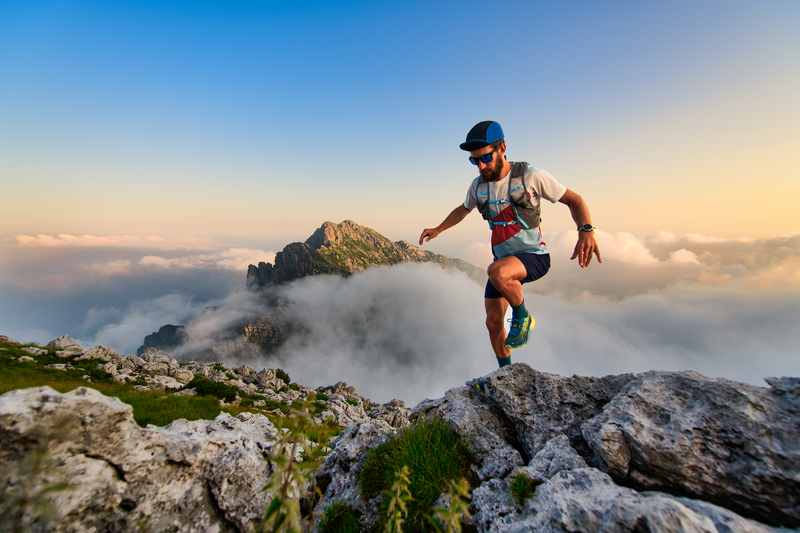
95% of researchers rate our articles as excellent or good
Learn more about the work of our research integrity team to safeguard the quality of each article we publish.
Find out more
ORIGINAL RESEARCH article
Front. Vet. Sci. , 06 February 2025
Sec. Animal Nutrition and Metabolism
Volume 12 - 2025 | https://doi.org/10.3389/fvets.2025.1542637
This article is part of the Research Topic Unlocking the Power of Gut Microbiota to Improving Health and Welfare in Non-Ruminant Livestock View all 9 articles
Lower intramuscular fat (IMF) and excessive abdominal fat reduce carcass quality in broilers. The study aimed to investigate the effects of dietary VD3 on growth performance, lipid metabolism and cecal microbiota in broilers over an 84-d feeding experiment. One-day-old male Luhua broilers (210) were randomly assigned to control (basal diet) and VD group (basal diet supplemented with 3,750 IU/kg VD3). Samples were collected after a 12-h fasted feeding on days 28, 56, and 84. Supplementary VD3 significantly enhanced average daily gain (ADG) in broilers aged 57-84 d and 1-84 d, and increased leg muscle rate and fat content in breast and leg muscles and reduced abdominal fat rate of broilers at 84 d. VD3 increased TG and glycogen content in the liver of 28- and 84-d-old broilers, serum TG and VLDL-C content at 56 and 84 d, and TC, HDL-C and LDL-C at 84 d. VD3 increased mRNA expressions of genes related to de novo lipogenesis (DNL) (mTOR, SREBP-1c, FAS and ACC), lipid oxidation (AMPK, PPARα, CPT-1α and ACO) and lipid transport (ApoB and MTTP), and FAS, ACC and CPT1 enzyme activities in the liver. However, mRNA levels of genes involved in DNL and cellular lipid uptake (LPL and FATP1) and LPL activity were decreased in abdominal adipose tissue, and that of genes involved in lipid oxidation and lipolysis (HSL and ATGL) was increased by VD3. LPL and FATP1 expression in breast and leg muscles was increased by VD3. Moreover, VD3 increased the abundance of cecum Bacteroides at 28 and 84 d, Rikenellaceae_RC9_gut_group and Faecalibacterium at 56 and 84 d, and Lachnoclostridium at 84 d. These bacteria were correlated with increased DNL, lipid oxidation and lipid transport in liver, and cellular lipid uptake in muscle, as well as decreased DNL and cellular lipid uptake, and increased lipid oxidation and lipolysis in abdominal adipose tissue. Altogether, supplementary VD3 in basal diet improved growth performance, increased IMF, and reduced abdominal fat rate, which is significant for enhancing feed utilization and improving the carcass quality of broilers. The regulation of VD3 on lipid metabolism could was associated with variation in cecal microbiota composition.
Chicken meat is the second most-consumed meat worldwide by humans. With continuous advancements in breeding and high-density feeding, the chicken production has significantly increased. However, a relatively lower intramuscular fat (IMF) content negatively impacts the meat quality (1), while excessive accumulation of abdominal fat reduces the carcass quality and feed conversion rate in broiler chickens (2), presenting a challenging issue in modern broiler production systems. Many factors, such as genetics, rearing systems, and dietary energy balance, influence fat deposition in broilers. The regulation of micronutrients on lipid metabolism is recognized as a crucial pathway for controlling body fat deposition and energy distribution in animals.
Vitamin D (VD) is a class of steroid derivatives that exist in multiple forms due to variations in their side chain structures. VD exhibits the functional characteristics of both vitamins and hormones, with ergocalciferol (vitamin D2, VD2) and cholecalciferol (vitamin D3, VD3) serving as the primary functional substances in animals. VD3 is the primary form of VD used by chickens, as they cannot efficiently metabolize plant-derived VD2 (3). VD3 can be synthesized endogenously by the skin at sufficient UV-B exposure, but its main source is dietary intake (4, 5). It is well known that VD plays a key regulatory role in the absorption, utilization and metabolic homeostasis of calcium and phosphorus, as well as in bone development and health of animals (3, 6, 7). However, recent studies have shown that adipose tissue, as a main storage site for VD, also expresses VD hydroxylase, catalyzing the generation of the active form of VD, 1,25-dihydroxyvitamin D3 [1,25 (OH)2D3] (8). This active form could influence biological functions, such as adipogenesis, in non-classical target tissues through autocrine, paracrine, or endocrine pathways. VD regulated various biological processes in its active form in adipose tissue through the mediation of the vitamin D receptor (VDR), including the proliferation and differentiation of adipocyte, lipogenesis, and the expression of adipokines and inflammatory responses (9, 10).
VD3 has been reported to improve glucose homeostasis and maintain metabolic balance in humans and rats (11, 12). VD3 enhanced glucose uptake in 3 T3-L1 cells by promoting AMPK phosphorylation and the expression and translocation of glucose transporter 4 (GLUT4), which was supported by in vivo experiments showing that VD3 increased glucose uptake in both adipocytes and adipose tissue of mice (13). VD3 reduced triglyceride (TG) levels in liver by inhibiting lipogenesis through the downregulation of sterol regulatory element binding protein 1-c (SREBP1-c) and fatty acid synthase (FAS) expression, while simultaneously enhancing β-oxidation of fatty acids by increasing the expression of carnitine palmitoyl transferase 1α (CPT-1α) and peroxisome proliferator-activated receptor (PPAR) α in mice (14). Supplementation of VD3 alleviated liver steatosis in high-fat-induced obese mice (12) and mitigated hepatic steatosis by regulating fatty acid uptake and β-oxidation through PPARα signaling pathway in mice (15). 1,25(OH)2D3 reduced lipid storage and lipid droplet aggregation by decreasing the expression of FAS and PPARγ, while increasing the expression of CPT-1α, PPARα and hormone-sensitive lipase (HSL) in 3 T3-L1 adipocytes (16). On the contrary, VD3 deficiency significantly decreased the expression of β-oxidation-related genes, such as CPT1α, PPARγ coactivator-1 α (PGC1α), and PPARα, as well as protein level of AMP-activated protein kinase (AMPK) in obese rats (17). Additionally, VD3 influenced gut microbiota to modulate the host’s metabolism through VDR, which was abundantly expressed in the gut (18). Dietary supplementation with VD3 reduced serum levels of HDL-C, TG, and TC, increased the abundance of gut microbiota, and improved carbohydrate and amino acid metabolism in mice (19). Zhang et al. (20) showed that VD3 promoted the restoration of gut microbiota dysbiosis and inhibited lipid accumulation caused by a high-fat diet by increasing the abundance of Lactobacillus while decreasing that of Oscillibacter and Flavonifractor in the gut of rats.
Previous studies have shown that VD3 regulated glucose and lipid metabolism in humans and rodents, and this effect was enduring and persistent (21, 22). It has also been demonstrated that VD3 was beneficial for bone quality (7), immunity (9), and antioxidants (23), which could be related to the gut microbiota. While VD3 supplementation improved growth and carcass performance, affected meat color, and decreased abdominal fat percentage (24, 25), current studies have not clarified the effect of VD3 on lipid metabolism and its relationship with the gut microbiota in broilers. In this study, we investigated the effects of VD3 supplementation on a basal diet on the growth performance and carcass quality in Luhua broilers, a medium-growing strain that is popular locally because of the chewy texture of the meat. Due to its lengthy growth period and significant fat deposition capability, this broiler is an excellent subject for studying fat deposition. Furthermore, we systematically explored the mechanism by which supplementary VD3 affected fat accumulation by investigating variations in lipid metabolism in the liver and adipose tissue, lipid transport, and cecal microbiota in the broilers.
All procedures involving in animals were performed following the Regulations for the Administration of Affairs Concerning Experimental Animals (Ministry of Science and Technology, China, 2004) and were approved and supervised by the Northwest Minzu University Animal Care and Use Committee (Permit No. xbmu-sm-20210130).
A total of 210 healthy one-day-old male Luhua chicken broilers with similar body weight were randomly divided into two groups, each with 7 replicates and 15 chickens per replicate. The broilers in the control group (CON group) were fed a basal diet, while the broilers in the VD3 group (VD group) received the basal diet supplemented with 3,750 IU/kg of VD3 (24). The VD3 product was supplied by Guangzhou Applon Biotechnology Co., Ltd. (Guangzhou, China). The basal diet was formulated according to the broiler Feeding Standard in China (GB/T 5916-2020) and was detailed in Table 1.
The trial was conducted at ShunHe Broiler Breeding Farm (Lanzhou, China). The broilers were raised in flat net-rearing system. Each replication was reared separately in a single pen (70 cm from the concrete floor) consisting of a stainless-steel frame with a flat wire net covered. Each pen was 200 × 100 cm with a round feeder pan and nipple drinkers (5 nipple drinkers in middle of pen). All broilers were housed in a windowed house with a controlled ventilation regime and a wet curtain cooling system. The temperature inside the house was maintained at 35 ~ 37°C with a relative humidity (RH) of 50% during the first week, gradually decreasing by 2°C each week until the coop temperature reached 24°C with RH at 45%. The lighting regime was 23-h light/1-h dark daily for chicks aged 1–28 days, and 16-h light/8-h dark daily for broilers aged 29–84 days. The experiment lasted 84 days. The broilers had ad libitum access to feed and water. The broilers received the Newcastle disease vaccine and the infectious bursal polyvalent vaccine on day 7 and 14 of the experiment, respectively.
The body weight (BW) of broilers was measured every 14 days after fasting for 12 h, and the feed intake on a pen basis was recorded daily. The average daily gain (ADG), average daily feed intake (ADFI) and feed conversion rate (FCR, feed/gain) were calculated. Mortality was recorded as it occurred.
On the last day of the experiment (day 84), two broilers with a BW close to the average in each replicate were selected and killed after fasting for 12 h. Following bleeding and plucking, the carcass was individually weighed. Subsequently, the birds were eviscerated, and weights were measured. The half-eviscerated carcass weight was calculated by excluding the trachea, esophagus, intestines, spleen, pancreas, gallbladder, reproductive organs, and gizzard contents and corneum from the carcass. The eviscerated weight was calculated by removing the heart, liver, proventriculus, gizzard, lungs, and abdominal fat from the half-eviscerated carcass. The rates of dressing, half-eviscerated carcass, eviscerated carcass, breast muscle, thigh muscle (thigh and drumstick), abdominal fat (fat around the abdomen), and liver were calculated as relative weight to the live BW.
On the morning of the last day of the starter stage (days 1 to 28), the grower stage (days 29 to 56), and the finisher stage (days 57 to 84), that was on days 28, 56, and 84, after a 12-h fasted feeding, two broilers in each pen (i.e., 14 birds per group) were randomly selected for sampling. 5 mL of blood samples were collected from the wing vein of each individual, and serum was collected through centrifuging at 3,000 rpm for 10 min. Afterwards, the broilers were slaughtered and quickly dissected, and approximately 5 g samples of liver, abdominal adipose tissue, and leg and breast muscle were collected. The cecal contents were carefully collected, homogenized using a sterile spatula, and transferred to CryoPure Tubes (Sarstedt AG + Co., Nümbrecht, Germany). The samples collected above were snap-frozen in liquid nitrogen and stored at −80°C until analysis.
Approximately 2.0 g of tissue samples were freeze-dried using a vacuum freeze dryer and then ground to a homogeneous powder. The fat (ether extract, EE) in the tissue samples was extracted using a Soxhlet extractor (SOX406, Hanon Advanced Technology Group Co., Ltd., Shandong, China) (GB 5009.6–2016, China). Fat content was expressed as a percentage of fat in fresh tissue samples.
Nine times the cooled physiological saline was added to 1 g of liver and adipose tissue samples, which were ground in a glass homogenizer to prepare a tissue homogenate. The homogenate was centrifuged at 4°C at 3000 × g for 10 min, and the supernatant was collected. The supernatant and serum samples were analyzed for triglyceride (TG), total cholesterol (TC), high-density lipoprotein cholesterol (HDL-C), low-density lipoprotein cholesterol (LDL-C), very low-density lipoprotein cholesterol (VLDL-C), free fatty acid (FFA), glycogen and glucose content using an automatic biochemical analyzer (BS-200; Shenzhen Mindray Bio-Medical Electronics Co., Ltd., Shenzhen, China). The contents of insulin and VD, and the activities of FAS, acetyl-CoA carboxylase (ACC), CPT-1α and lipoprotein lipase (LPL), were determined using chicken-specific enzyme-linked immunosorbent assay (ELISA) kits following the manufacturer’s protocol (Solarbio Science & Technology Co., Beijing, China).
Total RNA were extracted using TRIzol reagent and cDNA was synthesized with a reverse transcription kit according to the manufacturer’s protocol (TaKaRa Biotechnology, Dalian, China). Real-time RT-PCR was performed on a fluorescent quantitative detection system (FQD-96A; Hangzhou Bioer Technology Co., China). Amplification was conducted in a total volume of 10 μL containing 5 μL of SYBR Green PCR Master Mix (TaKaRa Biotechnology, Dalian, China), 0.4 μL of each forward and reverse primer, 1 μL of cDNA, and 3.2 μL of ddH2O. PCR reaction procedure: pre-denaturation at 95°C for 30 s; followed by 40 cycles of 95°C for 5 s, 60°C for 30 s, and 72°C for 30 s. The relative expression of each target gene was expressed using comparative threshold cycle (2−ΔΔCt) method and β-actin as an internal control. The specificity of the PCR amplification was verified with melting curve analysis. The information of primers used in this study was listed in Table 2.
Bacterial genomic DNA was isolated from the cecal contents using the TGuide S96 kit (DP812; Tiangen Biotech Co., Beijing, China) following the manufacturer’s instructions. The purity and quality of the DNA were verified in 0.8% agarose gels. Subsequently, the full-length 16S rRNA gene was amplified using the primers (27F: AGRGTTTGATYNTGGCTCAG and 1492 R: TASGGHTACCTTGTTASGACTT). The sequencing libraries (SMRT Bell) were constructed using purified PCR products and sequenced on PacBio Sequel II platform (Biomarker-Technologies Co., China). SMRT-Link v8.0 was used to correct the original subreads to obtain Circular Consensus Sequencing (CCS) sequence. The CCS sequences were identified using Lima v1.7.0 software and filtered with cutadapt 1.9.1 software, and the non-chimeric CCS were obtained by the DADA2 method of QIIME2 2020.6 software. BMK Cloud1 was used for Alpha diversity, Beta diversity, and microbial composition analysis.
Statistical analysis was performed using a two-tailed Student’s t-test in the SPSS software (version 26.0; IBM Corp., Armonk, NY, USA), with the results expressed as± Standard Error of Means (SEM). Statistical significance was established at p < 0.05. Spearman correlation analysis was performed using R version 3.5.1 to evaluate the correlations between cecal biomarker bacterial genera and significantly different lipid metabolism indicators. GraphPad Prism 8.02 (GraphPad, Inc. La Jolla, CA, USA) was used for data mapping charts.
The broilers remained in good health throughout the experiment. The effect of the supplementary VD3 on growth performance was presented in Table 3. There was no significant difference (p > 0.05) in the BW of broilers at 1, 28, and 56 days of age among the groups; however, the BW of broilers at 84 days of age significantly increased in the VD group compared to the CON group (p < 0.05). VD3 supplementation did not significantly affect (p > 0.05) the ADFI and FCR (feed/gain) of broilers at any growth stages, nor the ADG of broilers aged 1–28 days and 29–56 days. However, supplementary VD3 significantly increased (p < 0.05) the ADG in broilers aged 57–84 days and 1–84 days.
The carcass performance of broilers at 84 days of age and the fat deposition at 28, 56 and 84 days of age were evaluated (Table 4). Broilers fed a diet supplemented with VD3 exhibited a significantly higher (p < 0.05) leg muscle rate and liver organ index compared to the CON group at 84 days of age. However, there were no significant differences (p > 0.05) in the other tested traits.
Table 4. Effects of VD3 supplementation on the carcass performance and fat deposition in broilers (%).
Compared to the CON group, the supplementary VD3 significantly (p < 0.05) reduced the abdominal fat rate and increased the fat content in the liver, breast muscle, and leg muscle of broilers at 84 days of age, with no significant effects observed at 28 and 56 days of age (p > 0.05). This indicated that supplementation with VD3 in diet had varying effects on fat deposition in different tissues of broilers.
The supplementary VD3 significantly increased (p < 0.05) the content of TG and glycogen while decreasing (p < 0.05) the TC content in the liver of 28- and 84-day-old broilers, and also reduced (p < 0.05) the FFA content in the liver of 84-day-old broilers (Figures 1A–D). However, supplementary VD3 did not affect (p > 0.05) these parameters in the liver of 56-day-old broilers. Notably, all these parameters were significantly higher (p < 0.05) in 84-day-old broilers compared to those that were 28 and 56 days old.
Figure 1. Effect of dietary VD3 supplementation on the liver and serum parameters in broilers. (A) The content of TG in the liver; (B) TC content in the liver; (C) liver glycogen content in the liver; (D) FFA content in the liver; (E) TG content in serum; (F) TC content in serum; (G) HDL-C content in serum; (H) LDL-C content in serum; (I) VLDL-C content in serum; (J) glucose content in serum; (K) insulin content in serum; (L) VD content in serum. Data are presented as mean ± SEM, n = 14. *p < 0.05; **p < 0.01.
The supplementary VD3 significantly increased (p < 0.05) the content of TG, glucose, insulin and VD in serum of 28-, 56- and 84-day-old broilers, as well as the content of TC, HDL-C and LDL-C in serum of 84-day-old broilers, and the VLDL-C content in serum of 56- and 84-day-old broilers (Figures 1E–L). This indicates that at different growth stages, VD3 increased serum lipid levels and promoted lipid transport in the blood, especially in the middle and late stages of growth.
Considering that the liver is the primary site for lipid biosynthesis in poultry, the expression of genes and the activity of enzymes involved in lipid metabolism in the liver were detected (Figure 2). For broilers at 28, 56 and 84 d of age, supplementary VD3 increased significantly (p < 0.05) the mRNA expression of the lipogenic gene mTOR, SREBP-1c, FAS and ACC, the lipid oxidizing gene AMPK, PPARα, CPT-1α and ACO, the VLDL assembly protein encoding-gene ApoB and MTTP, the cholesterol catabolism gene CYP7A1. Conversely, the expression of the cholesterol synthesis gene SREBP2, and the lipolytic gene HSL and ATGL was significantly decreased (p < 0.01) by the supplementary VD3 (Figure 2A). Furthermore, the supplementary VD3 significantly increased (p < 0.01) the activity of FAS, ACC and CPT-1α in the liver of broilers at 28, 56 and 84 days of age (Figure 2B).
Figure 2. Effect of dietary VD3 supplementation on the expression of genes and the activity of enzymes involved in lipid metabolism in the liver. (A) The expression of lipid metabolism genes. (B) The activity of FAS, ACC and CPT-1α. Data are presented as mean ± SEM (n = 14). *p < 0.05; **p < 0.01.
To understand the effects of supplementary VD3 on lipid metabolism in extrahepatic tissues, the mRNA expression of lipid metabolism genes in abdominal adipose tissue, breast and leg muscle tissues was detected (Figure 3). Supplementary VD3 decreased significantly (p < 0.01) the expression of lipogenic gene mTOR, SREBP-1c, FAS and ACC, lipid uptake gene LPL and FATP1, and increased (p < 0.05) the expression of the lipolytic gene HSL and ATGL, and lipid oxidizing gene PPARα and CPT-1α in abdominal adipose tissue of broilers at 28, 56 and 84 days of age, as well as AMPK and ACO in broilers at 56 and 84 days of age (Figure 3A). Additionally, the LPL activity in the abdominal adipose tissue of broilers at 84 days of age was significantly decreased (p < 0.05) by supplementary VD3 (Figure 3D).
Figure 3. Effect of dietary VD3 supplementation on gene expression and enzyme activity related to lipid metabolism in extrahepatic tissues. (A) The expression of genes in abdominal adipose tissue. (B) The expression of genes in breast muscle. (C) The expression of genes in leg muscle. (D) The LPL activity in abdominal adipose tissue. Data are presented as mean ± SEM (n = 14). *p < 0.05; **p < 0.01.
The supplementary VD3 significantly increased (p < 0.01) the LPL expression in breast muscle of broilers at 56 and 84 days of age, and the expression of LPL in leg muscle and FATP1 in both breast muscle and leg muscle of broilers at 28, 56 and 84 days of age (Figures 3B,C).
A total of 15,411 operational taxonomic units (OTUs) were identified in 36 cecal content samples of broilers from the CON and VD groups with 97% confidence interval (Figure 4A). The Rarefaction curves and Shannon curves of cecal samples flattened out with the increasing numbers of sequences sampled, indicating that the sample size was reasonable, and the sequencing depth was sufficient for all samples based on a saturated trend (Figures 4B,C).
Figure 4. Effect of dietary VD3 supplementation on cecal microbiota diversity in broiler. (A) OUT number. (B) Rarefaction curve. (C) Shannon-Wiener curve. (D) ACE index. (E) Chao1 index. (F) Shannon index. (G) Simpson index. (H–J) PCoA based on OTUs at 28, 56 and 84 days of age, respectively. The percent variation explained by each principal coordinate is indicated on the axes. (K–M) NMDS based on OTUs at 28, 56 and 84 days of age. *p < 0.05; **p < 0.01.
Alpha diversity analysis was used to assess the richness and diversity of gut microbiota (26). Both the ACE and Chao1 indexes represent the richness of the microbial community. Dietary VD3 supplementation significantly increased ACE and Chao1 indexes in 28-day-old broilers compared to the CON group, while showing no effects in 56- and 84-day-old broilers (Figures 4D,E). Both Shannon and Simpson indexes were significantly higher in the VD group than in the CON group at 28 and 56 days of age (p < 0.05), while there was no significant difference at 84 days of age (Figures 4F,G). These results indicated that VD3 influenced α-diversity of the cecum microbiota in broiler chickens during the early growth stage.
Beta diversity, a measure of variance in taxa composition between sampling sites (27), was visualized by plotting the distances between samples using principal coordinates analysis (PCoA) and non-metric multi-dimensional scaling (NMDS) biplot. The PCoA and the NMSD analysis exhibited that at 28 days of age, there was not a complete separation in the cecal microbial community between the CON and VD groups (Figures 4H,K). At 56 and 84 days of age, a distinct separation between the groups was observed, with samples clustering within each group (Figures 4I,J,L,M). These results indicated that supplementary VD3 had an impact on the composition of cecal microbiota in broilers.
Based on the results of OUT delineation and taxonomic status identification, the specific composition of each sample at each taxonomic level could be obtained. At the phylum level, a total of 19 microbial phyla were identified in the cecal contents of broilers from the two groups (Figures 5A–C). We selected the dominant bacterial phyla that exhibited relatively high abundance across all three growth stages of broilers for analysis, resulting in the identification of six phyla: Bacteroidota, Firmicutes, Proteobacteria, Verrucomicrobiota, Deferribacterota, and Desulfobacterota (Figures 5D–I).
Figure 5. The relative abundance taxa of cecal microbiota in broilers at the phylum level. (A–C) Relative abundance taxa at 28, 56 and 84 days of age. (D–I) The relative abundance of Bacteroidota, Firmicutes, Proteobacteria, Verrucomicrobiota, Deferribacterota and Desulfobacterota, respectively. (J) The ratio of Firmicutes/ Bacteroidota. In panels (D–J), data are presented as mean ± SEM (n = 6). *p < 0.05; **p < 0.01.
At 28 days of age, compared to the CON group, supplementary VD3 increased significantly (p < 0.05) the relative abundance of Firmicutes (38.49% vs. 46.92%), while reduced (p < 0.05) that of Proteobacteria (1.04% vs. 0.74%), Deferribacterota (1.70% vs. 0.24%), and Desulfobacterota (1.72% vs. 1.21%). Additionally, the ratio of the Firmicutes / Bacteroidota (F/B; 0.72 vs. 0.95) was significantly increased (p < 0.05; Figure 5J), while there was no significant change in the relative abundance of Bacteroidota (53.75% vs. 49.53%). At 56 day of age, supplementary VD3 increased significantly (p < 0.01) the relative abundance of Bacteroidota (42.41% vs. 52.33%), and reduced (p < 0.01) that of Deferribacterta (3.74% vs. 0.33%), Desulfobacterota (1.90% vs. 1.15%), and Verrucomicrobiota (7.74% vs. 2.84%). The ratio of the F / B (0.93 vs. 0.73) was significantly decreased (p < 0.05), while there was no significant change in the relative abundance of Firmicutes (39.33% vs. 38.27%). At 84 days of age, supplementary VD3 increased significantly (p < 0.05) the relative abundance of Bacteroidota (43.49% vs. 48.62%), and reduced (p < 0.01) that of Proteobacteria (1.15% vs. 0.72%), Deferribacterota (0.95% vs. 0.27%), and Verrucomicrobiota (7.18% vs. 2.46%). However, there were no significant changes in the F / B ratio. Obviously, the VD3 supplementation had a notable impact on the cecal microbiota composition at the phylum level in broilers.
The top 15 genera by relative abundance in the cecal microbiota were presented in Figure 6. At 28 days of age, the most dominant bacterial genera in the CON and VD groups were Bacteroides, Megamonas, Rikenellaceae_RC9_gut_group, [Ruminococcus]_torques_group and Barnesiella. The relative abundance of Bacteroides significantly increased (p < 0.05), while that of Megamonas, Rikenellaceae_RC9_gut_group and Barnesiella significantly decreased (p < 0.05) in the VD group (Supplementary Table S1). At 56 days of age, the most dominant genera were Bacteroides, Rikenellaceae_RC9_gut_group, uncultured_Verrucomicrobia_bacterium, Parabacteroides, and unclassified_Prevotellaceae in the CON and VD groups. The relative abundance of Rikenellaceae_RC9_gut_group and unclassified_Prevotellaceae significantly increased (p < 0.05), while that of uncultured_Verrucomicrobia_bacterium significantly decreased (p < 0.05) in the VD group (Supplementary Table S2). At 84 days of age, the most dominant genera were Bacteroides, Rikenellaceae_RC9_gut_group, uncultured_rumen_bacterium, uncultured_ Verrucomicrobia_bacterium, and Parabacteroides. The relative abundance of Bacteroides and Rikenellaceae_RC9_gut_group significantly increased (p < 0.05), while that of uncultured_rumen_bacterium and uncultured_Verrucomicrobia_bacterium reduced significantly (p < 0.05) in the VD group (Supplementary Table S3). Moreover, supplementary VD3 also enhanced (p < 0.05) the relative abundance of Ligilactobacillus at day 28, Faecalibacterium and Phascolarctobacterium at day 56, and Faecalibacterium and Lachnoclostridium at day 84.
Figure 6. The relative abundance of cecal microbiota at the genus level. (A–C) Relative abundance taxa at 28, 56, and 84 days of age. The top 15 genera by relative abundance were shown, while the remaining genera were categorized as “Others”.
The Linear Discriminant Analysis (LDA) combined with LDA Effect Size (LEfSe) analysis was employed to further examine the changes in the cecum microbiota at both the genus and species levels in broilers (LDA scores >3.5). At 28 days of age, there were 11 biomarkers enriched in the VD group, such as g_Bacteroides, g_Ligilactobacillus, s_Ligilactobacillus_salivarius, s_Lactobacillus_intestinalis, and g_Veillonella, and 4 biomarkers enriched in CON group, such as g_Blautia, and g_ Fusobacterium (Figures 7A,B). At 56 days of age, g_Rikenellaceae_RC9_gut_group was identified as biomarker in the VD group (Figures 7C,D). At 84 days of age, there were 10 biomarkers in the VD group, including g_Bacteroides, g_Lachnoclostridium, g_Faecalibacterium and g_Ruminococcus_torques_group, and the CON group was enriched with g_Prevotellaceae_UCG_001, g_Prevotellaceae_Ga6A1_group, g_Mucispirillum (Figures 7E,F).
Figure 7. LEfSe taxonomic cladogram and LDA score of cecal microbiota. (A,B) LEfSe taxonomic cladogram and LDA score at 28 days of age. (C,D) LEfSe taxonomic cladogram and LDA score at 56 days of age. (E,F) LEfSe taxonomic cladogram and LDA score at 84 days of age. The circles radiating from the center to the outer edges of the evolutionary branch map represent classification levels from phylum to species, with the circle’s diameter proportional to the taxon’s abundance. The yellow nodes represent taxonomic units that exhibit no significant differences between groups. The LDA histograms display the LDA scores, and the difference is significant when LDA >3.5. g, genus; s, species.
To investigate the relationships between intestinal microbiota and lipid metabolism in broilers, we performed a Spearman correlation analysis (Figure 8) based on the aforementioned cecal biomarker microbiota at the genus level, lipid metabolism indicators, and gene expression data to determine the microbes linked to the regulatory effects of VD3 on lipid metabolism. At 28 days of age, the content of TG, glucose, and insulin in the serum, glycogen content and the expression of the mTORC1/SREBP-1c pathway (mTOR, SREBP-1c, and ACC), AMPK/PPARα/CPT1 pathway (AMPK, PPARα, CPT-1α, and ACO), MTTP and CYP7A1 in the liver, as well as the expression of lipolytic pathway (HSL and ATGL) in the abdominal adipose tissue, and FATP1 in breast muscle, LPL in leg muscle were positively correlated with the relative abundance of Bacteroides, Ligilactobacillus and Veillonella. Moreover, the liver TC content, and the expression of mTOR, SREBP-1c and FATP1 in the abdominal adipose tissue were negatively correlated with Bacteroides and Ligilactobacillus (Figure 8A).
Figure 8. Correlation heatmap of cecal biomarker bacteria and differential lipid metabolism indicators of broilers. (A) 28 days of age; (B) 56 days of age; (C) 84 days of age. Spearman’s correlations were calculated between cecal biomarker bacterial genera and all significantly different fat content, gene expression in tissues, and serum parameters. Colors of squares represent r values of Spearman’s correlation coefficient, with red indicating a positive correlation and green signifying a negative correlation. *p < 0.05, **p < 0.01, ***p < 0.001.
At 56 days of age, Rikenellaceae_RC9_gut_group was positively correlated with the expression of ApoB in the liver, as well as PPARα, HSL and ATGL in abdominal adipose tissue, exhibiting a negative correlation with the expression of SREBP2 in the liver, mTOR and FAS in the abdominal adipose tissue (Figure 8B).
At 84 days of age, Lachnoclostridium and Faecalibacterium exhibited a positive correlation with the fat content in the liver and leg muscle, serum metabolism parameters (TG, TC, LDL-C, VLDL-C, glucose, and insulin), liver TG, the expression of mTORC1/SREBP-1c pathway (mTOR, SREBP-1c, FAS, and ACC) and AMPK/PPARα/CPT1 pathway (AMPK, PPARα, and CPT1α), VLDL assembly (ApoB and MTTP), and CYP7A1 in liver, and AMPK/PPARα/CPT1 pathway (AMPK, PPARα, and ACO), lipolytic pathway (HSL and ATGL) in the abdominal adipose tissue, and extrahepatic lipid uptake (FATP1 in breast muscle and LPL in leg muscle), while exhibiting a negative correlation with other indicators, such as the abdominal fat rate, the TC and FFAs content and the expression of SREBP2 in the liver, and the expression of mTORC1/SREBP-1c pathway (mTOR, SREBP-1c, FAS, and ACC), LPL, and FATP1 in the abdominal adipose tissue (Figure 8C). However, contrasting results were observed for Prevotellaceae_UCG_001 in comparison to these two genera. Additionally, Bacteroides was positively correlated with fat content in breast muscle, serum insulin and liver glycogen content, the expression of mTOR, SREBP-1c, ACC, PPARα and MTTP in the liver, and ATGL in abdominal adipose tissue, and FATP1 in breast and leg muscle, while showing a negative correlation with liver TC, the expression of SREBP2 in the liver, and FATP1 in abdominal adipose tissue. However, the opposite result to Bacteroides was observed for Prevotellaceae_UCG_001.
To gain deeper insights into the variations in function of cecal microbiota between the CON and VD group, we performed KEGG enrichment prediction analysis on 16S rDNA sequencing data using the Integrated Microbial Genomes (IMG) database2 by PICRUST2. At 28 days of age, the relative abundance of pathways related to cellular processes (such as mismatch repair, pyrimidine metabolism and homologous recombination) and glycolysis / gluconeogenesis was significantly higher, while there was a decrease (p < 0.05) in the abundance of secondary metabolite biosynthesis in the VD group (Figure 9A). At 56 days of age, although several glucose metabolism and amino acid biosynthesis pathways were enriched, none were statistically significant (p > 0.05) between groups (Supplementary Figure S1). At 84 days of age, the relative abundance of energy metabolic pathways, such as fructose and mannose metabolism, pentose phosphate pathway, starch and sucrose metabolism, were significantly increased, while that of phenylalanine, tyrosine and tryptophan biosynthesis was decreased (p < 0.05) in the VD group (Figure 9B).
Figure 9. Differential KEGG level 3 metabolic pathways between groups. (A) 28 days of age; (B) 84 days of age. The p-value on the right is derived from G-test in the statistical analysis of taxonomic and functional profiles (STAMP) software, where p-value <0.05 indicates a significant difference.
In contemporary broiler production, excessive accumulation of abdominal fat is a significant factor influencing the carcass quality and feed conversion of broiler chickens. Increasing evidences have shown that VD3 regulated lipid metabolism in humans and animals (12, 28), and that there was a significant association between VD3 levels, gut microbiota and lipid metabolism (18–20). However, the effect of VD3 on fat accumulation and lipid metabolism in broilers has been less frequently reported. In this study, supplementation with VD3 increased ADG of broilers aged 57–84 and 1–84 days, and the final body weight and leg muscle rate at 84 days of age. However, the ADFI during each growth period was not significantly influenced by VD3 supplementation, resulting in a non-significant FCR. Additionally, the supplementary VD3 significantly reduced abdominal fat rate and increased the fat content in liver, leg and breast muscle of broilers at 84 days of age, while having no effects on those of broilers at 28 and 56 d of age. Consistent with this, it was reported that VD3 promoted growth performance and bone development by exerting a series of regulatory effects in broilers (24, 29). Considering that serum VD3 levels were continuously elevated by supplemental VD3, we proposed that the VD3 added to the basal diet enhanced growth performance, as well as improved carcass quality by differentially influencing fat accumulation in various tissues of broilers, with these effects mainly occurring during the finishing period.
Additionally, it should be noted that VD3 stored in edible parts, such as muscle and adipose tissue, did not present food safety issues under the supplementary dosage of 3,750 IU/kg of VD3 in this study. VD3 mainly store in fat-rich tissues and organs due to its fat-soluble property. The liver and adipose tissue of humans and animals expressed and secreted 24-hydroxylase CYP24A1 (30). CYP24A1 catalyzed the breakdown of 25(OH)D3 and 1,25(OH)2D3 into calcitroic acid and other inactive metabolites, and 1,25(OH)2D3 induced CYP24A1, promoting its own degradation and ultimately self-regulating through inactivation pathways to maintain homeostasis (31). Morrisey et al. (32) evaluated VD3 toxicity through renal histopathological examination, a sensitive method for detecting VD toxicity, and showed that a dose of 400,000 IU/kg VD3 resulted in calcification of renal tubules in chickens, whereas 40,000 IU/kg VD3 exhibited no toxicity, which was determined to be the maximum food safety level. Additionally, a VD3 addition level of 12,000 IU/kg showed no adverse effects on laying hens and their eggs (33).
The lipid metabolism in the animals is in dynamic equilibrium, with lipid levels in the liver, peripheral tissues, and blood being interconnected and mutually affecting each other (34). Liver lipid levels served as a crucial indicator of the overall lipid metabolism in broilers (35). The supplementary VD3 increased the liver TG and glycogen levels, as well as the liver organ index, while reducing TC levels in broilers at 28 and 84 days of age, along with a decrease in FFA levels at 84 days of age. The increased relative liver weight indicated a rise in lipid content rather than liver enlargement, as it has been established that VD3 doses below 40,000 IU/kg are non-toxic to broiler chickens (32).
Poultry primarily synthesize lipids in the liver, and substantial evidence has shown that the level of liver lipid metabolism depends on de novo lipogenesis (DNL), β-oxidation, and VLDL export (36, 37). To gain a deeper understanding of the effect of VD3 on liver lipid metabolism, we analyzed the expression of genes and the activity of enzymes related to lipid metabolism. The supplementary VD3 significantly up-regulated the expression of mTOR, SREBP-1c, FAS and ACC, and increased the activity of FAS and ACC enzymes in the liver. In the same way, the expression of AMPK, PPARα, CPT-1α and ACO and the activity of CPT-1α enzyme in the liver were significantly increased by the supplementary VD3. The mTORC1/SREBP-1c pathway was closely related to fat accumulation in animals (38). The transcription factor SREBP-1c regulated glycolysis and lipogenesis by modulating the expression of its target genes, such ACC and FAS (39, 40). The mTOR pathway was activated by signals like insulin, which led to the process of SREBP-1c from its precursor to a mature protein and its translocation to the nucleus, thereby promoting the transcription of lipogenic genes (39). PPARα, a type of nuclear receptor, is activated by free fatty acids and various lipid molecules, stimulating to the transcription of genes involved in fatty acid transport, β-oxidation, and lipoprotein metabolism (41). AMPK activated PPARα signaling pathways, promoting fatty acid oxidation (42). AMPK activation enhanced lipid metabolism by switching from carbohydrate metabolism to lipid oxidation, leading to a reduction of both liver TG and obesity in mice (43). Both CPT-1α and ACO, as the target genes of PPARα, were involved in β-oxidation of fatty acids. CPT-1α was the rate-limiting enzyme for fatty acid oxidation and served as the delivery of long-chain fatty acids from the cytoplasm into the mitochondria (44, 45). It has been demonstrated that the activation of the AMPK/PPARα/CPT1 pathway stimulated the lipid metabolism and reduced lipid accumulation in obese mice (46). Additionally, the supplementation with VD3 reduced the expression of SREBP2 and increased the expression of CYP7A1 in the liver. The transcription factor SREBP2 stimulated cholesterol biosynthesis, while the CYP7A1 enzyme was responsible for converting cholesterol into bile acids in the liver of poultry (47). Therefore, it is reasonable to assume that supplementation with VD3 could enhance glucose metabolism, DNL, and lipid oxidation, all of which contributed to an increase in TG and glycogen levels in the liver of broilers at 28 and 84 days of age.
This was also supported by elevated insulin concentration, and reduced the level of FFAs and the expression of lipolytic gene HSL and ATGL in liver. The main function of insulin in hepatic lipid metabolism is to regulate lipid storage by exerting control over DNL, FFA oxidation and VLDL export (48). Hormone-sensitive lipase (HSL) and adipose triglyceride lipase (ATGL) are key enzymes in the lipolytic pathway, hydrolyzing stored triglycerides to release FFAs for β-oxidation (49, 50). Insulin profoundly inhibited lipolysis primarily by suppressing the activity of HSL and stimulated glycogen accumulation through a coordinated increase in glucose transport and glycogen synthesis (51).
Conversely, VD3 could decrease TC levels by inhibiting cholesterol synthesis and promoting the conversion of cholesterol to bile acid efflux in the liver. However, the alterations in gene expression and enzyme activity related to lipid metabolism in the liver of broilers at 56 days of age did not result in significant changes in TG content, which could be attributed to the fact that this stage is in a period of rapid growth and requires increased energy to support growth.
The lipids produced in the liver were transported by the bloodstream to extrahepatic tissues for storage or oxidation. Serum TG and TC levels are important indicators of lipid transport in the body. HDL, LDL and VLDL are all types of lipoproteins responsible for transporting lipids through the bloodstream, which can provide an insight into overall lipid metabolism. HDL is involved in cholesterol removal and transport triglycerides back to the liver, LDL is responsible for delivering cholesterol to cells, and VLDL transports triglycerides from the liver to other tissues (52). Lipoproteins are composed of a lipid core encased in a layer of phospholipids and apolipoproteins, with the liver serving as a crucial site for the synthesis of apolipoproteins. Both Apolipoprotein B (ApoB) and Microsomal Triglyceride Transfer Protein (MTTP) are critical components involved in the assembly of lipoproteins (53). In this study, the supplementary VD3 elevated serum TG and VLDL levels, indicating that it could enhance the transport of triglycerides to peripheral tissues. Additionally, the elevated serum HDL levels at 84 days of age indicated an increase in lipid transport back to the liver, which contributed to a rise in liver TG levels, attributed to the rise in body fat mass during the later stage of growth. These results were also supported by the expression of ApoB and MTTP in the liver, which was elevated by the supplementary VD3 in this study.
The accumulation of triglycerides within lipid droplets was one of the causes of adipose tissue expansion, which was positively correlated with abdominal fat mass (54). The sources of fat in animal tissues include cellular uptake and DNL. DNL in the liver supplied the necessary fatty acids to other tissues by cellular uptake (55). However, the adipose tissue was also an important site for DNL in broilers (56). Fatty acids for cellular uptake are derived from the hydrolysis of blood triglycerides mediated by lipoprotein lipase (LPL) (54). Triglycerides produced in the liver were transported by apolipoproteins through the bloodstream to extrahepatic tissues (such as adipose tissue and muscle) (57). LPL catalyzed the hydrolysis of the triglycerides in VLDL to release FFAs, and promoted the uptake of fatty acids by tissues (54). FATP1 promoted cellular fatty acid uptake and adipocyte hypertrophy by mediating the synthesis of fatty acids into triglycerides (58). The supplementary VD3 suppressed the expression of mTOR/SREBP-1c pathway related genes (mTOR, SREBP-1c, FAS and ACC), while increased the expression of AMPK/PPARα/CPT-1α pathway related genes (AMPK, PPARα, CPT-1α and ACO) and lipolytic enzyme-encoding genes (HSL and ATGL), suggesting that VD3 could inhibit lipogenesis, and promote lipolysis and fatty acid β-oxidation in abdominal adipose tissue. Moreover, we found also that VD3 significantly downregulated the expression of LPL and FATP1, and decreased the activity of LPL enzyme in abdominal adipose tissue of broilers at 84 days of age, suggesting that VD3 suppressed the uptake of lipids transported from the liver by abdominal adipocytes in broilers. Consequently, the supplementary VD3 decreased the abdominal fat rate by inhibiting DNL and cellular uptake pathways while enhancing the lipolysis and β-oxidation pathway.
Unlike abdominal fat reduction, the supplementary VD3 significantly increased fat content in breast and leg muscles. Elevated liver TG levels, blood lipid transport, and the expression of LPL and FATP1 in breast and leg muscles due to VD3 could result in an increased cellular lipid uptake, which partially accounted for the rise in muscle fat content. However, muscle tissue mainly consists of myocytes, adipocytes, and connective tissue cells. Unlike abdominal fat, the formation of IMF is more complex due to the interference of myocytes (56). Thus, our experimental evidence was insufficient to fully clarify the promotion of muscle fat deposition by supplementary VD3 in broilers.
The supplementary VD3 differentially influenced lipid metabolism and fat deposition in the liver, muscle and adipose tissue of broilers, which could reflect tissue-specific effects of VD3. As previously discussed, VD3 enhanced both the mTORC1/SREBP-1c and AMPK/PPARα/CPT-1α signaling pathways while decreasing the expression of lipolytic genes in the liver. Conversely, in adipose tissue, the expression of the mTOR/SREBP-1c pathway was suppressed, whereas the expression of the AMPK/PPARα/CPT-1α pathway and lipolytic genes was elevated by supplementary VD3. Researches have confirmed that nutrients affect lipid metabolism and fat distribution by influencing the expression of related genes in the liver, muscle, and adipose tissues of animals in a tissue-specific manner (59, 60). VD3 could regulate lipid metabolism through multiple pathways, and its genomic and epigenetic effects that depend on VDR, as well as its non-genomic effects, have been reviewed in detail (61). The finding of VD3 that elevated insulin levels in this study could provide anther cue for understanding the regulation of VD on lipid metabolism, but further research is needed.
VD3 supplementation significantly altered α-diversity of cecal microbiota. We also observed that VD3 significantly affected the structure of cecal microbiota in broilers, especially in the grower and finisher stage. PICRUSt2 functional prediction for cecal microbiota in broilers at 28 days of age indicated that VD3 increased the abundance of metabolic pathways involved in the cellular processes and energy metabolism. The age of 1–28 days (early growth stage) is a critical period for shaping gut microbiome composition, and the enhancement of VD3 on the cellular processes could contribute to a healthier microbial population, reducing the risk of mutations and promoting overall microbiome stability. For broilers at 84 days of age, VD3 increased the abundance of energy metabolic pathways, while decreasing the biosynthesis of amino acids, all of which contributed an increased capacity of the microbiota to utilize energy substances. This indicated that VD3 could improve the availability of nutrients and energy storage by modulating the metabolic activities of the cecal microbiota in the late growth stage of broilers.
The cecal microbiota significantly contributed to fat deposition and could independently account for 21% of the variance in the abdominal fat mass after correcting for host genetic effects (62), and the enterohepatic axis had a significant effect on lipid metabolism in poultry (63). Previous study found that VD3 reduced the expression of lipogenic genes in the liver by regulating the gut microbiota in mice (64). Here, we primarily discussed the potential effects of VD3 on lipid metabolism by influencing cecal microbiota composition in broilers.
The composition of cecal microbiota at the phyla varied significantly across different growth stages in broilers, but Firmicutes and Bacteroidetes were always most predominant, which was consistent with previous study on broilers (26). The relative abundance of Firmicutes at 28 days of age and that of Bacteroidota at 56 and 84 days of age were increased by the supplementary VD3, while showing inconsistent changes in F/B ratio. Firmicutes and Bacteroidetes in the gut collectively influenced the host’s energy absorption and storage, and the F/B ratio affected the host’s ability to obtain energy from feed (65). Both Firmicutes and Bacteroidetes promoted fat deposition in animals, and Firmicutes exhibited a stronger promotion compared to Bacteroidetes (66). Nakamoto et al. (67) found that both Firmicutes and Bacteroidetes were associated with liver lipid metabolism and stimulated the expression of lipogenic enzyme-encoding gene such as FAS, stearoyl-CoA desaturase-1 (SCD1), and diacylglycerol O-acyltransferase 2 (DGAT2) in mice. An increase in Firmicutes enhanced carbohydrate metabolism and energy absorption (68), while Bacteroidetes fermented indigestible polysaccharides in the gut, providing energy to the host (69). Gut Firmicutes and Bacteroidetes have been shown to influence lipid metabolism and obesity in animals by producing SCFAs (70). Additionally, we also observed that VD3 reduced the relative abundance of these results suggested that the supplementary VD3 could enhance energy utilization and lipogenesis in broilers by affecting the composition of gut microbiota.
Based on the analysis of cecal microbial composition at the genus levels, we found that Bacteroides, Rikenellaceae_RC9_gut_group and Prevotellaceae_UCG_001 were all dominant bacterial genera in broilers at three different ages, all of which belong to the Bacteroidetes. VD3 significantly increased the abundance of Bacteroides in the cecum of broilers at 28 and 84 d of age, and LEfSe analysis also showed that Bacteroides was a biomarker for the VD group. Bacteroides species utilized various plant polysaccharides and host-derived glycans to produce beneficial end products, fostering the mutualistic relationship between humans and their resident intestinal Bacteroides (71). Bacteroides supplementation improved glucose tolerance and intestinal barrier structure, and reduced the adiposity in female mice fed a high-fat diet (HFD) (72). Moreover, Bacteroides reduced body weight gain, and cholesterol and triglyceride levels in the liver and serum in HFD-fed mice (73). In this study, we found that Bacteroides was positively correlated with glycogen content and the expression of the mTOR/SREBP-1c and AMPK/PPARα/CPT1 pathways in the liver of 28- and 84-day-old broilers. However, Bacteroides was negatively correlated with the mTOR/SREBP-1c pathway, while showing a positive correlation with the lipolysis pathway in the abdominal adipose tissue of 28-day-old broilers.
Rikenellaceae_RC9_gut_group produced SCFAs and was correlated with a lot of lipid metabolism pathways (74). It was found that the abundance of Rikenellaceae_RC9_gut_group was low in obese mice and negatively correlated with serum glucose, TG, TC, LDL-C, insulin, and hepatic TC (75), and positively correlated with fecal butyrate levels (76). In contrast, the reduction of Rikenellaceae_RC9_gut_group in the gut was accompanied by a decrease in serum levels of TC, TG, and LDL-C in weaned piglets (77). In this study, the supplementary VD3 significantly increased the abundance of Rikenellaceae_RC9_gut_group at 56 and 84 days of age, while decreasing it at 28 days of age, suggesting that this genus could be relevant one in VD3 regulation. Furthermore, Rikenellaceae_RC9_gut_group, identified as a marker genus of VD group at 56 days of age, showed a positive correlation with the expression of PPARα, HSL and ATGL, while exhibiting a negative correlation with the expression of mTOR and FAS in the abdominal adipose tissue.
Prevotellaceae_UCG_001, a member of the family Prevotellaceae, generated enzymes that degrade cellulose and xylan to produce SCFAs, which play a protective role against gut inflammation (78). The abundance of Prevotellaceae_UCG_001 was positively correlated with the AMPK signaling pathway that promoted glycolysis and fatty acid oxidation in mice (79). In this study, the abundance of Prevotellaceae_UCG_001 in broilers at 84 days of age was reduced by the supplementary VD3, and its correlation with lipid metabolism pathways was contrary to that of Bacteroides, except for a positive correlation with the abdominal fat rate. Bacteroides and Prevotellaceae have been reported to exhibit mutual antagonism or competition in the distal gut (69). The decrease in the abundance of Prevotellaceae_UCG_001 might be related to the increase in the abundance of Bacteroides by supplementation with VD3 in this study. These results indicated that the regulation of VD3 on lipid metabolism, including the enhanced lipid metabolism in the liver, and the reduced lipogenesis and the enhanced lipolysis and lipid oxidation in adipose tissue, was linked to an increased abundance of Bacteroides and Rikenellaceae_RC9_gut_group, along with a decreased abundance of Prevotellaceae_UCG_001 in the cecum of broilers.
In this study, we also observed that VD3 increased the abundance of Lachnoclostridium at 84 days of age and that of Faecalibacterium at 56 and 84 days of age. These two bacterial genera belong to the phylum Firmicutes and contribute to the production of acetate and butyrate (80), which serve as energy sources for gut epithelial cells, and influence host’s lipid metabolism by impacting glucagon-like peptide-1 (GLP-1) and peptide YY (PYY). Although SCFAs are not considered a major energy source for broilers, current evidence suggested that gut microbiota, including Faecalibacterium and Lachnoclostridium, affected overall lipid metabolism through the gut-brain axis in humans (81). SCFAs affected adipose tissue biology and physiology, potentially influencing lipid metabolism (82). Studies have demonstrated that the acetate inhibited body fat accumulation and hepatic lipid metabolism in mice by enhancing the expression of PPARα, CPT-1 and ACO in the liver (83). An increased abundance of gut Lachnoclostridium was associated with enhanced SCFAs production and reduced blood glucose levels in rats (84). However, Nogal et al. (85) reported that a higher abundance of Lachnoclostridium decreased circulating acetate levels, resulting in an increase in visceral fat in humans. Additionally, Faecalibacterium prausnitzii, the most recognized species in Faecalibacterium, enhanced fatty acid oxidation and adiponectin signaling in the liver and visceral adipose tissue, while reducing adipocyte size in HFD-fed mice (86). The correlation analysis revealed that both Lachnoclostridium and Faecalibacterium were positively correlated with the fat and TG content, the mTOR/SREBP-1c and the AMPK/PPARα/CPT1 pathway in the liver, as well as serum parameters related to lipid metabolism and VLDL assembly. However, they exhibited a negative correlation with abdominal fat rate, mTOR/SREBP-1c pathway, and cellular lipid uptake in abdominal adipose tissue, while showing a positive correlation with lipolysis and AMPK/PPARα/CPT1 pathway. Conversely, these two bacterial genera were positively correlated with the fat content and cellular lipid uptake in breast and leg muscles. These results indicated that VD3 could differentially regulate lipid metabolism in different tissues by increasing the abundance of Lachnoclostridium and Faecalibacterium in the cecum of broilers, including that enhanced the lipid metabolism and transport in the liver and fat accumulation in muscles, as well as reduced fat deposition in adipose tissue by inhibiting DNL and lipid uptake and promoting lipolysis and fatty acid oxidation.
To sum up, VD3 could differentially regulate lipid metabolism and fat deposition in different tissues by influencing the composition of intestine microbiota in broilers. The increased abundance of Bacteroides, Rikenellaceae_RC9_gut_group, Lachnoclostridium and Faecalibacterium was linked to enhanced lipid metabolism and transport in the liver, as well as fat deposition in muscles. However, the increased abundance of Bacteroides and Rikenellaceae_RC9_gut_group, and the decreased abundance of Prevotellaceae_UCG_001, was associated with the reduced lipogenesis and enhanced lipolysis and lipid oxidation in adipose tissue.
In summary, the addition of VD3 on the basal diet enhanced growth performance, increased IMF in breast and leg muscles, and reduced the abdominal fat rate leading to an improvement in carcass quality by differentially regulating lipid metabolism in various tissues of broilers, with these effects primarily occurring during the finishing period. Moreover, the regulation of supplementary VD3 on lipid metabolism could was closely associated with an increased abundance of Firmicutes including Lachnoclostridium and Faecalibacterium, and Bacteroidetes including Bacteroides and Rikenellaceae_RC9_gut_group.
The datasets presented in this study can be found in online repositories. The names of therepository/repositories and accession number(s) can be found at: https://www.ncbi.nlm.nih.gov/, PRJNA1195667.
The animal studies were approved by the Northwest Minzu University Animal Care and Use Committee. The studies were conducted in accordance with the local legislation and institutional requirements. Written informed consent was obtained from the owners for the participation of their animals in this study.
JLi: Conceptualization, Data curation, Formal analysis, Investigation, Software, Validation, Writing – original draft, Writing – review & editing. XL: Methodology, Resources, Validation, Writing – original draft. JT: Data curation, Methodology, Validation, Writing – original draft. LX: Software, Visualization, Writing – original draft. YC: Data curation, Methodology, Writing – original draft. SJ: Resources, Software, Writing – original draft. GZ: Conceptualization, Funding acquisition, Investigation, Supervision, Writing – review & editing. JLu: Conceptualization, Project administration, Supervision, Writing – review & editing, Methodology.
The author(s) declare financial support was received for the research, authorship, and/or publication of this article. This work was funded by the Central Universities of China (No. 31920240088).
We are grateful to Beijing Biomarker Technologies Corporation (Beijing, China) for assisting in sequencing.
The authors declare that the research was conducted in the absence of any commercial or financial relationships that could be construed as a potential conflict of interest.
The authors declare that no Gen AI was used in the creation of this manuscript.
All claims expressed in this article are solely those of the authors and do not necessarily represent those of their affiliated organizations, or those of the publisher, the editors and the reviewers. Any product that may be evaluated in this article, or claim that may be made by its manufacturer, is not guaranteed or endorsed by the publisher.
The Supplementary material for this article can be found online at: https://www.frontiersin.org/articles/10.3389/fvets.2025.1542637/full#supplementary-material
1. Weng, K, Li, Y, Huo, W, Zhang, Y, Cao, Z, Zhang, Y, et al. Comparative phosphoproteomic provides insights into meat quality differences between slow- and fast-growing broilers. Food Chem. (2022) 373:131408. doi: 10.1016/j.foodchem.2021.131408
2. Lan, R, Wang, Y, Wei, L, Wu, F, and Yin, F. Heat stress exposure changed liver lipid metabolism and abdominal fat deposition in broilers. Ital J Anim Sci. (2022) 21:1326–33. doi: 10.1080/1828051X.2022.2103461
3. Adhikari, R, White, D, House, JD, and Kim, WK. Effects of additional dosage of vitamin D3, vitamin D2, and 25-Hydroxyvitamin D3 on calcium and phosphorus utilization, egg quality and bone mineralization in laying hens. Poult Sci. (2020) 99:364–73. doi: 10.3382/ps/pez502
5. Deb, S, Reeves, AA, and Lafortune, S. Simulation of physicochemical and pharmacokinetic properties of vitamin D3 and its natural derivatives. Pharmaceuticals. (2020) 13:160. doi: 10.3390/ph13080160
6. Han, J, Lv, X, He, L, Liu, M, Qu, H, Xi, L, et al. Mapk signaling pathway participates in the regulation of intestinal phosphorus and calcium absorption in broiler chickens via 1,25-Dihydroxyvitamin D3. Poult Sci. (2024) 103:104052. doi: 10.1016/j.psj.2024.104052
7. Chen, C, Turner, B, Applegate, TJ, Litta, G, and Kim, WK. Role of Long-term supplementation of 25-Hydroxyvitamin D3 on laying hen bone 3-dimensional structural development. Poult Sci. (2020) 99:5771–82. doi: 10.1016/j.psj.2020.06.080
8. Szymczak-Pajor, I, Miazek, K, Selmi, A, Balcerczyk, A, and Śliwińska, A. The action of vitamin D in adipose tissue: is there the link between vitamin D deficiency and adipose tissue-related metabolic disorders? Int J Mol Sci. (2022) 23:956. doi: 10.3390/ijms23020956
9. Shojadoost, B, Yitbarek, A, Alizadeh, M, Kulkarni, RR, Astill, J, Boodhoo, N, et al. Centennial review: effects of vitamins a, D, E, and C on the chicken immune system. Poult Sci. (2021) 100:100930. doi: 10.1016/j.psj.2020.12.027
10. Nimitphong, H, Guo, W, Holick, MF, Fried, SK, and Lee, MJ. Vitamin D inhibits Adipokine production and inflammatory signaling through the vitamin D receptor in human adipocytes. Obesity. (2021) 29:562–8. doi: 10.1002/oby.23109
11. Stroia, CM, Ghitea, TC, Vrânceanu, M, Mureșan, M, Bimbo-Szuhai, E, Pallag, CR, et al. Relationship between vitamin D3 deficiency, metabolic syndrome and Vdr, Gc, and Cyp2r1 gene polymorphisms. Nutrients. (2024) 16:1272. doi: 10.3390/nu16091272
12. Mazzone, G, Morisco, C, Lembo, V, D'Argenio, G, D'Armiento, M, Rossi, A, et al. Dietary supplementation of vitamin D prevents the development of Western diet-induced metabolic, hepatic and cardiovascular abnormalities in rats. United European Gastroenterol J. (2018) 6:1056–64. doi: 10.1177/2050640618774140
13. Manna, P, Achari, AE, and Jain, SK. Vitamin D supplementation inhibits oxidative stress and upregulate Sirt1/Ampk/Glut4 Cascade in high glucose-treated 3t3l1 adipocytes and in adipose tissue of high fat diet-fed diabetic mice. Arch Biochem Biophys. (2017) 615:22–34. doi: 10.1016/j.abb.2017.01.002
14. Lim, H, Lee, H, and Lim, Y. Effect of vitamin D3 supplementation on hepatic lipid dysregulation associated with autophagy regulatory Ampk/Akt-Mtor signaling in type 2 diabetic mice. Exp Biol Med (Maywood). (2021) 246:1139–47. doi: 10.1177/1535370220987524
15. du, T, Xiang, L, Zhang, J, Yang, C, Zhao, W, Li, J, et al. Vitamin D improves hepatic steatosis in Nafld via regulation of fatty acid uptake and Β-oxidation. Front Endocrinol. (2023) 14:1138078. doi: 10.3389/fendo.2023.1138078
16. Chang, E, and Kim, Y. Vitamin D decreases adipocyte lipid storage and increases Nad-Sirt1 pathway in 3t3-L1 adipocytes. Nutrition. (2016) 32:702–8. doi: 10.1016/j.nut.2015.12.032
17. Chang, E, and Kim, Y. Vitamin D insufficiency exacerbates adipose tissue macrophage infiltration and decreases Ampk/Sirt1 activity in obese rats. Nutrients. (2017) 9:338. doi: 10.3390/nu9040338
18. Zhang, XL, Chen, L, Yang, J, Zhao, SS, Jin, S, Ao, N, et al. Vitamin D alleviates non-alcoholic fatty liver disease via restoring gut microbiota and metabolism. Front Microbiol. (2023) 14:1117644. doi: 10.3389/fmicb.2023.1117644
19. Xiang, L, Du, T, Zhang, J, Zhang, Y, Zhou, Y, Zhao, Y, et al. Vitamin D3 supplementation shapes the composition of gut microbiota and improves some obesity parameters induced by high-fat diet in mice. Eur J Nutr. (2024) 63:155–72. doi: 10.1007/s00394-023-03246-1
20. Zhang, X, Shang, X, Jin, S, Ma, Z, Wang, H, AO, N, et al. Vitamin D ameliorates high-fat-diet-induced hepatic injury via inhibiting Pyroptosis and alters gut microbiota in rats. Arch Biochem Biophys. (2021) 705:108894:108894. doi: 10.1016/j.abb.2021.108894
21. Belenchia, AM, Johnson, SA, Ellersieck, MR, Rosenfeld, CS, and Peterson, CA. In utero vitamin D deficiency predisposes offspring to Long-term adverse adipose tissue effects. J Endocrinol. (2017) 234:301–13. doi: 10.1530/joe-17-0015
22. Chen, Z, Zhu, Y, Wu, T, Qian, X, Hu, Y, and Hu, W. The effect of maternal vitamin D deficiency during pregnancy on glycolipid metabolism of offspring rats and the improvement of vitamin D intervention after weaning. Front Nutr. (2023) 10:1214040. doi: 10.3389/fnut.2023.1214040
23. Nong, K, Liu, Y, Fang, X, Qin, X, Liu, Z, and Zhang, H. Effects of the vitamin D3 on alleviating the oxidative stress induced by Diquat in Wenchang chickens. Animals. (2023) 13:711. doi: 10.3390/ani13040711
24. Wei, J, Li, L, Peng, Y, Luo, J, Chen, T, Xi, Q, et al. The effects of optimal dietary vitamin D3 on growth and carcass performance, tibia traits, meat quality, and intestinal morphology of Chinese yellow-feathered broiler chickens. Animals. (2024) 14:920. doi: 10.3390/ani14060920
25. Garcia, AF, Murakami, AE, Duarte, CR, Rojas, IC, Picoli, KP, and Puzotti, MM. Use of vitamin D3 and its metabolites in broiler chicken feed on performance, bone parameters and meat quality. Asian Australas J Anim Sci. (2013) 26:408–15. doi: 10.5713/ajas.2012.12455
26. Li, X, Li, J, Yuan, H, Chen, Y, Li, S, Jiang, S, et al. Effect of supplementation with Glycyrrhiza Uralensis extract and Lactobacillus Acidophilus on growth performance and intestinal health in broiler chickens. Front Vet Sci. (2024) 11:1436807. doi: 10.3389/fvets.2024.1436807
27. Wagner, BD, Grunwald, GK, Zerbe, GO, Mikulich-Gilbertson, SK, Robertson, CE, Zemanick, ET, et al. On the use of diversity measures in longitudinal sequencing studies of microbial communities. Front Microbiol. (2018) 9:1037. doi: 10.3389/fmicb.2018.01037
28. Sies, H, Fitzpatrick, PF, Newman, A, and Forman, HJ. Archives of biochemistry and biophysics: 80th anniversary. Arch Biochem Biophys. (2022) 726:109295. doi: 10.1016/j.abb.2022.109295
29. Abbasi, T, Shakeri, M, Zaghari, M, and Kohram, H. Growth performance parameters, bone calcification and immune response of in Ovo injection of 25-hydroxycholecalciferol and vitamin K3 in male Ross 308 broilers. Theriogenology. (2017) 90:260–5. doi: 10.1016/j.theriogenology.2016.12.016
30. Valle, YL, Almalki, SG, and Agrawal, DK. Vitamin D machinery and metabolism in porcine adipose-derived mesenchymal stem cells. Stem Cell Res Ther. (2016) 7:118. doi: 10.1186/s13287-016-0382-4
31. Shinya, S, Yohannes, YB, Ikenaka, Y, Nakayama, SM, Ishizuka, M, and Fujita, S. Characteristics of cytochrome P450-dependent metabolism in the liver of the wild raccoon, Procyon Lotor. J Vet Med Sci. (2022) 84:1665–72. doi: 10.1292/jvms.22-0182
32. Morrissey, RL, Cohn, RM, Empson, RN Jr, Greene, HL, Taunton, OD, and Ziporin, ZZ. Relative toxicity and metabolic effects of cholecalciferol and 25-hydroxycholecalciferol in chicks. J Nutr. (1977) 107:1027–34. doi: 10.1093/jn/107.6.1027
33. Mattila, P, Rokka, T, Könkö, K, Valaja, J, Rossow, L, and Ryhänen, EL. Effect of cholecalciferol-enriched hen feed on egg quality. J Agric Food Chem. (2003) 51:283–7. doi: 10.1021/jf020743z
34. Kim, YJ, Kim, KY, Kim, MS, Lee, JH, Lee, KP, and Park, T. A mixture of the aqueous extract of Garcinia Cambogia, soy peptide and L: -carnitine reduces the accumulation of visceral fat mass in rats rendered obese by a high fat diet. Genes Nutr. (2008) 2:353–8. doi: 10.1007/s12263-007-0070-1
35. Leveille, GA, Romsos, DR, Yeh, Y, and O'Hea, EK. Lipid biosynthesis in the Chick. A consideration of site of synthesis, influence of diet and possible regulatory mechanisms. Poult Sci. (1975) 54:1075–93. doi: 10.3382/ps.0541075
36. Yu, W, Gao, Y, Zhao, Z, Long, X, Yi, Y, and Ai, S. Fumigaclavine C ameliorates liver steatosis by attenuating hepatic De novo lipogenesis via modulation of the Rhoa/rock signaling pathway. BMC Complement Med Ther. (2023) 23:288. doi: 10.1186/s12906-023-04110-9
37. Lambert, JE, Ramos-Roman, MA, Browning, JD, and Parks, EJ. Increased De novo lipogenesis is a distinct characteristic of individuals with nonalcoholic fatty liver disease. Gastroenterology. (2014) 146:726–35. doi: 10.1053/j.gastro.2013.11.049
38. Quinn, WJ 3rd, and Birnbaum, MJ. Distinct Mtorc1 pathways for transcription and cleavage of Srebp-1c. Proc Natl Acad Sci U S A. (2012) 109:15974–5. doi: 10.1073/pnas.1214113109
39. Liu, H, Chen, Y, Wen, Y, Zhu, S, Huang, S, He, L, et al. Phloridzin ameliorates lipid deposition in high-fat-diet-fed mice with nonalcoholic fatty liver disease via inhibiting the Mtorc1/Srebp-1c pathway. J Agric Food Chem. (2021) 69:8671–83. doi: 10.1021/acs.jafc.1c01645
40. Zhang, Y, Meng, T, Zuo, L, Bei, Y, Zhang, Q, Su, Z, et al. Xyloketal B attenuates fatty acid-induced lipid accumulation via the Srebp-1c pathway in Nafld models. Mar Drugs. (2017) 15:163. doi: 10.3390/md15060163
41. Araki, M, Nakagawa, Y, Oishi, A, Han, SI, Wang, Y, Kumagai, K, et al. The peroxisome proliferator-activated receptor Α (Pparα) agonist Pemafibrate protects against diet-induced obesity in mice. Int J Mol Sci. (2018) 19:2148. doi: 10.3390/ijms19072148
42. Smith, BK, and Steinberg, GR. Amp-activated protein kinase, fatty acid metabolism, and insulin sensitivity. Curr Opin Clin Nutr Metab Care. (2017) 20:248–53. doi: 10.1097/mco.0000000000000380
43. Foretz, M, Even, PC, and Viollet, B. Ampk activation reduces hepatic lipid content by increasing fat oxidation in vivo. Int J Mol Sci. (2018) 19:2826. doi: 10.3390/ijms19092826
44. Wang, TY, and Wang, XH. [Effects of aerobic exercise on Pparα signaling in diabetes rats and its association with Pparγ]. Zhongguo Ying Yong Sheng Li Xue Za Zh. (2020) 36:312–7. doi: 10.12047/j.cjap.5945.2020.067
45. Ke, X, Zhang, R, Li, P, Zuo, L, Wang, M, Yang, J, et al. Hydrochloride Berberine ameliorates alcohol-induced liver injury by regulating inflammation and lipid metabolism. Biochem Biophys Res Commun. (2022) 610:49–55. doi: 10.1016/j.bbrc.2022.04.009
46. Kim, B, Woo, MJ, Park, CS, Lee, SH, Kim, JS, Kim, B, et al. Hovenia Dulcis extract reduces lipid accumulation in oleic acid-induced steatosis of Hep G2 cells via activation of Ampk and Pparα/Cpt-1 pathway and in acute hyperlipidemia mouse model. Phytother Res. (2017) 31:132–9. doi: 10.1002/ptr.5741
47. Hu, Y, Feng, Y, Ding, Z, Lv, L, Sui, Y, Sun, Q, et al. Maternal betaine supplementation decreases hepatic cholesterol deposition in chicken offspring with epigenetic modulation of Srebp2 and Cyp7a1 genes. Poult Sci. (2020) 99:3111–20. doi: 10.1016/j.psj.2019.12.058
48. Norton, L, Shannon, C, Gastaldelli, A, and DeFronzo, RA. Insulin: the master regulator of glucose metabolism. Metab Clin Exp. (2022) 129:155142. doi: 10.1016/j.metabol.2022.155142
49. Lee, K, Shin, J, Latshaw, JD, Suh, Y, and Serr, J. Cloning of adipose triglyceride lipase complementary deoxyribonucleic acid in poultry and expression of adipose triglyceride lipase during development of adipose in chickens. Poult Sci. (2009) 88:620–30. doi: 10.3382/ps.2008-00265
50. Shiping, B, Wanqiu, L, Huan, L, Keying, Z, Jianping, W, Xuemei, D, et al. Effects of high dietary Iron on the lipid metabolism in the liver and adipose tissue of male broiler chickens. Anim Feed Sci Technol. (2021) 282:115131. doi: 10.1016/J.ANIFEEDSCI.2021.115131
51. Saltiel, AR. Insulin signaling in the control of glucose and lipid homeostasis. Handb Exp Pharmacol. (2016) 233:51–71. doi: 10.1007/164_2015_14
52. Halliday, D, Venkatesan, S, and Pacy, P. Apolipoprotein metabolism: a stable-isotope approach. Am J Clin Nutr. (1993) 57:726S–31S; discussion 30S-31S. doi: 10.1093/ajcn/57.5.726S
53. Chen, Z, Wang, S, Pottekat, A, Duffey, A, Jang, I, Chang, BH, et al. Conditional hepatocyte ablation of Pdia1 uncovers indispensable roles in both Apob and Mttp folding to support Vldl secretion. Mol Metabolism. (2024) 80:101874. doi: 10.1016/j.molmet.2024.101874
54. Liu, Y, Yang, J, Liu, X, Liu, R, Wang, Y, Huang, X, et al. Dietary folic acid addition reduces abdominal fat deposition mediated by alterations in gut microbiota and Scfa production in broilers. Anim Nutr. (2023) 12:54–62. doi: 10.1016/j.aninu.2022.08.013
55. Hermier, D. Lipoprotein metabolism and fattening in poultry. J Nutr. (1997) 127:805s–8s. doi: 10.1093/jn/127.5.805S
56. Cui, H, Liu, L, Liu, X, Wang, Y, Luo, N, Tan, X, et al. A selected population study reveals the biochemical mechanism of intramuscular fat deposition in chicken meat. J Anim Sci Biotechnol. (2022) 13:54. doi: 10.1186/s40104-022-00705-3
57. Alvarenga, RR, Zangeronimo, MG, Pereira, LJ, Rodrigues, PB, and Gomide, EM. Lipoprotein metabolism in poultry. Worlds Poult Sci J. (2011) 67:431–40. doi: 10.1017/S0043933911000481
58. Huang, J, Zhu, R, and Shi, D. The role of Fatp1 in lipid accumulation: a review. Mol Cell Biochem. (2021) 476:1897–903. doi: 10.1007/s11010-021-04057-w
59. Tous, N, Theil, PK, Lauridsen, C, Lizardo, R, Vilà, B, and Esteve-Garcia, E. Dietary conjugated linoleic acid modify gene expression in liver, muscles, and fat tissues of finishing pigs. J Anim Sci. (2012) 90:340–2. doi: 10.2527/jas.53768
60. Vitali, M, Dimauro, C, Sirri, R, Zappaterra, M, Zambonelli, P, Manca, E, et al. Effect of dietary polyunsaturated fatty acid and antioxidant supplementation on the transcriptional level of genes involved in lipid and energy metabolism in swine. PLoS One. (2018) 13:e0204869. doi: 10.1371/journal.pone.0204869
61. Bennour, I, Haroun, N, Sicard, F, Mounien, L, and Landrier, JF. Recent insights into vitamin D, adipocyte, and adipose tissue biology. Obes Rev. (2022) 23:e13453. doi: 10.1111/obr.13453
62. Wen, C, Yan, W, Sun, C, Ji, C, Zhou, Q, Zhang, D, et al. The gut microbiota is largely independent of host genetics in regulating fat deposition in chickens. ISME J. (2019) 13:1422–36. doi: 10.1038/s41396-019-0367-2
63. Hu, H, Li, A, Shi, C, Chen, L, Zhao, Z, Yin, X, et al. Mulberry branch fiber improved lipid metabolism and egg yolk fatty acid composition of laying hens via the enterohepatic axis. Microbiome. (2024) 12:73. doi: 10.1186/s40168-024-01788-y
64. Chen, Q, Zhao, L, Mei, L, Zhao, X, Han, P, Liu, J, et al. Vitamin C and vitamin D3 alleviate metabolic-associated fatty liver disease by regulating the gut microbiota and bile acid metabolism via the gut-liver Axis. Front Pharmacol. (2023) 14:1163694. doi: 10.3389/fphar.2023.1163694
65. Han, GG, Lee, JY, Jin, GD, Park, J, Choi, YH, Chae, BJ, et al. Evaluating the association between body weight and the intestinal microbiota of weaned piglets via 16s Rrna sequencing. Appl Microbiol Biotechnol. (2017) 101:5903–11. doi: 10.1007/s00253-017-8304-7
66. Tilg, H, and Kaser, A. Gut microbiome, obesity, and metabolic dysfunction. J Clin Invest. (2011) 121:2126–32. doi: 10.1172/jci58109
67. Chen, YH, Chiu, CC, Hung, SW, Huang, WC, Lee, YP, Liu, JY, et al. Gnotobiotic mice inoculated with firmicutes, but not bacteroidetes, deteriorate nonalcoholic fatty liver disease severity by modulating hepatic lipid metabolism. Nutr Res. (2019) 69:20–9. doi: 10.1016/j.nutres.2019.07.001
68. Moreira Júnior, RE, de Carvalho, LM, Dos Reis, DC, Cassali, GD, Faria, AMC, Maioli, TU, et al. Diet-induced obesity leads to alterations in behavior and gut microbiota composition in mice. J Nutr Biochem. (2021) 92:108622. doi: 10.1016/j.jnutbio.2021.108622
69. Johnson, EL, Heaver, SL, Walters, WA, and Ley, RE. Microbiome and metabolic disease: revisiting the bacterial phylum bacteroidetes. J Mol Med. (2017) 95:1–8. doi: 10.1007/s00109-016-1492-2
70. Tseng, CH, and Wu, CY. The gut microbiome in obesity. J Formos Med Assoc. (2019) 118:S3–9. doi: 10.1016/j.jfma.2018.07.009
71. Comstock, LE. Importance of Glycans to the host-Bacteroides mutualism in the mammalian intestine. Cell Host Microbe. (2009) 5:522–6. doi: 10.1016/j.chom.2009.05.010
72. Lee, J, Wellenstein, K, and Kahn, BB. 1405-P: Bacteroides Thetaiotaomicron fortifies the gut mucosa and improves host metabolism in dietary obese mice. Diabetes. (2022) 71:1405-P. doi: 10.2337/DB22-1405-P
73. Gauffin Cano, P, Santacruz, A, Moya, Á, and Sanz, Y. Bacteroides uniformis Cect 7771 ameliorates metabolic and immunological dysfunction in mice with high-fat-diet induced obesity. PLoS One. (2012) 7:e41079. doi: 10.1371/journal.pone.0041079
74. Li, J, Li, Y, Xiao, H, Li, W, Ye, F, Wang, L, et al. The intestinal microflora diversity of aboriginal chickens in Jiangxi Province, China. Poult Sci. (2024) 103:103198. doi: 10.1016/j.psj.2023.103198
75. Song, H, Shen, X, Chu, Q, and Zheng, X. Vaccinium bracteatum Thunb. Fruit extract reduces high-fat diet-induced obesity with modulation of the gut microbiota in obese mice. J Food Biochem. (2021) 45:e13808. doi: 10.1111/jfbc.13808
76. Lan, Y, Sun, Q, Ma, Z, Peng, J, Zhang, M, Wang, C, et al. Seabuckthorn polysaccharide ameliorates high-fat diet-induced obesity by gut microbiota-Scfas-liver axis. Food Funct. (2022) 13:2925–37. doi: 10.1039/d1fo03147c
77. Tu, W, Nie, W, Yao, X, Zhang, J, Zhang, H, di, D, et al. Growth performance, lipid metabolism, and systemic immunity of weaned piglets were altered by buckwheat protein through the modulation of gut microbiota. Mol Genet Genomics. (2024) 299:15. doi: 10.1007/s00438-024-02103-y
78. De Filippo, C, Cavalieri, D, Di Paola, M, Ramazzotti, M, Poullet, JB, Massart, S, et al. Impact of diet in shaping gut microbiota revealed by a comparative study in children from Europe and rural Africa. Proc Natl Acad Sci U S A. (2010) 107:14691–6. doi: 10.1073/pnas.1005963107
79. Song, X, Zhong, L, Lyu, N, Liu, F, Li, B, Hao, Y, et al. Inulin can alleviate metabolism disorders in Ob/Ob mice by partially restoring leptin-related pathways mediated by gut microbiota. Genomics Proteomics Bioinformatics. (2019) 17:64–75. doi: 10.1016/j.gpb.2019.03.001
80. Faden, H. The role of Faecalibacterium, Roseburia, and butyrate in inflammatory bowel disease. Digestive Dis. (2022) 40:793–5. doi: 10.1159/000522247
81. Hu, J, Lin, S, Zheng, B, and Cheung, PCK. Short-chain fatty acids in control of energy metabolism. Crit Rev Food Sci Nutr. (2018) 58:1243–9. doi: 10.1080/10408398.2016.1245650
82. May, KS, and den Hartigh, LJ. Gut microbial-derived short chain fatty acids: impact on adipose tissue physiology. Nutrients. (2023) 15:272. doi: 10.3390/nu15020272
83. Kondo, T, Kishi, M, Fushimi, T, and Kaga, T. Acetic acid upregulates the expression of genes for fatty acid oxidation enzymes in liver to suppress body fat accumulation. J Agric Food Chem. (2009) 57:5982–6. doi: 10.1021/jf900470c
84. Deng, J, Zou, X, Liang, Y, Zhong, J, Zhou, K, Zhang, J, et al. Hypoglycemic effects of different molecular weight Konjac Glucomannans via intestinal microbiota and Scfas mediated mechanism. Int J Biol Macromol. (2023) 234:122941. doi: 10.1016/j.ijbiomac.2022.12.160
85. Nogal, A, Louca, P, Zhang, X, Wells, PM, Steves, CJ, Spector, TD, et al. Circulating levels of the short-chain fatty acid acetate mediate the effect of the gut microbiome on visceral fat. Front Microbiol. (2021) 12:711359. doi: 10.3389/fmicb.2021.711359
Keywords: broiler chicken, vitamin D3, growth performance, lipid metabolism, cecal microbiota
Citation: Li J, Li X, Tian J, Xu L, Chen Y, Jiang S, Zhang G and Lu J (2025) Effects of supplementation with vitamin D3 on growth performance, lipid metabolism and cecal microbiota in broiler chickens. Front. Vet. Sci. 12:1542637. doi: 10.3389/fvets.2025.1542637
Received: 10 December 2024; Accepted: 24 January 2025;
Published: 06 February 2025.
Edited by:
Mingyao Yang, Washington State University, United StatesCopyright © 2025 Li, Li, Tian, Xu, Chen, Jiang, Zhang and Lu. This is an open-access article distributed under the terms of the Creative Commons Attribution License (CC BY). The use, distribution or reproduction in other forums is permitted, provided the original author(s) and the copyright owner(s) are credited and that the original publication in this journal is cited, in accordance with accepted academic practice. No use, distribution or reproduction is permitted which does not comply with these terms.
*Correspondence: Guohua Zhang, MjgwMTEyMTAzQHhibXUuZWR1LmNu; Jianxiong Lu, MTY1ODYwNjE2QHhibXUuZWR1LmNu
Disclaimer: All claims expressed in this article are solely those of the authors and do not necessarily represent those of their affiliated organizations, or those of the publisher, the editors and the reviewers. Any product that may be evaluated in this article or claim that may be made by its manufacturer is not guaranteed or endorsed by the publisher.
Research integrity at Frontiers
Learn more about the work of our research integrity team to safeguard the quality of each article we publish.