- 1Department of Veterinary Microbiology and Pathology, College of Veterinary Medicine, Washington State University, Pullman, WA, United States
- 2Parasitology and Animal Diseases Department, Veterinary Research Institute, National Research Center, Giza, Egypt
- 3Animal Disease Research Unit, Agricultural Research Service, United States Department of Agriculture, Pullman, WA, United States
Parasite infections transmitted by vectors such as ticks and blood-sucking arthropods pose a significant threat to both human and animal health worldwide and have a substantial economic impact, particularly in the context of worsening environmental conditions. These infections can manifest in a variety of symptoms, including fever, anemia, jaundice, enlarged spleen, neurological disorders, and lymphatic issues, and can have varying mortality rates. In this review, we will focus on the current state of available vaccines, vaccine research approaches, and trials for diseases caused by vector-borne blood parasites, such as Babesia, Theileria, Anaplasma, and Trypanosoma, in farm animals. Control measures for these infections primarily rely on vector control, parasiticidal drug treatments, and vaccinations for disease prevention. However, many of these approaches have limitations, such as environmental concerns associated with the use of parasiticides, acaricides, and insecticides. Additionally, while some vaccines for blood parasites are already available, they still have several drawbacks, including practicality issues, unsuitability in non-endemic areas, and concerns about spreading other infectious agents, particularly in the case of live vaccines. This article highlights recent efforts to develop vaccines for controlling blood parasites in animals. The focus is on vaccine development approaches that show promise, including those based on recombinant antigens, vectored vaccines, and live attenuated or genetically modified parasites. Despite intensive research, developing effective subunit vaccines against blood stage parasites remains a challenge. By learning from previous vaccine development efforts and using emerging technologies to define immune mechanisms of protection, appropriate adjuvants, and protective antigens, we can expand our toolkit for controlling these burdensome diseases.
1 Introduction
The impact of blood parasites is scaling up globally. The increasing costs of control using acaricides, insecticides, and treatments of infected animals, associated with the emergence of drug resistance and environmental hazards, accelerated efforts to find effective vaccines against hemoparasites using rational approaches. Moreover, it is known that animals that resolve and recover from acute blood-borne infectious diseases, usually become asymptomatic carriers for the pathogens and may act as reservoirs of the infective agents that cannot be clinically differentiated from the non-infected ones (1). Alternative methods for the control of hemoparasites usually involve targeting their vectors, which is frequently a very difficult approach due to the existence of wildlife reservoirs and the development of acaricide resistance, but vector control could also be achieved by developing effective anti-vector vaccines.
Despite many efforts on the development of efficient vaccines, there are still many factors that can impact the efficacy of vaccines. These combined factors may include issues associated with the hosts, human-related activities, and the types of vaccines.
Among host related factors, it should be considered: (i) animal breed variations; (ii) age-related factors; and (iii) incubation periods in case of infected animals. Important human related factors are those related to vaccine design, formulation, and administration. This includes the nature of vaccine, antigen definition and concentration, vaccine stability, expiration date, vaccine material reconstitution, different administration routes (i.e.: intranasal, subcutaneous, intramuscular) and schedules of vaccination. Users will also need to be aware of appropriate vaccine storage, shelf life, and the logistics of shipment to different locations. Regarding vaccine-related factors, we should consider first the nature of the antigenic components of the vaccine which may include, the use of infectious pathogens or distinct noninfectious components of the pathogens, purified recombinant antigens representing molecules of the pathogens, or other components, including nucleic acids encoding for protective antigens. Using attenuated forms of infectious pathogens may elicit strong and long-lasting immunity, but this approach may result in undesirable spreading of the infectious agents among animals in the field and may convey the risk for reversion to virulence. Also, the degree of attenuation, either in vitro or in vivo using live animals, is considered an important feature in the preparation of these types of vaccines. On the other hand, using noninfectious forms, materials, or antigens derived from the pathogens, including killed parasites, purified or partially purified antigen extracts, toxoids, defined recombinant or native subunit vaccines, or nucleic acid-based vaccines, is usually considered safer than the infectious forms, although usually, these approaches are generally less effective compared to live vaccines. Another important factor is the definition of proper, non-toxic, and cost-effective adjuvants, since most non-living vaccines usually require them in order to stimulate adequate and strong immune responses. Finally, deciding on the possible need for vaccine booster doses requires, definition of the characteristics of the vaccine booster, and the number of vaccine inoculations and the frequency required for optimal coverage and efficiency. Importantly, determining these parameters also requires a previous assessment of the quantitative and qualitative responses of the immune systems of vaccinated animals. In addition, the costs associated with the applications of vaccine boosters could be an important consideration to evaluate vaccine practicality.
Achieving, licensing, and marketing efficient vaccines against hemoparasites is also not a trivial endeavor. The relatively slow pace for developing efficient vaccines is due, at least in part, to the typical complex life cycles of the responsible agents, our limited knowledge of protective immune mechanisms, and the fact that parasites are well adapted to their hosts and evolved complex and efficient mechanisms of immune evasion, including antigenic variation. The following sections describe features of selected blood-borne parasites of high impact currently occurring in animals globally (Babesia, Theileria, Anaplasma and Trypanosoma), their associated diseases, and different approaches employed for developing vaccines against these pathogens. Also, this review will briefly discuss the current vaccine and control measures of tick and blood-sucking vectors.
There is an urgent need for more research seeking effective strategies to produce durable and effective vaccines for better disease control. Understanding the parasite biology, the host immune responses against the infection, and the smart use of recently developed omics tools will undoubtedly facilitate vaccine development for improved disease control and parasite transmission.
2 Brief outlook on selected blood borne pathogens of veterinary relevance
2.1 Babesia parasites
Babesia (B) spp. are apicomplexan parasites that cause Babesiosis and can be transovarial transmitted by different kinds of Ixodes ticks. The disease can affect many mammals, including cattle, horses, sheep, goats, swine, cats, and dogs, and can also be fatal to humans (2). Babesiosis is considered as the second most important blood-borne parasitic disease of animals of veterinary importance, only behind Trypanosomiasis (3). Babesia sp. are transovarially transmitted parasites with a complex life cycle involving intracellular stages in at least two types of hosts, vertebrates, and tick vectors. Importantly, Babesia parasites undergo sexual reproduction in the gut of their definitive tick host, where they are also able to invade distinct types of cells (such as gut epithelial, ovary, and salivary gland cells), but they are only able to invade the erythrocytes of their vertebrate hosts, which can aid the parasites by providing adequate nutrition and a relatively protected environment for parasite expansion though asexual reproduction. Babesia comprises more than 100 species that infect erythrocytes in many vertebrates (4). Babesiosis can affect different livestock in tropical and semitropical regions worldwide, including ovine and caprine, but globally effective and fully safe vaccines against these species remain unavailable. Importantly, Babesia parasites have a life cycle that includes sexual reproduction in the midgut of their ixodid tick vectors, which eventually leads to transovarial transmission and the expansion of the parasites in the tick vectors. In this review we will focus mainly on Babesia species that infect cattle, equids, and dogs, since they have received more attention for vaccine development.
Bovine babesiosis can be caused by different Babesia spp. such as B. bovis, B. bigemina, B. divergens, B. orientalis, and B. major. In addition, B. divergens, which is prevalent in Europe, occasionally can also infect humans (5). However, B. bovis is considered as the most virulent Babesia spp. responsible for bovine babesiosis (6). This acute and persistent disease poses a significant global challenge for cattle production. Clinical signs range from mild to severe, depending on animal age, impacting animal health and productivity (7), and may include anemia, fever, ataxia, cerebral signs, abortions, anorexia and kidney damage, among others. Young calves ranging from 6–8 months old show some sort of increased resistance likely because they can generate more efficient innate immune responses against the parasites that prevent the devastating effects of acute infections. In contrast, infected adult cattle are more prone to display clinical signs of acute babesiosis, which are presented mainly as fever, anemia, loss of appetite, and weight loss (8). In severe cases of infection by B. bovis, animals suffer from neurological manifestations, due to parasite sequestration in the brain blood vessels, which may eventually lead to animal death (7, 8). Babesia species can also infect dogs and/or cats, as well as many wild animals, but none of them are known to be of zoonotic importance (9). Canine babesiosis is also a significant tick-borne disease caused by various species of the protozoan genus Babesia (10). Large and small forms of Babesia species (B. gibsoni, B. canis, B. vogeli, and B. microti-like isolates also referred to as “B. vulpes,” “Spanish dog isolate,” “Babesia cf. microti” and “Theileria annae”) can infect dogs with different levels of clinical signs (11, 12). Although canine babesiosis is mostly transmitted by tick vectors, it can also be transmitted to healthy hosts directly by blood transfusion, vertically (13), and by direct contact, as in case of fighting dogs, through wounds, saliva, or blood ingestion (14, 15). Equids also can be infected by Babesia spp., B. caballi, as one of etiological agents of equine piroplasmosis (EP) which is considered as an economically important disease of equids (16). In addition, EP can be caused by the Babesia-related Theileria equi parasites, as it will be discussed below, and vaccines remain needed for controlling this disease (16, 17). The small ruminant industry is a very significant component of livestock production especially in developing countries and in poor rural communities worldwide. B. ovis is the main causative agent of ovine babesiosis which has major economic impact on small ruminant (sheep and goat) industry in tropical and subtropical areas. Ovine babesiosis is transmitted by Rhipicephalus ticks, in particular R. bursa (18) and it could result in mortality if animals remain untreated (19, 20).
2.2 Theileria parasites
Theileria (T.) spp. are tick-borne apicomplexan parasites responsible for theileriosis, an important disease that affects cattle, equids and other animals worldwide with variable clinical signs. Theileria parasites are piroplasmid parasites which are closely related to Babesia that can also be transmitted by ixodid ticks. However, there are two key differences among these two parasites: one is the ability of Theileria parasites to infect more than a single type of cells in their vertebrate hosts, and another one is their transstadial, rather than transovarial, mode of transmission by ticks. Theileria parasites also have complex life cycles, and similar to Babesia parasites, they also develop sexual stages, kinetes and sporozoites inside its tick vectors. However, after the infection through the tick bite, Theileria parasites develop schizonts and piroplasms once established in their vertebrate hosts (21, 22). In the infected animals, schizonts form merozoites, which in turn parasitize the erythrocytes, which then develop into piroplasms. The piroplasms undergo sexual reproduction after being acquired by ticks during feeding on an infected host. Infected ticks can later infect another host with Theileria sporozoite stage parasites by transstadial transmission, regardless of their status of naïve or infected (21). T. annulata, T. parva and T. orientalis are cattle parasites (23) while T. equi and T. haneyi infect equines (16). T. lestoquardi and T. ovis can infect small ruminants (24). Theileria parva, T. annulata, and T. orientalis are the major causes of East Coast fever (ECF), tropical theileriosis, and oriental theileriosis, respectively (23). Based on their ability to infect leukocytes, Theileria parasites can be classified as host-cell transforming and non-transforming species. Thus, in the case of T. annulata, and T. parva infective sporozoites infect leukocytes, to develop into macro-schizonts causing uncontrolled leukocyte proliferation (25, 26). Eventually, these parasites produce merozoites that invade erythrocytes. In contrast, T. equi and T. orientalis do not induce uncontrolled leukocyte proliferation in horses and cattle, respectively (27). However, T. orientalis merozoites invade the host red blood cells, leading to anemia which is associated with the clinical signs of acute oriental theileriosis (28).
2.3 Anaplasma
Anaplasma (A) spp. is a vector-transmitted rickettsia, that resides in blood cells of its vertebrate hosts, leading to the disease anaplasmosis in tropical and semitropical areas (29). Anaplasmosis can be transmitted among animals by mechanical and/or biological vector transmission. Mechanical transmission may occur at least via two ways, either through contaminated surgical equipment (fomites) carrying the infected blood cells, or by mouthparts of biting flies who carry an Anaplasma species (30). Biological vector transmission mainly occurs by the bite of ticks (many different tick species) infected with the blood parasite. Inside the ticks, Anaplasma can survive and multiply, or it can stay dormant for months till transmission to another animal through a tick bite (31).
The clinical manifestations of anaplasmosis caused by A. marginale and A. centrale can be variable and similar to those caused by Babesia parasites. Depending on several factors, the intensity of disease can range from mild, lacking clinical signs, to severe, with elevated morbidity and mortality in affected ruminants. However, the infected animals become lifelong carriers that may become reservoirs for the pathogen (32). In addition, A. ovis can infect sheep, goats and some wild ruminants causing anaplasmosis (33, 34). Infections by A. phagocytophilum (transmitted by Ixodes ricinus) in dogs have been described mainly in northern and central Europe while infection with A. platys (transmitted by Rhipicephalus sanguineus) was identified in dogs from Mediterranean basin Romania, Turkey, Greece, Italy, France, Spain and Portugal (35). Both A. phagocytophilum and A. platys can infect other animals in addition to dogs, such as cats, sheep, goats, cows, equines, rodents, roe deer, deer, as well as other wild mammals, and even birds, as in case of A. phagocytophilum (36, 37). In general, it’s difficult to control anaplasmosis efficiently using vaccination approaches because of the ability of the responsible organisms for undergoing antigenic variation and their genetic variability. Also, the occurrence of multiple hosts and arthropod vectors, as well as the different mechanisms of transmission (biological, mechanical, and transplacental) may also impose important challenges for efficient control (38, 39).
2.4 Trypanosoma parasites
Trypanosoma (T) spp. is also an important blood parasite causing trypanosomiasis in animals and humans. These parasites are kinetoplastids, a monophyletic group of unicellular parasitic flagellate protozoa. Trypanosomiasis is a disease geographically constrained due to the nature of its arthropod vectors with strict requirements in terms of climate and environment. It is known as Surra diseases in South and Central America, Northern Africa, the Middle East, Asia, Indonesia, and Philippines, but it is also known as African animal trypanosomiasis in Central and Southern Africa (40). This disease is considered endemic in at least 37 of 54 countries in Africa (41). It can be transmitted biologically and mechanically by hematophagous insects by biting. The vectors involved in transmission include Stomoxys, Tabanids, and Hippoboscids. However, the parasite can also be sexually transmitted as in equine species infected with T. equiperdum. The signs of disease ranged from acute, with high mortalities, to chronic forms, which are frequently concomitant with reduction in body weight, anemia, and infertility. Also, the T. evansi’s may cause immunosuppression, usually accompanied with secondary infections, which makes clinical identification more difficult (42). It affects horses, camels, cattle, sheep, goats, pigs and humans. Wild animals also can be infected with Trypanosoma parasites, but they rarely suffer from the disease, and can act as infection reservoirs for domestic animals.
In Africa, T. congolense, T. vivax, and T. brucei brucei are the most important trypanosome species affecting domestic livestock in cattle, sheep, and goats, while T. simiae can infect pigs, and T. evansi infects camels. In South America, T. vivax also has an impact on cattle production. While T. evansi affects camels in Asia and horses, cattle, and domestic buffalo in South America, India, and Southeast Asia (43). It is interesting to note that T. evansi is more common in camels, in contrast to dogs, donkeys, and horses. This may be due to the chronic nature of trypanosomiasis in camels. While infected camels may become weaker and emaciated as the disease progresses, the infection manifests acutely and is usually fatal in dogs and equines (44, 45). Interestingly, although camels as well as donkeys, dogs and horses are similarly exposed to vector challenges, those animals are less prone to be infected by trypanosomes. These may be due to feeding preference of vectors for camels, or the greater ability of those animals to put away the flies through head movements, skin rippling, and other behavioral avoidance mechanisms (46).
In Egypt, T. brucei evansi (T. b. evansi) is an enzootic organism found in Egyptian camels, which is genetically classified into types A and B (47). T. evansi can cause high parasitemia, especially in camels, horses, and dogs (sometimes cattle and buffaloes), and might act both as blood and tissue parasite, because of its ability to invade the nervous system not only in horses and dogs, but also in cattle, buffaloes, and pigs (48, 49). Data on the presence of T. evans has been reported in many regions of Upper and Lower Egypt, however, its epidemiological significance in Egypt remains scarce (50).
3 Preventive control measures against tick-borne pathogens of veterinary relevance
Vaccines are among the most efficient methods available to prevent infectious diseases. Ideally, vaccines should fully protect the recipient against infections, but this goal is very difficult to achieve against the selected blood borne pathogens discussed hereby. However, current vaccines help ameliorate the risk and impact of acute diseases caused by these groups of selected parasites in animals. Effective stimulation of protective immune responses by vaccines may lead later to a more complete protection in the field, once the animals are exposed to the pathogens. In some specific cases, it could be at least possible to develop vaccines that can prevent infections, for example, by developing vaccines that can block the invading sporozoites, such as is the case of Babesia and Theileria parasites, preventing the vectors to infest their hosts, or prevent infection of the vectors by the parasites, such as in transmission blocking vaccines. As an alternative (or complementary) to these approaches, there are also research efforts focused on achieving vaccines that can block or control the development of the arthropod vectors, which ideally, could also be combined with pathogen-specific protective antigens.
So far, there are not globally effective, commercially available, vaccines against any of these blood parasites. In the next sections we will address vaccine trials performed against selected parasites of veterinary importance, and some new perspectives in vaccine development against these important blood parasites, mainly inspired by innovations and advancements in cell biology, vaccinology, immunology, and molecular biology, such as gene editing and recombinant protein production. The newly developed experimental vaccines discussed in the sections below typically consist either of subunit vaccines including native or recombinant versions of parasite proteins, crude antigen extracts derived from the parasites, or live parasites that are either attenuated or genetically edited. The goals pursued in the vaccine trials discussed in the next sections vary. While some of these vaccines are aimed to prevent acute disease symptoms, others are designed to prevent parasite transmission (also known as transmission blocking vaccines [“TBV”]). We will also address other approaches aimed at controlling both the parasite and its associated vector, known as dual vaccines (Figure 1).
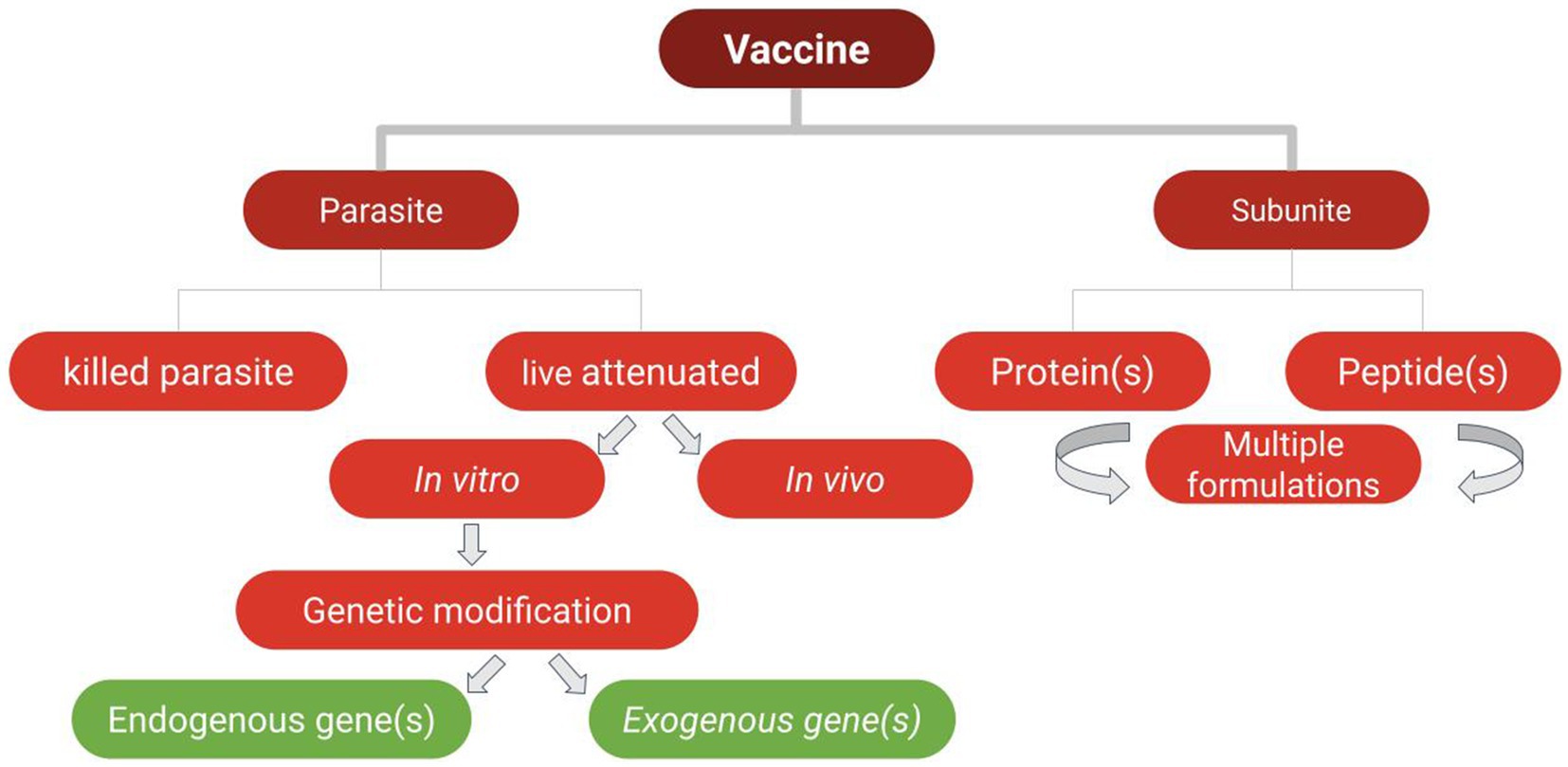
Figure 1. Types of vaccines used in vaccine trials against the selected parasites of veterinary importance addressed in this study.
3.1 Babesia parasite vaccines
Current measures aimed at the control of bovine babesiosis include individual or combined use of at least three approaches: tick vector control, animal therapeutic treatments, and vaccinations. However, each of these measures has its own pitfalls and limitations. Tick control using acaricidal drugs is a major approach to control babesiosis, but its intensive use invariably leads to the emergence of acaricide-resistant tick strains (51). In addition, this method of control may cause environmental hazards, and the introduction of dangerous chemical residues in the food chain (52). Babesicidal drugs currently used for the treatment of Babesia infected cattle, such as imidocarb, may also lead to the surge of drug-resistant parasites, especially when used in suboptimal doses, and worrisomely, the generation of drug residues or metabolites in milk and meat, This features make drug treatments expensive and inefficient as a disease-preventive tool in extensive production systems. Due at least in part, to these limitations, imidocarb is not licensed for use in some European countries (51).
Currently, live vaccines based on attenuated Babesia parasites are not available or allowed in many endemic countries. However, some countries with large cattle populations at risk, like South Africa, Argentina, Brazil, Uruguay and Australia opted for producing and using such attenuated vaccines. An important limitation of live attenuated vaccines is their safety, since that they can be potentially virulent, mainly for adult animals, and thus, these vaccines are in use for less than one-year-old calves (53). Moreover, some countries, including Australia and Argentina, use a trivalent vaccine which contain not only attenuated strains of B. bovis and B. bigemina, but also A. centrale, an organism of low virulence originally identified in South Africa (54), that can elicit partial protective responses against A. marginale. Trials involving live Babesia vaccines are discussed in detail below.
There are also a few vaccine options that are commercially available to prevent canine babesiosis in different countries. The Pirodog® (Merial) vaccine, currently available in the European market is based on soluble parasite antigens obtained from culture media supernatants (55, 56). This vaccine induces partial protection and can reduce severe clinical signs but does not prevent infections. The vaccine is recommended for administration into 5 months of age dogs, with annual booster doses (57, 58). It is worth mentioning that chemoprophylactic approaches are also used as alternatives to vaccination in dogs. For instance, the carbanilide derivative Imidocarb dipropionate has been shown to be effective against B. canis infection. The application of a single subcutaneous dose (6 mg/kg) of this drug demonstrated protection for two weeks (59). Doxycycline (5 mg/kg/day) also reduces the severity of disease in dogs that experimentally infected with an extremely pathogenic isolate of B. canis (60).
The following sections describe recent efforts toward developing vaccines against Babesia parasites using different approaches, including subunit, whole parasite, and culture supernatant vaccines (Figure 1).
3.1.1 Vaccine trials against Babesia based on subunit and native crude extracts antigens
Researchers have identified several potential recombinant and native subunit and crude antigenic extracts that have been proposed as vaccine candidates for bovine babesiosis, since they can prevent severe clinical signs (Table 1). Crude extract antigen and subunit vaccines have been designed and tested in several reported in vivo trials.
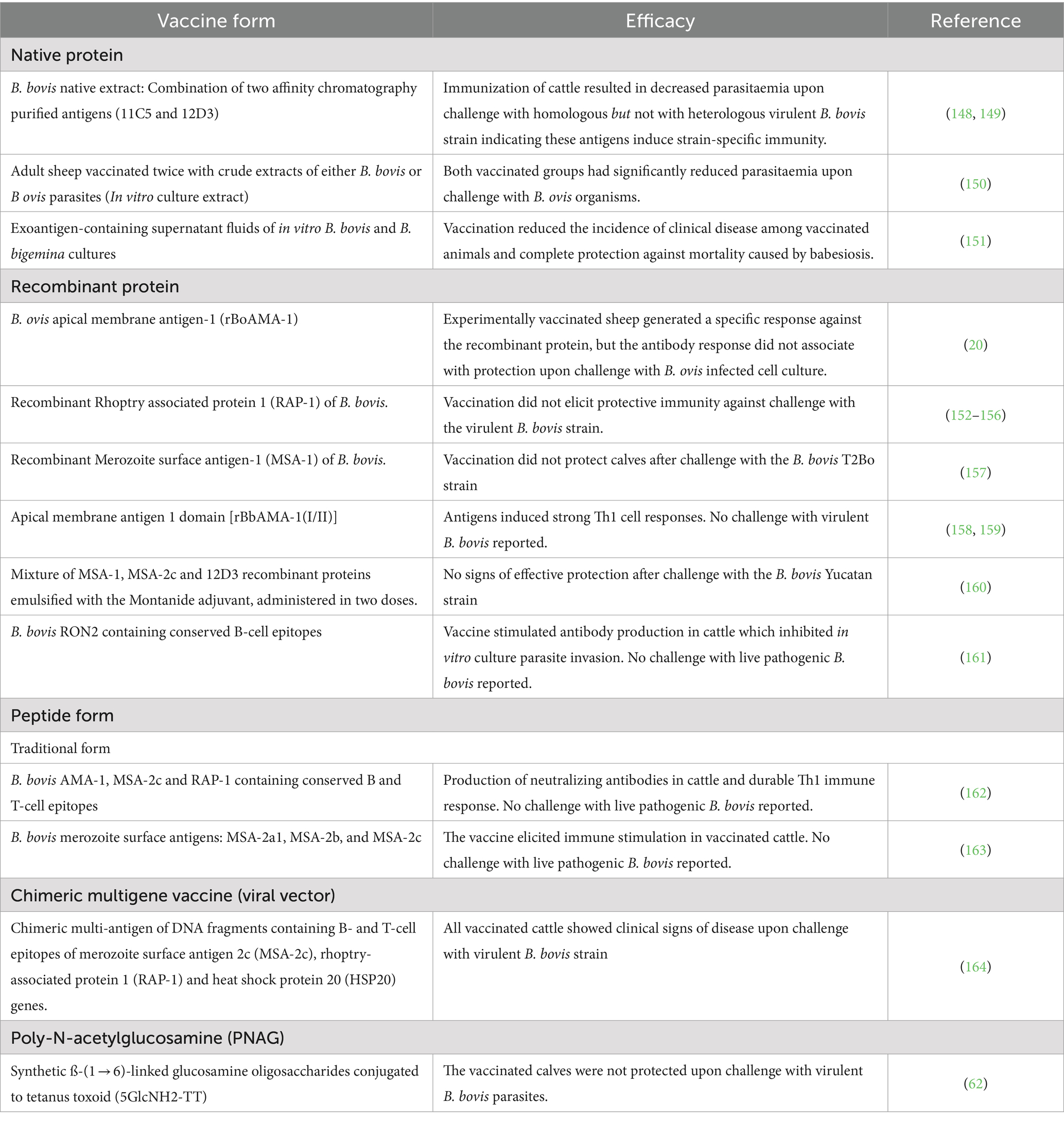
Table 1. Different forms of subunit and native crude extract vaccine trials against blood stage of Babesia parasites.
Typically, a subunit vaccine contains selected purified molecules derived from a target pathogen that are antigenic and able to elicit a protective immune response. These antigens may be either purified native or recombinant proteins, or in other forms, as described below. Unlike live attenuated or non-viable parasite derived vaccines (such as irradiated, or chemically inactivated), subunit vaccines typically include only specific and selected components such as proteins, polysaccharides, or peptides (61). As such, subunit vaccines are considered safer and more stable than live vaccines because they do not contain infectious components.
Because this property applies to these two types of antigens, vaccine trials involving subunit and native crude extracts derived from the parasites, are included together in this section.
Subunit vaccine candidates can take various forms, including purified recombinant protein(s), and/or synthetic peptide(s) representing relevant B and T cell epitopes, or mixed formulations. Also, custom designed synthetic genes encoding for a combination of several protective B and/or B and T cell epitopes can also be used for expression in prokaryotic or eukaryotic systems (62), or even for direct injection of coding DNA into the animals using specific devices such as gene guns (Table 1). In addition, animal vaccine trials have also been performed for experimental subunit vaccines based on recombinant proteins aimed at blocking pathogen transmission (Table 2).
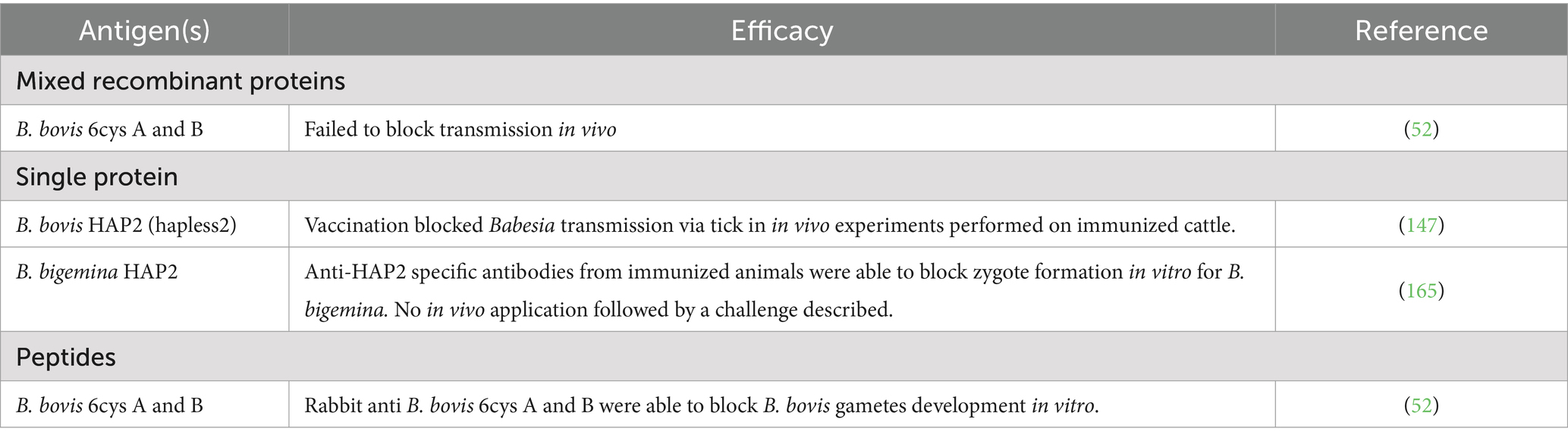
Table 2. Different forms of subunit vaccine trials against sexual stage of Babesia parasite (Transmission blocking vaccine).
Several strategies for selecting protective blood stage or transmission-blocking antigens were proposed, with some focused on functionally relevant molecules and others using pragmatic approaches like previously defined surface exposed, neutralization sensitive and conserved molecules. Protection in blood stage vaccines is typically defined by the ability of vaccinated animals to survive standardized challenges from Babesia merozoites from virulent strains, and the results and efficiency in these trials are usually compared to protection resulting from live vaccinations. The following Tables 1, 2 illustrate different vaccination trials for blood and sexual stage subunit and crude antigenic extracts so far described in the literature.
Regarding blood stage vaccines, none of the subunit vaccination trials targeting blood stage parasites described in the literature were able to elicit fully efficient and long-lasting protection against challenge with virulent Babesia strains. Some of these unsuccessful results may be due, at least in part, to the structural and antigenic differences between native and recombinant versions of the antigens, including parasite-specific protein folding and post-translational modifications of the native proteins, which may result in critical differences in their antigenicity, when compared to recombinant proteins produced in heterologous expression systems (63). For instance, immunization experiments using native purified B. bigemina antigens, including RAP-1 of B. bigemina resulted in partial protection (64).
There are other issues that might significantly influence the outcome of the blood stage vaccine trials involving recombinant proteins and synthetic peptides described in Table 1. One important factor is the nature and severity of the challenge used. While in nature animals get usually infected by ticks, which inoculate variable amounts of antigenically distinct sporozoite stage parasites in the blood of the vertebrate host, the challenges in all these trials were invariably performed using a large, fixed, amounts of blood stage merozoites from a defined highly virulent strain of Babesia. The current method of challenge using large numbers of virulent merozoites may be realistic for assessing immunity elicited by live vaccines, but that may not be the case for other cases involving recombinant proteins. We thus suggest that a more realistic and standardized method of parasite challenge, preferably using sporozoite stage parasites or natural Babesia-infected tick field challenge, should be also developed and adopted for future trials.
However, in case of TBV, while the full-size E. coli-derived recombinant version of the B. bovis Hap2 protein was able to block transmission via ticks, that was not the case for full size eukaryotic system-derived r6cys proteins. It was hypothesized that the transmission-functional regions of these proteins were poorly immunogenic when presented in the context of the full- size 6cys A and B proteins. This notion was supported by additional experiments that identified specific and poorly immunogenic transmission-sensitive regions of these proteins that may contain epitopes responsible for transmission reduction function (52). Future vaccination experiments using these specific regions might result in the definition of alternative candidates for TBVs based on 6cys proteins.
Worthy of note that there are other promising functional approaches toward Babesia vaccine candidate definition that had been proposed in the last few years (65). One approach is based on the selection of critical protein-derived conserved regions related to parasite adhesion. Interestingly, conserved high activity binding peptides (cHABPs) to the erythrocytes were identified in the genome of B. bovis using intensive in silico analysis (66). Similarly, using a bioinformatics approach researchers identified a sub-immunodominant B-cell epitope in a highly conserved 15 amino acid region of the Rhoptry Associated Protein Related Antigen (RRA) of B. bovis, that is also a candidate for inclusion in peptide-based subunit vaccines (67). These in silico subunit vaccine antigen candidate identification approaches, could also be extended not only against Babesia but also against other blood parasites, due to the availability of annotated genomes for most parasites of medical importance, with the addition of other omics analysis. Moreover, exploiting exosomes, which consists of small extracellular vesicles that serve as carriers for proteins, nucleic acids, and lipids in host-Babesia or parasite–parasite interaction should be also considered (68, 69). This approach was successfully applied in Plasmodium spp., and it allowed the identification of novel subunit vaccine candidates involved in non-classical protein secretion, pathogenesis, immune modulation, and parasite–host interactions (70). Its potential in disease pathogenesis was explored in two major human Babesia species, Babesia divergens, from in vitro culture and those from an in vivo B. microti mouse infection (71). Thus, further similar studies using this approach in Babesia vaccination are warranted.
3.1.2 Vaccine trials based on whole Babesia parasites
Using whole Babesia live or inactivated parasites in vaccination approaches has important advantages, since they should include most, if not all, antigens expressed at least in certain stages of the organisms, and in some cases, such as in live attenuated vaccines, they may overcome limitations due to antigenic variation and natural polymorphism (72), although this may depend on the method used to reduce the virulence of the parasites. These types of vaccines may contain live attenuated or genetically modified parasites, or whole inactivated (killed) parasites.
3.1.2.1 Inactivated (killed) parasite
Killed vaccines were achieved in different ways. One of those is the freeze-dried suspension of Babesia argentina (B. argentina) (currently known as B. bovis) parasite (73) and B. microti-killed parasites enclosed within liposomes which incorporated a mannosylated lipid core peptide. In both cases the vaccination induced protective immunity in cattle and in mice against B. argentina or B. microti, respectively. Also, chemical attenuation was performed on B. microti parasitized red blood cells from infected mice by using Tafuramycin-A (TF-A). A culture-based liposomal vaccine, a liposome containing killed parasite material, was also used as a vaccine in mice. The parasitemia was reduced in vaccinated animals upon challenge with the B. microti (74).
3.1.2.2 Live attenuated vaccines
Live vaccines based on attenuated parasites remain as the only preventive measure against bovine babesiosis caused by B. bovis and B. bigemina, and for canine babesiosis caused by B. gibsoni.
These live vaccines have several significant limitations, including the need of cold chain for transportation and short shelf-life, which is only about of 4 days from the production date when stored between 2 and 8°C. Also, live attenuated parasites strains may pose the risk of reversion to virulence upon exposure to the natural conditions in the field (52). Moreover, there is also the risk of transmitting other contaminating blood-borne pathogens during vaccination. This may occur because the process of attenuation requires serial infections passages on several splenectomized or spleen intact calves (for B. bovis and B. bigemina respectively) (75), and the large-scale production of the vaccine in some countries (i.e.: Australia) involves infecting splenectomized cattle as well. Yet, the method of vaccine production varies among countries, and in some cases such as in Argentina, the attenuated strains are expanded in in vitro cultures, rather than in splenectomized cattle, for vaccine production purposes. Finally, as mentioned before, there is an age restriction for the vaccination, and issues regarding dose standardizations (54).
Attenuation can be achieved from virulent B. bovis, B. bigemina, and B. gibsoni parasites by using different approaches (Tables 3, 4). Attenuation can be generated in vivo through successive rapid passages in splenectomised calves, in the case of B. bovis (76), or by slow passages in non-splenectomized calves for B. bigemina (77).
Attenuation can also be achieved in B. bovis by long term in vitro culturing of virulent parasites (6), by deep freezing for long periods in liquid nitrogen (78), using chemical treatments, or by irradiation (79). Tables 3, 4 show previously published vaccination trials performed using in vivo and in vitro live attenuated parasites in vaccine trials.
In general, live vaccines against cattle babesiosis are not recommended for use in adult animals, since they may induce acute disease. However, the three experimental in vitro culture immunizations (6–8) performed in cattle described in Table 4 suggest that the attenuated S74-T3Bo is also a potentially efficient and sustainable attenuated candidate vaccine strain not only because was able to prevent acute bovine babesiosis upon challenge with a homologous virulent strain in highly susceptible adult cattle and young animals, but also because it was shown to be non-tick transmissible. However, the effectiveness of this vaccine still needs to be tested against heterologous virulent strains. In summary, this in vitro cultured attenuated strain might become an optimal choice as a component of attenuated live vaccine because it is a clonal-like strain (6), and thus might be unlikely to revert to virulence. Nonetheless, more experimental vaccinations tests using the LTCP attenuated S74-T3Bo strain are still needed in a larger number of animals and using different challenge strains. Similarly, in vitro culture attenuated strains of B. bigemina and B. gibsoni (Table 4), may be also optimal candidates for “universal” and more standardized effective in vitro culture attenuated vaccines, but again, more testing, including studies on tick transmissibility, are warranted on these strains.
3.1.2.3 Genetically modified Babesia parasites
The advancements in the field of the gene editing, including transfection technologies and CRISPR/cas9, in combination with in vitro culture systems, and other related technologies, may lead eventually to the generation of genetically modified live vaccines. This type of vaccines can serve as dual vaccines, targeting both the parasites and their vectors, in an integral approach of control. Still, there are important challenges for approving, producing, and commercializing this type of vaccines. In addition, there is the need for large scale cultivation to produce the parasite, and being a live vaccine, its distribution may also generate costly and cumbersome logistic requirements, such as the need for a cold chain (80). Another limitation of this approach includes concerns derived from the application of the genetic modified vaccine into the field animals. Despite these potential obstacles, this approach may prove to be cost-effective under certain circumstances. The following scientific trials shown in Table 5 exemplify the innovations achieved in this area of research.
Both trials described in Table 5 presented evidence for the ability of transfected live attenuated parasites to protect against challenge with a virulent parasite strain. At the same time, the parasites used in these vaccination trials will not be able to be transmitted by competent ticks because these experimental vaccines are based on long term in vitro cultured B. bovis that likely lost their transmission phenotype (6). Moreover, the addition of a gene expressing protective antigens derived from the tick vectors to the B. bovis vaccine strain, increases the advantages of this live-vaccine approach by providing a dual effect against the pathogen and its vector.
3.2 Theileria parasite vaccines
The main strategies currently used to control theileriosis are based on the use of anti-theilerial drugs. Also, in the case of bovines, improved control includes the use of indigenous and cross breeds of cattle that are known to be more resistant to the parasites and its vectors, such as Bos indicus (21). Historically, vaccines based on attenuated macro-schizont cell lines were successfully used in case of T. annulata. However, since these are attenuated parasite lines, there is a concern of spreading infections in the field (81). Table 6 shows different antigens used in subunit, or combined subunit-live vaccine trials against Theileria parasites.
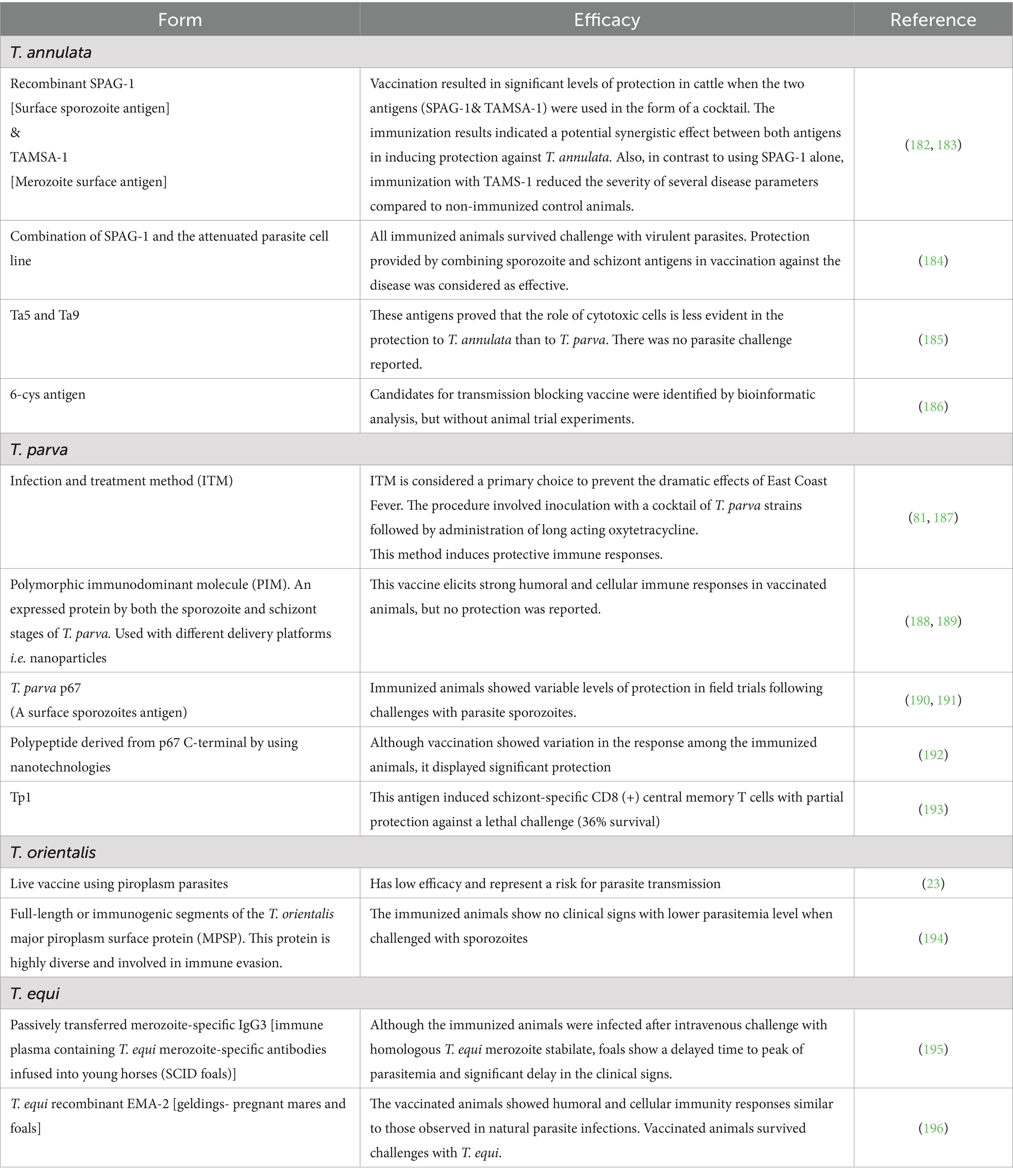
Table 6. Vaccine trials based on Theileria antigens associated with different parasite developmental stages.
Because of the current lack of commercial vaccines to control animal theileriosis, the variable therapeutics effectiveness (82), and the results derived from unsuccessful previous vaccination attempts, the main current control strategies against T. parva, T. annulata, and T. orientalis, in cattle, and T. equi in equines, still rely mainly on the control of their tick vectors. Ideally tick control should be done using environmentally friendly acaricidal drugs or tick vaccines, which can be complemented by parasite control using anti-theileria drugs. However, none of these options are currently available and more research in these fields is required.
Although the current use of ITM and cell line vaccines to control ECF and tropical theileriosis, respectively, are relatively effective, the procedure still has drawbacks. This includes the need for a cold chain for distribution, high costs, and the potential risk of tick transmission of Theileria parasites (83). Since ITM depends mainly on the use of oxytetracycline, there is also the concern of developing and expanding antibiotic resistances through food and milk contamination (84). The most widely used vaccines against T. annulata are attenuated schizont cell culture. The methodology of production and its safety evaluation had been evaluated (85, 86). This kind of vaccine is used in different countries such as Israel, Iran, Turkey, Spain, India, northern Africa, central Asia and the People’s Republic of China (87). A recent study in Egypt reported a vaccination trial in cattle using culture-attenuated schizont-infected cell lines isolated from Egypt. The vaccinated groups were inoculated with 4 mL (1 × 106 cells/ml) of the attenuated cell line. Three weeks after vaccination, calves of vaccinated and control non vaccinated groups were transported to the New Valley Governorate (Egyptian oasis), where they were kept under field conditions and exposed to natural Theileria annulata challenge. All animals in the control unvaccinated group showed severe clinical signs and died despite treatment with buparvaquone. In contrast, animals in the vaccinated group became seropositive without developing severe clinical signs other than transient fever. These findings indicate that the Egyptian attenuated cell line was successful in protecting against tropical theileriosis under field conditions (88). Although, cell culture vaccine against T. annulata has been recognized for more than three decades and has proven to be effective in the field, it still has limitations (87), and each country developed the vaccine from local isolates (89). Because of the continuous attenuation, some of the attenuated lines lost the ability to differentiate to erythrocytic merozoites (piroplasms) when inoculated to cattle, one example of that when Hyalomma nymphs fed on vaccinated cattle did not become infected (90). This pitfall, in addition to the problem in standardization of the antigenic composition of the cultured parasites and the need of a cold chain for distribution of the vaccine to the field are limiting factors in commercialization of this vaccine (89).
T. equi and B. caballi are both responsible for equine piroplasmosis, a disease that limits horse movement worldwide and seriously affects the development of horse industries and equestrian sports. Equine theileriosis caused by T. equi is mainly prevented in non-endemic areas worldwide by regulating the movement of horses from endemic countries. Also, for some countries, animals must be tested negative for T. equi serologically, as an obligatory procedure in animals’ importation (91). Thus, in order to generate potent vaccines, research should be focused ideally on targeting different parasite developmental stages, such as blood and sexual stages, and avoiding, if possible, antigens that may interfere with the current mandatory tests required for exportation of horses. Also, advances in reverse vaccinology should be applied. The similarities among different species of Theileria parasites genomes should be considered during vaccine approach. Importantly, identification of host immune correlates which are associated with protection against the parasite infection, would be of high value for vaccine design. Using effective adjuvants and innovative and practical vaccine delivery systems should also be considered as crucial aspects of vaccine development.
3.3 Anaplasma vaccines
Although Babesia parasites are apicomplexan protozoa and Anaplasma marginale is a rickettsia, they were historically linked since they form part of a complex of diseases also known as cattle tick fever or Bovine parasitic sadness (BPS) in several countries, sharing important features: they both infect exclusively erythrocytes in the vertebrate hosts causing a clinically related acute disease, and they are all transmitted by Rhiphicephallus (Boophilus) ticks, often co-occurring in endemic areas.
There are currently no worldwide effective vaccines to prevent canine anaplasmosis, caused by Anaplasma phagocytophilum and A. platys, nor for bovine anaplasmosis caused by A. marginale (92, 93). A. phagocytophilum has zoonotic potential and is responsible for human granulocytic anaplasmosis.
Many vaccine candidates have been evaluated for the control of bovine anaplasmosis, but the antigenic diversity of the pathogen has impaired many efforts to control the disease (92). However, a live heterologous vaccine based on A. centrale have been used since the early 20th century. A. centrale, is an organism isolated in South Africa, which is less virulent for bovines than A. marginale, and provides some degree of cross-protection against this pathogen in naive cattle. In some countries, A. centrale is added to live Babesia spp. vaccines, to make a trivalent vaccine (including B. bovis, B. bigemina, and A. centrale). This trivalent vaccine is in use as a live vaccine in countries such as South Africa, Australia, Argentina, Brazil, Uruguay (94). Despite the success of this vaccine to some extent, there are few reports indicating A. centrale was the cause of vaccine outbreaks, with fatalities (95). Furthermore, in some cases the vaccine failed to induce immunity against A. marginale challenge (96). Moreover, University Products LLC (Louisiana, USA) currently offers the only inactivated commercial vaccine against bovine anaplasmosis based on A. marginale1 (97). This vaccine has been field-tested for over 20 years (98). Although this vaccine does not prevent infection with virulent A. marginale, it induces enough protection in cattle against acute anaplasmosis (92). This vaccine requires only two doses in the first year, followed by one annual booster each year, and it is safe to be used in any stage of bovine pregnancy (98). Still this inactivated vaccine is not approved in many countries, but it is approved and established for use in the United States. The following sections provide a glimpse of some vaccine development efforts in the field of Anaplasma (Figure 1).
3.3.1 Whole organism vaccines
In this section, A. marginale vaccines based on whole organisms were introduced, which can be inactivated or live vaccines. Inactivated vaccine trials are shown in Table 7. The live vaccine contained the virulent form of the pathogen in a low dose, which may be derived from infected animals, or attenuated through multiple animal passages, or in long term in vitro culture. In case of Anaplasma, the mammalian in vitro culture system efforts, based on erythrocytes, have been explored without any success, either using bovine erythrocytes alone, or co-cultured with endothelial cells [bovine pulmonary artery] (99). Only several tick cell lines were successful to establish the Anaplasma in vitro culture for both A. marginale and A. centrale, but long-term in vitro cultures of these organisms in erythrocytes was not yet achieved (100, 101). However, cattle immunization with in vitro cultured A. marginale induced an antibody immune response but without the expected protection level (102). The trials of live Anaplasma vaccines are shown in Table 8.
3.3.2 Subunit vaccines against Anaplasma organisms
Novel omics technology has yielded a list of antigens that could help the researchers to explore new possible vaccine candidates. Protein function, localization, conservation, and either their dominant or subdominant antigenic characteristics, are the main criteria, among others, for antigen selection. Proteins as major surface proteins (MSPs), outer membrane proteins (OMPs) and several type 4 secretion system (TFSS) proteins (103) were intensively explored. Those proteins are involved in different function as adhesins to red blood cells and tick epithelial cells (MSP) (104), Adhesin/invasion (OMPs) (105) proteins can mediate transfer of DNA and proteins into eukaryotic host cells, may interfere and are important for the survival of intracellular bacteria (TFSS) (106, 107). The antigens were tested as vaccine candidates in a form of recombinant protein(s), DNA, plasmids, or synthetic peptides and even in some cases, as mixtures of different forms. Recently “quantum vaccinomics” was applied to identify and characterize A. phagocytophilum MSP4 protective epitopes by a microarray epitope mapping. The identified candidate protective epitopes, or immunological quantum, were used to design and produce a chimeric protective antigen that was used in vaccination trials of rabbits and sheep. The resulting antibodies from the two types of immunized animal hosts were equally effective to block cell infections in an in vitro inhibition assay of A. phagocytophilum. The results from these experiments supported the use of quantum vaccinomics as an effective tool for the design of new chimeric candidate protective antigens, as a better alternative to the use of a full-size single protein (MSP4) for vaccine development (108).
3.3.3 Genetically modified Anaplasma pathogens
Genetic modification was achieved in Anaplasma pathogens either by knocking out biologically important proteins, or by introducing an exogenous gene into its genome to express a protein able to generate protective immune responses. These modifications were performed in in vitro culture to achieve genetically modified organisms. A mutant strain was attained in A. marginale by transposon mutagenesis of the A. marginale Virginia strain to reduce the expression of OMP (109). Although this mutant strain can be transmitted by ticks, it showed reduced infectivity in both intact and splenectomized cattle (110). However, this mutant was not tested for protection against homologous or heterologous challenge. Another immunization trial was performed by the mutant of A. centrale with a transposon-mediated insertion of a construct containing Turbo GFP as a marker, and antibiotic resistance genes for selection. Upon animal immunization, it provided immunity and showed clinical signs like the infection with unmodified A. centrale but with lower percentage of infected RBCs (110). Both trials were considered as an advance for disease control, but the possibilities of other pathogen transmission like mycoplasmas and even viruses present in the culture systems should also be taken into consideration (92).
No universal vaccine is yet available to protect against diverse geographic strains of A. phagocytophilum and A. platys, Anaplasma species which are known to infect dogs (39) and other animals. Anaplasma spp. can be transmitted mainly by Rhipicephalus and Ixodes for A. platys (33, 111) and Dermacentor, Hyalomma and Rhipicephalus in case of A. phagocytophilum (112). Whole genome sequencing of both A. phagocytophilum and A. platys, allowed for the identification of several potential candidates for vaccine development. It was found that nine of proteins that have an immunogenic potential like the Asp14, Asp55, Msp5, Msp2, AipA, OmpA, APH 0032, and APH 1384 antigens of the type IV secretion system of A. phagocytophilum (113–117) can be rational candidates for vaccine development.
3.4 Trypanosoma parasite vaccines
Currently there is no available vaccine for trypanosomiasis prevention. One of the main challenges to develop a vaccine is the mechanism that Trypanosoma parasites use to evade the host immune system, by constantly varying the structure of their surface coating. The antigenic variation operated by the parasites makes it difficult to identify appropriate candidates for vaccine development, especially for the case of subunit vaccines.
There are limited options to prevent trypanosomiasis within vertebrate hosts. In addition, the increase of resistance toward trypanocidal drugs makes chemotherapy, the major means to control infection, difficult to use. Additionally current drugs have various other shortcomings, including toxicity and limited efficacy (118). The drugs commonly used for the treatment of infected domestic animals with T. evansi are Diminazene aceturate, which causes some toxicity to the host (119) and isometamidium chloride (120). In this scenario, vector control remains a very important step for disease prevention (121). Another important challenge, despite sporadic reports, is the lack of full knowledge on the geographic distribution of the disease and its transmitted vectors, which affects the control initiatives that require reliable information.
To control this disease, different approaches should be addressed, but the main and more practical strategy is just minimizing contact with the vector tsetse flies. The principal control method is targeting the tsetse fly using insecticides, which has environmental drawbacks. These drawbacks led to the development of bait technologies which include traps and insecticide-impregnated targets (122). Another approach is to use naturally trypano-tolerant breeds of livestock, which is considered as an economical addition to the intervention tools (41).
Previous attempts to develop subunit vaccines against African trypanosome infections have highlighted the difficulties in overcoming the immune evasion mechanisms that have been evolved by these parasites for survival (123). Many antigens were chosen as vaccine candidates. Such is the case of the variable surface glycoprotein (VSG) (124), which provided partial protection, but became non-effective after some time (125). Also, vaccine formulations based on invariant surface glycoproteins and subcellular proteins of the cytoskeleton, like actin and tubulin (123) generated unsatisfactory and non-protective immune responses. However, the advancement in the field of bioinformatics, together with the availability of omics data from different organisms is allowing the design of new generation vaccines that may offer better antigenicity and safety profile. The so-called reverse vaccinology approach, which depends mainly on omics data, permits the design of vaccines that can involve many antigens of different expected important functions. Here are some examples of vaccination trials performed against Trypanosoma parasites.
3.4.1 Subunit vaccines against Trypanosoma parasites
One vaccine trial was based on a single recombinant protein comprising the extracellular region of a conserved cell-surface protein that is localized to the flagellum membrane invariant flagellum antigen from T. vivax. When this protein was used in vaccination in mice it resulted in survival of 10 out of the 15 mice which also were protected beyond at least day 170 (126). This formulation is considered as the first ever successful vaccine trial against this devastating disease caused by Trypanosoma parasites (127).
3.4.2 Chimeric vaccine against Trypanosoma parasites
Advancements in computational modeling coupled with the availability of large amounts of omics data from different organisms have allowed the design of new generation vaccines. A multi-epitope vaccine (MEV) designed from a collection of antigenic peptides from conserved hypothetical plasma membrane proteins of Trypanosoma brucei gambiense. It was expected to give adequate immune stimulation but this vaccine was not tested with parasite challenge (128).
3.4.3 Genetically engineered Trypanosoma parasites
Since it is predicted that T. cruzi cyclophilin-19 (Cyp19) protein is important for parasite growth, a mutant parasite line lacking the Cyp19 gene was generated. The mutant parasites failed to replicate when inoculated into host cells in vitro or in mice, confirming that Cyp19 is critical for infectivity as well. Moreover, immunization with a T. cruzi Cyp19 deletion mutant protects 100% upon challenge of the mutant-immunized mice with virulent wild-type parasites, indicating the effectiveness of this line at preventing death from acute disease in mice. In addition, this mutant line did not cause clinical disease in immuno-deficient mice confirming their lack of virulence (129).
3.4.4 mRNA-based Trypanosoma vaccines
It is now known that there are many benefits of mRNA-based vaccines, which led researchers to use this approach into vaccine development against Trypanosoma parasites. The mRNA-based vaccine approach could prevent disease, but so far, no study was conducted regarding testing this concept (125).
4 Vaccines against vectors
4.1 Tick vaccines
Tick-borne diseases (TBDs), particularly those caused by blood parasites, pose a significant threat to global livestock industries. The widespread distribution of various tick species, combined with climate change and human activities, contributes to the outgoing expansion of tick populations. Anti-tick vaccines (ATVs) offer a promising, safe, and environmentally friendly approach to tick control. These vaccines not only reduce vector infestations on vertebrate hosts but also hinder the transmission of blood parasites, thereby mitigating the economic impact of tick-borne diseases at both herd and regional levels. ATVs also serve as an effective alternative to chemical tick control, as they are cost-efficient and can be applied across different host species (130). By minimizing the need for acaricides, ATVs help to prevent the emergence of acaricide-resistant tick strains, reduce contamination in the food chain, and promote environmental safety.
Two commercial vaccines were developed based on the glycoprotein Bm86 tick midgut protein, TickGARD Plus (131) and Gavac Plus (132) for use in cattle. This protein was derived from the Rhipicephalus microplus ticks, but it is also conserved in other related Rhipicephalus ticks. Those vaccines were developed and tested in Australia and Cuba, respectively (133), and were also used in other countries like Mexico and Venezuela against the cattle ticks R. microplus, R. australis and R. annulatus with different levels of efficacy, ranging between 10 and 89% (134, 135). In Cuba and Colombia, those vaccines were generally effective to reduce tick populations and the number of acaricide treatments, in addition to babesiosis and anaplasmosis infections, respectively (136). The TickGARD Plus vaccine is no longer used since 2021 in Australia because of the need for numerous applications (3–4 booster doses per year) (137). So, currently, the only commercially available anti-tick vaccine is Gavac® (138, 139).
Developing effective vaccines against ticks requires intensive research and costly experimental trials. Current approaches in tick vaccine research are focused on identifying potent candidates playing a critical role in tick biology that are capable of inducing cross-reactive immunity against multiple tick species. This requires the identification of highly conserved and functionally relevant antigens that are also exposed to antibodies in the host. It can be anticipated that recent progress in tick omics in addition to other novel molecular biology approaches, and a better understanding of tick biology, will be helpful for the identification of such antigens.
As it was described in the Babesia parasite vaccines section above, there is also the hope of developing dual anti-tick and anti-babesia vaccines.
4.2 Blood sucking flies’ control
To date, there is not a specific vaccine against blood-sucking flies. However, there are several other alternative methods available that can be applied and used in combination to approaches aimed at controlling the blood parasite transmission and its causing diseases, in order to protect livestock. Such methods include the following:
1-Physical barriers: Blood-sucking insects can cause allergic reactions in animals, and can also cause different diseases by transmitting several pathogens that are able to propagate in them. Physical barriers can be designed to prevent direct access of flies to animal skin (140). This approach includes the use of protective gear, typically fly sheets, leg wraps and masks. These methods are considered an easy, affordable, and reliable system to prevent insect bites and stings effectively and may prevent the use of chemicals such as insect repellents or insecticides decreasing the environmental hazard.
2-Insecticides: Insecticides can be applied to animals and their surroundings either to repel or to kill the blood-sucking flies. Application of insecticides should be used with caution and following the recommended doses to avoid the emergence of insect resistance strains and environmental pollution. Repellents can also be used effectively to prevent flies approaching the skin of target animals. Application of pesticides in animal treatments can be done using automatic sprayers, back rubbers and ear tags (141).
3-Biological control: this method, considered as one of the most environmentally friendly approaches, can potentially reduce the current reliance on insecticide-based control. This approach is based on the use of known natural predators or parasites that target blood-sucking flies. Non-insecticide-based strategies have been implemented in different blood sucking as mosquito (142) and ticks by entomopathogenic organisms such as nematodes (143) and protists or mites as biocontrol agents. However, little attention has been given so far to control methods based on biological control, and further exploration studies on the biocontrol of immature and adult stages of blood sucking flies are needed (144).
4-Environmental management: This approach involves adequate waste disposal and maintaining clean surroundings, which in turn discourages fly breeding. More importantly, both negative and positive impacts of insects on human and animal health and on the environment need to be addressed by public health professionals. It is also crucial to balance important aspects and goals in insect management approaches, which include regulating their production, exploiting their potential, and limiting their potential negative effect on animals and humans, and on the environment (145).
5 Conclusion
The more rational way to avoid blood parasites causing disease is to apply preventive measures against ticks and other transmitting vectors. To have effective and deployable vaccines is not a straightforward effort. There are many obstacles in the way of vaccine development, including the identification of proper vaccine antigen candidates. However, this task is currently facilitated by the availability of data derived from the application of available omics and other approaches, which can provide information on vaccine-targetable proteins that are exposed to the effectors of the immune system and may play key roles in the pathogens’ biology. Ideally, those antigens, in conjunction with proper adjuvants, should be able to induce desirable protective immune responses in immunized animals. Moreover, other aspects such as practicality of vaccine production, the choice of efficient and safe adjuvants (if needed), feasibility of shipment, shelf life, safety of the vaccine, licensing, and commercialization are also important points that also need to be considered for successful vaccine design.
Recombinant protein production is a technology usually applied to vaccine development. However, confirming that the structure of the recombinant protein faithfully resembles that of the native protein should be considered, so the relevant epitopes, including conformational ones, will be antigenic and reachable by the protective antibodies. With the advancements in bioinformatics, we can now predict quite reliably the presence of T or B cell epitopes, but that does not necessarily mean that these antigens will be immunogenic enough to be immunoreactive in the actual immunization trials. In addition, recent developments in vaccinology imply that researchers should also consider other alternative methods of vaccine delivery that do not require the production of recombinant antigens, including DNA and RNA vaccines.
Although live vaccines with or without the addition of genomic modifications had achieved promising results in several vaccine trials, they should be used with caution to avoid massive outbreaks in non-endemic areas populated with animals that were never exposed to such pathogens before. Also, there are still concerns regarding the application of GMOs in the vaccination field, fearing that genomic modifications may have unforeseeable consequences, either to the environment, in other unrelated commensal organisms, or in the animal genomes, with the subsequent impact on humans as well.
To test vaccine candidates and to get the relevant and valid results, researchers should be cautious with the route of pathogen challenge, either as an inoculum form, or by natural infection through the vector (ex. tick challenge). While the former one is considered as a fast way, the latter one acts in a more gradual way, representing a simulation of natural infection in the field.
Next,-Generation Technologies and Systems Biology is considered a modern molecular toolkit, and it is a leader guide for the future direction in vaccine candidate design. The traditional way to identify a novel antigen(s) is usually restricted to individual studies, an approach that can be considered as slow and limited. Sequencing technologies, bioinformatics, and statistics analysis facilitate the omics for lots of parasites. Omics such as genomics and transcriptomics have facilitated the functional annotation of the genome for many of these parasites, which significantly improved the understanding of the parasite biology, interactions with the host, as well as virulence and host immune response (146). To identify ideal vaccine component peptides or proteins, a comprehensive identification of the entire gene repertoire through genome, transcriptome, and proteome data mining, followed by the analysis of their encoding sequences using bioinformatics tools. The analysis should focus on in silico characteristics and assess both intra-species (from at least five genomes) and inter-species (among phylogenetically related organisms) conservation levels. Moreover, cutting-edge technologies such as gene editing by CRISPR-Cas9 have also allowed the discovery and functional characterization of potential novel vaccine antigens (146).
Finally, even if the vaccine candidates derived from omics technologies, computational approaches, or validated by using genetic manipulation approaches, they still need to be evaluated by clinical or field trial by animal experiments in order to test efficacy and safety. Therefore, systems vaccinology combined with experimental validation and evaluation in animal models through field trials can significantly improve the design of novel vaccines against blood parasites, opening a new era of vaccinology research that could lead to an expansion in licensed products after decades of slow advances (Figure 2).
In the Babesia bovis field, there are examples of the use of NGS technologies for the selection of vaccine candidates. The 6cys proteins and Hap2 protein were chosen as transmission blocking vaccine candidates after investigation of the genome annotation since these antigens were known to be involved in sexual stage development in other Babesia-related parasites, and then experimentally validated for Babesia parasites. Although the recombinant 6Cys proteins used in vaccination trials did not generate an effective transmission blocking response, a vaccine based on recombinant Hap2 was shown to be effective in blocking transmission of B. bovis (147), a finding that should be considered for the eventual production of a commercially available TBV against this parasite.
In conclusion, addressing the various challenges in vaccine development is of paramount importance. Recent advances in vaccine technology offer significant opportunities, particularly using multicomponent formulations that incorporate multiple antigens. Utilizing live or whole-cell immunogens, along with a combination of whole attenuated parasites and recombinant sexual stage antigens, generally enhances the effectiveness of vaccines in controlling blood parasite infections and their transmission by vectors. However, it is essential to proceed with caution. The inclusion of a greater number of antigenic subunits may lead to unfavorable three-dimensional structures, which could result in the loss of critical conformational epitopes. Therefore, while the multicomponent approach shows promise, careful attention must be paid to maintaining the structural integrity and immunogenicity of the antigens to ensure the development of an effective vaccine.
6 Recommendations
• Based on previous experiences, ideally, future research directions toward development of effective vaccines should involve the prevention of the acute disease, as well as prevention of parasite transmission.
• Sequence variations in vaccine target genes may result in vaccine failures. In order to achieve the goal to control blood parasites and its associated vectors using recombinant proteins, it is important to verify sequence conservation of the target antigens among distinct geographic isolates of the parasites, using, for instance, available sequence databases.
• It is likely that using a single antigen as a vaccine is disadvantageous to induce full protective immune responses. Thus, using multiple antigens as a multicomponent formulation of vital functions or using the live or whole-cell immunogens or a consolidation of the whole attenuated parasite in addition to recombinant sexual stage antigens, would be, in general, more effective vaccine to control blood parasites infection and its transmission by vectors.
• Finally, to ensure disease control and to reduce disease impact, it’s critical to have environmentally friendly vector control measures applied in addition to the use of safe vaccines, in the vector specific season.
Author contributions
HA: Conceptualization, Data curation, Investigation, Writing – original draft, Writing – review & editing. MM: Validation, Writing – review & editing. CS: Conceptualization, Data curation, Formal analysis, Funding acquisition, Resources, Visualization, Writing – review & editing.
Funding
The author(s) declare that financial support was received for the research, authorship, and/or publication of this article. This research was funded by the National Research Center, Cairo, Egypt, USDA National Institute of Food and Agriculture (NIFA) (Award Number: 2020-67015-31809; Proposal Number: 2019-05375, Accession Number: 1022541), and the United States Department of Agriculture (ARS-USDA CRIS 2090-32000-040-00-D).
Conflict of interest
The authors declare that the research was conducted in the absence of any commercial or financial relationships that could be construed as a potential conflict of interest.
Publisher’s note
All claims expressed in this article are solely those of the authors and do not necessarily represent those of their affiliated organizations, or those of the publisher, the editors and the reviewers. Any product that may be evaluated in this article, or claim that may be made by its manufacturer, is not guaranteed or endorsed by the publisher.
Footnotes
References
1. Uilenberg, G. Theileria species of domestic livestock In: AD Irvin, MP Cunningham, and AS Young, editors. Advances in the control of theileriosis: Proceedings of an international conference held at the International Laboratory for Research on animal diseases in Nairobi, 9–13th, 1981. Dordrecht: Springer Netherlands (1981). 4–37.
2. Jerzak, M, Gandurski, A, Tokaj, M, Stachera, W, Szuba, M, Dybicz, M, et al. Advances in Babesia vaccine development: an overview. Pathogens. (2023) 12:300. doi: 10.3390/pathogens12020300
3. Schnittger, L, Rodriguez, AE, Florin-Christensen, M, and Morrison, DA. Babesia: a world emerging. Infect Genet Evol. (2012) 12:1788–809. doi: 10.1016/j.meegid.2012.07.004
4. Spielman, A, Wilson, ML, Levine, JF, and Piesman, J. Ecology of Ixodes dammini-borne human babesiosis and Lyme disease. Annu Rev Entomol. (1985) 30:439–60. doi: 10.1146/annurev.en.30.010185.002255
6. Alzan, HF, Bastos, RG, Laughery, JM, Scoles, GA, Ueti, MW, Johnson, WC, et al. A culture-adapted strain of Babesia bovis has reduced subpopulation complexity and is unable to complete its natural life cycle in ticks. Front Cell Infect Microbiol. (2022) 12:827347. doi: 10.3389/fcimb.2022.827347
7. Bastos, RG, Laughery, JM, Ozubek, S, Alzan, HF, Taus, NS, Ueti, MW, et al. Identification of novel immune correlates of protection against acute bovine babesiosis by superinfecting cattle with in vitro culture attenuated and virulent Babesia bovis strains. Front Immunol. (2022) 13:1045608. doi: 10.3389/fimmu.2022.1045608
8. Bastos, RG, Capelli-Peixoto, J, Laughery, JM, Suarez, CE, and Ueti, MW. Vaccination with an in vitro culture attenuated Babesia bovis strain safely protects highly susceptible adult cattle against acute bovine babesiosis. Front Immunol. (2023) 14:1219913. doi: 10.3389/fimmu.2023.1219913
9. Homer, MJ, Aguilar-Delfin, I, Telford, SR, Krause, PJ, and Persing, DH. Babesiosis. Clin Microbiol Rev. (2000) 13:451–69. doi: 10.1128/CMR.13.3.451
10. Solano-Gallego, L, Sainz, Á, Roura, X, Estrada-Peña, A, and Miró, G. A review of canine babesiosis: the European perspective. Parasit Vectors. (2016) 9:1–18. doi: 10.1186/s13071-016-1596-0
11. Zahler, M, Rinder, H, Schein, E, and Gothe, R. Detection of a new pathogenic Babesia microti-like species in dogs. Vet Parasitol. (2000) 89:241–8. doi: 10.1016/S0304-4017(00)00202-8
12. García, ATC. Piroplasma infection in dogs in northern Spain. Vet Parasitol. (2006) 138:97–102. doi: 10.1016/j.vetpar.2006.01.043
13. Fukumoto, S, Suzuki, H, Igarashi, I, and Xuan, X. Fatal experimental transplacental Babesia gibsoni infections in dogs. Int J Parasitol. (2005) 35:1031–5. doi: 10.1016/j.ijpara.2005.03.018
14. Trotta, M, Carli, E, Novari, G, Furlanello, T, and Solano-Gallego, L. Clinicopathological findings, molecular detection and characterization of Babesia gibsoni infection in a sick dog from Italy. Vet Parasitol. (2009) 165:318–22. doi: 10.1016/j.vetpar.2009.07.022
15. Birkenheuer, AJ, Correa, MT, Levy, MG, and Breitschwerdt, EB. Geographic distribution of babesiosis among dogs in the United States and association with dog bites: 150 cases (2000-2003). J Am Vet Med Assoc. (2005) 227:942–7. doi: 10.2460/javma.2005.227.942
16. Mahmoud, MS, Kandil, OM, Abu El-Ezz, NT, Hendawy, SHM, Elsawy, BSM, Knowles, DP, et al. Identification and antigenicity of the Babesia caballi spherical body protein 4 (SBP4). Parasit Vectors. (2020) 13:1–10. doi: 10.1186/s13071-020-04241-9
17. Elsawy, BSM, Nassar, AM, Alzan, HF, Bhoora, RV, Ozubek, S, Mahmoud, MS, et al. Rapid detection of equine piroplasms using multiplex pcr and first genetic characterization of Theileria haneyi in Egypt. Pathogens. (2021) 10:1414. doi: 10.3390/pathogens10111414
18. Yeruham, I, Hadani, A, and Galker, F. Some epizootiological and clinical aspects of ovine babesiosis caused by Babesia ovis-a review. Vet Parasitol. (1998) 74:153–63. doi: 10.1016/S0304-4017(97)00143-X
19. Sevinc, F, Sevinc, M, Ekici, OD, Yildiz, R, Isik, N, and Aydogdu, U. Babesia ovis infections: detailed clinical and laboratory observations in the pre- and post-treatment periods of 97 field cases. Vet Parasitol. (2013) 191:35–43. doi: 10.1016/j.vetpar.2012.07.025
20. Bilgic, HB, Hacilarlioglu, S, Bakirci, S, Kose, O, Unlu, AH, Aksulu, A, et al. Comparison of protectiveness of recombinant Babesia ovis apical membrane antigen 1 and B. ovis-infected cell line as vaccines against ovine babesiosis. Ticks Tick Borne Dis. (2020) 11:101280. doi: 10.1016/j.ttbdis.2019.101280
21. Morrison, WI, Connelley, T, Hemmink, JD, and MacHugh, ND. Understanding the basis of parasite strain-restricted immunity to Theileria parva. Annu Rev Anim Biosci. (2015) 3:397–418. doi: 10.1146/annurev-animal-022513-114152
22. Ahmed, JS, and Mehlhorn, H. Review: the cellular basis of the immunity to and immunopathogenesis of tropical theileriosis. Parasitol Res. (1999) 85:539–49. doi: 10.1007/s004360050593
23. Florin-Christensen, M, Schnittger, L, Bastos, RG, Rathinasamy, VA, Cooke, BM, Alzan, HF, et al. Pursuing effective vaccines against cattle diseases caused by apicomplexan protozoa. CAB reviews: perspectives in agriculture, veterinary science, nutrition and natural. Resources. (2021) 16:6024. doi: 10.1079/PAVSNNR202116024
24. Al-Hosary, AA, ElSify, A, Salama, AA, Nayel, M, Elkhtam, A, Elmajdoub, LO, et al. Phylogenetic study of Theileria ovis and Theileria lestoquardi in sheep from Egypt: molecular evidence and genetic characterization. Vet World. (2021) 14:634–9. doi: 10.14202/vetworld.2021.634-639
25. Agina, OA, Shaari, MR, Isa, NMM, Ajat, M, Zamri-Saad, M, and Hamzah, H. Clinical pathology, immunopathology and advanced vaccine Technology in Bovine Theileriosis: A review. Pathogens. (2020) 9:1–22. doi: 10.3390/pathogens9090697
26. Onyiche, TE, Suganuma, K, Igarashi, I, Yokoyama, N, Xuan, X, and Thekisoe, O. A review on equine Piroplasmosis: epidemiology, vector ecology, risk factors, host immunity, diagnosis and control. Int J Environ Res Public Health. (2019) 16:736. doi: 10.3390/ijerph16101736
27. Ramsay, JD, Ueti, MW, Johnson, WC, Scoles, GA, Knowles, DP, and Mealey, RH. Lymphocytes and macrophages are infected by Theileria equi, but T cells and B cells are not required to establish infection in vivo. PLoS One. (2013) 8:e76996. doi: 10.1371/journal.pone.0076996
28. Kawamoto, S, Takahashi, K, Kurosawa, T, Sonoda, M, and Onuma, M. Intraerythrocytic schizogony of Theileria sergenti in cattle. Nihon Juigaku Zasshi. (1990) 52:1251–9. doi: 10.1292/jvms1939.52.1251
29. Cook, RA, and Karesh, WB. Emerging diseases at the Interface of people, domestic animals, and wildlife. Fowler’s Zoo Wild Anim Med. (2012) 7:136. doi: 10.1016/B978-1-4377-1986-4.00018-4
30. Bernard, Q, Helezen, E, and Boulanger, N. Tick-borne Bacteria and host skin Interface. Skin and Arthropod Vectors. (2018):293–324. doi: 10.1016/B978-0-12-811436-0.00009-5
31. Nicholson, WL, Sonenshine, DE, Noden, BH, and Brown, RN. Ticks (ixodida). Med Vet Entomol. (2018):603–72. doi: 10.1016/B978-0-12-814043-7.00027-3
32. Mahmoud, HYAH, Ali, AO, and Tanaka, T. Molecular detection and characterization of Anaplasma marginale infecting cattle, buffalo, and camel populations in southern Egypt. Front Vet Sci. (2023) 10:1169323. doi: 10.3389/fvets.2023.1169323
33. Li, H, Zheng, YC, Ma, L, Jia, N, Jiang, BG, Jiang, RR, et al. Human infection with a novel tick-borne Anaplasma species in China: a surveillance study. Lancet Infect Dis. (2015) 15:663–70. doi: 10.1016/S1473-3099(15)70051-4
34. Naeem, M, Amaro-Estrada, I, Taqadus, A, Swelum, AA, Alqhtani, AH, Asif, M, et al. Molecular prevalence and associated risk factors of Anaplasma ovis in Pakistani sheep. Front Vet Sci. (2023) 10:10. doi: 10.3389/fvets.2023.1096418
35. Sainz, Á, Roura, X, Miró, G, Estrada-Peña, A, Kohn, B, Harrus, S, et al. Guideline for veterinary practitioners on canine ehrlichiosis and anaplasmosis in Europe. Parasit Vectors. (2015) 8:75. doi: 10.1186/s13071-015-0649-0
36. Groen, J, Koraka, P, Nur, Y, Avsic-Zupanc, T, Goessens, W, Ott, A, et al. Serologic evidence of ehrlichiosis among humans and wild animals in the Netherlands. Eur J Clin Microbiol Infect Dis. (2002) 21:46–9. doi: 10.1007/s10096-001-0659-z
37. Nicholson, WL, Allen, KE, McQuiston, JH, Breitschwerdt, EB, and Little, SE. The increasing recognition of rickettsial pathogens in dogs and people. Trends Parasitol. (2010) 26:205–12. doi: 10.1016/j.pt.2010.01.007
38. Atif, FA. Alpha proteobacteria of genus Anaplasma (Rickettsiales: Anaplasmataceae): epidemiology and characteristics of Anaplasma species related to veterinary and public health importance. Parasitology. (2016) 143:659–85. doi: 10.1017/S0031182016000238
39. Atif, FA, Mehnaz, S, Qamar, MF, Roheen, T, Sajid, MS, Ehtisham-Ul-haque, S, et al. Epidemiology, diagnosis, and control of canine infectious cyclic thrombocytopenia and granulocytic Anaplasmosis: emerging diseases of veterinary and public health significance. Vet Sci. (2021) 8:312. doi: 10.3390/vetsci8120312
41. Yaro, M, Munyard, KA, Stear, MJ, and Groth, DM. Combatting African animal trypanosomiasis (AAT) in livestock: the potential role of trypanotolerance. Vet Parasitol. (2016) 225:43–52. doi: 10.1016/j.vetpar.2016.05.003
42. Selim, A, Alafari, HA, Attia, K, AlKahtani, MDF, Albohairy, FM, and Elsohaby, I. Prevalence and animal level risk factors associated with Trypanosoma evansi infection in dromedary camels. Sci Rep. (2022) 12:8933. doi: 10.1038/s41598-022-12817-x
43. Barrett, ADT, and Stanberry, LR. Vaccines for Biodefense and Emerging and Neglected Diseases. New York: Academic Press (2014).
44. Algehani, AMG, Jaber, FA, Khan, A, and Alsulami, MN. Review on trypanosomiasis and their prevalence in some country on the Red Sea. Braz J Biol. (2021) 83:83. doi: 10.1590/1519-6984.251671
45. Gillingwater, K, Kumar, A, Anbazhagan, M, Boykin, DW, Tidwell, RR, and Brun, R. In vivo investigations of selected Diamidine compounds against Trypanosoma evansi using a mouse model. Antimicrob Agents Chemother. (2009) 53:5074–9. doi: 10.1128/AAC.00422-09
46. Faye, D, Pereira de Almeida, PJL, Goossens, B, Osaer, S, Ndao, M, Berkvens, D, et al. Prevalence and incidence of trypanosomosis in horses and donkeys in the Gambia. Vet Parasitol. (2001) 101:101–14. doi: 10.1016/S0304-4017(01)00503-9
47. Behour, TS, and Abd EL Fattah, EM. Genotyping of Trypanosoma brucei evansi in Egyptian camels: detection of a different non-RoTat 1.2 Trypanosoma brucei evansi in Egyptian camels. Trop Anim Health Prod. (2023) 55:1–10. doi: 10.1007/s11250-023-03673-6
48. Holland, WG, Do, TT, Huong, NT, Dung, NT, Thanh, NG, Vercruysse, J, et al. The effect of Trypanosoma evansi infection on pig performance and vaccination against classical swine fever. Vet Parasitol. (2003) 111:115–23. doi: 10.1016/S0304-4017(02)00363-1
49. El-Bahnasawy, MMM, Khater, MK, and Morsy, TA. African trypanosomiasis with special reference to Egyptian Trypanosoma evansi: is it a neglected zoonosis? J Egypt Soc Parasitol. (2014) 44:741–8. doi: 10.21608/jesp.2014.90371
50. Alfaleh, FA, and Elhaig, MM. Molecular prevalence, associated risk factors and genetic characterization of Trypanosoma evansi in camels. Microb Pathog. (2023) 175:105967. doi: 10.1016/j.micpath.2022.105967
51. Alzan, HF, Lau, AOT, Knowles, DP, Herndon, DR, Ueti, MW, Scoles, GA, et al. Expression of 6-Cys gene superfamily defines Babesia bovis sexual stage development within Rhipicephalus microplus. PLoS One. (2016) 11:e0163791. doi: 10.1371/journal.pone.0163791
52. Alzan, HF, Bastos, RG, Ueti, MW, Laughery, JM, Rathinasamy, VA, Cooke, BM, et al. Assessment of Babesia bovis 6cys A and 6cys B as components of transmission blocking vaccines for babesiosis. Parasites Vectors. (2021) 14:210. doi: 10.1186/s13071-021-04712-7
53. De Castro, JJ. Sustainable tick and tickborne disease control in livestock improvement in developing countries. Vet Parasitol. (1997) 71:77–97. doi: 10.1016/S0304-4017(97)00033-2
54. Standfast, NF, Bock, RE, Wiecek, MM, DeVos, AJ, Jorgensen, WK, and Kingston, TG. Overcoming constraints to meeting increased demand for Babesia bigemina vaccine in Australia. Vet Parasitol. (2003) 115:213–22. doi: 10.1016/S0304-4017(03)00223-1
55. Schetters, T. Vaccination against canine babesiosis. Trends Parasitol. (2005) 21:179–84. doi: 10.1016/j.pt.2005.02.006
56. Vidor, E, Bissuel, G, and Dubreuil, N. Vaccination against canine babesiosis: an overview of field observations. Trans R Soc Trop Med Hyg. (1989) 83:95–6. doi: 10.1016/0035-9203(89)90611-1
57. Fukumoto, S, Tamaki, Y, Okamura, M, Bannai, H, Yokoyama, N, Suzuki, T, et al. Prime-boost immunization with DNA followed by a recombinant vaccinia virus expressing P50 induced protective immunity against Babesia gibsoni infection in dogs. Vaccine. (2007) 25:1334–41. doi: 10.1016/j.vaccine.2006.09.083
58. Fukumoto, S, Tamaki, Y, Igarashi, I, Suzuki, H, and Xuan, X. Immunogenicity and growth inhibitory efficacy of the prime–boost immunization regime with DNA followed by recombinant vaccinia virus carrying the P29 gene of Babesia gibsoni in dogs. Exp Parasitol. (2009) 123:296–301. doi: 10.1016/j.exppara.2009.08.012
59. Vercammen, F, De Deken, R, and Maes, L. Prophylactic treatment of experimental canine babesiosis (Babesia canis) with doxycycline. Vet Parasitol. (1996) 66:251–5. doi: 10.1016/S0304-4017(96)01016-3
60. Vercammen, F, De Deken, R, and Maes, L. Duration of protective immunity in experimental canine babesiosis after homologous and heterologous challenge. Vet Parasitol. (1997) 68:51–5. doi: 10.1016/S0304-4017(96)01063-1
61. WHO. (2024). Interim recommendations for the use of protein subunit COVID-19 vaccines. Available online at: https://www.who.int/publications/i/item/WHO-2019-nCoV-vaccines-SAGE_recommendation-protein_subunit-2023.1
62. Taus, NS, Cywes-Bentley, C, Johnson, WC, Pier, GB, Fry, LM, Mousel, MR, et al. Immunization against a conserved surface polysaccharide stimulates bovine antibodies with opsonic killing activity but does not protect against Babesia bovis challenge. Pathogens. (2021) 10:598. doi: 10.3390/pathogens10121598
63. Clark, TG, and Cassidy-Hanley, D. Recombinant subunit vaccines: potentials and constraints. Dev Biol (Basel). (2005) 121:153–63.
64. McElwain, TF, Perryman, LE, Musoke, AJ, and McGuire, TC. Molecular characterization and immunogenicity of neutralization-sensitive Babesia bigemina merozoite surface proteins. Mol Biochem Parasitol. (1991) 47:213–22. doi: 10.1016/0166-6851(91)90181-5
65. Cuy-Chaparro, L, Reyes, C, Díaz-Guiot, EV, Moreno-Pérez, DA, and Patarroyo, MA. Developing anti- Babesia bovis blood stage vaccines: A new perspective regarding synthetic vaccines. Int J Mol Sci. (2023) 24:5219. doi: 10.3390/ijms24065219
66. Patarroyo, ME, Arevalo-Pinzon, G, Reyes, C, Moreno-Vranich, A, and Patarroyo, MA. Malaria parasite survival depends on conserved binding peptides. Curr Issues Mol Biol. (2016) 18:57–78.
67. Rojas, MJ, Bastos, RG, Navas, J, Laughery, JM, Lacy, PA, and Suarez, CE. A conserved motif in the immune-subdominant RAP-1 related antigen of Babesia bovis contains a B-cell epitope recognized by antibodies from protected cattle. Front Immunol. (2024) 15:1380660. doi: 10.3389/fimmu.2024.1380660
68. Zhang, W, Jiang, X, Bao, J, Wang, Y, Liu, H, and Tang, L. Exosomes in pathogen infections: A bridge to deliver molecules and link functions. Front Immunol. (2018) 9:90. doi: 10.3389/fimmu.2018.00090
69. Marcilla, A, Martin-Jaular, L, Trelis, M, de Menezes-Neto, A, Osuna, A, Bernal, D, et al. Extracellular vesicles in parasitic diseases. J Extracell Vesicles. (2014) 3:10.3402. doi: 10.3402/jev.v3.25040
70. Martín-Jaular, L, de Menezes-Neto, A, Monguió-Tortajada, M, Elizalde-Torrent, A, Díaz-Varela, M, Fernández-Becerra, C, et al. Spleen-dependent immune protection elicited by CpG Adjuvanted reticulocyte-derived exosomes from malaria infection is associated with changes in T cell subsets’ distribution. Front Cell Dev Biol. (2016) 4:131. doi: 10.3389/fcell.2016.00131
71. Beri, D, Rodriguez, M, Singh, M, Liu, Y, Rasquinha, G, An, X, et al. Identification and characterization of extracellular vesicles from red cells infected with Babesia divergens and Babesia microti. Front Cell Infect Microbiol. (2022) 12:962944. doi: 10.3389/fcimb.2022.962944
72. Good, MF, and Stanisic, DI. Whole parasite vaccines for the asexual blood stages of plasmodium. Immunol Rev. (2020) 293:270–82. doi: 10.1111/imr.12819
73. Mahoney, DF. Bovine babesiosis: the immunization of cattle with killed Babesia argentina. Exp Parasitol. (1967) 20:125–9. doi: 10.1016/0014-4894(67)90030-6
74. Al-Nazal, HA, Cooper, E, Ho, MF, Eskandari, S, Majam, V, Giddam, AK, et al. Pre-clinical evaluation of a whole-parasite vaccine to control human babesiosis. Cell Host Microbe. (2021) 29:894–903.e5. doi: 10.1016/j.chom.2021.04.008
75. De Vos, AJ, and Bock, RE. Vaccination against bovine babesiosis. Ann N Y Acad Sci. (2000) 916:540–5. doi: 10.1111/j.1749-6632.2000.tb05333.x
76. de Waal, DT, and Combrink, MP. Live vaccines against bovine babesiosis. Vet Parasitol. (2006) 138:88–96. doi: 10.1016/j.vetpar.2006.01.042
77. Dalgliesh, RJ, Callow, LL, Mellors, LT, and McGregor, W. Development of a highly infective Babesia bigemina vaccine of reduced virulence. Aust Vet J. (1981) 57:8–11. doi: 10.1111/j.1751-0813.1981.tb07075.x
78. Jaramillo Ortiz, JM, Del Médico Zajac, MP, Zanetti, FA, Molinari, MP, Gravisaco, MJ, Calamante, G, et al. Vaccine strategies against Babesia bovis based on prime-boost immunizations in mice with modified vaccinia Ankara vector and recombinant proteins. Vaccine. (2014) 32:4625–32. doi: 10.1016/j.vaccine.2014.06.075
79. Rojas, C, Figueroa, JV, Alvarado, A, Mejia, P, Mosqueda, JJ, Falcon, A, et al. Bovine babesiosis live vaccine production: use of gamma irradiation on the substrate. Ann N Y Acad Sci. (2006) 1081:405–16. doi: 10.1196/annals.1373.059
80. Alvarez, JA, Rojas, C, and Figueroa, JV. An overview of current knowledge on in vitro Babesia cultivation for production of live attenuated vaccines for bovine Babesiosis in Mexico. Front Vet Sci. (2020) 7:364. doi: 10.3389/fvets.2020.00364
81. Nene, V, and Morrison, WI. Approaches to vaccination against Theileria parva and Theileria annulata. Parasite Immunol. (2016) 38:724–34. doi: 10.1111/pim.12388
82. Sears, K, Knowles, D, Dinkel, K, Mshelia, PW, Onzere, C, Silva, M, et al. Imidocarb Dipropionate lacks efficacy against Theileria haneyi and fails to consistently clear Theileria equi in horses co-infected with T. Haneyi. Pathogens. (2020) 9:1–12. doi: 10.3390/pathogens9121035
83. Gwakisa, P, Kindoro, F, Mwega, E, Kimera, S, Obara, I, Ahmed, J, et al. Monitoring vaccinated cattle for induction and longevity of persistent tick-transmissible infection: implications for wider deployment of live vaccination against East Coast fever in Tanzania. Transbound Emerg Dis. (2020) 67:79–87. doi: 10.1111/tbed.13405
84. Sharifiyazdi, H, Namazi, F, Oryan, A, Shahriari, R, and Razavi, M. Point mutations in the Theileria annulata cytochrome b gene is associated with buparvaquone treatment failure. Vet Parasitol. (2012) 187:431–5. doi: 10.1016/j.vetpar.2012.01.016
85. Hashemi-Fesharaki, R. Control of Theileria annulata in Iran. Parasitol Today. (1988) 4:36–40. doi: 10.1016/0169-4758(88)90062-2
86. Pipano, E. Vaccination against Theileria annulata theileriosis In: IG Wright, editor. Veterinary protozoan and Hemoparasitic vaccines. Boca Raton, FL: CRC Press (1989). 203–34.
87. WOAH Terrestrial Manual. (2024). Theileriosis in cattle (infection with Theileria annulata, T orientalis and T parva Chapter 3.4.13).
88. al-Hosary, A, Radwan, AM, Ahmed, LS, Abdelghaffar, SK, Fischer, S, Nijhof, AM, et al. Isolation and propagation of an Egyptian Theileria annulata infected cell line and evaluation of its use as a vaccine to protect cattle against field challenge. Sci Rep. (2024) 14:8565–11. doi: 10.1038/s41598-024-57325-2
89. Morrison, WI, and McKeever, DJ. Current status of vaccine development against Theileria parasites. Parasitology. (2006) 133:S169–87. doi: 10.1017/S0031182006001867
90. Kachani, M, El-Haj, N, Kahouache,, and Ouhelli, H. Vaccin vivant contre la theileriose bovine constitué par des macroschizonte de Theileria annulata: innocuité, durée de l’immunité et absence de portage. Revue Méd Vet. (2004) 155:624–31.
91. Muñoz Vianna, A, Paula, A, Stori De Lara, S, Borges Weege, G, Cunha, RC, Pereira, F, et al. Equine Theileriosis: Review Review Article. Ann Rev Resear. (2018) 3
92. Salinas-Estrella, E, Amaro-Estrada, I, Cobaxin-Cárdenas, ME, Preciado de la Torre, JF, and Rodríguez, SD. Bovine Anaplasmosis: will there ever be an almighty effective vaccine? Front Vet Sci. (2022) 9:946545. doi: 10.3389/fvets.2022.946545
93. Rudoler, N, Baneth, G, Eyal, O, van Straten, M, and Harrus, S. Evaluation of an attenuated strain of Ehrlichia canis as a vaccine for canine monocytic ehrlichiosis. Vaccine. (2012) 31:226–33. doi: 10.1016/j.vaccine.2012.10.003
94. Sarli, M, Novoa, MB, Mazzucco, MN, Signorini, ML, Echaide, IE, de Echaide, ST, et al. A vaccine using Anaplasma marginale subdominant type IV secretion system recombinant proteins was not protective against a virulent challenge. PLoS One. (2020) 15:e0229301. doi: 10.1371/journal.pone.0229301
95. Miraballes, C, Aráoz, V, and Riet-Correa, F. Rhipicephalus microplus, babesiosis and anaplasmosis in Uruguay: current situation and control or elimination programs on farms. Exp Appl Acarol. (2019) 78:579–93. doi: 10.1007/s10493-019-00405-0
96. Carelli, G, Decaro, N, Lorusso, E, Paradies, P, Elia, G, Martella, V, et al. First report of bovine anaplasmosis caused by Anaplasma centrale in Europe. Ann N Y Acad Sci. (2008) 1149:107–10. doi: 10.1196/annals.1428.069
97. Contact PR Newswire. (2024). Clinically effective A. marginale bovine vaccine makes international research news, causes global excitement for cattle ranchers currently battling Anaplasmosis epidemic. Available online at: https://www.prnewswire.com/news-releases/clinically-effective-a-marginale-bovine-vaccine-makes-international-research-news-causes-global-excitement-for-cattle-ranchers-currently-battling-anaplasmosis-epidemic-301558920.html
98. PR Newswire. (2024). University products offers affordable Anaplasmosis vaccines ahead of spring calving season, amidst FDA antibiotic crackdowns. Available online at: https://www.prnewswire.com/news-releases/university-products-offers-affordable-anaplasmosis-vaccines-ahead-of-spring-calving-season-amidst-fda-antibiotic-crackdowns-302068806.html
99. Waghela, SD, Cruz, D, Droleskey, RE, DeLoach, JR, and Wagner, GG. In vitro cultivation of Anaplasma marginale in bovine erythrocytes co-cultured with endothelial cells. Vet Parasitol. (1997) 73:43–52. doi: 10.1016/S0304-4017(97)00045-9
100. Lis, K, Fernández de Mera, IG, Popara, M, Cabezas-Cruz, A, Ayllón, N, Zweygarth, E, et al. Molecular and immunological characterization of three strains of Anaplasma marginale grown in cultured tick cells. Ticks Tick Borne Dis. (2015) 6:522–9. doi: 10.1016/j.ttbdis.2015.04.006
101. Lasmar, PVF, de Carvalho, AÚ, Filho, EJF, Bastos, CV, and Ribeiro, MFB. Evaluating the effectiveness of an inactivated vaccine from Anaplasma marginale derived from tick cell culture. Rev Bras Parasitol Vet. (2012) 21:112–7. doi: 10.1590/S1984-29612012000200008
102. Bell-Sakyi, L, Palomar, AM, Bradford, EL, and Shkap, V. Propagation of the Israeli vaccine strain of Anaplasma centrale in tick cell lines. Vet Microbiol. (2015) 179:270–6. doi: 10.1016/j.vetmic.2015.07.008
103. Amaro-Estrada, I, Rodríguez-Camarillo, SD, Amaro-Estrada, I, and Rodríguez-Camarillo, SD. (2018). Genome-based vaccinology applied to bovine Anaplasmosis. Farm Animals Diseases, Recent Omic Trends and New Strategies of Treatment.
104. Dark, MJ, Al-Khedery, B, and Barbet, AF. Multistrain genome analysis identifies candidate vaccine antigens of Anaplasma marginale. Vaccine. (2011) 29:4923–32. doi: 10.1016/j.vaccine.2011.04.131
105. Silvestre, BT, Da Silveira, JAG, Facury-Filho, EJ, De Carvalho, AÚ, Versiani, AF, Estevam, LGTM, et al. Immune response and biochemistry of calves immunized with rMSP1a (Anaplasma marginale) using carbon nanotubes as carrier molecules. Rev Bras Parasitol Vet. (2018) 27:191–202. doi: 10.1590/s1984-296120180029
106. Al-Khedery, B, Lundgren, AM, Stuen, S, Granquist, EG, Munderloh, UG, Nelson, CM, et al. Structure of the type IV secretion system in different strains of Anaplasma phagocytophilum. BMC Genomics. (2012) 13:1–15. doi: 10.1186/1471-2164-13-678
107. Waksman, G. From conjugation to T4S systems in gram-negative bacteria: a mechanistic biology perspective. EMBO Rep. (2019) 20:e47012. doi: 10.15252/embr.201847012
108. de la Fuente, J, Moraga-Fernández, A, Alberdi, P, Díaz-Sánchez, S, García-Álvarez, O, Fernández-Melgar, R, et al. A quantum Vaccinomics approach for the design and production of MSP4 chimeric antigen for the control of Anaplasma phagocytophilum infections. Vaccines. (2022) 10:1995. doi: 10.3390/vaccines10121995
109. Crosby, FL, Wamsley, HL, Pate, MG, Lundgren, AM, Noh, SM, Munderloh, UG, et al. Knockout of an outer membrane protein operon of Anaplasma marginale by transposon mutagenesis. BMC Genomics. (2014) 15:278. doi: 10.1186/1471-2164-15-278
110. Crosby, FL, Brayton, KA, Magunda, F, Munderloh, UG, Kelley, KL, and Barbet, AF. Reduced infectivity in cattle for an outer membrane protein mutant of Anaplasma marginale. Appl Environ Microbiol. (2015) 81:2206–14. doi: 10.1128/AEM.03241-14
111. Chandra, S, Smith, K, Alanazi, AD, Alyousif, MS, Emery, D, and Šlapeta, J. Rhipicephalus sanguineus sensu lato from dogs and dromedary camels in Riyadh, Saudi Arabia: low prevalence of vector-borne pathogens in dogs detected using multiplexed tandem PCR panel. Folia Parasitol (Praha). (2019) 66:66. doi: 10.14411/fp.2019.007
112. Stuen, S, Granquist, EG, and Silaghi, C. Anaplasma phagocytophilum a widespread multi-host pathogen with highly adaptive strategies. Front Cell Infect Microbiol. (2013) 3:31. doi: 10.3389/fcimb.2013.00031
113. Nelson, CM, Herron, MJ, Felsheim, RF, Schloeder, BR, Grindle, SM, Chavez, AO, et al. Whole genome transcription profiling of Anaplasma phagocytophilum in human and tick host cells by tiling array analysis. BMC Genomics. (2008) 9:364. doi: 10.1186/1471-2164-9-364
114. Ojogun, N, Kahlon, A, Ragland, SA, Troese, MJ, Mastronunzio, JE, Walker, NJ, et al. Anaplasma phagocytophilum outer membrane protein A interacts with Sialylated glycoproteins to promote infection of mammalian host cells. Infect Immun. (2012) 80:3748–60. doi: 10.1128/IAI.00654-12
115. Kahlon, A, Ojogun, N, Ragland, SA, Seidman, D, Troese, MJ, Ottens, AK, et al. Anaplasma phagocytophilum Asp14 is an Invasin that interacts with mammalian host cells via its C terminus to facilitate infection. Infect Immun. (2013) 81:65–79. doi: 10.1128/IAI.00932-12
116. Seidman, D, Ojogun, N, Walker, NJ, Mastronunzio, J, Kahlon, A, Hebert, KS, et al. Anaplasma phagocytophilum surface protein AipA mediates invasion of mammalian host cells. Cell Microbiol. (2014) 16:1133–45. doi: 10.1111/cmi.12286
117. He, M, Xu, W, Zhang, L, Liu, Z, Zhu, J, Li, Y, et al. Identification of novel immunoreactive proteins and delineation of a specific epitope of Anaplasma phagocytophilum. Microb Pathog. (2018) 125:183–8. doi: 10.1016/j.micpath.2018.09.017
118. Ahmed, SK, Rahman, AH, Hassan, MA, Salih, SEM, Paone, M, and Cecchi, G. An atlas of tsetse and bovine trypanosomosis in Sudan. Parasit Vectors. (2016) 9:1–8. doi: 10.1186/s13071-016-1485-6
120. Nuryady, MM, Widayanti, R, Nurcahyo, RW, Fadjrinatha, B, and Fahrurrozi, ZSA. Characterization and phylogenetic analysis of multidrug-resistant protein - encoding genes in Trypanosoma evansi isolated from buffaloes in Ngawi district, Indonesia. Vet World. (2019) 12:1573–7. doi: 10.14202/vetworld.2019.1573-1577
121. Behour, T, Aboelhadid, S, and Mousa, W. Molecular analysis and histopathological alterations during the evolution of Trypanosoma evansi infection in experimentally infected mice original. Egyptian Vet Med Soc Parasitol J. (2015) 11:1–11. doi: 10.21608/evmspj.2015.37896
122. Okello, WO, MacLeod, ET, Muhanguzi, D, Waiswa, C, and Welburn, SC. Controlling tsetse flies and ticks using insecticide treatment of cattle in Tororo District Uganda: cost benefit analysis. Front Vet Sci. (2021) 8:8. doi: 10.3389/fvets.2021.616865
123. La, GF, and Magez, S. Vaccination against trypanosomiasis: can it be done or is the trypanosome truly the ultimate immune destroyer and escape artist? Hum Vaccin. (2011) 7:1225–33. doi: 10.4161/hv.7.11.18203
124. Black, SJ, and Mansfield, JM. Prospects for vaccination against pathogenic African trypanosomes. Parasite Immunol. (2016) 38:735–43. doi: 10.1111/pim.12387
125. Munir, F, ZuD, S, Tayyeb, M, Abbas, R, Shakoor, A, AMA, K, et al. Rationale to Develop mRNA-based Vaccines for Trypanosoma brucei (a review). Continen Vet J. (2023) 3:26–35.
126. Autheman, D, Crosnier, C, Clare, S, Goulding, DA, Brandt, C, Harcourt, K, et al. An invariant Trypanosoma vivax vaccine antigen inducing protective immunity. bioRxiv. (2021) doi: 10.1038/s41586-021-03597-x
127. ScienceDaily. (2024). Vaccine target for devastating livestock disease could change lives of millions. Available online at: https://www.sciencedaily.com/releases/2021/05/210527112450.htm
128. Danazumi, AU, Iliyasu Gital, S, Idris, S, Bs Dibba, L, Balogun, EO, and Górna, MW. Immunoinformatic design of a putative multi-epitope vaccine candidate against Trypanosoma brucei gambiense. Comput Struct. Biotechnol J. (2022) 20:5574–85. doi: 10.1016/j.csbj.2022.10.002
129. Jha, BK, Varikuti, S, Verma, C, Shivahare, R, Bishop, N, Dos Santos, GP, et al. Immunization with a Trypanosoma cruzi cyclophilin-19 deletion mutant protects against acute Chagas disease in mice. npj Vaccines. (2023) 8:1–15. doi: 10.1038/s41541-023-00647-5
130. Ali, A, Fernando Parizi, L, Garcia Guizzo, M, Tirloni, L, Seixas, A, Silva Vaz, I Jr, et al. Immunoprotective potential of a Rhipicephalus (Boophilus) microplus metalloprotease. Vet Parasitol. (2015) 207:107–14. doi: 10.1016/j.vetpar.2014.11.007
131. Rodríguez, M, Rubiera, R, Penichet, M, Montesinos, R, Cremata, J, Falcón, V, et al. High-level expression of the B. microplus Bm86 antigen in the yeast Pichia pastoris forming highly immunogenic particles for cattle. J Biotechnol. (1994) 33:135–46. doi: 10.1016/0168-1656(94)90106-6
132. Willadsen, P, Bird, P, Cobon, GS, and Hungerford, J. Commercialization of a recombinant vaccine against Boophilus microplus. Parasitology. (1995) 110 Suppl:S43–50. doi: 10.1017/S0031182000001487
133. Mulenga, A, Sugimoto, C, and Onuma, M. Issues in tick vaccine development: identification and characterization of potential candidate vaccine antigens. Microbes Infect. (2000) 2:1353–61. doi: 10.1016/S1286-4579(00)01289-2
134. De La Fuente, J, Rodríguez, M, Redondo, M, Montero, C, García-García, JC, Méndez, L, et al. Field studies and cost-effectiveness analysis of vaccination with Gavac (TM) against the cattle tick Boophilus microplus. Vaccine. (1998) 16:366–73. doi: 10.1016/S0264-410X(97)00208-9
135. Willadsen, P. Antigen cocktails: valid hypothesis or unsubstantiated hope? Trends Parasitol. (2008) 24:164–7. doi: 10.1016/j.pt.2008.01.005
136. de la Fuente, J, Kocan, KM, Almazán, C, and Blouin, EF. RNA interference for the study and genetic manipulation of ticks. Trends Parasitol. (2007) 23:427–33. doi: 10.1016/j.pt.2007.07.002
137. Elsawy, BSM, Mahmoud, MS, Suarez, CE, and Alzan, HF. Impact of equine and camel Piroplasmosis in Egypt: how much Do we know about the current situation? Pathogens. (2023) 12:1318. doi: 10.3390/pathogens12111318
138. Nepveu-Traversy, ME, Fausther-Bovendo, H, and Babuadze, G. Human tick-borne diseases and advances in anti-tick vaccine approaches: A comprehensive review. Vaccine. (2024) 12:141. doi: 10.3390/vaccines12020141
139. Tabor, AE. A review of Australian tick vaccine research. Vaccines. (2021) 9:1030. doi: 10.3390/vaccines9091030
140. Hammer, T, Gerhardts, A, and Hoefer, D. A fast test system to evaluate the physical protection potential of textiles against blood-sucking insects. Journal of. Text Sci Technol. (2018) 4:79–84. doi: 10.4236/jtst.2018.43005
141. Cook, D. A historical review of management options used against the stable Fly (Diptera: Muscidae). Insects. (2020) 11:313. doi: 10.3390/insects11050313
142. Benelli, G, Jeffries, CL, and Walker, T. Biological control of mosquito vectors: past, present, and future. Insects. (2016) 7:52. doi: 10.3390/insects7040052
143. Abdel-Ghany, HSM, Alzan, HF, Hendawy, SHM, Elsadawy, HA, and Abdel-Shafy, S. In vitro evaluation of the effect of entomopathogenic nematodes on different developmental stages of the brown dog tick Rhipicephalus sanguineus (Acari: Ixodidae). Acarologia. (2024) 64:323–34. doi: 10.24349/1hyi-f1vq
144. Slavica, V. The role of protists, nematodes and mites as natural control agents of sandfly populations. Front Trop Dis. (2024) 5:1369007. doi: 10.3389/fitd.2024.1369007
145. Belluco, S, Bertola, M, Montarsi, F, Di Martino, G, Granato, A, Stella, R, et al. Insects and public health: An overview. Insects. (2023) 14:240. doi: 10.3390/insects14030240
146. Tomazic, ML, Marugan-Hernandez, V, and Rodriguez, AE. Next-generation technologies and systems biology for the Design of Novel Vaccines against Apicomplexan Parasites. Front Vet Sci. (2022) 8:800361. doi: 10.3389/fvets.2021.800361
147. Silva, MG, Bastos, RG, Laughery, JM, Alzan, HF, Rathinasamy, VA, Cooke, BM, et al. Vaccination of cattle with the Babesia bovis sexual-stage protein HAP2 abrogates parasite transmission by Rhipicephalus microplus ticks. npj Vaccines. (2023) 8:1–9. doi: 10.1038/s41541-023-00741-8
148. Wright, IG, Casu, R, Commins, MA, Dalrymple, BP, Gale, KR, Goodger, BV, et al. The development of a recombinant Babesia vaccine. Vet Parasitol. (1992) 44:3–13. doi: 10.1016/0304-4017(92)90138-Y
149. Hope, M, Riding, G, Menzies, M, Colditz, I, Reverter, A, and Willadsen, P. Potential for recombinant Babesia bovis antigens to protect against a highly virulent isolate. Parasite Immunol. (2005) 27:439–45. doi: 10.1111/j.1365-3024.2005.00795.x
150. Alabay, M, Duzgun, A, Cerci, H, Wright, IG, Waltisbuhl, DJ, and Goodger, BV. Ovine babesiosis: induction of a protective immune response with crude extracts of either Babesia bovis or B. ovis. Res Vet Sci. (1987) 43:401–2. doi: 10.1016/S0034-5288(18)30812-9
151. Montenegro-James, S, Toro, M, Leon, E, and Guillen, AT. Field evaluation of an exoantigen-containing Babesia vaccine in Venezuela. Mem Inst Oswaldo Cruz. (1992) 87:283–8. doi: 10.1590/S0074-02761992000700048
152. Brown, WC, McElwain, TF, Ruef, BJ, Suarez, CE, Shkap, VA, Chitko-McKown, CG, et al. Babesia bovis rhoptry-associated protein 1 is immunodominant for T helper cells of immune cattle and contains T-cell epitopes conserved among geographically distant B. bovis strains. Infect Immun. (1996) 64:3341–50. doi: 10.1128/iai.64.8.3341-3350.1996
153. Norimine, J, Suarez, CE, McElwain, TF, Florin-Christensen, M, and Brown, WC. Immunodominant epitopes in Babesia bovis rhoptry-associated protein 1 that elicit memory CD4(+)-T-lymphocyte responses in B. bovis-immune individuals are located in the amino-terminal domain. Infect Immun. (2002) 70:2039–48. doi: 10.1128/IAI.70.4.2039-2048.2002
154. Brown, WC, McElwain, TF, Palmer, GH, Chantler, SE, and Estes, DM. Bovine CD4+ T-lymphocyte clones specific for Rhoptry-associated protein 1 of Babesia bigemina stimulate enhanced immunoglobulin G1 (IgG1) and IgG2 synthesis. Infect Immun. (1999) 67:155–64. doi: 10.1128/IAI.67.1.155-164.1999
155. Norimine, J, Mosqueda, J, Suarez, C, Palmer, GH, McElwain, TF, Mbassa, G, et al. Stimulation of T-helper cell gamma interferon and immunoglobulin G responses specific for Babesia bovis rhoptry-associated protein 1 (RAP-1) or a RAP-1 protein lacking the carboxy-terminal repeat region is insufficient to provide protective immunity against virulent B. bovis challenge. Infect Immun. (2003) 71:5021–32. doi: 10.1128/IAI.71.9.5021-5032.2003
156. Fish, L, Leibovich, B, Krigel, Y, McElwain, T, and Shkap, V. Vaccination of cattle against B. bovis infection with live attenuated parasites and non-viable immunogens. Vaccine. (2008) 26:G29–33. doi: 10.1016/j.vaccine.2008.09.070
157. Hines, SA, Palmer, GH, Jasmer, DP, Goff, WL, and McElwain, TF. Immunization of cattle with recombinant Babesia bovis merozoite surface antigen-1. Infect Immun. (1995) 63:349–52. doi: 10.1128/iai.63.1.349-352.1995
158. Terkawi, MA, Ratthanophart, J, Salama, A, AbouLaila, M, Asada, M, Ueno, A, et al. Molecular characterization of a new Babesia bovis thrombospondin-related anonymous protein (BbTRAP2). PLoS One. (2013) 8:e83305. doi: 10.1371/journal.pone.0083305
159. Rittipornlertrak, A, Nambooppha, B, Muenthaisong, A, Apinda, N, Koonyosying, P, Srisawat, W, et al. Immunization of cattle with recombinant structural Ectodomains I and II of Babesia bovis apical membrane antigen 1 [BbAMA-1(I/II)] induces strong Th1 immune response. Front Vet Sci. (2022) 9:9. doi: 10.3389/fvets.2022.917389
160. Alvarez, JA, Lopez, U, Rojas, C, Borgonio, VM, Sanchez, V, Castañeda, R, et al. Immunization of Bos taurus steers with Babesia bovis recombinant antigens MSA-1, MSA-2c and 12D3. Transbound Emerg Dis. (2010) 57:87–90. doi: 10.1111/j.1865-1682.2010.01117.x
161. Hidalgo-Ruiz, M, Suarez, CE, Mercado-Uriostegui, MA, Hernandez-Ortiz, R, Ramos, JA, Galindo-Velasco, E, et al. Babesia bovis RON2 contains conserved B-cell epitopes that induce an invasion-blocking humoral immune response in immunized cattle. Parasit Vectors. (2018) 11:575. doi: 10.1186/s13071-018-3164-2
162. Hidalgo-Ruiz, M, Mejia-López, S, Pérez-Serrano, RM, Zaldívar-Lelo de Larrea, G, Ganzinelli, S, Florin-Christensen, M, et al. Babesia bovis AMA-1, MSA-2c and RAP-1 contain conserved B and T-cell epitopes, which generate neutralizing antibodies and a long-lasting Th1 immune response in vaccinated cattle. Vaccine. (2022) 40:1108–15. doi: 10.1016/j.vaccine.2022.01.023
163. Gimenez, AM, Françoso, KS, Ersching, J, Icimoto, MY, Oliveira, V, Rodriguez, AE, et al. A recombinant multi-antigen vaccine formulation containing Babesia bovis merozoite surface antigens MSA-2a1, MSA-2b and MSA-2c elicits invasion-inhibitory antibodies and IFN-γ producing cells. Parasit Vectors. (2016) 9:1–13. doi: 10.1186/s13071-016-1862-1
164. Jaramillo Ortiz, JM, Paoletta, MS, Gravisaco, MJ, López Arias, LS, Montenegro, VN, de la Fournière, SAM, et al. Immunisation of cattle against Babesia bovis combining a multi-epitope modified vaccinia Ankara virus and a recombinant protein induce strong Th1 cell responses but fails to trigger neutralizing antibodies required for protection. Ticks Tick Borne Dis. (2019) 10:101270. doi: 10.1016/j.ttbdis.2019.101270
165. Camacho-Nuez, M, Hernández-Silva, DJ, Castañeda-Ortiz, EJ, Paredes-Martínez, ME, Rocha-Martínez, MK, Alvarez-Sánchez, ME, et al. Hap2, a novel gene in Babesia bigemina is expressed in tick stages, and specific antibodies block zygote formation. Parasit Vectors. (2017) 10:1–9. doi: 10.1186/s13071-017-2510-0
166. The inability of a south African Babesia bovis vaccine strain to infect Boophilus microplus. Onderstepoort J Vet Res. (1986) 53:143–5.
167. Combrink, MP, Troskie, PC, de Klerk, DG, Pienaar, R, Latif, AA, and Mans, BJ. Co-transmission of the non-transmissible south African Babesia bovis S24 vaccine strain during mixed infection with a field isolate. Ticks Tick Borne Dis. (2015) 6:158–63. doi: 10.1016/j.ttbdis.2014.11.007
168. Mangold, AJ, Aguirre, DH, Cafrune, MM, de Echaide, ST, and Guglielmone, AA. Evaluation of the infectivity of a vaccinal and a pathogenic Babesia bovis strain from Argentina to Boophilus microplus. Vet Parasitol. (1993) 51:143–8. doi: 10.1016/0304-4017(93)90205-2
169. Echaide, IE, de Echaide, ST, and Guglielmone, AA. Live and soluble antigens for cattle protection to Babesia bigemina. Vet Parasitol. (1993) 51:35–40. doi: 10.1016/0304-4017(93)90193-Q
170. De Vos, AJ, Combrink, MP, and Bessenger, R. Babesia bigemina vaccine: Comparison of the efficacy and safety of Australian and south African strains under experimental conditions in South Africa. Onderstepoort J Vet Res. (1982) 49:155–8.
171. Brizuela, CM, Ortellado, CA, Sanabria, E, Torres, O, and Ortigosa, D. The safety and efficacy of Australian tick-borne disease vaccine strains in cattle in Paraguay. Vet Parasitol. (1998) 76:27–41. doi: 10.1016/S0304-4017(97)00047-2
172. Buening, GM, Kuttler, KL, and Rodriguez, SD. Evaluation of a cloned Babesia bovis organism as a live immunogen. Vet Parasitol. (1986) 22:235–42. doi: 10.1016/0304-4017(86)90110-X
173. Yunker, CE, Kuttler, KL, and Johnson, LW. Attenuation of Babesia bovis by in vitro cultivation. Vet Parasitol. (1987) 24:7–13. doi: 10.1016/0304-4017(87)90125-7
174. Jorge, G, Alarcón, C, Vicente, J, Millan, F, Alberto, J, Aragón, R, et al. Evaluation of pathogenicity and protection capability of a fresh combined immunogen of Babesia bigemina and Babesia bovis. Veterinaria México. (1999) 30:215–20.
175. Alarcón, GJC, Martínez, JAÁ, Ramírez, EER, Aragón, JAR, Gualito, JJM, Murguía, CAV, et al. Protection against bovine babesiosis with a mixed in vitro culture-derived Babesia bovis and Babesia bigemina vaccine under field challenge. Immunization in a disease-free area. Veterinaria México. (2003) 34:323–32. doi: 10.21753/vmoa.34.004.98
176. Bautista-Garfias, CR, Lozano, AR, Martínez, CR, Martínez, JAÁ, Millán, JVF, García, GRR, et al. Co-immunization of cattle with a vaccine against babesiosis and Lactobacillus casei increases specific IgG1 levels to Babesia bovis and B. bigemina. Parasitol Int. (2015) 64:319–23. doi: 10.1016/j.parint.2015.04.005
177. Rojas-Martínez, C, Rodríguez-Vivas, RI, Millán, JVF, Bautista-Garfias, CR, Castañeda-Arriola, RO, Lira-Amaya, JJ, et al. Bovine babesiosis: cattle protected in the field with a frozen vaccine containing Babesia bovis and Babesia bigemina cultured in vitro with a serum-free medium. Parasitol Int. (2018) 67:190–5. doi: 10.1016/j.parint.2017.11.004
178. Rauf, U, Suleman, M, Abid, A, Jamil, H, Menghwar, H, Durrani, AZ, et al. Humoral and cell-mediated immune response validation in calves after a live attenuated vaccine of Babesia bigemina. Pathogens. (2020) 9:1–16. doi: 10.3390/pathogens9110936
179. Sunaga, F, Taharaguchi, S, Arai, S, Itoh, S, and Kanno, Y. Virulence attenuation of Babesia gibsoni by serial passages in vitro and assessment of the protection provided by the immunization against the passaged isolate in dogs. Vet Parasitol. (2013) 197:565–70. doi: 10.1016/j.vetpar.2013.05.015
180. Mazuz, ML, Laughery, JM, Lebovitz, B, Yasur-Landau, D, Rot, A, Bastos, RG, et al. Experimental infection of calves with transfected attenuated Babesia bovis expressing the Rhipicephalus microplus Bm86 antigen and eGFP marker: preliminary studies towards a dual anti-tick/Babesia vaccine. Pathogens. (2021) 10:1–16. doi: 10.3390/pathogens10020135
181. Oldiges, DP, Laughery, JM, Tagliari, NJ, Leite Filho, RV, Davis, WC, da Silva, VI, et al. Transfected Babesia bovis expressing a tick GST as a live vector vaccine. PLoS Negl Trop Dis. (2016) 10:e0005152. doi: 10.1371/journal.pntd.0005152
182. Boulter, NR, Brown, CGD, Kirvar, E, Glass, E, Campbell, J, Morzaria, S, et al. Different vaccine strategies used to protect against Theileria annulata. Ann N Y Acad Sci. (1998) 849:234–46. doi: 10.1111/j.1749-6632.1998.tb11054.x
183. Gharbi, M, Darghouth, MA, Weir, W, Katzer, F, Boulter, N, Adamson, R, et al. Prime-boost immunisation against tropical theileriosis with two parasite surface antigens: evidence for protection and antigen synergy. Vaccine. (2011) 29:6620–8. doi: 10.1016/j.vaccine.2011.06.109
184. Darghouth, MA, Boulter, NR, Gharbi, M, Sassi, L, Tait, A, and Hall, R. Vaccination of calves with an attenuated cell line of Theileria annulata and the sporozoite antigen SPAG-1 produces a synergistic effect. Vet Parasitol. (2006) 142:54–62. doi: 10.1016/j.vetpar.2006.06.010
185. MacHugh, ND, Weir, W, Burrells, A, Lizundia, R, Graham, SP, Taracha, EL, et al. Extensive polymorphism and evidence of immune selection in a highly dominant antigen recognized by bovine CD8 T cells specific for Theileria annulata. Infect Immun. (2011) 79:2059–69. doi: 10.1128/IAI.01285-10
186. Lempereur, L, Larcombe, SD, Durrani, Z, Karagenc, T, Bilgic, HB, Bakirci, S, et al. Identification of candidate transmission-blocking antigen genes in Theileria annulata and related vector-borne apicomplexan parasites. BMC Genomics. (2017) 18:1–14. doi: 10.1186/s12864-017-3788-1
187. Di Giulio, G, Lynen, G, Morzaria, S, Oura, C, and Bishop, R. Live immunization against East Coast fever – current status. Trends Parasitol. (2009) 25:85–92. doi: 10.1016/j.pt.2008.11.007
188. Fry, LM, Bastos, RG, Stone, BC, Williams, LB, Knowles, DP, and Murphy, SC. Gene gun DNA immunization of cattle induces humoral and CD4 T-cell-mediated immune responses against the Theileria parva polymorphic immunodominant molecule. Vaccine. (2019) 37:1546–53. doi: 10.1016/j.vaccine.2019.02.009
189. Ververken, C, Geysen, D, Loots, K, Janssens, ME, Guisez, Y, and Goddeeris, BM. Orientation of bovine CTL responses towards PIM, an antibody-inducing surface molecule of Theileria parva, by DNA subunit immunization. Vet Immunol Immunopathol. (2008) 124:253–63. doi: 10.1016/j.vetimm.2008.03.009
190. Kaba, SA, Hemmes, JC, Van Lent, JWM, Vlak, JM, Nene, V, Musoke, AJ, et al. Baculovirus surface display of Theileria parva p67 antigen preserves the conformation of sporozoite-neutralizing epitopes. Protein Eng Des Sel. (2003) 16:73–8. doi: 10.1093/proeng/gzg004
191. Musoke, A, Rowlands, J, Nene, V, Nyanjui, J, Katende, J, Spooner, P, et al. Subunit vaccine based on the p67 major surface protein of Theileria parva sporozoites reduces severity of infection derived from field tick challenge. Vaccine. (2005) 23:3084–95. doi: 10.1016/j.vaccine.2004.09.039
192. Lacasta, A, Mody, KT, De Goeyse, I, Yu, C, Zhang, J, Nyagwange, J, et al. Synergistic effect of two nanotechnologies enhances the protective capacity of the Theileria parva Sporozoite p67C antigen in cattle. J Immunol. (2021) 206:686–99. doi: 10.4049/jimmunol.2000442
193. Svitek, N, Saya, R, Awino, E, Munyao, S, Muriuki, R, Njoroge, T, et al. An ad/MVA vectored Theileria parva antigen induces schizont-specific CD8+ central memory T cells and confers partial protection against a lethal challenge. npj Vaccines. (2018) 3:1–11. doi: 10.1038/s41541-018-0073-5
194. Onuma, M, Kakuda, T, and Sugimoto, C. Theileria parasite infection in East Asia and control of the disease. Comp Immunol Microbiol Infect Dis. (1998) 21:165–77. doi: 10.1016/S0147-9571(98)00008-3
195. Mealey, RH, Kappmeyer, LS, Ueti, MW, Wagner, B, and Knowles, DP. Protective effects of passively transferred merozoite-specific antibodies against Theileria equi in horses with severe combined immunodeficiency. Clin Vaccine Immunol. (2012) 19:100–4. doi: 10.1128/CVI.05301-11
196. Santos, AC, Nogueira, CEW, dos Santos Suñe Moraes, B, Müller, V, Mousquer, MA, and Leite, FPL. Immune response of adult horses, pregnant mares and foals to an experimental vaccine with recombinant EMA-2 protein of Theileria equi. Res Vet Sci. (2021) 139:186–92. doi: 10.1016/j.rvsc.2021.07.013
197. Rodríguez-Vivas, RI, Grisi, L, Pérez de León, AA, Silva Villela, H, Torres-Acosta, JFJ, Fragoso Sánchez, H, et al. Potential economic impact assessment for cattle parasites in Mexico. Rev Mex Cienc Pecu. (2017) 8:61–74. doi: 10.22319/rmcp.v8i1.4305
198. Cobaxin-Cardenas, ME, Díaz, HA, Avelino, PO, Salinas-Estrella, E, De La, TJFP, Quiroz-Castañeda, RE, et al. Primer abordaje para la propagación de Anaplasma marginale (MEX-31-096) en células de garrapata Rm-sus. Revista del Centro de Investigación de la Universidad la Salle. (2019) 13:67–80. doi: 10.26457/recein.v13i51.2200
199. Rodríguez, SD, García Ortiz, MA, Hernández Salgado, G, Santos Cerda, NA, Aboytes Torres, R, and Cantó Alarcón, GJ. Anaplasma marginale inactivated vaccine: dose titration against a homologous challenge. Comp Immunol Microbiol Infect Dis. (2000) 23:239–52. doi: 10.1016/S0147-9571(99)00076-4
200. Ocampo Espinoza, V, Vázquez, JES, Aguilar, MD, Ortiz, MÁG, Alarcón, GJC, and Rodríguez, SD. Anaplasma marginale: lack of cross-protection between strains that share MSP1a variable region and MSP4. Vet Microbiol. (2006) 114:34–40. doi: 10.1016/j.vetmic.2005.11.058
201. Franklin, TE, and Huff, JW. A proposed method of Premunizing cattle with minimum Inocula of Anaplasma marginale. Res Vet Sci. (1967) 8:415–8. doi: 10.1016/S0034-5288(18)34600-9
202. Osorno, BM, Solana, P, Ristic, M, and Serrano, E. Estudio de una vacuna atenuada de Anaplasma marginale en México. Rev Mex Cienc Pecu. (1973) 1:57.
203. Camarillo, SDR, Ortiz, MÁG, Ramírez, EER, Alarcón, GJC, De La Torre, JFP, Cruz, RR, et al. Anaplasma marginale Yucatan (Mexico) strain. Assessment of low virulence and potential use as a live vaccine. Ann N Y Acad Sci. (2008) 1149:98–102. doi: 10.1196/annals.1428.067
Keywords: vaccine, farm animals, vector borne, ticks, blood sucking flies, parasites
Citation: Alzan HF, Mahmoud MS and Suarez CE (2024) Current vaccines, experimental immunization trials, and new perspectives to control selected vector borne blood parasites of veterinary importance. Front. Vet. Sci. 11:1484787. doi: 10.3389/fvets.2024.1484787
Edited by:
Rosa Estela Quiroz Castañeda, Instituto Nacional de Investigaciones Forestales, Agrícolas y Pecuarias (INIFAP), MexicoReviewed by:
Saeid Fathi, Razi Vaccine and Serum Research Institute, IranHugo Aguilar-Díaz, Instituto Nacional de Investigaciones Forestales, Agrícolas y Pecuarias (INIFAP), Mexico
Copyright © 2024 Alzan, Mahmoud and Suarez. This is an open-access article distributed under the terms of the Creative Commons Attribution License (CC BY). The use, distribution or reproduction in other forums is permitted, provided the original author(s) and the copyright owner(s) are credited and that the original publication in this journal is cited, in accordance with accepted academic practice. No use, distribution or reproduction is permitted which does not comply with these terms.
*Correspondence: Heba F. Alzan, aGViYS5hbHphbkB3c3UuZWR1; Carlos E. Suarez, Y2FybG9zLnN1YXJlekB1c2RhLmdvdg==