until the new circulating strain called ZIKVBR, in 2015, there was no association between the virus and neurological symptoms or brain damage in humans (48). Up to 12 weeks postconception, the maternal blood and tissue face the fetal membranes within the placenta due to the restructured maternal circulation (49), which allows the ZIKVBR to target the NPCs after crossing the placenta, inducing cell apoptosis and autophagy (5). The Brazilian ZIKV strain was revealed as more aggressive and more harmful to the neurogenesis when compared to the first isolated ZIKV strain, the MR766 (5, 8, 29).
The differentiation of NPCs reaches the development process and populates the growing brain with neurons during prenatal development (50). Modeling the neurodifferentiation process by NPCs iPSC-derived helped to elucidate the mechanisms underlying ZIKV pathogenesis. ZIKV prejudices brain development, impairing cell division, proliferation and inducing apoptosis, leading to potentially disastrous consequences for CNS development (51, 52). Human neural stem cells (NSCs) isolated between 18 and 22 weeks of gestational age after conception unveil the suppression of host AKT-mTOR signaling by the cooperation of proteins NS4A and NS4B, upregulating autophagy for viral replication (29). The importance of autophagy relies on homeostasis control, being an efficient mechanism to limit pathogen infection. An AKT-mTOR signaling pathway is critical for cortical development (53) and AKT constitutive activation or loss of function is related to disorders as megalencephaly and microcephaly, respectively (54).
Inductive pathways and signaling shared between two surrounding embryonic structures may influence brain development by paracrine effects (55), like brain and face integrated development. As that craniofacial disproportion is related to ZIKV congenital infection (3), another work used cranial neural crest cells (CNCCs) signaling molecules. This approach provided evidence that the addition of leukemia inhibitory factor (LIF) or vascular endothelial growth factor (VEGF) cytokines in equivalent levels as the one produced during ZIKV infection results in precocious neurogenesis. This precocious neurogenesis contributes to a microcephaly phenotype, as migration and proliferation deregulated timing may affect brain size (56).
Neurons
In vitro neurons have also contributed to the effort to understand ZIKV infection effects over the CNS. ZIKV can infect mature neurons that express AXL receptors causing neurological disorders (30). Recent findings exhibited impaired neurogenesis and synaptogenesis process over neurons derived from iPSCs infected by the Brazilian ZIKV strain (57). Studies revealed a global downregulation of synaptic proteins, such as postsynaptic density protein SHANK2 (58, 59), and proteins associated with presynaptic precursors and presynaptic active zone, as VAMP2 (Vesicle-associated Membrane Protein 2) and complexin 2, Piccolo, Basson, and the Soluble NSF Attachment Receptor (SNARE) proteins (60–62). Those findings highlighted the vulnerability of the synaptic formation to the virus, leading to synaptic loss and contributing to mental and motor disabilities.
In another study, researchers analyzed miRNA profiles of primary mouse neurons after ZIKV infection. They showed that ZIKV causes a global downregulation of miRNAs with only a few upregulated miRNAs. On the other hand, ZIKV infection induces upregulation of antiviral, inflammatory, and apoptotic genes (63).
Glia Cells
Besides the destructive effect on neuronal structures, the pathogen also compromises glia cells functioning in the fetus, negatively impacting brain development. Astrocytes extending into the subarachnoid space were identified in affected brain region slices from a 32-week fetus infected with the virus (4). This period comprises extensive neurogenesis and gliogenesis, suggesting a significant contribution of the astrocyte impaired growth, contributing to microcephaly (64).
Human microglia cell line (CHME3), human astrocytes, and NPCs were challenged with the African ZIKV strain HD78788 for the study of AXL receptor role in ZIKV infection, reporting it as a crucial receptor for the virus infection on human glial cells by promoting viral entry after binding ZIKV-Gas6 complex, also damping innate immune responses in glial cells (65). AXL belongs to a group of tyrosine kinases receptors related to innate immunity regulation and mediates phagocytosis of apoptotic cells (66).
Underlying entry factors, like AXL, have been studied to elucidate flavivirus mechanisms of infection (67). Although signaling mechanisms that promote the disease outcome are still not well understood, further investigations should unveil mechanisms that could be the basis for developing suitable therapeutic strategies.
Brain Organoids
Brain organoids are a three-dimensional structure derived from human iPSC. Brain organoids can be differentiated in various regions from the brain, as hindbrain, midbrain, and forebrain neuron subtypes (68–71). These tools have been a breakthrough in studying neurodegenerative and neurodevelopmental disorders, allowing the modeling of several conditions such as autism and microcephaly (71–73).
Brain organoids have also proved advantageous to study the mechanisms involved in ZIKV pathogenesis. Important findings from ZIKV infection using brain organoids were possible due to the model's physiological relevance and its capacity to mimic the developing fetal brain. ZIKV infection reduces the neuronal cell layer in human brain organoids (52).
Cerebral organoids generated from H9 hESCs (Human Embryonic Stem Cells) were treated with MR766 ZIKV to investigate the role of TLR3 (toll-like receptor 3), an innate immune receptor, in the ZIKV infection, unveiling the TLR3 upregulation after infection on organoids. This upregulation causes dysregulation of neurogenesis, apoptosis, and organoid shrinkage, contributing to impaired neurogenesis and microcephaly (36). TLR3 has also been linked with negative activation of axogenesis (74).
In another study, Brazilian and African ZIKV strains were used to infect three-dimensional neural cell cultures, neurospheres, and cerebral organoids generated from hiPSCs. Brazilian ZIKV infected neurospheres presented significantly more morphological abnormalities than African ZIKV infected at 96 h post-infection (5). Cortical plate thickness and dividing cells reduction on ventricular zone were more significant in organoids infected by Brazilian ZIKV strain, as was the increased number of apoptotic cells. Decreased number of dorsal forebrain progenitors cells was verified in both ZIKV infections. Those findings verify the capacity of cerebral organoids to support analysis of different parts of the brain and highlight neurodevelopmental disorders mechanisms.
Author Contributions
PB-B conceptually designed the manuscript, wrote, and edited. FR wrote the manuscript and guided the other three co-authors. CT, FT, and GS wrote the manuscript. All authors contributed to the article and approved the submitted version.
Funding
This work was sponsored by FAPESP (18/16748-8 and 16/02978-6), the Brazilian NGO the tooth fairy project and in part by the Coordenação de Aperfeiçoamento de Pessoal de Nivel Superior-Brasil (Capes)-Finance Code 001; and Conselho Nacional de Desenvolvimento Científico e Tecnológico (CNPq), and a post doc fellowship supported by the Institute Pasteur.
Conflict of Interest
The authors declare that the research was conducted in the absence of any commercial or financial relationships that could be construed as a potential conflict of interest.
Publisher's Note
All claims expressed in this article are solely those of the authors and do not necessarily represent those of their affiliated organizations, or those of the publisher, the editors and the reviewers. Any product that may be evaluated in this article, or claim that may be made by its manufacturer, is not guaranteed or endorsed by the publisher.
Acknowledgments
We want to thank the University of São Paulo for its support and Beatriz McGilvray for manuscript revision.
Abbreviations
ATP, adenosine triphosphate; AXL, AXL receptor tyrosine kinase; BAX, Bcl-2-associated protein X; CNS, central nervous system; CSF, cerebrospinal fluid; CZS, congenital Zika syndrome; CNCCs, cranial neural crest cells; CHME3, human microglia cell line; ER, endoplasmic reticulum; ERAD, ER-associated degradation; hESCs, human embryonic stem cells; iPSC, induced pluripotent stem cell; IRF3, Interferon Regulatory Factor 3; LIF, leukemia inhibitory factor; NF-κB, nuclear factor kappa B; NPCs, neural progenitor cells; SHANK2, SH3 and multiple ankyrin repeat domains 2; SNARE, soluble NSF attachment receptor; TORCH, toxoplasmosis, Other (syphilis, varicella-zoster, parvovirus B19), Rubella, Cytomegalovirus and Herpes; TLR3, toll-like receptor 3; WHO, World Health Organization; VAMP2, Vesicle-Associated Membrane Protein 2; VEGF, vascular endothelial growth factor; UPR, unfolded protein response; ZIKV, Zika virus.
References
1. Duffy MR, Chen TH, Hancock WT, Powers AM, Kool JL, Lanciotti RS, et al. Zika virus outbreak on Yap Island, Federated States of Micronesia. N Engl J Med. (2009) 360:2536–43. doi: 10.1056/NEJMoa0805715
2. Carteaux G, Maquart M, Bedet A, Contou D, Brugières P, Fourati S, et al. Zika virus associated with meningoencephalitis. N Engl J Med. (2016) 374:1595–6. doi: 10.1056/NEJMc1602964
3. de Fatima Vasco Aragao M, van der Linden V, Brainer-Lima AM, Coeli RR, Rocha MA, Sobral da., Silva P, et al. Clinical features and neuroimaging (CT and MRI) findings in presumed Zika virus related congenital infection and microcephaly: retrospective case series study. BMJ. (2016) 353:i1901. doi: 10.1136/bmj.i1901
4. Mlakar J, Korva M, Tul N, Popović M, Poljšak-Prijatelj M, Mraz J, et al. Zika virus associated with microcephaly. N Engl J Med. (2016) 374:951–8. doi: 10.1056/nejmoa1600651
5. Cugola FR, Fernandes IR, Russo FB, Freitas BC, Dias JL, Guimarães KP, et al. The Brazilian Zika virus strain causes birth defects in experimental models. Nature. (2016) 534:267–71. doi: 10.1038/nature18296
6. Nowakowski TJ, Pollen AA, Di Lullo E, Sandoval-Espinosa C, Bershteyn M, Kriegstein AR. Expression analysis highlights AXL as a candidate zika virus entry receptor in neural stem cells. Cell Stem Cell. (2016) 18:591–6. doi: 10.1016/j.stem.2016.03.012
7. Saad T, PennaeCosta AA, de Góes FV, de Freitas M, de Almeida JV, de Santa Ignêz LJ, et al. Neurological manifestations of congenital Zika virus infection. Childs Nerv Syst. (2017) 34:73–8. doi: 10.1007/s00381-017-3634-4
8. Brasil P., Pereira JP Jr, Moreira ME, Ribeiro Nogueira RM, Damasceno L, Wakimoto M, et al. Zika Virus infection in pregnant women in Rio de Janeiro. N Engl J Med. (2016) 375:2321–34. doi: 10.1056/NEJMoa1602412
9. Song BH, Yun SI, Woolley M, Lee YM. Zika virus: history, epidemiology, transmission, and clinical presentation. J Neuroimmunol. (2017) 308:50–64. doi: 10.1016/j.jneuroim.2017.03.001
10. Driggers RW, Ho CY, Korhonen EM, Kuivanen S, Jääskeläinen AJ, Smura T, et al. Zika virus infection with prolonged maternal viremia and fetal brain abnormalities. N Engl J Med. (2016) 374:2142–51. doi: 10.1056/NEJMoa1601824
11. Arechavaleta-Velasco F, Koi H, Strauss JF, Parry S. Viral infection of the trophoblast: time to take a serious look at its role in abnormal implantation and placentation? J Reprod Immunol. (2002) 55:113–21. doi: 10.1016/s0165-0378(01)00143-7
12. Miner JJ, Diamond MS. Understanding how Zika virus enters and infects neural target cells. Cell Stem Cell. (2016) 18:559–60.
13. Musso D, Roche C, Robin E, Nhan T, Teissier A, Cao-Lormeau VM. Potential sexual transmission of Zika virus. Emerg Infect Dis. (2015) 21:359–61. doi: 10.3201/eid2102.141363
14. Culjat M, Darling SE, Nerurkar VR, Ching N, Kumar M, Min SK, et al. Clinical and imaging findings in an infant with Zika embryopathy. Clin Infect Dis. (2016) 63:805–11. doi: 10.1093/cid/ciw324
15. Dain Gandelman Horovitz D, da Silva Pone MV, Moura Pone S, Dias Saad Salles TR, Bastos Boechat MC. Cranial bone collapse in microcephalic infants prenatally exposed to Zika virus infection. Neurology. (2016) 87:118–9. doi: 10.1212/WNL.0000000000002814
16. Besnard M, Lastere S, Teissier A, Cao-Lormeau V, Musso D. Evidence of perinatal transmission of Zika virus, French Polynesia, December 2013 and February 2014. Euro Surveill. (2014) 19:20751.
17. Chimelli L, Melo ASO, Avvad-Portari E, Wiley CA, Camacho AHS, Lopes VS, et al. The spectrum of neuropathological changes associated with congenital Zika virus infection. Acta Neuropathol. (2017). 133:983–99. doi: 10.1007/s00401-017-1699-5
18. van der Linden V, Filho EL, Lins OG, van der Linden A, Aragão M, Brainer-Lima AM, et al. Congenital Zika syndrome with arthrogryposis: retrospective case series study. BMJ. (2016). 354:i3899. doi: 10.1136/bmj.i3899
19. Nem de Oliveira Souza I, Frost PS, França JV, Nascimento-Viana JB, Neris RLS, Freitas L, et al. Acute and chronic neurological consequences of early-life Zika virus infection in mice. Sci Transl Med. (2018). 10:eaar2749. doi: 10.1126/scitranslmed.aar2749
20. Mavigner M, Raper J, Kovacs-Balint Z, Gumber S, O'Neal JT, Bhaumik SK, et al. Postnatal Zika virus infection is associated with persistent abnormalities in brain structure, function, and behavior in infant macaques. Sci Transl Med. (2018). 10:eaao6975. doi: 10.1126/scitranslmed.aao6975
21. Acosta-Ampudia Y, Monsalve DM, Castillo-Medina LF, Rodríguez Y, Pacheco Y, Halstead S, et al. Autoimmune neurological conditions associated with Zika virus infection. Front Mol Neurosci. (2018) 11:116. doi: 10.3389/fnmol.2018.00116
22. Bido-Medina R, Wirsich J, Rodríguez M, Oviedo J, Miches I, Bido P, et al. Impact of Zika Virus on adult human brain structure and functional organization. Ann Clin Transl Neurol. (2018) 5:752–62. doi: 10.1002/acn3.575
23. Mécharles S, Herrmann C, Poullain P, Tran TH, Deschamps N, Mathon G, et al. Acute myelitis due to Zika virus infection. Lancet. (2016) 387:1481. doi: 10.1016/S0140-6736(16)00644-9
24. de Paula Freitas B, de Oliveira Dias JR, Prazeres J, Sacramento GA, Ko AI, Maia M, et al. Ocular findings in infants with microcephaly associated with presumed Zika virus congenital infection in Salvador, Brazil. JAMA Ophthalmol. (2016) 134:529–35. doi: 10.1001/jamaophthalmol.2016.0267
25. Ventura CV, Maia M, Ventura BV, Linden VV, Araújo EB, Ramos RC, et al. Ophthalmological findings in infants with microcephaly and presumable intra-uterus Zika virus infection. Arq Bras Oftalmol. (2016) 79:1–3. doi: 10.5935/0004-2749.20160002
26. Tahotná A, Brucknerová J, Brucknerová I. Zika virus infection from a newborn point of view. TORCH, or TORZiCH? Interdiscip Toxicol. (2018) 11:241–6. doi: 10.2478/intox-2018-0023
27. Faizan MI, Abdullah M, Ali S, Naqvi IH, Ahmed A, Parveen S. Zika virus-induced microcephaly and its possible molecular mechanism. Intervirology. (2016) 59:152–8. doi: 10.1159/000452950
28. Oyarzún-Arrau A, Alonso-Palomares L, Valiente-Echeverría F, Osorio F, Soto-Rifo R. Crosstalk between RNA metabolism and cellular stress responses during Zika virus replication. Pathogens. (2020) 9:158. doi: 10.3390/pathogens9030158
29. Liang Q, Luo Z, Zeng J, Chen W, Foo SS, Lee SA, et al. Zika Virus NS4A and NS4B Proteins deregulate Akt-mTOR signaling in human fetal neural stem cells to inhibit neurogenesis and induce autophagy. Cell Stem Cell. (2016) 19:663–71. doi: 10.1016/j.stem.2016.07.019
30. Rothan HA, Fang S, Mahesh M, Byrareddy SN. Zika virus and the metabolism of neuronal cells. Mol Neurobiol. (2019) 56:2551–7. doi: 10.1007/s12035-018-1263-x
31. Almeida LT, Ferraz AC, da Silva Caetano CC, da Silva Menegatto MB, dos Santos Pereira Andrade AC, Lima RLS, et al. Zika virus induces oxidative stress and decreases antioxidant enzyme activities in vitro and in vivo. Virus Res. (2020) 286:198084. doi: 10.1016/j.virusres.2020.198084
32. Ghouzzi VE, Bianchi FT, Molineris I, Mounce BC, Berto GE, Rak M, et al. ZIKA virus elicits P53 activation and genotoxic stress in human neural progenitors similar to mutations involved in severe forms of genetic microcephaly and p53. Cell Death Dis. (2016) 7:e2440. doi: 10.1038/cddis.2016.266
34. Han X, Wang J, Yang Y, Qu S, Wan F, Zhang Z, et al. Zika virus infection induced apoptosis by modulating the recruitment and activation of proapoptotic protein bax. J Virol. (2021) 95:e01445. doi: 10.1128/jvi.01445-20
35. Liu L, Chen Z, Zhang X, Li S, Hui Y, Feng H, et al. Protection of ZIKV infection-induced neuropathy by abrogation of acute antiviral response in human neural progenitors. Cell Death Differ. (2019) 26:2607–21. doi: 10.1038/s41418-019-0324-7
36. Dang J, Tiwari SK, Lichinchi G, Qin Y, Patil VS, Eroshkin AM, et al. Zika virus depletes neural progenitors in human cerebral organoids through activation of the innate immune receptor TLR3. Cell Stem Cell. (2016) 19:258–65. doi: 10.1016/j.stem.2016.04.014
37. Gerrelli D, Lisgo S, Copp AJ, Lindsay S. Enabling research with human embryonic and fetal tissue resources. Development. (2015) 142:3073–6. doi: 10.1242/dev.122820
38. Zhu Z, Huangfu D. Human pluripotent stem cells: an emerging model in developmental biology. Development. (2013) 140:705–17. doi: 10.1242/dev.086165
39. Fougerousse F. Human-mouse differences in the embryonic expression patterns of developmental control genes and disease genes. Hum Mol Genet. (2000) 9:165–73. doi doi: 10.1093/hmg/9.2.165
40. Rossi SL, Vasilakis N. Modeling Zika virus infection in mice. Cell Stem Cell. (2016) 19:4–6. doi: 10.1016/j.stem.2016.06.009
41. Mysorekar IU, Diamond MS. Modeling Zika virus infection in pregnancy. N Engl J Med. (2016) 375:481–4. doi: 10.1056/nejmcibr1605445
42. Yamanaka S. Induced pluripotent stem cells: past, present, and future. Cell Stem Cell. (2012) 10:678–84.
43. Claus C, Jung M, Hübschen JM. Pluripotent stem cell-based models: a peephole into virus infections during early pregnancy. Cells. (2020) 9:542. doi: 10.3390/cells9030542
44. Alvarado MG, Schwartz DA. Zika virus infection in pregnancy, microcephaly, and maternal and fetal health: what we think, what we know, and what we think we know. Arch Pathol Lab Med. (2016) 141:26–32. doi: 10.5858/arpa.2016-0382-ra
45. Ming G, Tang H, Song H. Advances in Zika virus research: stem cell models, challenges, and opportunities. Cell Stem Cell. (2016) 19:690–702. doi: 10.1016/j.stem.2016.11.014
46. Tang H, Hammack C, Ogden SC, Wen Z, Qian X, Li Y, et al. Zika virus infects human cortical neural progenitors and attenuates their growth. Cell Stem Cell. (2016) 18:587–90. doi: 10.1016/j.stem.2016.02.016
47. Rasmussen SA, Jamieson DJ, Honein MA, Petersen LR. Zika virus and birth defects—reviewing the evidence for causality. N Engl J Med. (2016) 374:1981–7. doi: 10.1056/NEJMsr1604338
48. Russo FB, Jungmann P, Beltrão-Braga PCB. Zika infection and the development of neurological defects. Cell Microbiol. (2017) 19:e12744. doi: 10.1111/cmi.12744
49. Arora N, Sadovsky Y, Dermody TS, Coyne CB. Microbial vertical transmission during human pregnancy. Cell Host Microbe. (2017) 21:561–7. doi: 10.1016/j.chom.2017.04.007
50. Götz M, Huttner WB. The cell biology of neurogenesis. Nat Rev Mol Cell Biol. (2005) 6:777–88. doi: 10.1038/nrm1739
51. Garcez PP, Loiola EC, Madeiro da Costa R, Higa LM, Trindade P, Delvecchio R, et al. (2016). Zika virus impairs growth in human neurospheres and brain organoids. Science. 352:816–8. doi: 10.1126/science.aaf6116
52. Qian X, Nguyen HN, Song MM, Hadiono C, Ogden SC, Hammack C, et al. Brain-region-specific organoids using mini-bioreactors for modeling ZIKV exposure. Cell. (2016) 165:1238–54. doi: 10.1016/j.cell.2016.04.032
53. Franke T. PI3K/Akt: getting it right matters. Oncogene. (2008) 27:6473–88. doi: 10.1038/onc.2008.313
54. Mirzaa GM, Rivière JB, Dobyns WB. Megalencephaly syndromes and activating mutations in the PI3K-AKT pathway: MPPH and MCAP. Am J Med Genet C Semin Med Genet. (2013) 163C:122–30. doi: 10.1002/ajmg.c.31361
55. Marcucio R, Hallgrimsson B, Young NM. Facial morphogenesis. In: Current Topics in Developmental Biology. Amsterdam: Elsevier (2015), p. 299–320. doi: 10.1016/bs.ctdb.2015.09.001
56. Bayless NL, Greenberg RS, Swigut T, Wysocka J, Blish CA. Zika virus infection induces cranial neural crest cells to produce cytokines at levels detrimental for neurogenesis. Cell Host Microbe. (2016) 20:423–8. doi: 10.1016/j.chom.2016.09.006
57. Rosa-Fernandes L, Cugola FR, Russo FB, Kawahara R, de Melo Freire CC, Leite PEC, et al. Zika virus impairs neurogenesis and synaptogenesis pathways in human neural stem cells and neurons. Front Cell Neurosci. (2019) 13:64. doi: 10.3389/fncel.2019.00064
58. Li Z, Sheng M. Some assembly required: the development of neuronal synapses. Nat Rev Mol Cell Biol. (2003) 4:833–41. doi: 10.1038/nrm1242
59. Rizo J, Xu J. The synaptic vesicle release machinery. Annu Rev Biophys. (2015) 44:339–67. doi: 10.1146/annurev-biophys-060414-034057
60. Waites CL, Leal-Ortiz SA, Okerlund N, Dalke H, Fejtova A, Altrock WD, et al. Bassoon and Piccolo maintain synapse integrity by regulating protein ubiquitination and degradation. EMBO J. (2013) 32:954–69. doi: 10.1038/emboj.2013.27
61. Mishima T, Fujiwara T, Sanada M, Kofuji T, Kanai-Azuma M, Akagawa K. Syntaxin 1B, but not syntaxin 1A, is necessary for the regulation of synaptic vesicle exocytosis and of the readily releasable pool at central synapses. PLoS ONE. (2014) 9:e90004. doi: 10.1371/journal.pone.0090004
62. Trimbuch T, Rosenmund C. Should I stop or should I go? The role of complexin in neurotransmitter release. Nat Rev Neurosci. (2016) 17:118–25. doi: 10.1038/nrn.2015.16
63. Azouz F, Arora K, Krause K, Nerurkar VR, Kumar M. Integrated microRNA and mRNA profiling in Zika virus-infected neurons. Viruses. (2019) 11:162. doi: 10.3390/v11020162
64. Potokar M, Jorgačevski J, Zorec R. Astrocytes in flavivirus infections. IJMS. (2019) 20:691. doi: 10.3390/ijms20030691
65. Meertens L, Labeau A, Dejarnac O, Cipriani S, Sinigaglia L, Bonnet-Madin L, et al. Axl mediates ZIKA virus entry in human glial cells and modulates innate immune responses. Cell Rep. (2017) 18:324–33. doi: 10.1016/j.celrep.2016.12.045
66. Lemke G, Rothlin C. Immunobiology of the TAM receptors. Nat Rev Immunol. 8:327–36. doi: 10.1038/nri2303
67. Hamel R, Dejarnac O, Wichit S, Ekchariyawat P, Neyret A, Luplertlop N, et al. Biology of Zika virus infection in human skin cells. J Virol. (2015) 89:8880–96. doi: 10.1128/jvi.00354-15
68. Muguruma K, Nishiyama A, Kawakami H, Hashimoto K, Sasai Y. Self-organization of polarized cerebellar tissue in 3D culture of human pluripotent stem cells. Cell Rep. (2015) 10:537–50. doi: 10.1016/j.celrep.2014.12.051
69. Lee CT, Bendriem RM, Wu WW, Shen RF. 3D brain Organoids derived from pluripotent stem cells: promising experimental models for brain development and neurodegenerative disorders. J Biomed Sci. (2017) 24:59. doi: 10.1186/s12929-017-0362-8
70. Bagley JA, Reumann D, Bian S, Lévi-Strauss J, Knoblich JA. Fused cerebral organoids model interactions between brain regions. Nat Methods14. (2017) 743–51. doi: 10.1038/nmeth.4304
71. Sloan SA, Andersen J, Pa?ca AM, Birey F, Pa?ca SP. Generation and assembly of human brain region-specific three-dimensional cultures. Nat Protoc. (2018) 13:2062–85. doi: 10.1038/s41596-018-0032-7
72. Lancaster M, Renner M, Martin CA, Wenzel D, Bicknell LS, Hurles ME, et al. Cerebral organoids model human brain development and microcephaly. Nature. (2013) 501:373–9. doi: 10.1038/nature12517
73. Mariani J, Coppola G, Zhang P, Abyzov A, Provini L, Tomasini L, et al. FOXG1-Dependent dysregulation of GABA/glutamate neuron differentiation in autism spectrum disorders. Cell. (2015) 162:375–90. doi: 10.1016/j.cell.2015.06.034
Keywords: Zika virus, ZIKV, teratogenesis, mechanisms, disease modeling, in vitro models, brain development
Citation: Russo FB, Toledo CM, Tocantins FR, Souza GV and Beltrão-Braga PCB (2022) ZIKV Teratogenesis: Clinical Findings in Humans, Mechanisms and Experimental Models. Front. Virol. 1:775361. doi: 10.3389/fviro.2021.775361
Received: 28 September 2021; Accepted: 29 November 2021;
Published: 11 March 2022.
Edited by:
Jun Wang, Rutgers, The State University of New Jersey, United StatesReviewed by:
Ivan Toplak, University of Ljubljana, SloveniaAlvaro Mallagaray, University of Lübeck, Germany
Copyright © 2022 Russo, Toledo, Tocantins, Souza and Beltrão-Braga. This is an open-access article distributed under the terms of the Creative Commons Attribution License (CC BY). The use, distribution or reproduction in other forums is permitted, provided the original author(s) and the copyright owner(s) are credited and that the original publication in this journal is cited, in accordance with accepted academic practice. No use, distribution or reproduction is permitted which does not comply with these terms.
*Correspondence: Patricia C. B. Beltrão-Braga, cGF0cmljaWFjYmJicmFnYUB1c3AuYnI=