- Department of Molecular and Cellular Biology, University of Guelph, Guelph, ON, Canada
European Foulbrood (EFB) is a severe bacterial disease affecting honeybees, primarily caused by the Gram-positive bacterium Melissococcus plutonius. Although the presence of M. plutonius is associated with EFB, it does not consistently predict the manifestation of symptoms, and the role of ‘secondary invaders’ in the disease’s development remains a subject of ongoing debate. This review provides an updated synthesis of the microbial ecological factors that influence the expression of EFB symptoms, which have often been overlooked in previous research. In addition, this review examines the potential negative health consequences of prolonged antibiotic use in bee colonies for treating EFB, and proposes innovative and sustainable alternatives. These include the development of probiotics and targeted microbiota management techniques, aiming to enhance the overall resilience of bee populations to this debilitating disease.
1 Introduction
The pollination services of honeybees contributes to revenue of over USD $18 billion in crop production annually in the United States and more than 80% of all crops depend, to varying extents, on insect pollination (1, 2). Overall global honeybee numbers have grown since 1961, yet in that same time period, the area of insect pollinated crops has grown by more than 300%, highlighting the increased demand for such pollinators (2, 3). Over the past few decades, beekeepers from many nations around the world (including, for example, EU, Mexico, Canada, New Zealand and Iran) have experienced an alarming and continually increasing rate of honeybee mortality (4–6). In 2022–2023, total Canadian honeybee colony loss was estimated at 48%, over three times the approximate sustainable limit (5). Major colony losses have been attributed to a combinatorial effect of pesticide exposure, natural habitat loss, nutritional deficiencies, pathogen infection, and more (7). Due to the importance of honeybees to the environment and the agricultural system, researchers and industrialists seek strategies to reduce and treat the stressors challenging honeybees.
One stressor, European Foulbrood (EFB), is a major honeybee disease which affects larvae of managed Western and Asian honeybees (8). Despite the serious consequences of infection (larval mortality and subsequent colony population decline) this disease is poorly understood.
EFB is caused by the pathogenic bacterium, Melissococcus plutonius, where larvae become infected through oral ingestion via dietary contamination by asymptomatic worker bees. The pathogen then colonizes the larval gut, outcompeting the host for nutritional substrates, ultimately leading to host starvation. New evidence suggests M. plutonius crosses the host gut epithelium layer to cause septic infection (9). Infected larvae undergo a distinctive transformation, transitioning in color from a white to a yellow hue and manifesting a deflated appearance before succumbing to the infection. A colony affected by EFB experiences sporadic and irregular brood development, leading to diminished honey output, reduced pollination efficiency, and, in severe cases, complete colony collapse. For a beekeeper, the direct monetary impact of a single infected colony exhibiting mild disease is estimated to be ~ USD $215 in treatment and equipment replacement costs (10).
Due to its potential economic impact, and the ease of spread of the disease between hives, EFB is categorized as a reportable disease in many countries, as listed in the World Organization for Animal Health (WOAH). In terms of treatment for infected hives, beekeepers have rather limited options. Oxytetracycline, a broad-spectrum antibiotic that interferes with bacterial protein synthesis, has been used for years in many countries as both a treatment for, and a prophylactic against, infection. However, this antibiotic is associated with high rates of EFB recurrence (27%) and honeybee larval toxicity (11–13). In addition, despite strict adherence to current treatment guidelines by beekeepers, honeybee colonies continue to be commonly infected by M. plutonius, demonstrating an acute need for research to elucidate strategies for effective EFB treatment and eradication.
2 History, cultivation challenges, and pathogen diversity
Although M. plutonius was identified as the causative agent of EFB over 100 years ago, researchers have faced significant challenges in studying this pathogenic bacterium because of its fastidious growth requirements. Most M. plutonius strains require strict anaerobic conditions for growth as well as a culture medium containing specific nutrients (14). Although recent advances have been made with regard to improved isolation methods and media types, many strains of M. plutonius are still difficult to cultivate and tend to grow very poorly under laboratory conditions, thereby hindering research.
Further complicating matters is the fact that M. plutonius can often be detected in asymptomatic hives, suggesting that it is a common pathobiont of honeybees, i.e., its presence may not necessarily cause apparent signs of disease. It is been long speculated that the pathobiont behavior of M. plutonius is a result of distinct genetic determinants impacting virulence (15). Consistent with this, Arai et al. performed comparative characterization of 33 M. plutonius field isolates and identified two clear phenotypic groupings, denoted as ‘typical’ or ‘atypical’ based on their biochemical and culture characteristics relative to the features of the type strain, M. plutonius ATCC 35311 (Table 1). Using in vitro in larvae infection assays, it was found that atypical isolates caused higher mortality rates compared to typical isolates; specifically, atypical isolates killed between 70–90% of infected larvae within 5 days, whereas typical isolates killed less than 20% even when given the same infectious dose (16). From the limited number of studies that have been done, it can be surmised that typical strains have a wide variance in their infectious capabilities, whereas atypical isolates consistently cause high mortality (17).
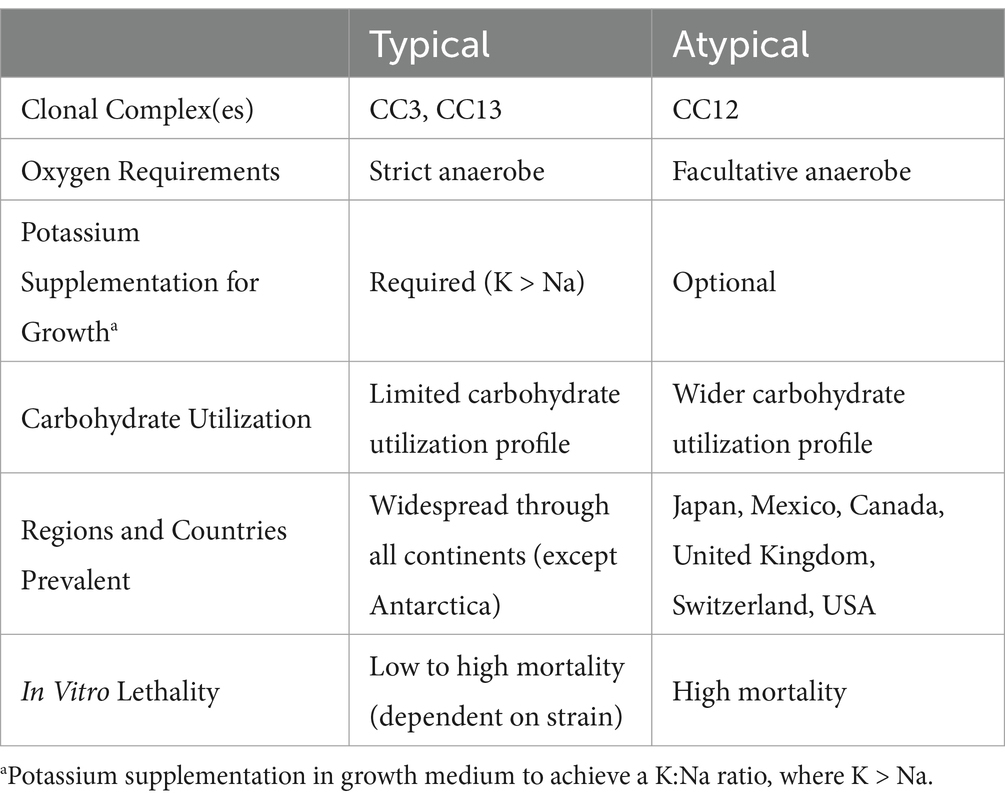
Table 1. Phenotypic differences between typical and atypical Melissococcus plutonius as described in Arai et al. (2012) and Takamatsu (2023).
Genomic investigations have shown that M. plutonius strains can be grouped into three ‘clonal’ complexes of M. plutonius (CC3, CC12, and CC13; Table 1). CC12 strains are of particular interest due to their virulence and prevalence in certain regions, and all display ‘atypical’ characteristics (18). CC3 and CC13 strains, seen as more predominant, display the ‘typical’ phenotypic characteristics, such as required low sodium and high potassium growth conditions. A summation of this can be found in Table 1.
3 Geographic distribution of EFB and detection of antibiotic resistance
Some of the oldest and most well-documented reports of EFB come from Canada in the early 20th century. In 1916, the “Apiary Inspection in Ontario” indicated that out of 5,367 colonies inspected, a total of 1,387 colonies (~25%) displayed symptoms of EFB (19). Following the introduction of oxytetracycline in the 1940s, EFB either disappeared or dropped to undetectable levels in many regions, and when disease outbreaks did occur, they were generally considered self-limiting with clinical symptoms often found to resolve spontaneously (20, 21).
In the past several years, there has been a re-emergence of severe EFB outbreaks in several regions of the world including the US, Canada, and Switzerland (22–24). The extent of this re-emergence, however, is likely underestimated, since EFB symptoms can be ambiguous and the disease itself is reportable in many countries, as per its assignment in WOAH. In Canada, the increase in symptomatic EFB infections was first observed in 2019 in colonies used for commercial blueberry pollination (23, 25). The phenomenon was initially thought to be related to immune deficits of bees feeding on nutrient-poor blueberry pollen. However, colonies not necessarily exposed to blueberry crops have also been affected, for example, a three-year molecular survey conducted in Alberta recently detected EFB in over 30% of the province’s colonies (10).
Insight regarding the prevalence of typical and atypical M. plutonius within hives can be gleaned from two studies using genetic surveillance. Arai et al. conducted random sampling of honeybee colonies across Japan and found that of infected colonies, 40% contained only typical M. plutonius strains, 6% contained only atypical M. plutonius strains and 54% were co-infected with both typical and atypical strains (26). De León-Door et al. confirmed similar findings (27). The higher proportion of co-infected hives suggests that atypical M. plutonius strains may gain an advantage when co-infecting with typical strains. Diverse, thorough sampling in regionally distinct areas is required to confirm this phenomenon. Additionally, further research is needed to elucidate why typical/atypical strain co-infections may be beneficial for disease progression.
Compared to other areas of the world (e.g., the United States Japan, Switzerland, and United Kingdom) (17, 28) there has been limited genetic investigation of the EFB strains present in Canada or their proportionality. One exception is the recent study by Thebeau et al., which assessed pathogenicity and determined clonal complex genotypes of 4 clinical M. plutonius isolates derived from EFB outbreaks in Saskatchewan, Alberta, and British Columbia (29). Notably, 75% of the M. plutonius isolates found were identified as CC12 strains that were highly pathogenic (associated with ~58–70% mortality of larvae) based on in vitro larval infection assays (note that the convention for the use of ‘in vitro larval’ in this field tends to refer to studies of larvae within a lab environment).
One Canadian CC12 isolate, 2019 BC1, has displayed a minimum inhibitory concentration (MIC) of 16 μg/mL to oxytetracycline (30). This MIC is higher than the concentration used for standard EFB treatment, and therefore could constitute this strain as oxytetracycline resistant. In a later study by Takamatsu et al., 77 M. plutonius isolates from Japan were examined, revealing an additional four strains [comprising three CC3 and one CC12 strain(s)] resistant to oxytetracycline, based on MICs of 16 μg/mL (31). The emergence of oxytetracycline resistance in M. plutonius appears independent of clonal complex type, and dates to at least 2008 when the Japanese isolates were first isolated. Elucidation of the mechanism of resistance to oxytetracycline in M. plutonius is warranted to obtain a comprehensive understanding of its prevalence and risk and to explore potential avenues for intervention. Genome level analysis of M. plutonius isolates from EFB-infected hives sampled globally are needed in order to better understand the relationship between genetic diversity and virulence in this pathogen.
4 Virulence determinants of Melissococcus plutonius
4.1 Molecular determinants
As described above, M. plutonius isolates can be grouped into three clonal complexes with distinct phenotypic and genomic characteristics. Nonetheless, data collected from over 500 isolates across 18 countries show a lack of consensus with regard to pathogenicity of M. plutonius based solely on clonal complex genotype (32). These inconsistencies may be related to the acquisition by some strains of a virulence plasmid (pMP19) which encodes a putative ETX/MTX2 toxin family protein with insecticidal properties, provisionally named ‘melissotoxin A’. For example, pMP19 positive CC3 strains produce mortality rates of >91% during larval infection assays (32). Demonstrating a causal link, Nakamura et al. showed that loss of the pMP19 plasmid abrogated pathogenicity and that larvae infected with pMP19-cured CC3 strains experienced only a 6% mortality rate (33). In the same study, pMP19 failed to explain the virulence of CC12, with both pMP19-positive and -negative strains resulting in up to 90–100% larval mortality rates (33). Given this extreme virulence combined with a lack of understanding of the underlying mechanisms, ‘atypical’ CC12 M. plutonius strains are considered the most dangerous strains which pose the greatest risk for severe EFB outbreaks.
Other genetic determinants have been proposed to play a role in virulence, such as the presence of the gene encoding tyrosine decarboxylase, a catalytic enzyme responsible for the conversion of tyrosine to tyramine and carbon dioxide (34). A buildup of tyramine has been linked to development of classic EFB symptoms in larvae (35). The tyrosine decarboxylase gene is present and functional in strains belonging to CC12 and CC3 only, and presents a compelling potential virulence determinant of these clonal complexes compared to CC13 (16, 36).
4.2 Interplay between ‘secondary invaders’ and EFB disease onset
EFB disease severity may be influenced by larval co-infection with so-called ‘secondary invaders’, i.e., other microbial species that can work in tandem with M. plutonius to cause symptoms. Several studies have focused on this phenomenon and the major findings can be found in Figure 1.
Originally, Bacillus alvei (since reclassified as Paenibacillus alvei) was indicated as the causal organism responsible for producing EFB, since this bacterial species was found abundantly in larvae with EFB symptoms (37). This finding was called into question by White, who proposed the causal organism of EFB as M. plutonius (38). More than four decades later, Bailey failed to reproduce EFB symptoms in larvae when using only pure cultures of M. plutonius (39), but later was the first to suggest that M. plutonius may not act alone to cause disease; M. plutonius was identified as the primary etiological agent of EFB in addition to a supplementary role of several other bacterial species, including Enterococcus faecalis, ‘Achromobacter eurydice’, or Paenibacillus alvei (40). The source of these accomplice strains is not always clear, but several reports have suggested that Paenibacillus and Enterococcus spp. can be vectored by ectoparasites such as Varroa mites and small hive beetles (41–44). However, the roles and impact of E. faecalis, A. eurydice and P. alvei on EFB disease progression have been contentious, with contradictory evidence of their involvement.
Not discussed in detail in this review, confusion surrounding the actual identity of the proposed secondary invader first described as ‘A. eurydice’ has been called into question, thus rendering past research regarding its role in EFB progression contentious. This controversy is discussed in depth in Erler et al. (45).
Testing the secondary invader hypothesis in vitro in larvae, Giersch et al. reported that the specific combination of M. plutonius and P. alvei field isolates derived from the same EFB infected colony could reliably produce symptoms characteristic of EFB (46). Furthermore, this combination increased mortality in lab-reared larvae when compared to monoculture infection with only M. plutonius (46). Conversely, Lewkowski and Erler found no effect of experimental co-infection when using strains of M. plutonius 49.3 and P. alvei LMG 13253 in larvae in vitro (47). Anderson et al. confirmed that both P. alvei and E. faecalis were present in EFB diseased colonies, but did not necessarily find that the presence of one or both species correlated with disease progression (41). In vitro in larvae it has been shown that E. faecalis co-infection with M. plutonius did not result in more severe infection compared to each species alone, however this may have been because the strains used for this work were not isolated from a diseased colony (47). Analysis of the role of ‘A. eurydice’ in EFB, has not been further investigated, to the best of our knowledge.
Although there has not been much work done to understand synergistic infections with multiple microbes in cases of EFB disease, the work that has been done offers some compelling evidence that in addition to M. plutonius, other microbes are involved. Whether microbial adaptation is required of M. plutonius in order to infect with a given secondary invader, or whether existing strain-level differences influence the likelihood of disease is undetermined.
The combined effects of primary and secondary pathogens, along with unknowns about clonal complex (CC3/CC12/CC13), create a complex web of interactions driving the probability of EFB disease incidence and severity that is yet to be fully deciphered. Unraveling this complexity will require comprehensive research but is necessary in order to devise effective EFB management strategies and ensure honeybee health and sustainability.
5 Use of honeybee symbionts in the development of alternative EFB treatments
5.1 Natural defense mechanisms of the honeybee gut microbiota
The adult honeybee gut microbiota is highly conserved and plays a pivotal role in nutrient absorption, detoxification, and immune system modulation (48). Although it also provides host benefits, the gut microbiota of honeybee larvae is more variable than that of adults, showing lower abundance of microbial species, and often the presence of environmental microbes (41). In terms of the role of the honeybee microbiota in EFB interactions, only a limited number of studies have been completed to date.
One study assessed the microbiomes of two types of adult worker bees derived from apiaries with clinical signs of EFB infection: a symptomatic type and an asymptomatic type (49). Unsurprisingly, there were large differences in the abundance of M. plutonius among the bees, with symptomatic bees harboring a 75-fold higher load of M. plutonius compared to asymptomatic bees (49). However, significant differences in the abundance of other microbial taxa among the two types were also identified (49). Symptomatic bees not only possessed a higher incidence of M. plutonius, but also higher proportions of Fructobacillus fructosus, Apilactobacillus kunkeei, Gilliamella apicola, Frischella perrara, and Bifidobacterium indicum (49). Species of Snodgrassella alvi, Lactobacillus melliventris, L. helsingborgensis and L. kullabergensis, the latter 3 of which are lactic acid-producing bacteria (LAB), were found in higher abundance in worker bees from asymptomatic colonies compared to their counterparts and it is therefore possible that their presence plays a role in EFB disease protection or suppression, especially early on in development (49). It is notable that in a further study where microbiomes of larva were screened, LAB were also negatively associated with M. plutonius (50). In addition, the lack of a single acetic acid-producing bacterium (Bombella apis) was found to be uniquely associated with atypical EFB symptomatology (50).
Due to the correlative nature of typical microbiome research, results indicating the positive or negative correlation of a certain species with M. plutonius load is a cause-and-effect dilemma. One member being in higher abundance when M. plutonius levels are higher could indicate that this species is spurring growth of M. plutonius, or, the growth of that member could be a response to independent M. plutonius growth. As such, mechanistic microbiome research must be carried out to determine the nature of this correlation, through methods such as infection assays with individual gain or loss of specific microbiome members or crosswise microbiota transplants.
Overall, these results raise questions about whether symbiotic bacteria may play a central role in the promotion of colony health in the presence of M. plutonius, mitigating the risk of EFB development. While our understanding of bee microbiota ecology and interactions in EFB is still nascent, these findings suggest that certain beneficial bacteria may impede the colonization of M. plutonius and act as a natural barrier against infection. As such, the potential for harnessing certain beneficial microbes as prophylactics for EFB disease prevention is of interest.
5.2 Probiotics as a promising therapeutic alternative
The development of beneficial microbes as probiotics for use in hive management is a relatively new and expanding strategy for apiarists. Several groups have successfully identified honeybee-derived strains of LAB representative of Lactobacillus, Apilactobacillus, and Pediococcus spp. which were able to strongly inhibit M. plutonius growth in vitro (51–53). The mechanism(s) of this inhibition remains unexplored, however some LAB produce metabolites, such as organic acids, bacteriocins (a class of small antimicrobial peptides), or bacteriocin-like inhibitory substances (BLIS) that are known to inhibit pathogen growth. For example, it has been found that the cell-free supernatant of an isolate of Apilactobacillus kunkeei (formerly Lactobacillus kunkeei), thought to be one of the most abundant LAB species in honeybees, has growth inhibitory effects against M. plutonius (54). This finding was later confirmed by Zendo et al., who also demonstrated that the mechanism of M. plutonius growth inhibition was through the production of a bacteriocin, kunkecin A, by A. kunkeei (52). Additionally, a recent study by Leska et al. revealed that 55 out of 103 tested LAB strains showed antagonistic activity against M. plutonius, with one strain of Ligilactobacillus salivarius demonstrating the most notable growth inhibition among them (51). As well, the cell-free supernatant of L. salivarius, as well as strains of Pediococcus parvulus and Levilactobacillus brevis also notably inhibited M. plutonius growth (51). Mojgani et al. also found that the cell-free supernatants from LAB species Lactobacillus acidophilus, Lacticaseibacillus rhamnosus, Lactiplantibacillus plantarum, Lactobacillus apis and Pediococcus acidilactici potently inhibited M. plutonius growth, with the production of organic acids, bacteriocins or BLIS being proposed as critical mediators of this activity (53).
These results support microbiota associations and suggest that certain LAB may be prime probiotic candidates. However, each of these in vitro studies used a single M. plutonius strain as their test organism: the type strain ATCC 35311. Since (as described above) atypical M. plutonius strains have divergent virulence factors and culture requirements, the inhibitory activity seen with the type strain may not be generalizable to all EFB etiologic agents.
That said, two studies have screened honeybee-derived bacterial strains for antagonistic activity toward atypical M. plutonius strains. Wu et al. found that one Lactobacillus sp. strain (Acaj3) exhibited strong inhibitory activity against atypical M. plutonius (strain DAT 561) in vitro (55). In direct comparison with antibiotics, the same authors found that Acja3 was comparable to tetracycline in its antibacterial activity against M. plutonius and could reduce in vitro larval mortality by ~50% during infection assays. A notable advantage of some beneficial bacteria is their bactericidal properties (i.e., killing capacity) in comparison to tetracycline, which exerts bacteriostatic effects (i.e., growth inhibitory without killing) (56). Various Bifidobacterium spp. have also been investigated for their antagonism against two atypical M. plutonius strains (DAT 561 and DAT 351), and some were found to exhibit antagonistic effects against its growth (57).
To date, in total, only three M. plutonius strains (ATCC 35311, DAT 561, and DAT 351) have been tested for their direct inhibition by potential probiotic strains in vitro. As it is becoming clear that different M. plutonius strains can possess a multitude of different virulence factors and culture requirements, it is also becoming clear that there is a gap in knowledge about the likely applicability of these probiotic strains to protect against EFB disease in general. Furthermore, while work in the field shows intriguing results, future studies will be imperative to understand the effect of these candidate strains in vivo, especially to account for the impact of other gut microbial members and host-factors on probiotic efficacy.
In support of this, in the field, a recent study in Italy (979 colonies/22 apiaries) showed that Lactiplantibacillus plantarum LMG P-21806 treatment reduced EFB prevalence from 4.5 to 2.5% over a 6 month period in vivo (58). Another in vivo study in California (33 colonies/2 apiaries) indicated a three-strain lactobacilli consortium (LX3; composed of Lactiplantibacillus plantarum Lp39, Lactobacillus rhamnosus GR-1 and Apilactobacillus kunkeei BR-1) could reduce M. plutonius burden by five-fold over a 20 week period (59). This work is an especially promising start to understanding the role of probiotic treatment in vivo; however, future studies are required to better characterize these interactions and outcomes on EFB. Furthermore, since these studies utilized natural EFB infections, no genotype or M. plutonius strain information was provided, which is an area in need of further characterization.
While the potential effectiveness of probiotic strains/treatments have been described for mitigating M. plutonius growth and honeybee disease, it should be noted that not all probiotics show equally promising results. In recent years, commercial bee probiotics (e.g., SuperDFM®-HoneyBee™, the most popular honeybee probiotic in the United States) have been found unable to colonize or restore/antibiotic rescue the honeybee gut microbiome (60–62).
A strong hypothesis regarding this discrepancy is due to the composition of probiotics, as commercial products are typically comprised of non-native microbiota that have a slim chance of establishment and subsequent survival in the honeybee gut (60–62). The efficacy of probiotics composed of a mixture of native bee microbiota, however, have been shown to colonize the honeybee gut with success and are a promising area of future work, which could potentially be applied in the treatment of EFB (62, 63). That said, it is important to consider that further in vivo work is imperative before drawing conclusions regarding the therapeutic use of probiotic strains for disease management in honeybees.
Additionally, the influence of geographic variation and environmental factors is crucial to the interpretation of research outcomes, and there is not enough data to evaluate this at present. This limitation leaves substantial gaps in our understanding of how probiotics interact with M. plutonius strains, both typical and atypical. Understanding these dynamics, and others, is essential for developing effective treatment strategies that can be adapted to diverse environmental conditions and regional characteristics. A summary of candidate species and tested strains discussed in this section are found in Table 2.
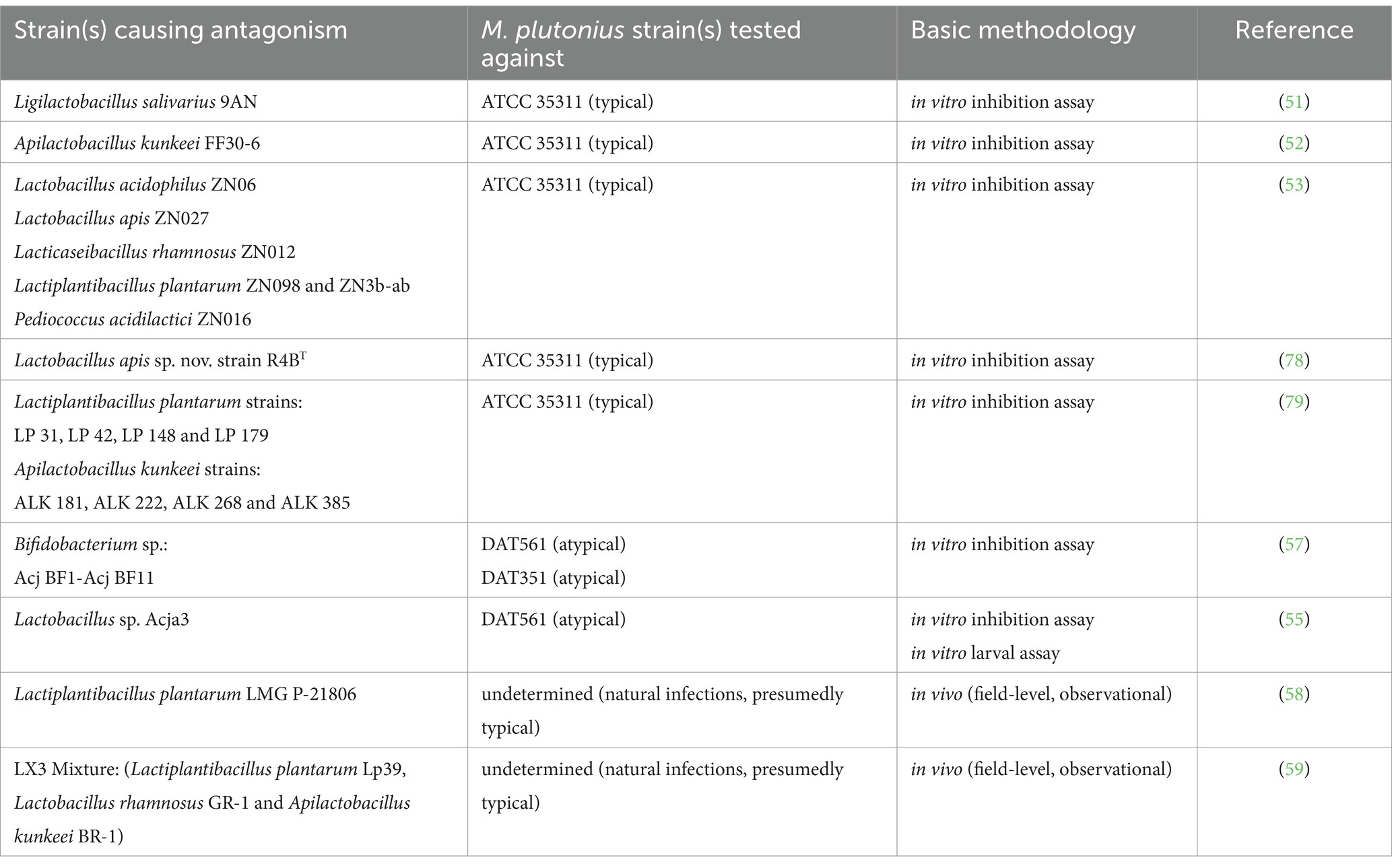
Table 2. Candidate probiotics demonstrating strain-level in vitro or in vivo activity against select strains of Melissococcus plutonius.
5.3 Other therapeutic alternatives to counteract EFB disease
There are several approaches currently used to try to control the incidence of EFB disease. Hygienic breeding techniques can generate bees that efficiently detect and remove dead and infected larvae, which is expected to reduce EFB spread. Palacio et al. found that colonies selectively bred for hygienic behavior experience diminished rates of EFB, yet Fowler et al. has since suggested that there was no effect of hygienic bee behavior on the development of EFB (64, 65). These conflicting studies may be a result of the rate at which a given M. plutonius strain is able to kill larvae. For example, CC12 strains tend to kill larvae before capping, whereas some CC3 M. plutonius can cause mortality at a slower rate such that larvae are capped before death (66); all honeybees will dispose of dead larvae in uncapped cells, suggesting that hygienic behavior would not be an advantage in infections caused by CC12 M. plutonius (67).
Some honeybee lines also naturally resist EFB, hinting at a potential for advancements in bee breeding, however the host genetic determinants of resistance are currently unknown (47, 68). Additionally, natural substances such as essential oils show antimicrobial properties against several microbes and parasites that cause various bee diseases, but these substances require careful use to avoid toxicity in the bees themselves, and to prevent the development of microbial resistance (69). In this respect, tea tree oil application has been shown to inhibit M. plutonius in vitro and was determined to be non-toxic to honeybee larvae at effective levels (70).
A proposed factor in the development and progression of EFB is malnutrition. As previously stated, a suggested mechanism of disease involves host-pathogen competition for nutrients. During infection, malnutrition may also result from the immune system’s energy demands, which divert resources away from nutrient uptake and metabolism (71). Pollen, a critical component of royal jelly and a key dietary addition during later larval development, may significantly influence disease outcomes. Outside the context of infection, larvae fed different pollen sources during the pre-pupal stage exhibit varying survival rates (72). Additionally, pollen type has been shown to affect pre-pupal weight, while pollen dose impacts development speed (72). These findings underscore the importance of pollen type for healthy larval development, even under normal conditions.
This evidence supports the notion that supplementing honeybee colonies with pollen or pollen substitutes could improve overall health and potentially reduce disease susceptibility, especially in colonies with insufficient pollen sources or storage. Researchers have demonstrated that pollen supplementation reduces Nosema spp. infestations in adult bees; however, it also shortens the bees’ average lifespan (73). While this approach shows promise as a preventative measure, further research and refinement are needed to optimize its benefits. To our knowledge, no studies have directly examined the effects of pollen supplementation on M. plutonius infections.
Lastly, a treatment known as the ‘shook swarm’ method, involves transfer of EFB-infected adult bees to a new brood box supplemented with a sugar-oxytetracycline solution. This method results in decreased EFB symptoms and significantly reduces the reoccurrence rate compared to hygienic breeding, or the use of natural substances (11), although it runs the risk of contributing toward the development of antimicrobial resistance in bacteria associated with treated hives.
6 Areas requiring further research and future directions
6.1 Strain diversity and its role in disease progression
The escalating EFB crisis jeopardizes the honeybee population and, subsequently, their pollination services that contribute to agricultural efficiency. Partly attributed to the presence of atypical M. plutonius strains, EFB infections are becoming steadily more problematic and rising in occurrence. Although many atypical strains have been isolated from Japan, their presence has recently become apparent in other regions, including North America and Europe. Already determined to be phenotypically different in terms of growth requirements, the extent to which atypical strains differ in disease progression from their typical counterparts is still unclear. Continued genomic investigations of distinct strains M. plutonius strains, their associated virulence factors, and requirements for virulence expression need to be completed alongside confirmatory in vitro assays to clarify these discrepancies.
6.2 Defining the secondary invaders and their impact
Conflicting in vitro reports of the impact of secondary invaders could be explained by several different experimental factors which have been proposed to affect larval survival in other settings, such as: different larval rearing protocols (74), differing honeybee lineages with disparate innate resistance (68), or different experimental inoculation methods (75). To limit the impact of differing experimental design, future studies should look to incorporating a broader array of M. plutonius strains and using multiple larval rearing and larval inoculation protocols, akin to treatment groups. This would aid in determining whether virulence is indeed enhanced or remains unchanged in the presence of a variety of proposed secondary invaders, and not merely an effect of experimental design.
A further reason for the conflicting results so far seen between studies may be strain-specific co-adaptation within a given hive environment. For example, when M. plutonius and purported secondary invader strains were isolated from the same infected colony, synergism in EFB infection outcomes was seen; this was not repeated in a separate study using strains isolated from different sources (46, 47, 76). To determine this argument’s validity, a co-infection study should be conducted using M. plutonius-secondary invader pairings from unrelated and single isolation source(s). Furthermore, whether M. plutonius genotype also plays a role in interactions with secondary invaders remains in question. To the extent of our knowledge, no research to date has comprehensively compared the interactions of different M. plutonius clonal complexes with secondary invader partners. Studying the role of genotype in these interactions may also explain discrepancies seen in work to date, and is an area requiring future research.
These contradictory findings could also be a result of the misidentification of the secondary invader itself. Giersch et al. indicated P. alvei identity via Gram stain and morphological comparison of vegetative cells and endospores, yet several Paenibacillus spp. are highly similar in these characteristics. For example, the recently-described species Paenibacillus melissococcoides, also isolated from a honeybee colony experiencing EFB, is closely related to P. alvei as determined through 16S rRNA gene sequence comparison, and also forms endospores (77). Therefore, it is possible that the secondary invader which produced EFB symptoms and increased virulence when co-infected with M. plutonius in Giersch et al.’s study may be representative of another Paenibacillus spp. such as P. melissococcoides. Detailed molecular investigation regarding the true identity of strains used in these studies, would help to resolve species and strain co-occurrence in EFB-infected larva.
6.3 Probiotic efficacy to emerging strains
Discoveries regarding the determinants of EFB disease can be used to inform future alternative treatments. Current treatment options for EFB, such as oxytetracycline application, pose several unacceptable limitations, particularly in an era of increasing antimicrobial resistance, and with a dawning understanding of the importance of the honeybee microbiome in health. In contrast, probiotic approaches offer exciting new avenues, with many potential benefits. However, only limited work on the effectiveness of probiotics against EFB disease has been undertaken, and there remains a critical gap in understanding probiotic efficacy against divergent M. plutonius strains. Going forward, probiotic efficacy studies in the treatment and prevention of EFB should encompass multiple M. plutonius strains, including representatives of typical and atypical strains to demonstrate applicability in the field. Moreover, when considering prophylactic probiotic use in honeybees, developing researchers should aim to understand how these probiotics affect beneficial gut flora and as well as potential secondary invaders.
Author contributions
EM: Conceptualization, Formal analysis, Investigation, Visualization, Writing – original draft. GF: Conceptualization, Formal analysis, Investigation, Writing – original draft. BD: Conceptualization, Supervision, Writing – review & editing. EA-V: Funding acquisition, Project administration, Supervision, Writing – review & editing.
Funding
The author(s) declare that financial support was received for the research, authorship, and/or publication of this article. This work was supported by a Canadian Institutes of Health Research (CIHR) Banting Postdoctoral Fellowship Award (PDF-402947–2023, award to BAD) and Canada First Research Excellence Fund (CFREF) Food from Thought (FfT) Thematic III Research Grant (THE3-00525) to EA-V. EM and GF were supported by scholarships from Ontario Graduate Scholarships, respectively.
Conflict of interest
EA-V is co-founder of NuBioyota, a company developing novel microbiome-based therapeutics for human use.
The remaining authors declare that the research was conducted in the absence of any commercial or financial relationships that could be construed as a potential conflict of interest.
Publisher’s note
All claims expressed in this article are solely those of the authors and do not necessarily represent those of their affiliated organizations, or those of the publisher, the editors and the reviewers. Any product that may be evaluated in this article, or claim that may be made by its manufacturer, is not guaranteed or endorsed by the publisher.
References
1. Khalifa, SAM, Elshafiey, EH, Shetaia, AA, El-Wahed, AAA, Algethami, AF, Musharraf, SG, et al. Overview of bee pollination and its economic value for crop production. Insects. (2021) 12:688. doi: 10.3390/insects12080688
2. Aizen, MA, and Harder, LD. The global stock of domesticated honey bees is growing slower than agricultural demand for pollination. Curr Biol. (2009) 19:915–8. doi: 10.1016/j.cub.2009.03.071
3. Aizen, MA, Garibaldi, LA, Cunningham, SA, and Klein, AM. Long-term global trends in crop yield and production reveal no current pollination shortage but increasing pollinator dependency. Curr Biol. (2008) 18:1572–5. doi: 10.1016/j.cub.2008.08.066
4. Gray, A, Adjlane, N, Arab, A, Ballis, A, Brusbardis, V, Bugeja Douglas, A, et al. Honey bee colony loss rates in 37 countries using the COLOSS survey for winter 2019–2020: the combined effects of operation size, migration and queen replacement. J Apic Res. (2023) 62:204–10. doi: 10.1080/00218839.2022.2113329
5. Ferland, Julie, Wilson, Geoff, and Nasr, Medhat. Canadian Association of Professional Apiculturists Statement on Honey Bee Wintering Losses in Canada (2022). Available from: https://capabees.com/shared/CAPA-Statement-on-Colony-Losses-2021-2022-FV.pdf (Accessed August 7, 2024).
6. Hristov, P, Shumkova, R, Palova, N, and Neov, B. Factors associated with honey bee Colony losses: a Mini-review. Vet Sci. (2020) 7:166. doi: 10.3390/vetsci7040166
7. Daisley, BA, Pitek, AP, Mallory, E, Chernyshova, AM, Allen-Vercoe, E, Reid, G, et al. Disentangling the microbial ecological factors impacting honey bee susceptibility to Paenibacillus larvae infection. Trends Microbiol. (2023) 31:521–34. doi: 10.1016/j.tim.2022.11.012
8. Takamatsu, D, Morinishi, K, Arai, R, Sakamoto, A, Okura, M, and Osaki, M. Typing of Melissococcus plutonius isolated from European and Japanese honeybees suggests spread of sequence types across borders and between different Apis species. Vet Microbiol. (2014) 171:221–6. doi: 10.1016/j.vetmic.2014.03.036
9. Takamatsu, D, Sato, M, and Yoshiyama, M. Infection of Melissococcus plutonius clonal complex 12 strain in European honeybee larvae is essentially confined to the digestive tract. J Vet Med Sci. (2016) 78:29–34. doi: 10.1292/jvms.15-0405
10. Laate, E. Potential economic impact of European and American foulbrood on Alberta’s beekeeping industry. Canada: agriculture and forestry, government of Alberta; (2020). Available from: https://open.alberta.ca/dataset/029a345b-8621-4986-ad78-7fc6ddcd8b17/resource/25f7b78d-a359-428c-9648-9175c3634720/download/af-potential-economic-impact-european-american-foulbrood-on-albertas-beekeeping-industry.pdf (Accessed August 7, 2024).
11. Budge, GE, Barrett, B, Jones, B, Pietravalle, S, Marris, G, Chantawannakul, P, et al. The occurrence of Melissococcus plutonius in healthy colonies of Apis mellifera and the efficacy of European foulbrood control measures. J Invertebr Pathol. (2010) 105:164–70. doi: 10.1016/j.jip.2010.06.004
12. Thompson, HM, Waite, RJ, Wilkins, S, Brown, MA, Bigwood, T, Shaw, M, et al. Effects of shook swarm and supplementary feeding on oxytetracycline levels in honey extracted from treated colonies. Apidologie. (2006) 37:51–7. doi: 10.1051/apido:2005058
13. Pettis, JS, Kochansky, J, and Feldlaufer, MF. Larval Apis mellifera L. (Hymenoptera: Apidae) mortality after topical application of antibiotics and dusts. J Econ Entomol. (2004) 97:171–6. doi: 10.1603/0022-0493-97.2.171
14. Djordjevic, S, Noone, K, Smith, L, and Hornitzky, MAZ. Development of a hemi-nested PCR assay for the specific detection ofMelissococcus pluton. J Apic Res. (1998) 37:165–74. doi: 10.1080/00218839.1998.11100968
15. McKee, BA, David Goodman, R, and Alan, HM. The transmission of European foulbrood (Melissococcus plutonius) to artificially reared honey bee larvae (Apis mellifera). J Apic Res. (2004) 43:93–100. doi: 10.1080/00218839.2004.11101117
16. Arai, R, Tominaga, K, Wu, M, Okura, M, Ito, K, Okamura, N, et al. Diversity of Melissococcus plutonius from honeybee larvae in Japan and experimental reproduction of European foulbrood with cultured atypical isolates. PLoS One. (2012) 7:e33708. doi: 10.1371/journal.pone.0033708
17. Takamatsu, D. Atypical Melissococcus plutonius strains: their characteristics, virulence, epidemiology, and mysteries. J Vet Med Sci. (2023) 85:880–94. doi: 10.1292/jvms.23-0180
18. Haynes, E, Helgason, T, Young, JPW, Thwaites, R, and Budge, GE. A typing scheme for the honeybee pathogen Melissococcus plutonius allows detection of disease transmission events and a study of the distribution of variants. Environ Microbiol Rep. (2013) 5:525–9. doi: 10.1111/1758-2229.12057
19. Pettit, M. Outline of apiary inspection in Ontario. J Econ Entomol. (1916) 9:196–9. doi: 10.1093/jee/9.1.196
20. Bailey, L, and Locher, N. Experiments on the etiology of European foul brood of the honeybee. J Apic Res. (1968) 7:103–7. doi: 10.1080/00218839.1968.11100197
21. Chopra, I, and Roberts, M. Tetracycline antibiotics: mode of action, applications, molecular biology, and epidemiology of bacterial resistance. Microbiol Mol Biol Rev. (2001) 65:260. doi: 10.1128/mmbr.232.2.232-260.2001
22. Alburaki, M, Abban, SK, Evans, JD, and Chen, YP. Occurrence and distribution of two bacterial brood diseases (American and European foulbrood) in US honey bee colonies and resistance to antibiotics from 2015 to 2022. J Apic Res. (2024) 63:701–10. doi: 10.1080/00218839.2024.2329854
23. Olmstead, S, McCallum, R, and Shaw, J. Evaluating the effect of feeding pollen substitute to honey bee colonies destined for wild blueberry pollination in Colchester County, Nova Scotia. Nova Scotia, Canada: Atlantic Tech Transfer Team for Apiculture; (2019). p. 1–4. Available from: https://www.perennia.ca/wp-content/uploads/2019/10/ATTTA-FactSheet-Oct-2019.pdf (Accessed August 1, 2024).
24. Roetschi, A, Berthoud, H, Kuhn, R, and Imdorf, A. Infection rate based on quantitative real-time PCR Ofmelissococcus plutonius, the causal agent of European foulbrood, in honeybee colonies before and after apiary sanitation. Apidologie. (2008) 39:362–71. doi: 10.1051/apido:200819
25. Guarna, M, Higo, H, Foster, L, Pernal, S, and Wolf, VP. Bee health and blueberry pollination. Hive Lights. (2019) 32:14.
26. Arai, R, Miyoshi-Akiyama, T, Okumura, K, Morinaga, Y, Wu, M, Sugimura, Y, et al. Development of duplex PCR assay for detection and differentiation of typical and atypical Melissococcus plutonius strains. J Vet Med Sci. (2014) 76:491–8. doi: 10.1292/jvms.13-0386
27. de León-Door, AP, Romo-Chacón, A, Rios-Velasco, C, Zamudio-Flores, PB, Ornelas-Paz, JJ, and Acosta-Muñiz, CH. Prevalence, typing and phylogenetic analysis of Melissococcus plutonius strains from bee colonies of the state of Chihuahua, Mexico. J Invertebr Pathol. (2018) 159:71–7. doi: 10.1016/j.jip.2018.10.006
28. Grossar, D, Haynes, E, Budge, GE, Parejo, M, Gauthier, L, Charrière, JD, et al. Population genetic diversity and dynamics of the honey bee brood pathogen Melissococcus plutonius in a region with high prevalence. J Invertebr Pathol. (2023) 196:107867. doi: 10.1016/j.jip.2022.107867
29. Thebeau, JM, Liebe, D, Masood, F, Kozii, IV, Klein, CD, Zabrodski, MW, et al. Investigation of Melissococcus plutonius isolates from 3 outbreaks of European foulbrood disease in commercial beekeeping operations in western Canada. Can Vet J. (2022) 63:935–42.
30. Masood, F, Thebeau, JM, Cloet, A, Kozii, IV, Zabrodski, MW, Biganski, S, et al. Evaluating approved and alternative treatments against an oxytetracycline-resistant bacterium responsible for European foulbrood disease in honey bees. Sci Rep. (2022) 12:5906. doi: 10.1038/s41598-022-09796-4
31. Takamatsu, D, Yoshida, E, Watando, E, and Ueno, Y. Oxytetracycline resistance Ofmelissococcus plutoniusstrains in Japan. J Apic Res. (2024) 63:306–9. doi: 10.1080/00218839.2023.2213401
32. Grossar, D, Kilchenmann, V, Forsgren, E, Charrière, JD, Gauthier, L, Chapuisat, M, et al. Putative determinants of virulence in Melissococcus plutonius, the bacterial agent causing European foulbrood in honey bees. Virulence. (2020) 11:554–67. doi: 10.1080/21505594.2020.1768338
33. Nakamura, K, Okumura, K, Harada, M, Okamoto, M, Okura, M, and Takamatsu, D. Different impacts ofpMP19on the virulence Ofmelissococcus plutoniusstrains with different genetic backgrounds. Environ Microbiol. (2020) 22:2756–70. doi: 10.1111/1462-2920.14999
34. Connil, N, Le Breton, Y, Dousset, X, Auffray, Y, Rincé, A, and Prévost, H. Identification of the Enterococcus faecalis tyrosine decarboxylase operon involved in tyramine production. Appl Environ Microbiol. (2002) 68:3537–44. doi: 10.1128/AEM.68.7.3537-3544.2002
35. Kanbar, G, Engels, W, Nicholson, GJ, Hertle, R, and Winkelmann, G. Tyramine functions as a toxin in honey bee larvae during Varroa-transmitted infection by Melissococcus pluton. FEMS Microbiol Lett. (2004) 234:149–54. doi: 10.1111/j.1574-6968.2004.tb09526.x
36. Djukic, M, Erler, S, Leimbach, A, Grossar, D, Charrière, JD, Gauthier, L, et al. Comparative genomics and description of putative virulence factors of Melissococcus plutonius, the causative agent of European foulbrood disease in honey bees. Gen Dent. (2018) 9:419. doi: 10.3390/genes9080419
37. Cheshire, FR, and Watson Cheyne, W. The Pathogenic History and History under Cultivation of a new Bacillus (B. alvei), the Cause of a Disease of the Hive Bee hitherto known as Foul Brood - Cheshire - 1885 - Journal of the Royal Microscopical Society - Wiley Online Library. (2024). Available from: https://onlinelibrary.wiley.com/doi/abs/10.1111/j.1365-2818.1885.tb05794.x (Accessed July 30, 2024).
38. White, GF. The cause of European foul brood. U.S. government printing. Office. (1912) 157:24. doi: 10.5962/bhl.title.63494
39. Bailey, L. The isolation and cultural characteristics of Streptococcus pluton and further observations on bacterium eurydice. Microbiology. (1957) 17:39–48.
40. Bailey, L. The pathogenicity for honey-bee larvae of microorganisms associated with European foulbrood. J insect pathol. (1963) 5:198–205.
41. Anderson, KE, Copeland, DC, Erickson, RJ, Floyd, AS, Maes, PC, and Mott, BM. A high-throughput sequencing survey characterizing European foulbrood disease and varroosis in honey bees. Sci Rep. (2023) 13:1162. doi: 10.1038/s41598-023-28085-2
42. Pakwan, C, Kaltenpoth, M, Weiss, B, Chantawannakul, P, Jun, G, and Disayathanoowat, T. Bacterial communities associated with the ectoparasitic mites Varroa destructor and Tropilaelaps mercedesae of the honey bee (Apis mellifera). FEMS Microbiol Ecol. (2018) 94:fix160. doi: 10.1093/femsec/fix160
43. Hubert, J, Erban, T, Kamler, M, Kopecky, J, Nesvorna, M, Hejdankova, S, et al. Bacteria detected in the honeybee parasitic mite Varroa destructor collected from beehive winter debris. J Appl Microbiol. (2015) 119:640–54. doi: 10.1111/jam.12899
44. Huang, Q, Lopez, D, and Evans, JD. Shared and unique microbes between small hive beetles (Aethina tumida) and their honey bee hosts. MicrobiologyOpen. (2019) 8:e899. doi: 10.1002/mbo3.899
45. Erler, S, Lewkowski, O, Poehlein, A, and Forsgren, E. The curious case of Achromobacter eurydice, a gram-variable pleomorphic bacterium associated with European foulbrood disease in honeybees. Microb Ecol. (2018) 75:1–6. doi: 10.1007/s00248-017-1007-x
46. Giersch, T, Barchia, I, and Hornitzky, M. Can fatty acids and oxytetracycline protect artificially raised larvae from developing European foulbrood? Apidologie. (2010) 41:151–9. doi: 10.1051/apido/2009066
47. Lewkowski, O, and Erler, S. Virulence of Melissococcus plutonius and secondary invaders associated with European foulbrood disease of the honey bee. Microbiology. (2019) 8:e00649. doi: 10.1002/mbo3.649
48. Daisley, BA, Chmiel, JA, Pitek, AP, Thompson, GJ, and Reid, G. Missing microbes in bees: how systematic depletion of key symbionts erodes immunity. Trends Microbiol. (2020) 28:1010–21. doi: 10.1016/j.tim.2020.06.006
49. Erban, T, Ledvinka, O, Kamler, M, Hortova, B, Nesvorna, M, Tyl, J, et al. Bacterial community associated with worker honeybees (Apis mellifera) affected by European foulbrood. PeerJ. (2017) 5:e3816. doi: 10.7717/peerj.3816
50. Anderson, K, Floyd, A, Maes, P, Mott, B, and Copeland, D. Microbiomes associated with European foul brood disease, and idiopathic brood disease syndrome in honey bees. (2022). Available from: https://www.researchsquare.com/article/rs-1436205/v1 (Accessed June 13, 2024).
51. Leska, A, Nowak, A, Szulc, J, Motyl, I, and Czarnecka-Chrebelska, KH. Antagonistic activity of potentially probiotic lactic acid Bacteria against honeybee (Apis mellifera L.) pathogens. Pathogens. (2022) 11:1367. doi: 10.3390/pathogens11111367
52. Zendo, T, Ohashi, C, Maeno, S, Piao, X, Salminen, S, Sonomoto, K, et al. Kunkecin a, a new Nisin variant Bacteriocin produced by the Fructophilic lactic acid bacterium, Apilactobacillus kunkeei FF30-6 isolated from honey bees. Front Microbiol. (2020) 11:11. doi: 10.3389/fmicb.2020.571903
53. Mojgani, N, Bagheri, M, Moharrami, M, Toutiaee, S, and Rousta, HM. Bioactive metabolites from autochthonous lactic acid bacteria inhibit the growth Ofmelissococcus plutonius, causal agent of European foulbrood disease in honey bees. Entomol Exp Appl. (2023) 171:386–94. doi: 10.1111/eea.13295
54. Endo, A, and Salminen, S. Honeybees and beehives are rich sources for fructophilic lactic acid bacteria. Syst Appl Microbiol. (2013) 36:444–8. doi: 10.1016/j.syapm.2013.06.002
55. Wu, M, Sugimura, Y, Iwata, K, Takaya, N, Takamatsu, D, Kobayashi, M, et al. Inhibitory effect of gut bacteria from the Japanese honey bee, Apis cerana japonica, against Melissococcus plutonius, the causal agent of European foulbrood disease. J Insect Sci. (2014) 14:129. doi: 10.1093/jis/14.1.129
56. Chukwudi, CU. rRNA binding sites and the molecular mechanism of action of the Tetracyclines. Antimicrob Agents Chemother. (2016) 60:4433–41. doi: 10.1128/AAC.00594-16
57. Wu, M, Sugimura, Y, Takaya, N, Takamatsu, D, Kobayashi, M, Taylor, D, et al. Characterization of bifidobacteria in the digestive tract of the Japanese honeybee, Apis cerana japonica. J Invertebr Pathol. (2013) 112:88–93. doi: 10.1016/j.jip.2012.09.005
58. Pietropaoli, M, Carpana, E, Milito, M, Palazzetti, M, Guarducci, M, Croppi, S, et al. Use of Lactobacillus plantarum in preventing clinical cases of American and European foulbrood in Central Italy. Appl Sci. (2022) 12:1388. doi: 10.3390/app12031388
59. Daisley, BA, Pitek, AP, Chmiel, JA, Al, KF, Chernyshova, AM, Faragalla, KM, et al. Novel probiotic approach to counter Paenibacillus larvae infection in honey bees. ISME J. (2020) 14:476–91. doi: 10.1038/s41396-019-0541-6
60. Damico, ME, Beasley, B, Greenstein, D, and Raymann, K. Testing the effectiveness of a commercially sold probiotic on restoring the gut microbiota of honey bees: a field study. Probiotics & Antimicro Prot. (2023). doi: 10.1007/s12602-023-10203-1
61. Anderson, KE, Allen, NO, Copeland, DC, Kortenkamp, OL, Erickson, R, Mott, BM, et al. A longitudinal field study of commercial honey bees shows that non-native probiotics do not rescue antibiotic treatment, and are generally not beneficial. Sci Rep. (2024) 14:1954. doi: 10.1038/s41598-024-52118-z
62. Motta, EVS, Powell, JE, Leonard, SP, and Moran, NA. Prospects for probiotics in social bees. Philosophical Trans Royal Society B: Biolog Sci. (2022) 377:20210156. doi: 10.1098/rstb.2021.0156
63. Powell, JE, Carver, Z, Leonard, SP, and Moran, NA. Field-realistic Tylosin exposure impacts honey bee microbiota and pathogen susceptibility, which is ameliorated by native gut probiotics. Microbiol Spectr. (2021) 9:e0010321. doi: 10.1128/Spectrum.00103-21
64. Palacio, MA, Figini, EE, Ruffinengo, SR, Rodriguez, EM, Del, HML, and Bedascarrasbure, EL. Changes in a population Ofapis melliferal. Selected for hygienic behaviour and its relation to brood disease tolerance. Apidologie. (2000) 31:471–8. doi: 10.1051/apido:2000139
65. Fowler, PD, Schroeder, DC, Kevill, JL, and Milbrath, MOG. No impact of hygienic behavior and viral coinfection on the development of European foulbrood in honey bee (Apis mellifera) colonies during blueberry pollination in Michigan. J Insect Sci. (2023) 23:21. doi: 10.1093/jisesa/iead094
66. Nakamura, K, Yamazaki, Y, Shiraishi, A, Kobayashi, S, Harada, M, Yoshiyama, M, et al. Virulence differences among Melissococcus plutonius strains with different genetic backgrounds in Apis mellifera larvae under an improved experimental condition. Sci Rep. (2016) 6:33329. doi: 10.1038/srep33329
67. Al Toufailia, H, Evison, SEF, Hughes, WOH, and Ratnieks, FLW. Both hygienic and non-hygienic honeybee, Apis mellifera, colonies remove dead and diseased larvae from open brood cells. Philos Trans R Soc Lond Ser B Biol Sci. (2018) 373:20170201. doi: 10.1098/rstb.2017.0201
68. Ameline, C, Beaurepaire, A, Ory, F, de La Harpe, M, Dainat, B, and Dietemann, V. Differential resistance across paternal genotypes of honey bee brood to the pathogenic Bacteriummelissococcus plutonius. J Appl Entomol. (2023) 147:85–93. doi: 10.1111/jen.13087
69. Hýbl, M, Bohatá, A, Rádsetoulalová, I, Kopecký, M, Hoštičková, I, Vaníčková, A, et al. Evaluating the efficacy of 30 different essential oils against Varroa destructor and honey bee workers (Apis mellifera). Insects. (2021) 12:1045. doi: 10.3390/insects12111045
70. Santos, RCV, Lopes, LQS, Alves, CFS, Fausto, VP, Pizzutti, K, Barboza, V, et al. Antimicrobial activity of tea tree oil nanoparticles against American and European foulbrood diseases agents. J Asia Pac Entomol. (2014) 17:343–7. doi: 10.1016/j.aspen.2014.02.003
71. Arrese, EL, and Soulages, JL. Insect fat body: energy, metabolism, and regulation. Annu Rev Entomol. (2010) 55:207–25. doi: 10.1146/annurev-ento-112408-085356
72. Pang, C, Dong, K, Guo, Y, Ding, G, Lu, Y, Guo, Z, et al. Effects of three types of pollen on the growth and development of honey bee larvae (Hymenoptera, Apidae). Front Ecol Evol. (2022) 10. doi: 10.3389/fevo.2022.870081
73. Lamontagne-Drolet, M, Samson-Robert, O, Giovenazzo, P, and Fournier, V. The impacts of two protein supplements on commercial honey bee (Apis melliferal.) colonies. J Apic Res. (2019) 58:800–13. doi: 10.1080/00218839.2019.1644938
74. Crailsheim, K, Brodschneider, R, Aupinel, P, Behrens, D, Genersch, E, Vollmann, J, et al. Standard methods for artificial rearing Ofapis melliferalarvae. J Apic Res. (2013) 52:1–16. doi: 10.3896/IBRA.1.52.1.05
75. Brødsgaard, CJ, Ritter, W, and Hansen, H. Response of in vitro reared honey bee larvae to various doses of Paenibacillus larvae larvae spores. Apidologie. (1998) 29:569–78. doi: 10.1051/apido:19980609
76. Bellah, H, Seiler, NF, and Croll, D. Divergent outcomes of direct conspecific pathogen strain interaction and plant co-infection suggest consequences for disease dynamics. Microbiol Spectrum. (2023) 11:e0444322–2. doi: 10.1128/spectrum.04443-22
77. Ory, F, Dietemann, V, Guisolan, A, von Ah, U, Fleuti, C, Oberhaensli, S, et al. Paenibacillus melissococcoides sp. nov., isolated from a honey bee colony affected by European foulbrood disease. Int J Syst Evol Microbiol. (2023) 73:005829. doi: 10.1099/ijsem.0.005829
78. Killer, J, Dubná, S, Sedláček, I, and Švec, P. Lactobacillus apis sp. nov., from the stomach of honeybees (Apis mellifera), having an in vitro inhibitory effect on the causative agents of American and European foulbrood. Int J Syst Evol Microbiol. (2014) 64:152–7. doi: 10.1099/ijs.0.053033-0
Keywords: European foulbrood, Melissococcus plutonius , clonal complex, secondary invaders, microbial ecological interactions, probiotics
Citation: Mallory E, Freeze G, Daisley BA and Allen-Vercoe E (2024) Revisiting the role of pathogen diversity and microbial interactions in honeybee susceptibility and treatment of Melissococcus plutonius infection. Front. Vet. Sci. 11:1495010. doi: 10.3389/fvets.2024.1495010
Edited by:
Izhar Hyder Qazi, South China Agricultural University, ChinaReviewed by:
Abraham Loera Muro, Centro de Investigación Biológica del Noroeste (CIBNOR), MexicoMichael Lattorff, University of KwaZulu-Natal, South Africa
Copyright © 2024 Mallory, Freeze, Daisley and Allen-Vercoe. This is an open-access article distributed under the terms of the Creative Commons Attribution License (CC BY). The use, distribution or reproduction in other forums is permitted, provided the original author(s) and the copyright owner(s) are credited and that the original publication in this journal is cited, in accordance with accepted academic practice. No use, distribution or reproduction is permitted which does not comply with these terms.
*Correspondence: Emma Allen-Vercoe, ZWF2QHVvZ3VlbHBoLmNh