- 1Department of Animal Biosciences, University of Guelph, Guelph, ON, Canada
- 2Guelph Research and Development Center, Agriculture and Agri-Food Canada, Guelph, ON, Canada
Shift in laying hens housing from conventional cage-based systems to alternatives has impacted their health and performance. Microorganisms colonize young chick in the early stages of their physiological and immune development. These colonizing microbes originate from parent and the environment. Escherichia coli is among the normal gut colonizing bacteria however, some E. coli strains known as avian pathogenic E. coli (APEC), cause local or systemic infections (colibacillosis) responsible of significant economic losses to the poultry industry. Potential APEC strains and other poultry gut microbiota are influenced by several factors such as housing system, and the use of feed additives (prebiotics, probiotics, symbiotic, among others). This review will discuss the status of pullets and layers immunity, gut health, and predisposing factors of colibacillosis. Dietary interventions and some colibacillosis mitigation strategies in pullets and laying hens are reviewed and discussed. With the development of sequencing technologies and the use of feed additives as alternatives to antibiotics, future studies need to understand some of the complex associations between the feed additives, the rearing environment, and their selective pressure on gut microbiota, including E. coli, and their impacts on immune development in pullets and hens.
Introduction
The egg industry is one of the major sectors in the poultry value chain that attracts investment globally, and several countries have regarded eggs as the cheapest and healthiest source of animal protein (1, 2). Pullet rearing plays an essential role in the egg supply chain. Growers are mandated to raise healthy pullets to set a firm foundation for egg production that meets consumer’s demand (1). A major challenge encountered during pullet rearing is early mortality caused mainly by APEC infections, which interferes with flock uniformity, sexual maturity and performance (3, 4). Moreover, the poultry industry faces constant pressure to generate high-quality protein under different challenges, such as economic recession, animal welfare, and food safety (pathogens and antimicrobial resistance) (5). Conventional housing systems are still used globally in hens rearing despite being phased out in Europe since 2012 (6, 7). In Canada, more than 1,200 egg farms are raising about 24 million laying birds in conventional (battery) cages (57%) that produce 9 billion eggs annually (8). Raising hens in conventional low battery cages does not offer adequate space for the birds to behave naturally, which is a fundamental requirement according to the new legislations in some countries (9, 10). Thus, egg farmers of Canada are expected to phase out this cage system while establishing new Code of Practices and guidelines for pullet rearing (10–12). The adoptions of furnished and non-furnished cage systems decreased diseases prevalence and mortality rates (12).
Studies in Sweden between 2001 and 2004 on causes of mortality in laying hens revealed that colibacillosis was prevalent in layers (between the start of lay and 30 weeks) reared in litter-based housing system (38.7%) and conventional battery cages (38.6%) (10). Efforts have been deployed to phase out conventional low-battery cages and replace them with furnished and non-furnished cages systems to reduce mortality and improve performance. However, there is a need to adopt nutritional strategies, including the use of feed additives (pre-and pro-biotics, symbiotics, and phytobiotics) that have shown promising results in enhancing immunity and decreasing pathogens in the gut, especially APEC in laying hens. The aim of this review is to discuss the pullets/layers’ immune development, gut health, pathogenesis, and predisposing factors to colibacillosis. How dietary interventions could help in bolstering the immune system and mitigate infection in pullets and laying hens to improve productivity are also discussed.
Avian pathogenic Escherichia coli
Escherichia coli (E. coli) is a commensal bacterium of the gastrointestinal tract (GIT), the pharynx and trachea of birds, animals and human (13). However, some E. coli strains are known to cause serious diseases such as cystitis, colibacillosis in birds and animals, pyelonephritis, meningitis/sepsis, and gastroenteritis in humans due to their possession and expression of various virulence factors (14). Extraintestinal Pathogenic E. coli (ExPEC) strains which causes diseases outside the GIT, are epidemiologically and phylogenetically distinct from intestinal pathogenic E. coli (15). These ExPEC pathotypes, including uropathogenic pathogenic E. coli (UPEC), Sepsis-Associated Pathogenic E. coli (SEPEC), Neonatal Meningitis E. coli (NMEC) and Avian Pathogenic E. coli (APEC) pose considerable threats to human and animal health (16, 17). The APEC isolates harbor more virulence genes including adhesins (fimH, focG, hra, iha, kii, papA, papC, papEFG, sfa, sfaS), toxins (cdts, EAST11, pic, tsh, vat), protectins (iss, kpsMT K1, kpsMT KII, kpsMT K5, traT), iron regulated systems (ireA, iroN, iutA, fyuA) and miscellaneous genes having various functions [clbB, clbN, cvaC, H7 fliC, hemF, ibe10, ompT, malX (PAI), uidA, usp] than the commensal E. coli strains (18, 19).
Colibacillosis is characterized by respiratory, systemic, and reproductive tract infections caused by APEC (16). It can affect poultry of all ages, leading to significant economic losses and compromising animal welfare in poultry production (20, 21). Colibacillosis requires predisposing factors such as infections by Mycoplasma gallisepticum, compromised mucosal barriers, immunosuppression related to stressors (vaccinations and viral infections), poor hygiene and ventilation in hatchery and poultry barns (21). Newly hatched chicks and pullets have weak and underdeveloped immune systems, making them more susceptible to APEC infections. This results in early mortalities of birds and decreased egg production in laying hens due to salpingitis-peritonitis syndrome (9).
Transmission and pathogenesis of APEC
The main transmission route of APEC in pullets and laying hens is via the fecal-oral route through inhaling contaminated dust (horizontal transmission) in hatcheries or production houses (22). The vertical transmission route is hypothesized to emanate from the infected reproductive tract (22–24). However, the pathogenesis of APEC and how it causes yolk sac infection are unclear. Investigations indicated that the APEC mortality rate ranges between 10 and 20% in 48 h after hatch, primarily due to septicemia (25). Pathological signs can include lung congestion, splenitis, and edematous serous membranes. A couple of days after the infection, fibrin-heterophilic polyserositis appears within the pericardium, air sacs, pleura, and perihepatic tissues. The incubation period ranges between three to five days after the infection (Figure 1). Previous studies showed a close association between the first-week mortality and the breeder hen age; the older the breeder, the higher the risk of first-week chicks’ mortalities (16). Despite understanding their source and transmission route, the mechanism by which APEC establishes infections needs to be clarified, and experimental findings disclose substantial inconsistencies (26, 27). Studies indicated that APEC can colonize the chicken gastrointestinal and respiratory tracts without causing diseases and only their translocation to extra-intestinal sites in the presence of production-related stress induced the disease (Table 1). As shown, E. coli isolates from colibacillosis harbor virulence genes that encode adhesins, invasins, iron acquisition systems, toxins and protectins, which play an important role in its pathogenesis (16). Adhesion to the host cells is an important stage of APEC pathogenesis and is mediated by various adhesins (Table 1). Type 1 fimbriae play a crucial role in the adherence of APEC on the epithelial cells of the respiratory tract during the initial stages of the infection (14, 16, 28–30). In contrast, the expression of P fimbriae and S fimbriae contributes later to APEC pathogenesis. Anti-type 1 fimbriae serum and D-mannose, cellular receptors for type 1 fimbriae, could block chicken tracheal colonization by certain APEC strains. Curli fimbria is associated with bacterial biofilm formation and cell invasion (31). Temperature-sensitive hemagglutinin (tsh) mediates colonization during the first stages of respiratory tract infections (32). Invasins virulence genes facilitate the entry of APEC into host cells (32–34). These virulence-associated factors facilitate APEC adhesion, host cell invasions, evasions from phagocytic cells, colonization, multiplications/proliferation, cell lysis and damage, as well as systemic dissemination of APEC infections, and eventually colibacillosis infection in chicken (Table 1).
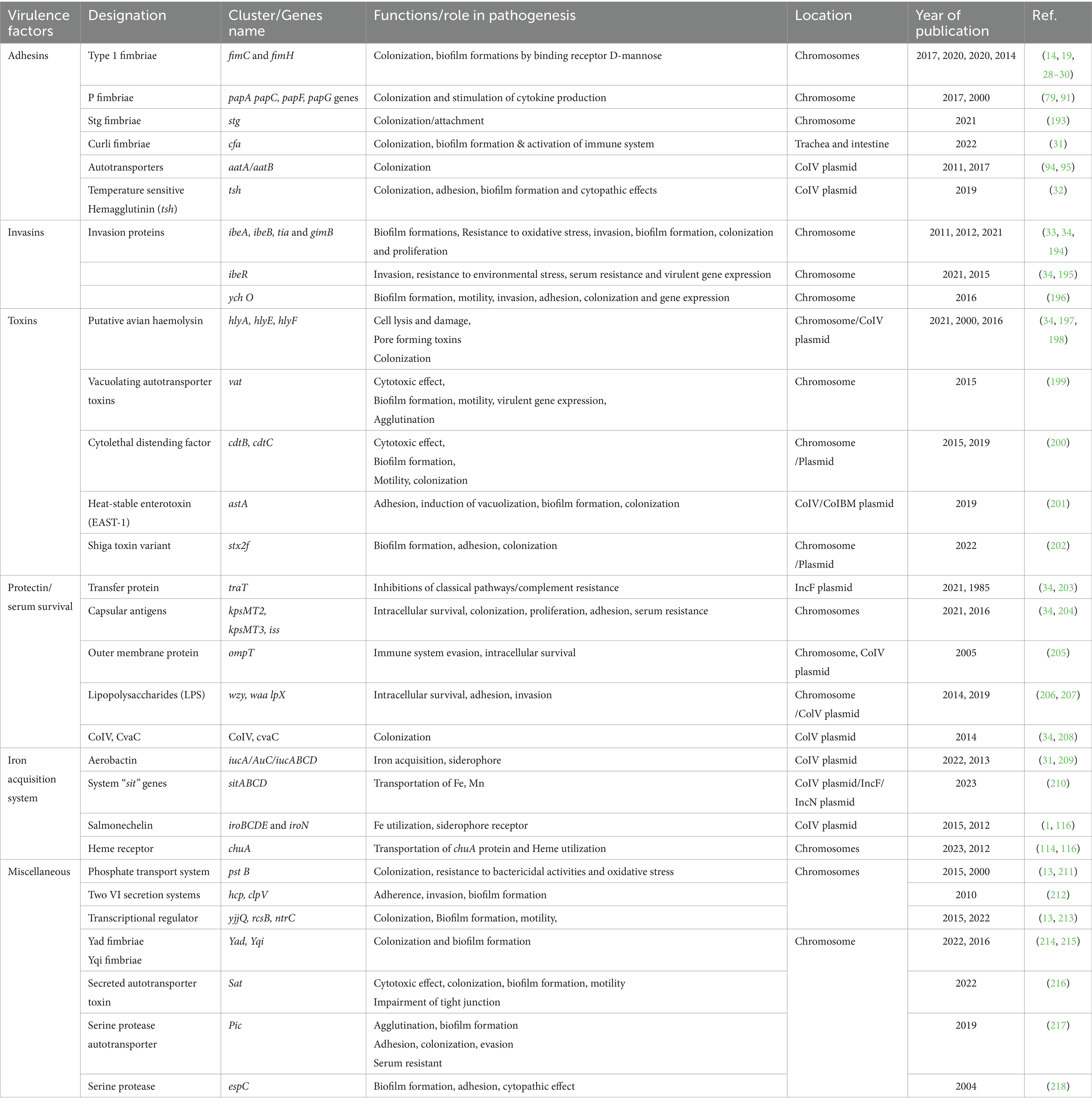
Table 1. The roles of virulence associated factors/gene in pathogenesis of colibacillosis/APEC infections.
Pullet and layers immune system development
The development of birds’ defense mechanisms starts during the embryonic stage, with eggshells offering physical barriers against damage and infections. Apart from the eggshell, the four main parts of an egg’s inner structure (the yolk, vitelline membrane, and egg white) offers protections to the developing embryo. Throughout the embryo’s 21-day development, they supply energy and nutrients as well as chemical and molecular defenses against microbial invasion. Embryonic development (ED) takes 21 days and the first sign of developing immune system is observed at day 10 (ED10) and at ED18, chicken embryo is immunocompetent and able to produce both innate and adaptive immune response to pathogens (35). Innate immune system of chicken embryo starts to develop in early embryonic stage (ED2), with key immune cells (CD2+ AND CD45+ cells) present in in yolk sac, before liver and thymus are fully developed (36). Granulopoiesis begins early in embryonic development with the expansion of the granulocytic lineage (between ED7 to ED20) in the yolk and splenic primordium and granulocyte differentiation in the liver at ED15 (35). Chicken embryos are unable to mount adequate immune response until ED12. In chicks, analysis of heterophil functionality allows to describe their activities, such as phagocytic activities, degranulation, microbial killings, and oxidative burst, which appears to decrease in mature birds compared to newly hatched chicks. At ED18, the chicken embryo is stimulated with sufficient heterophils that enhance immune functions suggesting that they are already functional at that time (35). However, uncertainties exist about when heterophils begin to be fully functional.
Chicken embryo is immunocompetent and able to produce both innate and adaptive immune responses to pathogens. However, as the embryo develops, innate defenses in the egg disappear, allowing the growth of pathogenic bacteria however, this needs to be fully characterized. Moreover, immunocompetence appears a few days post-hatch, and during this period, the chicks rely on maternal antibodies for protection against microbial infections (20, 21). Innate immunity is non-specific but, it offers the first line of defense against a wide range of pathogens in birds and does not confer long-lasting immunity. The components of the innate immune system are physical and chemical barriers, including blood proteins and cellular components. The innate immune system reacts promptly against invading pathogens by quickly instructing antigen-presenting cells (APC) to activate and secrete cytokines that regulate T and B cells to mount suitable adaptive immune responses. Activation of APCs in response to pathogens is mediated by pattern recognition receptors (PRR) resulting in the production of pro-inflammatory cytokine and co-stimulatory molecules, which are involved in the activation of adaptive immune response (37).
Maternal antibodies and innate immune cells such as macrophages and monocytes, play important roles in the chicks’ defenses against pathogens since adaptive immune functions have not yet been fully developed during the first three weeks of the chicks life, which rely solely on maternal antibodies. The maternal antibodies reduce gradually as chicks age resulting in an increased susceptibility to disease (9). Thus, early interventions such as nutrition to promote the development and maturity of the immune system of chicks are needed. The development and maturity of the immune system in laying hens takes about eight weeks after hatch, and this underdeveloped immune system in young chicks increases the susceptibility to yolk sac infections (38, 39). Studies using ileum and jejunum in laying hens revealed that IgM and IgY gene expressions peak at weeks one and five of age respectively, while IgA expressions increased with the hen’s age during the growing period (40). At the week eight, the sexually immature hens undergo bone and reproductive development until about 17 weeks of age. Contrary to the laying phase, which is characterized by decreased cytotoxic T lymphocyte (CTL) and γδ T cell levels and increased innate immunity (38, 40). During the onset of lay and at the egg production peak, the estrogen and corticosterone hormone levels rise along with a remarkable change in the immune cells proportion and a reduction in cellular response, leading to an increased susceptibility to pathogenic bacteria (E. coli and Salmonella) (38). Currently, limited studies exist on immune parameters during the pullets, and laying phase.
Immune system and the predisposition factors to APEC
Hatchery hygiene
The hatcheries occupy a central position between breeder and producers thus, optimized hatchery hygiene plays an essential role in pathogen prevention across the production chain (41). The first week is crucial in transforming chick’s life and its overall performance (42). From the hatcheries to the brooders’ house, the chicks grow and adapt to new feeds, and environmental changes while fighting against diseases. The large-scale production in commercial hatchery and transportation of chicks over to brooder house, predisposes them to infections especially by pathogenic E. coli (42). During the first week of life both layers and broilers are highly susceptible to infections caused by APEC or Enterococcus faecalis, which accounts for more than 50% mortalities during this period (4). These infections are thought to originate from hatcheries and parent flocks. Kemmett et al. (43) has demonstrated that intestinal tract of day-old broilers chick can be colonized by APEC carrying at least 10 virulence-associated genes. Breeder hens raised on floor/litter get the eggshell and eggs contaminated with bacteria such as E. coli which could infect the chicks during the incubation (44). While in the hatchery, the chicks infected via the vertical route succumb to death from yolk sac infection (omphalitis) (16, 42). Thus, it is important to maintain a pathogen-free hatching environment to prevent contamination and the spread of pathogens in poultry production chain. Figure 2 shows potential sources of infections including the hatchery and transportation however, improper cleaning, disinfection and people handling the chicks could result in infection as well.
Poultry houses
The initial exposure to APEC could happen in the hatchery from the contaminated/infected eggs (4, 25). Systemic infection usually needs predisposing factors (infectious/non-infectious) such as nutritional deficiencies, toxins, other diseases, and the under-developed immune system of the bird. Such stressors are experienced mostly in commercial set-ups, and they increase corticosterone levels and susceptibilities to colibacillosis. A high prevalence of bacterial, viral, and parasitic infections could occur in litter-based and free-range systems (45). In commercial farms, layer hens are reared in a cage system, classified into conventional and enriched cages or an alternative housing system (46). Conventional cages have limited spaces for bird’s movement while lacking nests and perches to allow the expression of natural behavior (18, 30). The alternative housing system includes indoor (single-tier and multi-tier), and outdoor (free range and organic) housing systems designed to meet bird’s comfort (10). More research is needed to ascertain effects of these housing designs and stocking density on colibacillosis in pullets and laying hens.
The Canadian Code of Practice for the Care and Handling Pullets and Layer, established in 2017, offers the detailed guidelines on housing design and stocking density of laying hens (11). High-stocking density generate dust and ammonia in these housing systems (10), which causes health problems for birds (47). The increase in ammonia levels in poultry houses damages the respiratory system of the birds, predisposing them to infectious agents such as bronchitis virus (IBV), Newcastle disease virus and Mycoplasma gallisepticum, which may play a role in the APEC pathogenesis (47). Therefore, unfavorable housing environments that generate a lot of dust and ammonia result in the inhalation of large quantities of contaminants which are not completely cleared in the respiratory system making birds highly susceptible to colibacillosis (48). Inhalable dust concentration varies with housing system: aviary ranges (1.3–9.5 mg/m3), conventional cages (0.2–2.3 mg/m3), and enriched system (0.4–3.5 mg/m3) (49). Bacterial counts ranged between 10,000 – 8,000,000 cfu/m3 in different housing systems, and indoor E. coli counts have been reported to reach to1,000 cfu/m3 with slightly a higher concentration in the aviary (50). In the dust, E. coli can survive for extended period, and its airborne transmissions can result in its spread within and between poultry houses (26, 50).
Dust generation and ammonia emissions above 70 ppm can reduce spleen weight, lysozyme and globulin concentration while limiting lymphocyte proliferation (51). High ammonia level of more than 25 ppm in laying houses has been reported to decrease feed intake and growth rate (35). The appearance of a NH3 concentration of 15 ppm affected the trachea microbiota of chicken and increased the possibility of upper respiratory tract infections. In broilers, NH3 increased E. coli and Shigella numbers in lung tissue and activated inflammation (52). Moreover, high concentrations of NH3 over a long time resulted in respiratory illness, increased susceptibility to APEC infection, affected egg quality and reduced egg production (51). An investigation of Hy-Line Brown laying hens showed that high NH3 and temperature jointly increased IgG and decreased IgA (53). Generally, NH3, respirable dust and bacteria are much higher in the aviary and floor housing system than in conventional cages, with the least in furnished cages (54).
Bird stocking density
Stocking density refers to the number of hens or hens’ body weights per unit area, and space allowance is the space provided for each hen (55). Providing adequate space relative to a hen’s body area would increase freedom of movement and allow it to adequately perform all behavior in their repertoire (56). The current recommended and approved stocking density by the National Farm Animal Care Council (NFACC) in Canada for poultry rearing ranges between 284 cm2/bird for high density and 854 cm2/bird for low density (11). Spacing allowances, types of housing systems, and their effect on performance of laying hens are presented on (Table 2). These factors influence birds’ welfare while increasing stress level and diseases susceptibilities (57). A study on impact of cage density revealed that there is an increased risk of mortality in high-stocked density barns, but the causes of mortalities were not established (56). The findings indicated that the housing system could influence bird’s performance and health (58). Furthermore, high stocking density was negatively correlated with the development of immune organs (59).
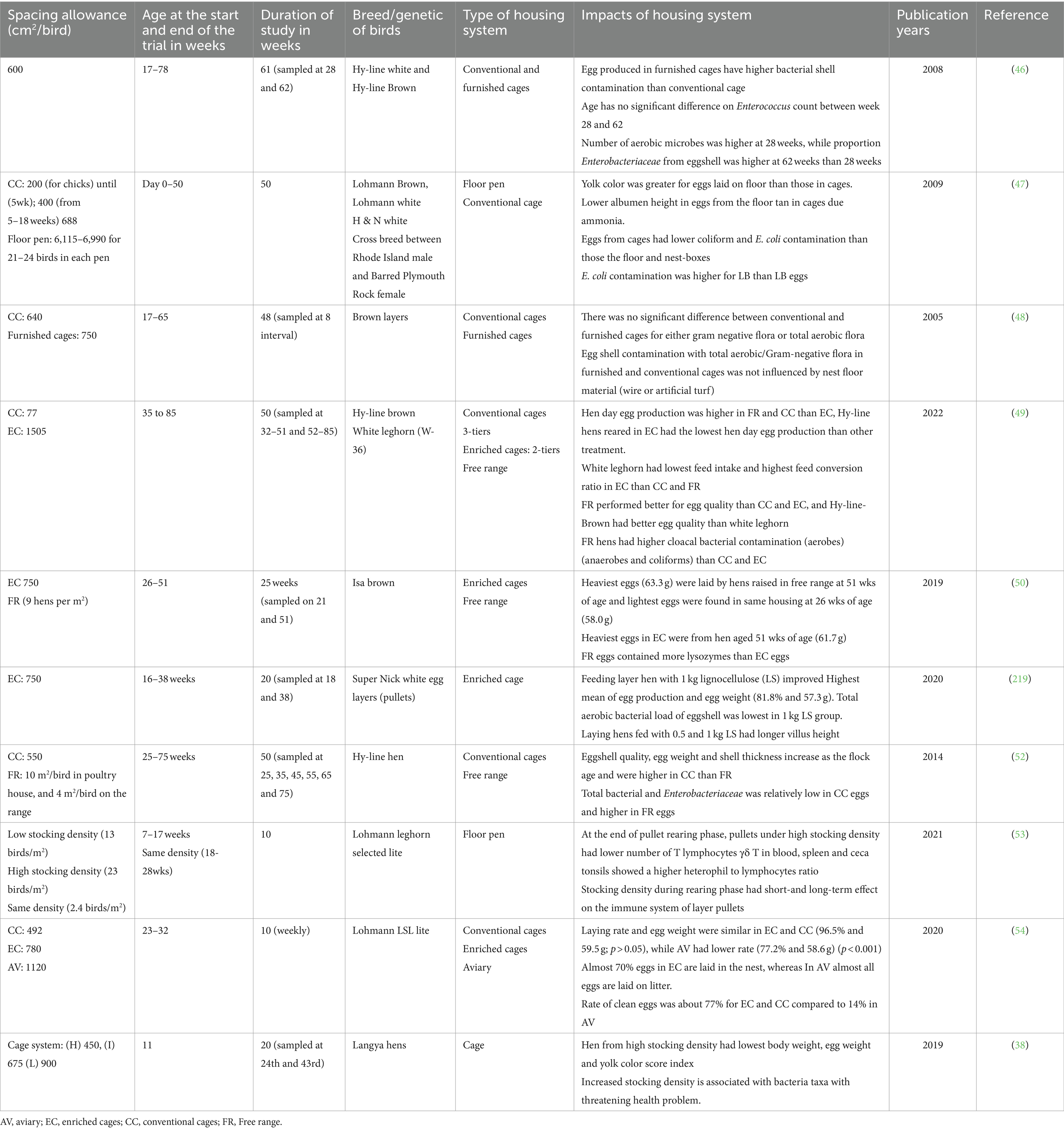
Table 2. Spacing allowance, types of housing system and their effects on performance in laying hens.
Infectious diseases are a significant concern for hens raised in high stocking density (57). Gast and his colleagues (60) observed higher Salmonella Enteritidis counts in the liver and ovaries of experimentally infected hen housed in a high stocking density enriched cage system (648 cm2 per bird) than in hens housed at lower (973 cm2 per bird) stocking density (60). High stocking density (284 cm2/bird) induces physiological stress by stimulating the production of corticosterone (CORT) hormones from the hypothalamic pituitary adrenal axis in response to appetitive and aversive stimuli, which act upon behavior, metabolism and immunity (51). Eugen et al. (61) reported high anxiety, blood pressure, and CORT hormones in pullets, when the stocking density (SD) increased from low SD (500 to 1,429 cm2/chick) to high SD/overcrowding (56 to 167 cm2/chick) during the first 10 weeks of age in conventional housing system. Overcrowded barns were found to increase the Salmonella Enteriditis population in chicken caeca, which might contribute to food safety concerns (62). Stress and unconducive housing conditions are understood to predispose both young birds and adult birds to colibacillosis (63). As summarized in Table 2, the adoption and use of enriched cages might help improve performance and immunity of layer hens.
Stressors
Stress is an adaptive response to threats on animal homeostasis; changes in immune status and response reflect animals’ reaction to stress (64). Poultry are confronted with a wide range of acute and chronic stressors in their housing environment that might threaten their welfare and health by modulating their immune system (65). The parameters used to measure these stressors are heterophil and lymphocyte ratio, corticosterone, glucose, and catecholamine levels (66). Studies have demonstrated that immunological stress induced by E. coli endotoxin lipopolysaccharide (LPS) causes pullets sickness, including high fever, reduced feed intake, and changes in birds’ behaviors (67). Nutritional factors, including high levels of dietary polyunsaturated fatty acids (PUFA), deficiencies of vitamins (A E), selenium, zinc, and manganese, and the presence of mycotoxins, and other toxic substances, are the most important stressors during growth and development. Reproductive stages, and dietary changes (starter, grower, and finisher) also interfere with bird’s immune functions. For example, poor feed formulation with deficiency or excessive vitamin A increases chicks’ susceptibility to E. coli infections, followed by a decreased immune response (68). Additionally, birds can be affected by other stressors including environmental conditions (temperature, relative humidity, heat and cold stress), infectious agents (bacterial/viral), and transportation stressors.
The immune response in bird differs according to the period and severity of the stressor and its physiological and nutritional status (Figure 2). Birds are known to maintain relatively constant temperatures of their internal organs. The housing system for laying hens has been shown to influence the immune cells (basophils, lymphocytes, eosinophils, and phagocytes) functions against pathogenic bacteria (Salmonella spp.) due to intestinal damages and the increase of inflammatory cytokine concentrations (69). A high ambient temperature increases chickens’ energy requirement, resulting in a significant loss for a less efficient feed conversion to eggs or meat, which has a detrimental effect on health and performance (70). High relative humidity and ambient temperature induce heat stress (HS), affecting performance, welfare, and bird’s health (70). Similar to HS, cold stressors interfere with bird’s performance by decreasing body weight and egg production in laying hens, and all these factors contribute to increased susceptibility to infectious diseases (38). However, the mechanism by which it causes colibacillosis is unclear and needs more research.
Heat stress occurs when the heat generated in the body surpasses its dissipation capacity, and the body cannot get rid of excess heat, resulting in reduced feed intake, body weight, egg production, and increased susceptibility to infection (71). Heat stress also negatively affects intestinal development, particularly intestinal epithelium integrity. It destroys crypt depth and stripping, resulting in reduced villi height, and decreases epithelial cell area ratio, promoting growth of pathogenic bacteria such as Salmonella and E. coli (72). Very high temperature influences a bird’s immune responses by inhibiting lymphocyte functions (38). Heat stress is reported to increase intestinal permeability through the physiological adaptation process. It increases the peripheral blood flow and decreases intestinal blood supply, resulting in hypoxia and oxidative stress (73). Continuous exposure to cold stress results in increased corticosteroids that cause an imbalance of the bird’s metabolism, increasing the susceptibility of the birds to diseases, especially colibacillosis (74).
Naturally, living cells balance the formation and inactivation of reactive oxygen species (ROS) and reactive nitrogen species (RNS) from mitochondria. However, due to different stressors, the free radicals’ productions exceed the ability of the antioxidant system to neutralize them, resulting in oxidative stress that causes damage to unsaturated lipids in plasma membranes, leading to DNA damage and disruption of membranes and cell integrity. Membrane damages are linked with reduced efficient nutrient absorption, leading to decreased performance, immunity suppression, and increased susceptibility to infection (75). For instance, hens are characterized by weak immune systems during their first week of life and peak production phase (32–35 weeks of age) and are highly susceptible to pathological alterations caused by E. coli. At the peak production phase, E. coli can colonize the oviduct (salpingitis) when estrogen levels are high, along with a weakened immune system (76).
Poultry transportation is one of the technological stresses encountered in the commercial industry (77), and the size and age of the bird greatly influence tolerance to challenges experienced during handling and transportation (78). Transportation is a multifactorial and stressful process that subject poultry birds to a noisy environment, poor handling during loading and offloading in trucks, highly stocked into designated net cages, deprivation of food, water and long transportation hours where they are exposed to vibration, heat, noise and cold. Watts et al. (79), reported higher moisture and heat production in smaller birds than larger birds. Heat stress was confounded in birds stocked at high density during transportation, which change the stress parameters in birds’ body predisposing them to bacterial infection and mortality (79). Thermal stressors experienced during birds’ transport have the potential to severely reduce welfare, contributing greatly to stress, disrupting homeostasis that increases the susceptibility to infection (80). Improper disinfection of net cages used initially for flock transportation might harbor infectious agents such as APEC, which can be transmitted to birds. Moreover, frequent social encounters could exacerbate negative stress induced behaviors for example cannibalism and feather pecking in high stocking density barn, which predisposes the hens to colibacillosis (10, 11). Stress in chicken can have a profound long-lasting effect on behaviors especially when experienced at early life and pullet stage (81, 82). However, the stress levels and its consequences vary during different stages of egg production (82). There is need for research to understand the correlation between stressors and chicken health and egg production.
Nutritional requirements and colibacillosis
Nutritional requirements for pullets and laying hens are complex and should be managed carefully to ensure optimal health, productivity, and robust immunity. The lifecycle of laying hen is divided into pre-and post-sexual maturity (83). During pre-sexual maturity (pullet phase), poultry nutritionist formulates feeds to build a firm foundation for future production by ensuring the least mortality, optimum health status, proper sexual maturity time, and flock uniformity (84). Diets for laying hens are formulated to optimize performance, prolonged peak laying period, and optimum immune functions. The pullet phase is the most rapidly growing and important period of the hen’s life, which is categorized into four stages by age: 0–6 weeks, 6–12 weeks, 12–18 weeks, and 18 weeks to the age of first lay (83). The initial post-hatch phase of the chick can utilize nutrients from the yolk sac for 72 h and thereafter rely on exogenous nutrients. During this period, the quality of feedstuffs is of great significance to pullet health as a strong correlation exists between body development and laying performance and its ability to extend lay up to 100 weeks of age (83).
Chicks are usually fed crumbles starter diets for the first 4 weeks of post-hatch, with a relatively high-energy content (12.3–1.4 MJ/kg) derived from carbohydrates and fats and with an increased calcium concentration (1.05–1.10%) to promote skeletal development and crude protein (18–20%) for rapid structural and immune development (85). Sometimes, starter diets can be prolonged to 6 weeks, depending on the chick’s weight. Certain feed additives such as fatty acid, probiotics, prebiotics, and symbiotics are used in starter diets to enhance gut barriers and immune response against pathogens such as E. coli. Grower diets are generally fed from four to 10 weeks of age (WOA) and have lower energy (11.9–12.0 MJ/kg) and calcium (0.9–1.10%) content than starter diets (85). As about 95% of the skeletal development occurs during the grower phase, an adequate amount of calcium and phosphorous needs to be supplied to prevent osteoporosis in mature hens (86). At 10 WOA, pullets are fed on diets that contain low energy density to minimize incidences of overweight and increase gut holding capacity by promoting gastrointestinal organ development (87). The onset of sexual maturity begins at ~17 WOA, and the commencement of egg production is determined by age, body weight, body fat content, and increased light intensity.
Nutritional stressors can play a significant role in predisposing pullets and hens to colibacillosis. Imbalances of nutrient intake, for example, vitamins (A and E), proteins, and minerals, can compromise the immune system of hens, making them susceptible to colibacillosis (68). Studies on the resistance to E. coli infection using chicks fed diet depleted (0 μg/kg), sufficient (0.85 μg/kg) or excess (1,000 mg/kg) in vitamin A (59), revealed that excess or insufficient vitamin A resulted in an increased susceptibility of chicks to E. coli infection accompanied by reduced immune response by impairing IgA and IgG production (68). A study on the effect of dietary vitamin E type (synthetic 22.00 mg), vitamin E level (natural 220 IU/kg) in broiler male chicken challenged with LPS showed that natural vitamin E had a significantly lower LPS-induced inflammatory response than synthetic vitamin E, this suggests a protective effect from vitamin E (natural type) in case of bacterial components (88). These studies indicate that vitamins A and E have anti-inflammatory responses that protect chicken health against bacterial infection. However, nutritional deficiencies caused by linolenic acid, iron, and selenium impair immune functioning and increase susceptibility to infectious diseases (89, 90). Thus, these nutrients are required during the acute or chronic stages of the disease. Certain nutritional compounds, such as fatty acids, vitamins (A, C, D, and E) have anti-inflammatory properties, whereas others, including probiotics, prebiotics, herbal and plant extracts, and long polyunsaturated fatty acids, have immunomodulatory features (88). Diets that contain inadequate levels of protein or amino acids can interfere with immune functions and increase susceptibility to microbial infections, especially in pullets and laying hens. Therefore, there is a need to identify nutritional strategies to enhance the immune system of chickens against microbial infection.
Control measures against APEC
Antibiotics
The most common approach for controlling bacterial infectious diseases, including colibacillosis, is the use of antibiotics (91). However, the use of antibiotics in animal production has been linked to an increased antimicrobial resistance (AMR) prevalence (91, 92). Despite the presence of APEC in the oviduct, 62% of hens would continue producing eggs (93, 94). Antimicrobial-resistant E. coli isolates from laying hens have been reported in Switzerland (94), Belgium, Germany, and Italy (95, 96). Isolates were resistant to sulfamethoxazole, nalidixic, tetracycline, trimethoprim, ciprofloxacin, and ampicillin (94, 95). The egg industry has made significant progress in reducing antimicrobial use (AMU) and transitioning toward more humane husbandry practices such as using furnished cages, cage-free production, and pasture-raised hens. A study by Aguinos (97) reported tetracycline and gentamycin resistant Salmonella, Campylobacter, and E. coli in laying hens. Based on this study, more surveillance data on AMU and AMR in the egg industry is warranted (hatchery, pullet, and laying phase). In the United States of America (USA) most antimicrobials administered in egg-laying hens are via the feed; ionophores (monensin and salinomycin) are used in the pullet phase, and bacitracin is utilized in both pullets and layers to control Clostridium perfringens infections, and chlortetracycline for treatment of E. coli-associated infections (98). In the United Kingdom, tetracycline, penicillin, macrolides, aminoglycosides, pleuromutilins, fluoroquinolones, polymyxins, and lincosamides are used in laying hens (99). In the European Union colistin, neomycin, tylosin, oxytetracycline, chlortetracycline, and erythromycin are approved for use in laying hens while, the recommended antimicrobial use in the Canadian poultry industry includes penicillin G, neomycin, chlortetracycline and oxytetracycline (97, 100).
Tetracycline
The Tetracycline class of antibiotics includes chlortetracycline, oxytetracycline, and doxycycline, which inhibit bacterial protein synthesis (101). Chlortetracycline (CTC) is the most common antibiotic used in feeds for pullets and laying hens. According to a 2021 DANMAP report from Denmark, penicillin and tetracycline were the only two antibiotics used in layers (102). Chlortetracycline is the second in-feed antimicrobials approved in USA against E. coli associated infections during the laying period (98). In Spain, Moreno (97) showed that day-old chicks are regarded as the source of antimicrobial-resistant bacteria for laying hens, with tetracycline resistance (75%) being the most prevalent (103). In Ontario, tetracycline resistance in E. coli isolates in sentinel sites ranged from 26 to 69% in hens (97). Tetracycline-resistant E. coli isolated from poultry has a likelihood to become resistant to additional antibiotics (104). Moreover, the resistance might be conserved in bacterial populations over time, regardless of selection pressure, which might lead to an overall increase over time. Tetracycline resistance can be plasmid-mediated via horizontal gene transfer by mobile genetic element such as transposons, and integrons (104). The identified genes include those conferring resistance by efflux pumps such as tet(A), tet(B), tet(C), tet(D), and tet(G) (105).
Moreover, rearing layers in conventional battery cages at close proximities and high stocking density can heighten the spread of these resistance genes (105, 106). Studies have revealed that tet(A) and tet(B) are the most detected tetracycline resistance genes in commensal and pathogenic E. coli isolates from poultry, with tet(A) being the most prevalent (105). For example, a study in broiler chickens examining E. coli isolates from colibacillosis lesions identified a high prevalence of tet(A) and tet(B), with 96.7 and 38.3%, respectively (105, 106). These two genes have also been found in chicken manure and soil samples around poultry farms, emphasizing the environmental dissemination of this resistance determinant (105, 106). The presence of tetracycline resistance genes in such environments highlights the importance of prudent use of antibiotics, application of biosecurity measures and dietary interventions to mitigate the development and spread of resistance (107).
Gentamycin
Gentamycin is a broad-spectrum bactericidal aminoglycoside that inhibits protein synthesis and used widely against Gram-negative and some Gram-positive bacteria (108). In Canada and USA gentamycin is approved in day-old chicks at the hatchery to prevent early mortalities (109), while controlling E. coli, Salmonella, and Pseudomonas infections (98). A high prevalence of gentamycin resistant E. coli have been reported in Ontario in chicks of less than 10 days old. The increase in the use and resistance of gentamycin warrants further surveillance to determine whether production stage, season, age, and year are predictors of resistance to this antibiotic (110). The increased use of gentamycin at the hatchery to control E. coli (omphalitis) infection has been associated with its higher resistance level during the brooding stage (109). Further studies are required to ascertain AMR at the hatchery and brooding stage. Comparative phenotypic and genotypic analyses of resistant and susceptible E. coli strains in these rearing environments would provide insight into a genetic adaptation that confers resistance. A study on AMR phenotype and genotype in E. coli isolates from lesions of colibacillosis chicken has highlighted the difference in gene expression and the presence of specific resistance genes in resistant strains (105). Gentamycin resistance is commonly due to aminoglycoside-modifying enzymes acetyltransferases (aac) and O-phosphotransferases (aph). The aminoglycosides resistance aph(2″), aad(A), and aac(6″) genes are widespread in E. coli and prevalent in poultry and their environment (111). These genes are frequently found on plasmids, integrons, and transposons that contribute to their dissemination (112).
The lincomycin-Spectinomycin combination is used against respiratory and gastrointestinal infections in poultry. This practice has been linked to an increased gentamycin resistance mediated by aac(3), aph(2″), and aad(A) on plasmids, indicating potential horizontal gene transfer (113–115). Genomic investigation of gentamycin resistance in E. coli isolates from human and chicken sources in Canada between 2014 and 2017 revealed that the use of lincomycin-spectinomycin on poultry farms might be co-selecting for gentamycin-resistant plasmid in E. coli in broilers (116). Due to the development and increased AMR, the implementation of guidelines to restrict the use of gentamycin and other antibiotics in poultry is required. Alternative options include dietary supplementation with feed additives, vaccines, and biosecurity measures (117).
The 16S rRNA genes coding for the 16S ribosomal RNA are used in bacterial phylogeny building. The methylations of the 16S RNA due to methylases, including ArmA, RmtA/B/C/D/E/F/G/H, and NmpA, have been shown to induce, high level resistance to amikacin, tobramycin, gentamicin, and netilmicin. Aminoglycoside resistance due to ArmA and RmtB has been reported in poultry E. coli isolates (118).
Beta-lactams
Amoxicillin has been used in some countries such as Australia against infectious coryza (Avibacterium paragallinarum); fowl cholera (Pasteurella multocida), Ornithobacterium rhinotracheale and E. coli (119). Despite a reported low level of AMR, beta-lactam resistant E. coli harboring the beta-lactamase blaTEM-1B gene have been detected in Australian layer hens (120). Extended spectrum beta-lactamase (ESBL) genes including CTX-M and SHV alleles have been reported in poultry E. coli isolates in Europe, United Kingdom, China and Nigeria (118). With the emergence of multidrug-resistant E. coli strains in poultry, a search for vaccines against APEC has been conducted.
Vaccines
The current strategy against APEC is based on good sanitation and hygiene, which seem to be adequate but insufficient to control colibacillosis in commercial setups. Given their antigenic variability, finding efficient vaccines to prevent APEC infections has proved challenging (121). The vaccine is deemed successful in protecting against APEC infections with homologous strains but less effective against heterologous strains (63). Various virulence-associated genes have recently been evaluated as vaccine candidates to prevent colibacillosis in layer hen (122–124). The use of a live attenuated Salmonella delivery system with a recombinant construct that harbors genes encoding different virulence factors of APEC induced a robust immune response to prevent colibacillosis (84, 125, 126). Despite that reports showed the efficacy of the ΔaroA vaccine and its potential to reduce the virulence of APEC, the large molecular diversity of APEC strains that have hampered its effectiveness (127, 128). A combination of several virulence-associated genes (VAG) is required to confer pathogenicity in APEC, and no single or sets of certain VAG are associated with APEC, hence making it difficult to designs vaccines that would target all APEC strains. With these challenges, control measures and strategies, including suitable housing infrastructure and dietary intervention, are deemed helpful in bolstering the immunity and survival capability in pullets and layers.
Non-antibiotic dietary interventions
Probiotics: bacteria and yeast
The use of probiotics as alternatives to antibiotics and immune modulators is gaining tremendous interest in the poultry industry. According to the FAO/WHO, probiotics are defined as “live microorganisms which when administered in adequate amounts confer a health benefit on the host” (129). Probiotic bacteria used in animal production are generally Gram-positive and belong to Bacillus spp. (B. cereus var. toyoi, B. licheniformis, B. subtilis), Enterococcus spp. (E. faecium), Lactobacillus spp. (L. acidophilus, L. casei, L. farciminis, L. plantarum, L. reuteri, L. rhamnosus), Pediococcus spp. (P. acidilactici), and Streptococcus spp., (S. infantarius). Lactobacillus spp., Enterococcus spp., Pediococcus spp., Bacillus spp. Saccharomyces are the most common probiotics used in the poultry industry to improve product safety, feed efficiency, bird health, performance, and immunity (116, 130). Bifidobacterium spp. probiotics are widely used in combination with Lactobacillus spp. and other combination products. Bifidobacterium directly increases secretions of IgA in the intestinal tracts and stimulates phagocytes and pancreatic elastase productions through secretion of the serine protease inhibitor serine-production. This pro-inflammatory response mechanism suggests Bifidobacterium spp. and serine productions participate in gut microbiota homeostasis (131). Furthermore, Bifidobacterium spp. produces lactate and acetate, which are later used as gut fermenters to produce butyrate and propionate that aid in improving gut health (132). A fiber-rich diet could lead to high acetate levels generated by beneficial gut bacteria such as Bifidobacterium to provide significant protective effects (133).
In laying hens, B. subtilis supplemented diets (0.5 g/kg) of laying hens promote growth performance and balance the gut microbiota by inhibiting E. coli and Clostridium perfringens colonization Table 3 (134). More studies have shown that Bacillus-based probiotics could decrease E. coli in chickens, by altering microbiota composition and community structure (121, 135, 136). Lei et al. (135), and Hetab et al. (136) demonstrated that a B. subtilis-supplemented diet bolsters the immunity of chicks and enhances the performance and egg quality in layers. Lactobacillus spp. is produced during the sugar metabolism process; lactate inhibits the growth of pathogenic bacteria by reducing intestinal pH or directly interfering with normal bacteria metabolism. Certain Lactobacillus spp. species produce bacteriocin compounds, which are bactericidal to pathogenic microbes such as APEC, Salmonella spp., and Clostridium spp. (137). However, the type of Lactobacillus probiotics supplemented in the diet, for instance, L. acidophilus probiotic-fed hens at 18 weeks, 5 months, and 7 months, yielded an extremely low number of coliforms, E. coli, Clostridium spp., and Staphylococci spp. in ceca and ileal contents (137, 138). Although the total anaerobes population was less affected by the probiotics.
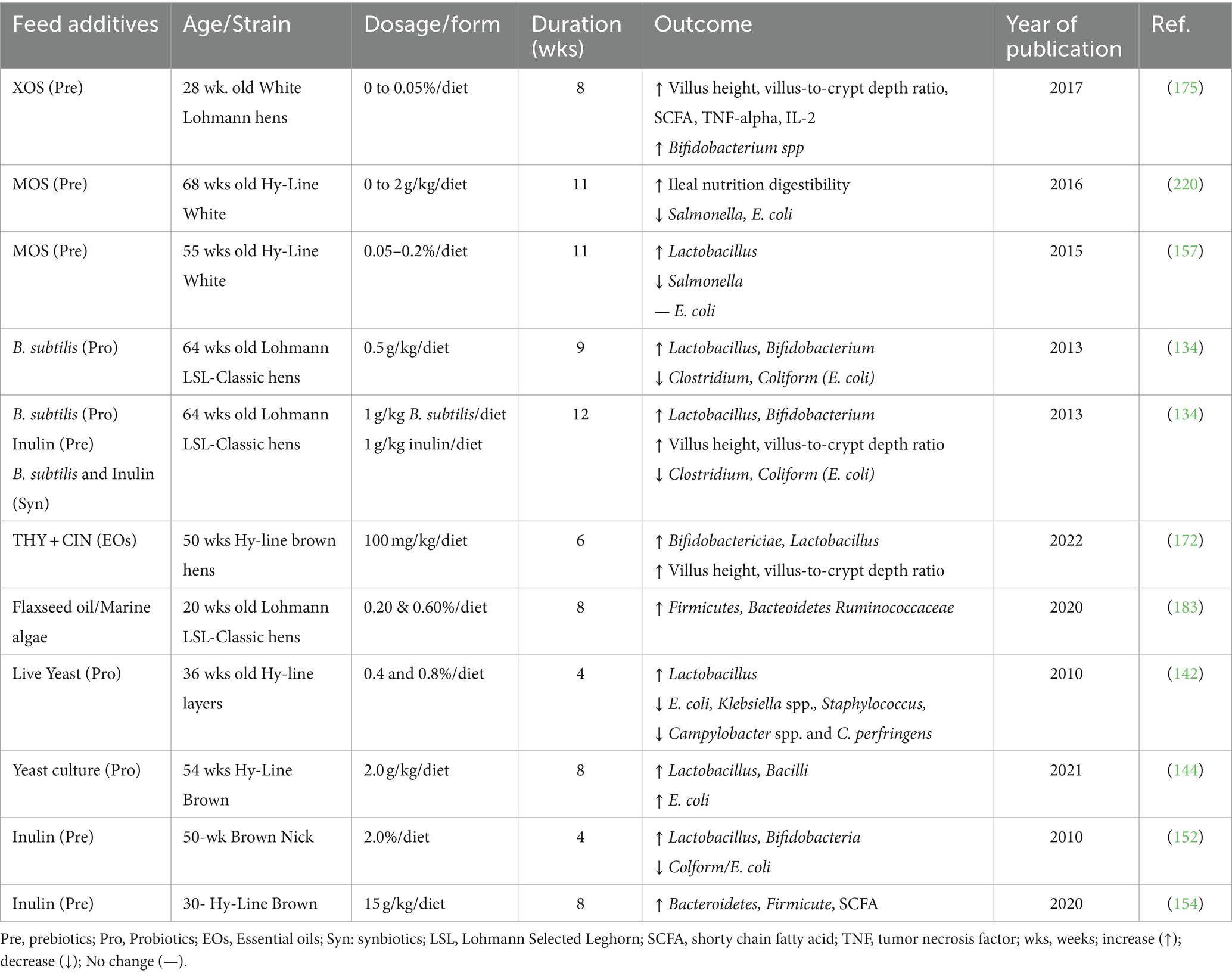
Table 3. Dietary inclusions of functional feed additives for laying hens and their effects on some gut microbiota and E. coli.
Saccharomyces cerevisiae is among the most yeast probiotics investigated for its potential beneficial effects in layers (139). Feeding pullets with diets supplemented with live yeast probiotic improves gut health and bolster immunity by increasing hematological profiles for total erythrocytes and leukocyte cell counts, marked by an increase in lymphocyte percentage (140). It has been found that the live yeast probiotics stimulated the immune system of pullets by increasing lymphocyte proliferation which helps increasing infection resistance in pullets (141). Dietary supplementation with live yeast probiotics (2.0 g/kg) for 8 weeks in laying hens has been shown to modulate the bird intestinal microflora by inhibiting the colonization of the GIT by enteric pathogens (E. coli, Salmonella, Campylobacter jejuni, and C. perfringens) (142), this promotes the immune response of the birds and enhances performance (143). Feeding poultry with live yeast supplements improves the ileum’s microbial population by increasing lactic acid bacteria and reducing E. coli, as shown in Table 3 (144).
Probiotics mechanism of actions include improving the microbial environment of a bird’s intestinal tract by displacing pathogenic microbes (145), competing for receptors on the gut mucosa necessary for attachment proliferation and colonization by beneficial microorganisms, which prevents the establishment of pathogens in the gut, and inhibiting growth of competitors by the production of primary and secondary (antimicrobial and immune modulation) metabolites (145). Dietary probiotics stimulate both cell-and humoral-mediated immunity through enhancing production cytokines/interferon, increased macrophages, lymphocytes, and natural killer cells (NK) activity, upregulated oxidative burst in heterophils, and increased immunoglobulins production/functions. In the gut, probiotics increase the number of lymphocytes and gut-associated lymphoid tissue in lamina propria and intra-epithelial lymphocytes, which could inhibit the growth of pathogenic organisms, especially during the transition from pullets to hens (38). However, stressors in poultry reduce the growth and functions of immune organs such as the bursa of Fabricius, thymus, and spleen (146), impairing immune responses and disease resistance (109).
Prebiotics
Prebiotics are defined as non-digestible ingredients that selectively promote the growth of beneficial bacteria in GIT, enhancing gut health and potentially improving the host’s health (111). Prebiotic compounds encompass numerous indigestible oligosaccharides such as fructooligosaccharides (FOS) products (oligofructose, inulin), trans-galactooligosaccharides (GOS), glycooligocharides, maltooligosaccharides, xylo-oligosaccharides (XOS), yeast cell wall (mannan oligosaccharides), glucooliogasaccharides and glycooligosacharids (115). The mechanism of actions of prebiotics in improving poultry health include pathogen’s inhibition by binding to the intestinal epithelium and generation of short-chain fatty acids (SCFA). The production of SCFA in the host intestine by prebiotic fermentation provides energy for epithelial cells, which reduces luminal pH and acts as the signaling molecule influencing the immune cells’ activities (147).
Dietary prebiotics have been shown to enhance the immunity in poultry. A significant upregulation of IL-10 (interleukin-10) was demonstrated in non-challenged Lohmann pullets fed yeast-derived carbohydrates for two weeks after post-hatch (148). This interleukin (IL-10) exerts its effects by modifying the immune response of different immune cells such as T, B, APC, and natural killer cells. Dietary beta-glucan has been shown to induce the expression of immune regulatory cytokines such as IL-10, transforming the growth factor-β1 and IL-2 in the bone marrow and spleen (149). Prebiotics (beta-glucan, FOS, inulin, and MOS) interact with pathogen-associated molecular pattern (PAMP), activating immune cells including macrophages, dendritic cells, and neutrophils, resulting in an increased the cytokines and other immune mediators’ production (150, 151). These activities of prebiotics can change the expression of genes associated with immune responses, enhancing birds’ ability to combat infections.
Dietary supplementation with inulin at 1, 1.5, and 2% has been shown to decrease the egg cholesterol contents in 50 to 54 weeks brown Nick laying hens (152). Furthermore, diet supplementation with inulin for 8 weeks in 30-weeks old Hy-Line brown laying hen induced an improved egg production and serum antioxidant activities in the group of 10, 15, and 20 g/kg (153), the improved egg production was due to an increased nutrient digestibility and the selective modulation of cecal microbial communities Table 3 (154). Prebiotics mannan oligosaccharides (MOS) are derived from yeast (S. cerevisiae); the mechanism of MOS in prevention against Gram-negative bacteria such as E. coli and Salmonella, is through to be through binding to type 1 fimbria (155). If the pathogen fails to bind to the receptor, they are flushed out constantly via excreta. In vitro studies have shown that additional MOS inhibits the attachment of enteropathogenic E. coli to the gut mucosa and removes the attached E. coli from the mucosa (156). In addition, FOS or MOS have been reported to decrease feed conversion, increase body weight gain, and egg production (157).
Feed enzymes (FEs) routinely used in poultry nutrition to aid in the hydrolysis of specific chemical bonds in feedstuffs, elimination of nutrients encapsulating effects of cell wall polysaccharides, resulting in the availability for absorption, and breakdown of feed antinutritional factors (158). Dietary inclusion with FEs in viscous feedstuffs could promoted growth performance, improved digestibility, and decreased feed cost. Short-chain xylo-oligosaccharides derived from in vitro hydrolysis of wheat bran by endoxylanases then fed to broilers resulted in an increased population of Bifidobacteria in the caeca and enhanced feed conversion ratio (159). Beta-mannanase modulates the feed-induced immune response and gastrointestinal ecology by hydrolyzing native beta-mannan to smaller fragments with reduced ability to stimulate the innate as demonstrated by the mucosal permeability, modulation of oxidative stress and concentration of acute phase protein and immunoglobulins in poultry (160).
Symbiotics
Prebiotics and probiotics combinations are known as symbiotics, which synergistically enhance gut health and immune functions (161). Studies have demonstrated the potential benefits of symbiotics on the intestinal microbiota and immune functions of pullets and laying hens (Table 3). The symbiotic mechanism of actions seems to be like prebiotics and probiotics (162). It is hypothesized that prebiotics and probiotics act independently in the gut. Prebiotics (indigestible oligosaccharides) are fermented in the gut, whereas probiotics (beneficial live microorganisms) colonize the gut. Moreover, other mechanisms of symbiotics might be to promote both pro-and anti-inflammatory responses in the host by regulating gut microbiota, increasing the population of beneficial bacteria, which up-regulates immune metabolic pathways and affects T cell maturation (163).
Lactobacillus salivarius and GOS have been shown to stimulate the gut-associated lymphocyte tissue (GALT) by T and B cells of broiler chicken (164). Al-Fataftah et al. (134) study has revealed that dietary inclusion of B. subtilis (1 g/kg) and inulin (1 g/kg) supplementation in (symbiotic) in laying hens improves eggshell quality, gut morphology, and growth of beneficial bacteria, as shown in Table 3. Bifidobacterium spp. and Lactobacillus spp. are some gut microbiotas that utilize inulin to ferment indigestible carbohydrates, produce SCFAs, stimulate immunoglobulin production, and help in competitive exclusions of pathogens by promoting the growth of beneficial bacteria (165). Dietary supplementation with inulin significantly increased Bifidobacterium spp., lowered pH, and reduced E. coli/coliform count in caeca of laying hens (152). Gut microbiota is pH sensitive; hence, its reduction is accompanied by changes in bacteria composition, such as inhibiting the proliferation of pathogenic E. coli and Salmonella spp. (165). Dietary supplementation with Lactobacillus spp. and S. cerevisiae derivatives increased Bifidobacterium and Lactobacillus populations in broiler’s gut while decreasing population of potentially pathogenic microbes such as Clostridium spp. and APEC (166). A study by Wareth (167) on Thymol and the symbiotics (Enterococcus faecium and fructooligosaccharides), alone or in combination in diet, improved egg production, egg mass, and feed conversion ratio from 24 to 36 weeks of age.
Phytogenics
Phytogenic feed additives, also known as botanicals or phytobiotics/phytochemicals are derived from plants and utilized in animal nutrition to promote growth and health (168). They include herbs, spices, other plants, or their extracts, such as essential oils (EOs). Phytobiotic compounds can be grouped into five categories: phenolic, alkaloids, nitrogen-containing agents, phytosterols, and carotenoids, each acting differently in improving the poultry immunity and health (169). Their actions include inhibiting microorganisms, disrupting metabolic processes, modulating signal transduction pathways, and immunomodulation via gene expressions (65). Phytobiotics could decrease intestinal inflammation and improve barrier functions by inhibiting toll-like receptors and subsequent activate NF-kb, the xenobiotics detoxifying system and the nuclear factor erythroid-related factor 2 (Nrf2) pathway as well as inhibit pathogenic bacteria. This enhancement of intestinal functions prevents the translocation of pathogens and harmful substances into the bloodstream by induction of systemic inflammation via excess secretion of cytokine and glucocorticoids (169). Dietary supplementation with cranberry pomace and extract resulted in upregulation of anti-inflammatory IL-10 in broilers (170). This interleukin (cytokine) also is involved in improving immune response including immunoglobulin production, increase NK cells and CD8+ T cells cytotoxic activities, as well as the thymocytes proliferation (171). These could help explain the decreased gut enteric bacteria including E. coli counts following berry products feeding as reported by Das et al. (170).
Previous studies on dietary EOs (thyme, rosemary, garlic, and sage) in laying hens (Table 3) reported improved performance, enhanced immune response, and promoted the growth of beneficial bacteria (172–174). Dietary inclusions of EOs mixtures at 24 mg/kg improved egg production, feed efficiency, hens while reducing the percentage of cracked eggs (175). The effectiveness of EOs’ depends on chemical structure, pH, concentration of bioactive compounds, and microbial population and strains present (176). EOs are considered more effective against Gram-negative than Gram-positive bacteria because their outer membrane is enclosed by a cell wall that restricts entry of hydrophobic compounds by lipopolysaccharides cell structure. Most EOs promote the growth of beneficial bacteria in the gut and moderate pathogenic bacteria in poultry, for example, Clostridium perfringens and E. coli (177). EOs from Thymus and Origanum-species have a high antimicrobial activity associated with their phenolic compounds such as thymol and carvacrol. The combination of EOs eugenol/carvacrol and eugenol/thymol showed a synergistic effect, whereby thymols and carvacrol disintegrated the outer membrane of E. coli, making it easier for eugenol to access bacterial cytoplasm to inhibit their growth (31). It has been reported that dietary encapsulated cinnamaldehyde could influence AMR virulence of E. coli in broiler however, more research would be needed to establish the involved mechanisms (178).
Fatty acids
Fatty acids are essential components of diets which are crucial in energy provision, cell structure, and regulation of body functions. Fats are the building blocks for fatty acids and are categorized based on their chemical structure and properties: saturated fatty acids have no double-bonds, monosaturated fatty acids (MUFA) have one double-bonds between carbon atoms, and polyunsaturated fatty acids (PUFA) have multiple bonds between carbon atoms (179). Dietary inclusion of n-3 PUFA has gained interest due to its potential health benefits by enhancing immunity in broiler chickens. Higher antibody titer against Newcastle disease virus were observed in 60-week n-3 PUFA fed-hen than the control corn-based diet (180). Similarly, feeding pullets breeders with n-3 PUFA (DHA and ALA) elevated embryogenic utilization of docosahexaenoic acid (DHA) and antibody titers against Newcastle and infectious bronchitis disease during the post-vaccination period (181, 182). However, further studies are needed to ascertain whether the antibody titer depends on the dosage or source of n-3 PUFA.
Dietary flaxseed oil (0.20 and 0.60%) in laying hens has been shown to promote the growth of beneficial bacteria, as shown in Table 3 (183). Feeding 46-weeks Lohmann layers with algae oil increased the concentration of DHA with no effects on egg production and eggshell quality (184). However, more studies are required to understand the correlation between gut microbiota, egg production, and enrichment in DHA. Effects of increasing concentration of n-3 PUFA (0.2, 0.4, 0.6, and 0.8%) from either flaxseeds oil or preformed docosahexaenoic acid on fatty acid composition and immune response of 20-week of age LSL lite laying hen, challenged with E coli-derived LPS (8 mg/kg via intravenous injection) were investigated (185). These authors showed that LPS increased the mRNA expression of proinflammatory cytokine IFN-γ and receptor TLR-4 (185). Similarly, n-3 FA was found to influence LSL lite pullets’ body weight, development of lymphoid organs, and some plasma metabolites (67). Wang et al. (186), found that dietary supplementation of n-3 PUFA alters lymphocyte subset proportion and immune tissue development of chick by promoting the growth of the spleen, thymus, and bursa of Fabricius before 4 weeks. The bursa withers or becomes damaged during 4 to 8 weeks of age. Despite variations in formulation and dosage, these findings suggest a strong link between n-3 PUFA acid and immune development, indicating its potential immunomodulatory feature as feed additives in pullets and laying hens. However, long-term effects induced by dietary n-3 PUFA on chicken immunity, resistance to relevant infectious challenges, and chicken performance remain the subject of research.
Challenges of nutritional interventions and future direction
Heat treatment during feed pelleting at the feed mill does not totally kill bacteria such as E. coli and Salmonella. The bacteria might be present in the final feed, representing a potential risk of transmission via feed (187, 188). A fiber-rich diet results in higher production of butyrate by the intestinal microbiota, which promotes the expression of the host’s globotriaosylceramide, an enterocyte receptor for the Shiga toxins (189). Therefore, promoting the growth of acetate-generating bacteria by dietary interventions could be a valuable strategy in mitigating the risk and severity of toxin-mediated diseases.
Dietary supplements have shown inconsistency in improving the performance and health of pullets and hen’s response which could be influenced by of dosage, age, sex, and breeds. As discussed earlier, a broad array of possibilities contributes to variation in feed supplement efficacy. Feed additives have pleiotropic actions including competitive exclusion, promoting growth of beneficial bacterial and producing antimicrobial metabolites. More detailed information is needed to help understand how combining nutritional intervention with optimal age-specific dosage, improved biosecurity, vaccination, and other health management practices in pullets and laying hens can provide a synergistic effect, reducing the overreliance on single strategies, and this might help in improving the health of laying hens and reducing APEC associated infections.
The restriction of AMU in poultry production and the search of other alternative strategies could have a complex impact on AMR in APEC infections (190). Alternatives, such as prebiotics, probiotics, and phytogenic, among other feed additives, cannot completely protect against pathogens, potentially resulting in increased use of therapeutic antibiotics. Thus, controlling measures to mitigate AMR must include enhanced biosecurity measures, responsible AMU, and further research in dietary interventions is required (191). The findings from breeder grandparent flocks have established them to be a possible source and reservoir for APEC strains (192). These strains can harbor and transmit E. coli vertically down the breeding pyramid to their progeny (192). A thorough and comparative analysis of the E. coli genome is necessary to ascertain it. Additionally, a longitudinal and molecular diversity study of the E. coli genome is required to evaluate and understand how the rearing environment and dietary supplements contribute to shaping adaptation and transmission of colibacillosis-associated E. coli in layers production system (192).
Conclusion
Colibacillosis induced by APEC affects pullets and hens of all age groups. The risks of infection with APEC and its evolution increase with the increased environmental pressure (housing, density, bird immunity, among other stressors). Hatchery hygiene, a good housing environment, and early feeding to boost immunity are important in controlling colibacillosis. Dietary supplementation with functional feed additives benefits pullets and layer hens. It results in improved egg production, bolsters immunity, promotes a healthy gut, and protects against colibacillosis. However, there is a need to standardize the dosage, breed, time, health status, and age of birds while administering these functional feed additives as management strategies for colibacillosis. Avian colibacillosis in chicks causes high mortality in the first week after hatching; it may be logical to introduce feed additives (probiotics, prebiotics, symbiotics and phytobiotics) early in the life of chicks, as this would help bolster immunity and promote a healthy gut. Improved gut health aided by gut microbiota plays a significant role in disease dynamics, host-pathogen interaction, host-commensal interaction, and commensal pathogen interaction in the GIT of the birds under dietary supplemented with functional feed additives. Furthermore, the development of sequencing technologies and bioinformatics pipelines would also make it possible to delineate some of the complex associations between the wide range of feed additives and poultry GIT microbial response to dietary feed additive as well as to identify drug and/or vaccine target against APEC.
Author contributions
PW: Writing – original draft, Conceptualization. EK: Funding acquisition, Supervision, Writing – review & editing. MD: Conceptualization, Funding acquisition, Supervision, Writing – review & editing.
Funding
The author(s) declare that financial support was received for the research, authorship, and/or publication of this article. This work was supported by the Natural Sciences and Engineering Research Council of Canada (NSERC) - Alliance programs, the Ontario Agri-Food Innovation Alliance, Egg Farmers of Canada, Egg Farmers of Ontario, CBS Bio Platforms Inc., and O & T Farms Ltd. and the Canadian Federal Research an Development Initiative Research and Development Initiative on AMR (GRDI-AMR mitigation project through Agriculture and Agri-Food Canada. The authors declare that this study received funding from CBS Bio Platforms Inc and O & T Farms Ltd. The funders were not involved in the study design, collection, analysis, interpretation of data, the writing of this article, or the decision to submit it for publication.
Acknowledgments
The authors would like to thank Anderson N. Maina, Felix N. Muchiri and Xianhua Yin for technical assistances.
Conflict of interest
The authors declare that the research was conducted in the absence of any commercial or financial relationships that could be construed as a potential conflict of interest.
Publisher’s note
All claims expressed in this article are solely those of the authors and do not necessarily represent those of their affiliated organizations, or those of the publisher, the editors and the reviewers. Any product that may be evaluated in this article, or claim that may be made by its manufacturer, is not guaranteed or endorsed by the publisher.
References
1. Ehrlich, PR, and Harte, J. Opinion: to feed the world in 2050 will require a global revolution. Proc Natl Acad Sci U S A. (2015) 112:14743–4. doi: 10.1073/pnas.1519841112
2. Gateway to Poultry Production and Products. Food and Agriculture Organization of the United Nations. (2024). Available at: https://www.fao.org/poultry-production-products/en/ (Accessed June 28, 2024).
3. Mageiros, L, Méric, G, Bayliss, SC, Pensar, J, Pascoe, B, Mourkas, E, et al. Genome evolution and the emergence of pathogenicity in avian Escherichia coli. Nat Commun. (2021) 12:1–13. doi: 10.1038/s41467-021-20988-w
4. Olsen, RH, Frantzen, C, Christensen, H, and Bisgaard, M. An investigation on first-week mortality in layers (2012) 7:e56–7. doi: 10.1637/10031-977711-DIGEST.1,
5. Vaccines. Special Issue: Poultry Infectious Diseases: Immunity and microbiota. (2024). Available at: https://www.mdpi.com/journal/vaccines/special_issues/Poultry_Immunity (Accessed June 28, 2024).
6. Lay, DC, Fulton, RM, Hester, PY, Karcher, DM, Kjaer, JB, Mench, JA, et al. Hen welfare in different housing systems. Poult Sci. (2011) 90:278–94. doi: 10.3382/PS.2010-00962
7. Mottet, A, and Tempio, G. Global poultry production: current state and future outlook and challenges. Worlds Poult Sci J. (2017) 73:245–56. doi: 10.1017/S0043933917000071
8. Hofmann, T, Schmucker, SS, Bessei, W, Grashorn, M, and Stefanski, V. Impact of housing environment on the immune system in chickens: a review. Animals (Basel). (2020) 10:1–26. doi: 10.3390/ANI10071138
10. Fossum, O, Jansson, DS, Etterlin, PE, and Vgsholm, I. Causes of mortality in laying hens in different housing systems in 2001 to 2004. Acta Vet Scand. (2009) 51:1–9. doi: 10.1186/1751-0147-51-3/TABLES/6
11. National Farm Animal Care Council. Codes of Practice for the care and handling of farm animals. (2024). Available at: https://www.nfacc.ca/codes-of-practice (Accessed June 28, 2024).
12. Van Staaveren, N, Decina, C, Baes, CF, Widowski, TM, Berke, O, and Harlander-Matauschek, A. Housing and management practices on 33 pullet farms in Canada. Animals. (2019) 9:49. doi: 10.3390/ANI9020049
13. Guabiraba, R, and Schouler, C. Avian colibacillosis: still many black holes. FEMS Microbiol Lett. (2015) 362:118. doi: 10.1093/femsle/fnv118
14. Stromberg, ZR, Johnson, JR, Fairbrother, JM, Kilbourne, J, Van Goor, A, Curtiss, R, et al. Evaluation of Escherichia coli isolates from healthy chickens to determine their potential risk to poultry and human health. PLoS One. (2017) 12:e0180599. doi: 10.1371/journal.pone.0180599
15. Fancher, CA, Thames, HT, Colvin, MG, Smith, M, Easterling, A, Nuthalapati, N, et al. Prevalence and molecular characteristics of avian pathogenic Escherichia coli in “no antibiotics ever” broiler farms. Microbiol Spectr. (2021) 9:e0083421. doi: 10.1128/Spectrum.00834-21
16. Swelum, AA, El-Saadony, MT, Abd El-Hack, ME, Abo Ghanima, MM, Shukry, M, Alhotan, RA, et al. Ammonia emissions in poultry houses and microbial nitrification as a promising reduction strategy. Sci Total Environ. (2021) 781:146978. doi: 10.1016/J.SCITOTENV.2021.146978
17. Alberdi, O, Arriaga, H, Calvet, S, Estellés, F, and Merino, P. Ammonia and greenhouse gas emissions from an enriched cage laying hen facility. Biosyst Eng. (2016) 144:1–12. doi: 10.1016/J.BIOSYSTEMSENG.2016.01.009
18. Rehman, MA, Rempel, H, Carrillo, CD, Ziebell, K, Allen, K, Manges, AR, et al. Virulence genotype and phenotype of multiple antimicrobial-resistant Escherichia coli isolates from broilers assessed from a “one-health” perspective. J Food Prot. (2022) 85:273. doi: 10.4315/JFP-21-273
19. Aslam, M, Toufeer, M, Narvaez Bravo, C, Lai, V, Rempel, H, Manges, A, et al. Characterization of Extraintestinal pathogenic Escherichia coli isolated from retail poultry meats from Alberta, Canada. Int J Food Microbiol. (2014) 177:49–56. doi: 10.1016/J.IJFOODMICRO.2014.02.006
20. Swelum, AA, Elbestawy, AR, El-Saadony, MT, Hussein, EOS, Alhotan, R, Suliman, GM, et al. Ways to minimize bacterial infections, with special reference to Escherichia coli, to cope with the first-week mortality in chicks: an updated overview. Poult Sci. (2021) 100:101039. doi: 10.1016/j.psj.2021.101039
21. Spalding, M. Diseases of poultry, 12th edition. J Wildl Dis. (2009) 45:251. doi: 10.7589/0090-3558-45.1.251
22. Merk Manual Veterinary Manual. Colibacillosis in Poultry - Poultry - Merck Veterinary Manual. Available at: https://www.merckvetmanual.com/poultry/colibacillosis/colibacillosis-in-poultry (Accessed June 29, 2024).
23. Giovanardi, D, Campagnari, E, Sperati Ruffoni, L, Pesente, P, Ortali, G, and Furlattini, V. Avian pathogenic Escherichia coli transmission from broiler breeders to their progeny in an integrated poultry production chain. Avian Pathol. (2005) 34:313–8. doi: 10.1080/03079450500179046
24. Karunarathna, R, Ahmed, KA, Goonewardene, K, Gunawardana, T, Kurukulasuriya, S, Liu, M, et al. Exposure of embryonating eggs to Enterococcus faecalis and Escherichia coli potentiates E. coli pathogenicity and increases mortality of neonatal chickens. Poult Sci. (2022) 101:101983. doi: 10.1016/J.PSJ.2022.101983
25. Molenaar, R, Stockhofe-Zurwieden, N, Giersberg, MF, Rodenburg, TB, Kemp, B, van den Brand, H, et al. Effects of hatching system on chick quality, welfare and health of young breeder flock offspring. Poult Sci. (2023) 102:102448. doi: 10.1016/J.PSJ.2022.102448
26. Paudel, S, Fink, D, Abdelhamid, MK, Zöggeler, A, Liebhart, D, Hess, M, et al. Aerosol is the optimal route of respiratory tract infection to induce pathological lesions of colibacillosis by a lux-tagged avian pathogenic Escherichia coli in chickens. Avian Pathol. (2021) 50:417–26. doi: 10.1080/03079457.2021.1978392/ASSET/149E0C96-0FCB-48FF-A9AF-BD6FC86B5273/ASSETS/IMAGES/CAVP_A_1978392_F0004_OB.JPG
27. Landman, WJM, Heuvelink, A, and van Eck, JHH. Reproduction of the Escherichia coli peritonitis syndrome in laying hens. Avian Pathol. (2013) 42:157–62. doi: 10.1080/03079457.2013.775694
28. de Oliveira, AL, Newman, DM, Sato, Y, Noel, A, Rauk, B, Nolan, LK, et al. Characterization of avian pathogenic Escherichia coli (APEC) associated with Turkey cellulitis in Iowa. Front Vet Sci. (2020) 7:550217. doi: 10.3389/FVETS.2020.00380/BIBTEX
29. Desvaux, M, Dalmasso, G, Beyrouthy, R, Barnich, N, Delmas, J, and Bonnet, R. Pathogenicity factors of Genomic Islands in intestinal and Extraintestinal Escherichia coli. Front Microbiol. (2020) 11:2065. doi: 10.3389/fmicb.2020.02065
30. Bessaiah, H, Anamalé, C, Sung, J, and Dozois, CM. What flips the switch? Signals and stress regulating extraintestinal pathogenic Escherichia coli type 1 fimbriae (pili). Microorganisms. (2022) 10:5. doi: 10.3390/microorganisms10010005
31. Hu, J, Afayibo, DJA, Zhang, B, Zhu, H, Yao, L, Guo, W, et al. Characteristics, pathogenic mechanism, zoonotic potential, drug resistance, and prevention of avian pathogenic Escherichia coli (APEC). Front Microbiol. (2022) 13:1049391. doi: 10.3389/FMICB.2022.1049391/BIBTEX
32. Tapader, R, Basu, S, and Pal, A. Secreted proteases: a new insight in the pathogenesis of extraintestinal pathogenic Escherichia coli. Int J Med Microbiol. (2019) 309:159–68. doi: 10.1016/J.IJMM.2019.03.002
33. Wang, S, Niu, C, Shi, Z, Xia, Y, Yaqoob, M, Dai, J, et al. Effects of ibeA deletion on virulence and biofilm formation of avian pathogenic Escherichia coli. Infect Immun. (2011) 79:279–87. doi: 10.1128/IAI.00821-10/ASSET/3D85B272-BAE8-4FD9-B4F9-2431B7AD5C2A/ASSETS/GRAPHIC/ZII9990989350005.JPEG
34. Kathayat, D, Lokesh, D, Ranjit, S, and Rajashekara, G. Avian pathogenic Escherichia coli (APEC): An overview of virulence and pathogenesis factors, zoonotic potential, and control strategies. Pathogens. (2021) 10:467. doi: 10.3390/PATHOGENS10040467
35. Garcia, P, Wang, Y, Viallet, J, and Macek, JZ. The chicken embryo model: a novel and relevant model for immune-based studies. Front Immunol. (2021) 12:791081. doi: 10.3389/FIMMU.2021.791081/BIBTEX
36. Alkie, TN, Yitbarek, A, Hodgins, DC, Kulkarni, RR, Taha-Abdelaziz, K, and Sharif, S. Development of innate immunity in chicken embryos and newly hatched chicks: a disease control perspective. Avian Pathol. (2019) 48:288–310. doi: 10.1080/03079457.2019.1607966
37. Juul-Madsen, HR, Norup, LR, Jørgensen, PH, Handberg, KJ, Wattrang, E, and Dalgaard, TS. Crosstalk between innate and adaptive immune responses to infectious bronchitis virus after vaccination and challenge of chickens varying in serum mannose-binding lectin concentrations. Vaccine. (2011) 29:9499–507. doi: 10.1016/J.VACCINE.2011.10.016
38. Schmucker, S, Hofmann, T, Sommerfeld, V, Huber, K, Rodehutscord, M, and Stefanski, V. Immune parameters in two different laying hen strains during five production periods. Poult Sci. (2021) 100:101408. doi: 10.1016/J.PSJ.2021.101408
39. Nguyen, TTT, Shahin, K, Allan, B, Sarfraz, M, Wheler, C, Gerdts, V, et al. Enhancement of protective efficacy of innate immunostimulant based formulations against yolk sac infection in young chicks. Poult Sci. (2022) 101:102119. doi: 10.1016/J.PSJ.2022.102119
40. Lammers, A, Wieland, WH, Kruijt, L, Jansma, A, Straetemans, T, Schots, A, et al. Successive immunoglobulin and cytokine expression in the small intestine of juvenile chicken. Dev Comp Immunol. (2010) 34:1254–62. doi: 10.1016/J.DCI.2010.07.001
41. Jibril, AH, Okeke, IN, Dalsgaard, A, and Olsen, JE. Prevalence and whole genome phylogenetic analysis reveal genetic relatedness between antibiotic resistance Salmonella in hatchlings and older chickens from farms in Nigeria. Poult Sci. (2023) 102:102427. doi: 10.1016/J.PSJ.2022.102427
42. Osman, KM, Kappell, AD, Elhadidy, M, Elmougy, F, El-Ghany, WAA, Orabi, A, et al. Poultry hatcheries as potential reservoirs for antimicrobial-resistant Escherichia coli: a risk to public health and food safety. Sci Rep. (2018) 8:1–14. doi: 10.1038/s41598-018-23962-7
43. Kemmett, K, Williams, NJ, Chaloner, G, Humphrey, S, Wigley, P, and Humphrey, T. The contribution of systemic Escherichia coli infection to the early mortalities of commercial broiler chickens. Avian Pathol. (2013) 43:37–42. doi: 10.1080/03079457.2013.866213
44. Poulsen, LL, Thøfner, I, Bisgaard, M, Christensen, JP, Olsen, RH, and Christensen, H. Longitudinal study of transmission of Escherichia coli from broiler breeders to broilers. Vet Microbiol. (2017) 207:13–8. doi: 10.1016/J.VETMIC.2017.05.029
45. Hartcher, KM, and Jones, B. The welfare of layer hens in cage and cage-free housing systems. Worlds Poult Sci J. (2017) 73:767–82. doi: 10.1017/S0043933917000812
46. Rodenburg, TB, Tuyttens, FAM, Sonck, B, De Reu, K, Herman, L, and Zoons, J. Welfare, health, and hygiene of laying hens housed in furnished cages and in alternative housing systems. J Appl Anim Welf Sci. (2005) 8:211–26. doi: 10.1207/S15327604JAWS0803_5
47. Bilal, RM, Hassan, F, Farag, MR, Nasir, TA, Ragni, M, Mahgoub, HAM, et al. Thermal stress and high stocking densities in poultry farms: potential effects and mitigation strategies. J Therm Biol. (2021) 99:102944. doi: 10.1016/J.JTHERBIO.2021.102944
48. Lutful Kabir, SM. Avian Colibacillosis and salmonellosis: a closer look at epidemiology, pathogenesis, diagnosis, control and public health concerns. Int J Environ Res Public Health. (2010) 7:89–114. doi: 10.3390/IJERPH7010089
49. Paura, L, Arhipova, I, Jankovska, L, Bumanis, N, Vitols, G, and Adjutovs, M. Evaluation and association of laying hen performance, environmental conditions and gas concentrations in barn housing system. Ital J Anim Sci. (2022) 21:694–701. doi: 10.1080/1828051X.2022.2056528
50. Kirychuk, SP, Reynolds, SJ, Koehncke, NK, Lawson, J, Willson, P, Senthilselvan, A, et al. Endotoxin and dust at respirable and nonrespirable particle sizes are not consistent between cage-and floor-housed poultry operations. Ann Occup Hyg. (2010) 54:824–32. doi: 10.1093/ANNHYG/MEQ047
51. Gonzalez-Mora, AF, Rousseau, AN, Larios, AD, Godbout, S, and Fournel, S. Assessing environmental control strategies in cage-free aviary housing systems: egg production analysis and random Forest modeling. Comput Electron Agric. (2022) 196:106854. doi: 10.1016/J.COMPAG.2022.106854
52. Zhou, Y, Zhang, M, Liu, Q, and Feng, J. The alterations of tracheal microbiota and inflammation caused by different levels of ammonia exposure in broiler chickens. Poult Sci. (2021) 100:685–96. doi: 10.1016/J.PSJ.2020.11.026
53. Li, D, Tong, Q, Shi, Z, Li, H, Wang, Y, Li, B, et al. Effects of chronic heat stress and ammonia concentration on blood parameters of laying hens. Poult Sci. (2020) 99:3784–92. doi: 10.1016/J.PSJ.2020.03.060
54. Mench, JA, and Rodenburg, TB. Sustainability of laying hen housing systems In: JA Mench, editor. Advances in Poultry Welfare. Sawston, UK: Woodhead Publishing (2018). 199–225.
55. Weimer, SL, Robison, CI, Tempelman, RJ, Jones, DR, and Karcher, DM. Laying hen production and welfare in enriched colony cages at different stocking densities. Poult Sci. (2019) 98:3578–86. doi: 10.3382/PS/PEZ107
56. Widowski, TM, Hemsworth, PH, Barnett, JL, and Rault, JL. Laying hen welfare I. Social environment and space. Worlds Poult Sci J. (2016) 72:333–42. doi: 10.1017/S0043933916000027
57. Cheng, HW, Freire, R, and Pajor, EA. Endotoxin stress responses in chickens from different genetic lines. 1. Sickness, behavioral, and physical responses. Poult Sci. (2004) 83:707–15. doi: 10.1093/PS/83.5.707
58. Patterson, PH, and Siegel, HS. Impact of cage density on pullet performance and blood parameters of stress. Poult Sci. (1998) 77:32–40. doi: 10.1093/PS/77.1.32
59. Heckert, RA, Estevez, I, Russek-Cohen, E, and Pettit-Riley, R. Effects of density and perch availability on the immune status of broilers. Poult Sci. (2002) 81:451–7. doi: 10.1093/PS/81.4.451
60. Gast, RK, Jones, DR, Guraya, R, Garcia, JS, and Karcher, DM. Research note: internal organ colonization by Salmonella Enteritidis in experimentally infected layer pullets reared at different stocking densities in indoor cage-free housing. Poult Sci. (2022) 101:102104. doi: 10.1016/J.PSJ.2022.102104
61. Von Eugen, K, Nordquist, RE, Zeinstra, E, and van der Staay, FJ. Stocking density affects stress and anxious behavior in the laying hen chick during rearing. Animals. (2019) 9:53. doi: 10.3390/ANI9020053
62. Asakura, H, Tajima, O, Watarai, M, Shirahata, T, Kurazono, H, and Makino, SI. Effects of rearing conditions on the colonization of Salmonella Enteritidis in the cecum of chicks. J Vet Med Sci. (2001) 63:1221–4. doi: 10.1292/JVMS.63.1221
63. Vandekerchove, D, De Herdt, P, Laevens, H, and Pasmans, F. Risk factors associated with colibacillosis outbreaks in caged layer flocks. Avian Pathol. (2004) 33:337–42. doi: 10.1080/0307945042000220679
64. Lentfer, TL, Pendl, H, Gebhardt-Henrich, SG, Fröhlich, EKF, and Von Borell, E. H/L ratio as a measurement of stress in laying hens – methodology and reliability. Br Poult Sci. (2015) 56:157–63. doi: 10.1080/00071668.2015.1008993
65. Hofmann, T, Schmucker, S, Grashorn, M, and Stefanski, V. Short-and long-term consequences of stocking density during rearing on the immune system and welfare of laying hens. Poult Sci. (2021) 100:101243. doi: 10.1016/j.psj.2021.101243
66. Campo, JL, Gil, MG, Dávila, SG, and Muñoz, I. Influence of perches and footpad dermatitis on tonic immobility and heterophil to lymphocyte ratio of chickens. Poult Sci. (2005) 84:1004–9. doi: 10.1093/PS/84.7.1004
67. Lee, J, Cheng, V, and Kiarie, EG. Growth and response to Escherichia coli lipopolysaccharide challenge in Lohmann LSL-lite pullets when fed a source of omega-3 fatty acids and yeast bioactives from hatch through to 16 weeks of age. Poult Sci. (2023) 102:102940. doi: 10.1016/J.PSJ.2023.102940
68. Friedman, A, Meidovsky, A, Leitner, G, and Sklan, D. Decreased resistance and immune response to Escherichia coli infection in chicks with low or high intakes of vitamin a. J Nutr. (1991) 121:395–400. doi: 10.1093/JN/121.3.395
69. Alhenaky, A, Abdelqader, A, Abuajamieh, M, and Al-Fataftah, AR. The effect of heat stress on intestinal integrity and Salmonella invasion in broiler birds. J Therm Biol. (2017) 70:9–14. doi: 10.1016/J.JTHERBIO.2017.10.015
70. Kim, DH, Lee, YK, Lee, SD, and Lee, KW. Impact of relative humidity on the laying performance, egg quality, and physiological stress responses of laying hens exposed to high ambient temperature. J Therm Biol. (2022) 103:103167. doi: 10.1016/J.JTHERBIO.2021.103167
71. El-Naggar, K, El-Kassas, S, Abdo, SE, Kirrella, AAK, and Al Wakeel, RA. Role of gamma-aminobutyric acid in regulating feed intake in commercial broilers reared under normal and heat stress conditions. J Therm Biol. (2019) 84:164–75. doi: 10.1016/J.JTHERBIO.2019.07.004
72. Kammon, A, Alzentani, S, Tarhuni, O, and Asheg, A. Effect of some organic acids on body weight, immunity and cecal bacterial count of chicken during heat stress. Int J Poult Sci. (2019) 18:293–300. doi: 10.3923/IJPS.2019.293.300
73. Schreier, J, Rychlik, I, Karasova, D, Crhanova, M, Breves, G, Rautenschlein, S, et al. Influence of heat stress on intestinal integrity and the caecal microbiota during Enterococcus cecorum infection in broilers. Vet Res. (2022) 53:110. doi: 10.1186/S13567-022-01132-Y/FIGURES/8
74. Huff, GR, Huff, WE, Rath, NC, De Los, S, Santos, F, Farnell, MB, et al. Influence of hen age on the response of Turkey Poults to cold stress, Escherichia coli challenge, and treatment with a yeast extract antibiotic alternative. Poult Sci. (2007) 86:636–42. doi: 10.1093/PS/86.4.636
75. Surai, PF. Antioxidant Systems in Poultry Biology: superoxide dismutase. J Anim Res Nutr. (2015) 1:8. doi: 10.21767/2572-5459.100008
76. da Rosa, G, Da Silva, AS, Souza, CF, Baldissera, MD, Mendes, RE, Araujo, DN, et al. Impact of colibacillosis on production in laying hens associated with interference of the phosphotransfer network and oxidative stress. Microb Pathog. (2019) 130:131–6. doi: 10.1016/J.MICPATH.2019.03.004
77. Mitchell, MA. Chick transport and welfare. Avian Biol Res. (2009) 2:99–105. doi: 10.3184/175815509X431894/ASSET/175815509X431894.FP.PNG_V03
78. Schwartzkopf-Genswein, KS, Faucitano, L, Dadgar, S, Shand, P, González, LA, and Crowe, TG. Road transport of cattle, swine and poultry in North America and its impact on animal welfare, carcass and meat quality: a review. Meat Sci. (2012) 92:227–43. doi: 10.1016/J.MEATSCI.2012.04.010
79. Watts, JM, Graff, LJ, Strawford, ML, Crowe, TG, Burlinguette, NA, Classen, HL, et al. Heat and moisture production by broilers during simulated cold weather transport. Poult Sci. (2011) 90:1890–9. doi: 10.3382/PS.2010-01314
80. Rioja-Lang, FC, Galbraith, JK, McCorkell, RB, Spooner, JM, and Church, JS. Review of priority welfare issues of commercially raised bison in North America. Appl Anim Behav Sci. (2019) 210:1–8. doi: 10.1016/J.APPLANIM.2018.10.014
81. Ericsson, M, Henriksen, R, Bélteky, J, Sundman, AS, Shionoya, K, and Jensen, P. Long-term and transgenerational effects of stress experienced during different life phases in chickens (Gallus gallus). PLoS One. (2016) 11:e0153879. doi: 10.1371/JOURNAL.PONE.0153879
82. Peixoto, MRLV, Cooley, L, and Widowski, TM. Maternal age and maternal environment affect stress reactivity and measures of social behaviour in laying hens. Sci Rep. 11:17499. doi: 10.1038/s41598-021-96323-6
83. Unniford, ME, and Widowski, TM. Rearing environment and laying location affect pre-laying behaviour in enriched cages. Applied Animal Behaviour Science. (2016) 181:205–213. doi: 10.1016/j.applanim.2016.0
84. Lynne, AM, Kariyawasam, S, Wannemuehler, Y, Johnson, TJ, Johnson, SJ, Sinha, AS, et al. Recombinant Iss as a potential vaccine for avian Colibacillosis (2012) 56:192–9. doi: 10.1637/9861-072111-REG.1,
85. Bryden, WL, Li, X, Ruhnke, I, Zhang, D, and Shini, S. Nutrition, feeding and laying hen welfare. Anim Prod Sci. (2021) 61:893–914. doi: 10.1071/AN20396
86. Pines, M, and Reshef, R. Poultry bone development and bone disorders. In: CG Scanes. Sturkie’s Avian Physiology. 6th Edn., pp. 367–377. (2015).
87. Yokhana, JS, Parkinson, G, and Frankel, TL. Effect of insoluble fiber supplementation applied at different ages on digestive organ weight and digestive enzymes of layer-strain poultry. Poult Sci. (2016) 95:550–9. doi: 10.3382/PS/PEV336
88. Kaiser, MG, Block, SS, Ciraci, C, Fang, W, Sifri, M, and Lamont, SJ. Effects of dietary vitamin E type and level on lipopolysaccharide-induced cytokine mRNA expression in broiler chicks. Poult Sci. (2012) 91:1893–8. doi: 10.3382/PS.2011-02116
89. Calder, PC. The relationship between the fatty acid composition of immune cells and their function. Prostaglandins Leukot Essent Fatty Acids. (2008) 79:101–8. doi: 10.1016/J.PLEFA.2008.09.016
90. Arthur, JR, McKenzie, RC, and Beckett, GJ. Selenium in the immune system. J Nutr. (2003) 133:1457S–9S. doi: 10.1093/JN/133.5.1457S
91. Bin, KY, Yoon, MY, Ha, JS, Seo, KW, Noh, EB, Son, SH, et al. Molecular characterization of avian pathogenic Escherichia coli from broiler chickens with colibacillosis. Poult Sci. (2020) 99:1088–95. doi: 10.1016/J.PSJ.2019.10.047
92. Kasimanickam, V, Kasimanickam, M, and Kasimanickam, R. Antibiotics use in food animal production: escalation of antimicrobial resistance: where are we now in combating AMR? Med Sci. (2021) 9:14. doi: 10.3390/MEDSCI9010014
93. Wang, C, Pors, SE, Christensen, JP, Bojesen, AM, and Thøfner, I. Comparison and assessment of necropsy lesions in end-of-lay laying hens from different housing systems in Denmark. Poult Sci. (2020) 99:119–28. doi: 10.3382/PS/PEZ569
94. Harisberger, M, Gobeli, S, Hoop, R, Dewulf, J, Perreten, V, and Regula, G. Antimicrobial resistance in Swiss laying hens, prevalence and risk factors. Zoonoses Public Health. (2011) 58:377–87. doi: 10.1111/J.1863-2378.2010.01376.X
95. Kaufmann-Bat, M, and Hoop, RK. Diseases in chicks and laying hens during the first 12 years after battery cages were banned in Switzerland. Vet Rec. (2009) 164:203–7. doi: 10.1136/VR.164.7.203
96. Van Hoorebeke, S, Van Immerseel, F, Berge, AC, Persoons, D, Schulz, J, Hartung, J, et al. Antimicrobial resistance of Escherichia coli and Enterococcus faecalis in housed laying-hen flocks in Europe. Epidemiol Infect. (2011) 139:1610–20. doi: 10.1017/S0950268810002700
97. Agunos, A, Gow, SP, Léger, DF, Flockhart, L, Daignault, D, Desruisseau, A, et al. Antimicrobial resistance and recovery of Salmonella, Campylobacter, and Escherichia coli from chicken egg layer flocks in Canadian sentinel surveillance sites using 2 types of sample matrices. Can J Vet Res. (2021) 85:27–35. doi: 10.1016/j.ijfoodmicro.2023.110541
98. Singer, RS. Estimates of on-farm antimicrobial usage in egg production in the United States, 2016–2021. Front Vet Sci. (2023) 10:1135377. doi: 10.3389/FVETS.2023.1135377/BIBTEX
99. Sophie, S. UK veterinary antibiotic resistance and sales surveillance report – UK-VARSS 2021, published November 2022. (2022). Available at: https://www.bing.com/search?pglt=41&q=Sophie+S.+UK+veterinary+antibiotic+resistance+and+sales+surveillance+report+%e2%80%93+UK-VARSS+2021%2c+published+November+2022.+(2022).+Available+at%3a+www.nationalarchives.gov.uk%2fdoc%2fopen-government-licence%2fversion%2f3%2foremailPSI%40nationalarchives&cvid=68b4e9b0b00e479883ccb1e618b60e48&gs_lcrp=EgZjaHJvbWUyBggAEEUYOTII (Accessed June 30, 2024).
100. Ćupić, VN, Ivanović, SR, Borozan, SZ, Mujezinović, IA, Ćupić-Miladinović, DV, and Aleksić, JZ. Antimicrobial agents in laying hens. Zb Matice Srp Prir Nauk. (2022) 142:61–71. doi: 10.2298/ZMSPN2242061C
101. Markley, JL, and Wencewicz, TA. Tetracycline-inactivating enzymes. Front Microbiol. (2018) 9:370057. doi: 10.3389/FMICB.2018.01058/BIBTEX
102. Duarte, R, Sofia, A, Høg, B, Bisgaard, H, Emilie, A, and Larsen, R. General rights DANMAP 2020 Use of antimicrobial agents and occurrence of antimicrobial resistance in bacteria from food animals, food and humans in Denmark Use of antimicrobial agents and occurrence of antimicrobial resistance in bacteria from food animals, food and humans in. (2021). Available at: www.danmap.org (Accessed June 30, 2024).
103. Moreno, MA, García-Soto, S, Hernández, M, Bárcena, C, Rodríguez-Lázaro, D, Ugarte-Ruíz, M, et al. Day-old chicks are a source of antimicrobial resistant bacteria for laying hen farms. Vet Microbiol. (2019) 230:221–7. doi: 10.1016/J.VETMIC.2019.02.007
104. Ljubojevic, D, Pelic, M, Puvača, N, and Milanov, D. Resistance to tetracycline in Escherichia coli isolates from poultry meat: epidemiology, policy and perspective. Worlds Poult Sci J. (2017) 73:409–17. doi: 10.1017/S0043933917000216
105. Jahantigh, M, Samadi, K, Dizaji, RE, and Salari, S. Antimicrobial resistance and prevalence of tetracycline resistance genes in Escherichia coli isolated from lesions of colibacillosis in broiler chickens in Sistan, Iran. BMC Vet Res. (2020) 16:1–6. doi: 10.1186/S12917-020-02488-Z/TABLES/3
106. Nogrado, K, Unno, T, Hur, HG, and Lee, JH. Tetracycline-resistant bacteria and ribosomal protection protein genes in soils from selected agricultural fields and livestock farms. Appl Biol Chem. (2021) 64:1–9. doi: 10.1186/S13765-021-00613-6/FIGURES/4
107. Santamaría, J, López, L, and Soto, CY. Detection and diversity evaluation of tetracycline resistance genes in grassland-based production systems in Colombia, South America. Front Microbiol. (2011) 2:15715. doi: 10.3389/FMICB.2011.00252/BIBTEX
108. Cox, GW, Parmley, EJ, Avery, BP, Irwin, RJ, Reid-Smith, RJ, Deckert, AE, et al. A one-health genomic investigation of gentamicin resistance in salmonella from human and chicken sources in Canada, 2014 to 2017. Antimicrob Agents Chemother. (2021) 65:966. doi: 10.1128/AAC.00966-21/SUPPL_FILE/AAC.00966-21-S0001.XLSX
109. Roushdy, EM, Zaglool, AW, and Hassan, FAM. Thermal stress consequences on growth performance, immunological response, antioxidant status, and profitability of finishing broilers: transcriptomic profile change of stress-related genes. Trop Anim Health Prod. (2020) 52:3685–96. doi: 10.1007/S11250-020-02405-4/METRICS
110. Hincke, MT, Da Silva, M, Guyot, N, Gautron, J, McKee, MD, Guabiraba-Brito, R, et al. Dynamics of structural barriers and innate immune components during incubation of the avian egg: critical interplay between autonomous embryonic development and maternal anticipation. J Innate Immun. (2019) 11:111–24. doi: 10.1159/000493719
111. Swiatkiewicz, S, and Arczewska-Wlosek, A. Prebiotic fructans and organic acids as feed additives improving mineral availability. Worlds Poult Sci J. (2012) 68:269–79. doi: 10.1017/S0043933912000323
112. Feng, A, Akter, S, Leigh, SA, Wang, H, Pharr, GT, Evans, J, et al. Genomic diversity, pathogenicity and antimicrobial resistance of Escherichia coli isolated from poultry in the southern United States. BMC Microbiol. (2023) 23:1–15. doi: 10.1186/S12866-022-02721-9/FIGURES/6
113. Xiao, S, Mi, J, Chen, Y, Feng, K, Mei, L, Liao, X, et al. The abundance and diversity of antibiotic resistance genes in layer chicken ceca is associated with farm enviroment. Front Microbiol. (2023) 14:1177404. doi: 10.3389/FMICB.2023.1177404/BIBTEX
114. Yang, QE, Sun, J, Li, L, Deng, H, Liu, BT, Fang, LX, et al. IncF plasmid diversity in multi-drug resistant Escherichia coli strains from animals in China. Front Microbiol. (2015) 6:964. doi: 10.3389/FMICB.2015.00964
115. Ricke, SC. Potential of fructooligosaccharide prebiotics in alternative and nonconventional poultry production systems. Poult Sci. (2015) 94:1411–8. doi: 10.3382/PS/PEV049
116. Cox, GW, Avery, BP, Parmley, EJ, Irwin, RJ, Reid-Smith, RJ, Deckert, AE, et al. A one health genomic investigation of gentamicin resistance in Escherichia coli from human and chicken sources in Canada, 2014 to 2017. Antimicrob Agents Chemother. (2022) 66:3. doi: 10.1128/AAC.00677-22/SUPPL_FILE/AAC.00677-22-S0003.XLSX
117. Agyare, C, Etsiapa Boamah, V, Ngofi Zumbi, C, and Boateng Osei, F. Antibiotic use in poultry production and its effects on bacterial resistance In: C Agyare, editor. Antimicrobial Resistance - A Global Threat. London: Intechopen (2019)
118. Poirel, L, Madec, J-Y, Lupo, A, Schink, A-K, Kieffer, N, Nordmann, P, et al. Antimicrobial resistance in Escherichia coli. Microbiol Spectr. (2018) 6:17. doi: 10.1128/MICROBIOLSPEC.ARBA-0026-2017
119. Gray, P, Jenner, R, Norris, J, and Page, S. Antimicrobial prescribing guidelines for poultry. Aust Vet J. (2021) 99:181–235. doi: 10.1111/avj.13034
120. Abraham, R, Allison, HS, Lee, T, Pavic, IA, Chia, R, Hewson, K, et al. A national study confirms that Escherichia coli from Australian commercial layer hens remain susceptible to critically important antimicrobials (2023) 18:e0281848. doi: 10.1371/journal.pone.0281848,
121. Redweik, GAJ, Jochum, J, and Mellata, M. Live bacterial prophylactics in modern poultry. Front Vet Sci. (2020) 7:592312. doi: 10.3389/FVETS.2020.592312/BIBTEX
122. Cavero, D, Schmutz, M, Philipp, HC, and Preisinger, R. Breeding to reduce susceptibility to Escherichia coli in layers. Poult Sci. (2009) 88:2063–8. doi: 10.3382/PS.2009-00168
123. Oh, JY, Kang, MS, Kim, JM, An, BK, Song, EA, Kim, JY, et al. Characterization of Escherichia coli isolates from laying hens with colibacillosis on 2 commercial egg-producing farms in Korea. Poult Sci. (2011) 90:1948–54. doi: 10.3382/PS.2011-01509
124. Uotani, Y, Kitahara, R, Imai, T, Tsutsumi, N, Sasakawa, C, Nagai, S, et al. Efficacy of an avian colibacillosis live vaccine for layer breeder in Japan. J Vet Med Sci. (2017) 79:1215. doi: 10.1292/JVMS.17-0189
125. Chaudhari, AA, Matsuda, K, and Lee, JH. Construction of an attenuated salmonella delivery system harboring genes encoding various virulence factors of avian pathogenic Escherichia coli and its potential as a candidate vaccine for chicken colibacillosis. Avian Dis. (2012) 57:88–96. doi: 10.1637/10277-061312-REG.1
126. Lee, JH, Chaudhari, AA, Oh, IG, Eo, SK, Park, SY, and Jawale, CV. Immune responses to oral vaccination with Salmonella-delivered avian pathogenic Escherichia coli antigens and protective efficacy against colibacillosis. Can J Vet Res. (2015) 79:229.
127. Schouler, C, Schaeffer, B, Brée, A, Mora, A, Dahbi, G, Biet, F, et al. Diagnostic strategy for identifying avian pathogenic Escherichia coli based on four patterns of virulence genes. J Clin Microbiol. (2012) 50:1673–8. doi: 10.1128/JCM.05057-11/SUPPL_FILE/JCM-JCM05057-11-S04.PDF
128. Van, GA, Stromberg, ZR, and Mellata, M. A recombinant multi-antigen vaccine with broad protection potential against avian pathogenic Escherichia coli. PLoS One. (2017) 12:e0183929. doi: 10.1371/JOURNAL.PONE.0183929
129. FAO. Probiotics in food Health and nutritional properties and guidelines for evaluation. FAO FOOD AND NUTRITION PAPER. (2001).
130. El, JR, Dittoe, DK, Olson, EG, Lourenco, J, Corcionivoschi, N, Ricke, SC, et al. Probiotics and potential applications for alternative poultry production systems. Poult Sci. (2021) 100:101156. doi: 10.1016/J.PSJ.2021.101156
131. Ivanov, D, Emonet, C, Foata, F, Affolter, M, Delley, M, Fisseha, M, et al. A serpin from the gut bacterium Bifidobacterium longum inhibits eukaryotic elastase-like serine proteases. J Biol Chem. (2006) 281:17246–52. doi: 10.1074/JBC.M601678200
132. Flint, HJ, Duncan, SH, Scott, KP, and Louis, P. Links between diet, gut microbiota composition and gut metabolism. Proc Nutr Soc. (2015) 74:13–22. doi: 10.1017/S0029665114001463
133. Khan, I, Bai, Y, Zha, L, Ullah, N, Ullah, H, Shah, SRH, et al. Mechanism of the gut microbiota colonization resistance and enteric pathogen infection. Front Cell Infect Microbiol. (2021) 11:6299. doi: 10.3389/FCIMB.2021.716299
134. Abdelqader, A, Irshaid, R, and Al-Fataftah, AR. Effects of dietary probiotic inclusion on performance, eggshell quality, cecal microflora composition, and tibia traits of laying hens in the late phase of production. Trop Anim Health Prod. (2013) 45:1017–24. doi: 10.1007/S11250-012-0326-7/METRICS
135. Lei, K, Li, YL, Yu, DY, Rajput, IR, and Li, WF. Influence of dietary inclusion of Bacillus licheniformis on laying performance, egg quality, antioxidant enzyme activities, and intestinal barrier function of laying hens. Poult Sci. (2013) 92:2389–95. doi: 10.3382/PS.2012-02686
136. Hatab, MH, Elsayed, MA, and Ibrahim, NS. Effect of some biological supplementation on productive performance, physiological and immunological response of layer chicks. J Radiat Res Appl Sci. (2016) 9:185–92. doi: 10.1016/J.JRRAS.2015.12.008
137. Lokapirnasari, WP, Pribadi, TB, Al, AA, Soeharsono, S, Hidanah, S, Harijani, N, et al. Potency of probiotics Bifidobacterium spp. and Lactobacillus casei to improve growth performance and business analysis in organic laying hens. Vet World. (2019) 12:860–7. doi: 10.14202/VETWORLD.2019.860-867
138. Forte, C, Acuti, G, Manuali, E, Casagrande Proietti, P, Pavone, S, Trabalza-Marinucci, M, et al. Effects of two different probiotics on microflora, morphology, and morphometry of gut in organic laying hens. Poult Sci. (2016) 95:2528–35. doi: 10.3382/PS/PEW164
139. Alagawany, M, Bilal, RM, Elnesr, SS, Elwan, HAM, Farag, MR, Dhama, K, et al. Yeast in layer diets: its effect on production, health, egg composition and economics. Worlds Poult Sci J. (2023) 79:135–53. doi: 10.1080/00439339.2023.2164235
140. Kiarie, EG, Cheng, V, Tan, Z, Chen, W, Xu, X, Peng, Y, et al. Comparative impact of bacitracin and select feed additives in the feeding program of Lohmann LSL-lite pullets at the onset of lay through to 31 weeks of age. Transl Anim Sci. (2024) 8:txae013. doi: 10.1093/tas/txae013
141. Ezema, C, Ihedioha, OC, Ihedioha, JI, Okorie-Kanu, CO, and Kamalu, TN. Probiotic effect of yeast (Saccharomyces cerevisiae) on haematological parameters and growth performance of pullets fed palm kernel cake-based diet. Comp Clin Path. (2012) 21:1145–8. doi: 10.1007/S00580-011-1250-3/METRICS
142. Hassanein, SM, and Soliman, NK. Effect of probiotic (Saccharomyces cerevisiae) adding to diets on intestinal microflora and performance of Hy-line layers hens. J Am Sci. (2010) 6, 30
143. Elghandour, MMY, Tan, ZL, Abu Hafsa, SH, Adegbeye, MJ, Greiner, R, Ugbogu, EA, et al. Saccharomyces cerevisiae as a probiotic feed additive to non-and pseudo-ruminant feeding: a review. J Appl Microbiol. (2020) 128:658–74. doi: 10.1111/JAM.14416
144. Liu, Y, Cheng, X, Zhen, W, Zeng, D, Qu, L, Wang, Z, et al. Yeast culture improves egg quality and reproductive performance of aged breeder layers by regulating gut microbes. Front Microbiol. (2021) 12:633276. doi: 10.3389/FMICB.2021.633276/BIBTEX
145. Latif, A, Shehzad, A, Niazi, S, Zahid, A, Ashraf, W, Iqbal, MW, et al. Probiotics: mechanism of action, health benefits and their application in food industries. Front Microbiol. (2023) 14:1216674. doi: 10.3389/FMICB.2023.1216674/BIBTEX
146. Monson, MS, Van Goor, AG, Persia, ME, Rothschild, MF, Schmidt, CJ, and Lamont, SJ. Genetic lines respond uniquely within the chicken thymic transcriptome to acute heat stress and low dose lipopolysaccharide. Sci Rep. (2019) 9:1–12. doi: 10.1038/s41598-019-50051-0
147. Yaqoob, MU, El-Hack, MEA, Hassan, F, El-Saadony, MT, Khafaga, AF, Batiha, GE, et al. The potential mechanistic insights and future implications for the effect of prebiotics on poultry performance, gut microbiome, and intestinal morphology. Poult Sci. (2021) 100:101143. doi: 10.1016/J.PSJ.2021.101143
148. Yitbarek, A, Echeverry, H, Munyaka, P, and Rodriguez-Lecompte, JC. Innate immune response of pullets fed diets supplemented with prebiotics and synbiotics. Poult Sci. (2015) 94:1802–11. doi: 10.3382/PS/PEV147
149. Karumuthil-Melethil, S, Gudi, R, Johnson, BM, Perez, N, and Vasu, C. Fungal β-Glucan, a Dectin-1 ligand, promotes protection from type 1 diabetes by inducing regulatory innate immune response. J Immunol. (2014) 193:3308–21. doi: 10.4049/JIMMUNOL.1400186
150. Al-Surrayai, T, and Al-Khalaifah, H. Dietary supplementation of Fructooligosaccharides enhanced antioxidant activity and cellular immune response in broiler chickens. Front Vet Sci. (2022) 9:857294. doi: 10.3389/FVETS.2022.857294/BIBTEX
151. Youssef, IM, Khalil, HA, Jaber, FA, Alhazzaa, RA, Alkholy, SO, Almehmadi, AM, et al. Influence of dietary mannan-oligosaccharides supplementation on hematological characteristics, blood biochemical parameters, immune response and histological state of laying hens. Poult Sci. (2023) 102:103071. doi: 10.1016/J.PSJ.2023.103071
152. Shang, HM, Hu, TM, Lu, YJ, and Wu, HX. Effects of inulin on performance, egg quality, gut microflora and serum and yolk cholesterol in laying hens. Br Poult Sci. (2010) 51:791–6. doi: 10.1080/00071668.2010.531005
153. Shang, HM, Zhou, HZ, Yang, JY, Li, R, Song, H, and Wu, HX. In vitro and in vivo antioxidant activities of inulin. PLoS One. (2018) 13:e0192273. doi: 10.1371/JOURNAL.PONE.0192273
154. Shang, H, Zhao, J, Dong, X, Guo, Y, Zhang, H, Cheng, J, et al. Inulin improves the egg production performance and affects the cecum microbiota of laying hens. Int J Biol Macromol. (2020) 155:1599–609. doi: 10.1016/J.IJBIOMAC.2019.11.137
155. Spring, P, Wenk, C, Dawson, KA, and Newman, KE. The effects of dietary mannaoligosaccharides on cecal parameters and the concentrations of enteric bacteria in the ceca of Salmonella-challenged broiler chicks. Poult Sci. (2000) 79:205–11. doi: 10.1093/PS/79.2.205
156. Rhoades, J, Manderson, K, Wells, A, Hotchkiss, AT, Gibson, GR, Formentin, K, et al. Oligosaccharide-mediated inhibition of the adhesion of pathogenic Escherichia coli strains to human gut epithelial cells in vitro. J Food Prot. (2008) 71:2272–7. doi: 10.4315/0362-028X-71.11.2272
157. Jahanian, R, and Ashnagar, M. Effect of dietary supplementation of mannan-oligosaccharides on performance, blood metabolites, ileal nutrient digestibility, and gut microflora in Escherichia coli-challenged laying hens. Poult Sci. (2015) 94:2165–72. doi: 10.3382/PS/PEV180
158. Kiarie, E, Romero, LF, and Nyachoti, CM. The role of added feed enzymes in promoting gut health in swine and poultry. Nutr Res Rev. 26:71–88. doi: 10.1017/S0954422413000048
159. Kiarie, EG, Leung, H, Akbari Moghaddam Kakhki, R, Patterson, R, and Barta, JR. Utility of feed enzymes and yeast derivatives in ameliorating deleterious effects of coccidiosis on intestinal health and function in broiler chickens. Front Vet Sci. (2019) 6:473. doi: 10.3389/FVETS.2019.00473
160. Kiarie, EG, Steelman, S, and Martinez, M. Does supplementing β-mannanase modulate the feed-induced immune response and gastrointestinal ecology in poultry and pigs? An appraisal. Front Anim Sci. (2022) 3:875095. doi: 10.3389/FANIM.2022.875095/BIBTEX
161. Quigley, EMM. Prebiotics and probiotics in digestive health. Clin Gastroenterol Hepatol. (2019) 17:333–44. doi: 10.1016/J.CGH.2018.09.028
162. Sikorska, M, Siwek, M, Slawinska, A, and Dunislawska, A. miRNA profiling in the chicken liver under the influence of early microbiota stimulation with probiotic, prebiotic, and Synbiotic. Genes. (2021) 12:685. doi: 10.3390/GENES12050685
163. Shang, Y, Regassa, A, Kim, JH, and Kim, WK. The effect of dietary fructooligosaccharide supplementation on growth performance, intestinal morphology, and immune responses in broiler chickens challenged with Salmonella Enteritidis lipopolysaccharides. Poult Sci. (2015) 94:2887–97. doi: 10.3382/PS/PEV275
164. Dunislawska, A, Slawinska, A, Stadnicka, K, Bednarczyk, M, Gulewicz, P, Jozefiak, D, et al. Synbiotics for broiler chickens—in vitro design and evaluation of the influence on host and selected microbiota populations following in Ovo delivery. PLoS One. (2017) 12:e0168587. doi: 10.1371/JOURNAL.PONE.0168587
165. Samanta, AK, Jayapal, N, Senani, S, Kolte, AP, and Sridhar, M. Prebiotic inulin: useful dietary adjuncts to manipulate the livestock gut microflora. Braz J Microbiol. (2013) 44:1. doi: 10.1590/S1517-83822013005000023
166. Śliżewska, K, Markowiak-Kopeć, P, Żbikowski, A, and Szeleszczuk, P. The effect of synbiotic preparations on the intestinal microbiota and her metabolism in broiler chickens. Sci Rep. (2020) 10:1–13. doi: 10.1038/s41598-020-61256-z
167. Abdel-Wareth, AAA. Effect of dietary supplementation of thymol, synbiotic and their combination on performance, egg quality and serum metabolic profile of Hy-line Brown hens. Br Poult Sci. (2016) 57:114–22. doi: 10.1080/00071668.2015.1123219
168. Das, Q, Islam, MR, Lepp, D, Tang, J, Yin, X, Mats, L, et al. Gut microbiota, blood metabolites, and spleen immunity in broiler chickens fed berry pomaces and phenolic-enriched extractives. Front Vet Sci. (2020) 7:526484. doi: 10.3389/FVETS.2020.00150/BIBTEX
169. Kikusato, M. Phytobiotics to improve health and production of broiler chickens: functions beyond the antioxidant activity. Anim Biosci. (2021) 34:345–53. doi: 10.5713/AB.20.0842
170. Das, Q, Tang, J, Yin, X, Ross, K, Warriner, K, Marcone, MF, et al. Organic cranberry pomace and its ethanolic extractives as feed supplement in broiler: impacts on serum Ig titers, liver and bursal immunity. Poult Sci. (2021) 100:517–26. doi: 10.1016/J.PSJ.2020.09.044
171. Nagata, K, and Nishiyama, C. IL-10 in mast cell-mediated immune responses: anti-inflammatory and proinflammatory roles. Int J Mol Sci. (2021) 22:972. doi: 10.3390/IJMS22094972
172. Wang, Y, Wang, Y, Su, C, Wang, L, Lv, X, Cui, G, et al. Dietary cinnamaldehyde with carvacrol or thymol improves the egg quality and intestinal health independent of gut microbiota in post-peak laying hens. Front Vet Sci. (2022) 9:994089. doi: 10.3389/FVETS.2022.994089/BIBTEX
173. Brenes, A, and Roura, E. Essential oils in poultry nutrition: Main effects and modes of action. Anim Feed Sci Technol. (2010) 158:1–14. doi: 10.1016/J.ANIFEEDSCI.2010.03.007
174. Gopi, M, Karthik, K, Manjunathachar, HV, Tamilmahan, P, Kesavan, M, Dashprakash, M, et al. A feed additive in poultry nutrition ARTICLE HISTORY ABSTRACT. Adv Anim Vet Sci. (2014) 2:17. doi: 10.14737/journal.aavs/2014.2.1.1.7
175. Ding, X, Yu, Y, Su, Z, and Zhang, K. Effects of essential oils on performance, egg quality, nutrient digestibility and yolk fatty acid profile in laying hens. Anim Nutr. (2017) 3:127–31. doi: 10.1016/J.ANINU.2017.03.005
176. Burt, S. Essential oils: their antibacterial properties and potential applications in foods—a review. Int J Food Microbiol. (2004) 94:223–53. doi: 10.1016/J.IJFOODMICRO.2004.03.022
177. Jamroz, D, Wertelecki, T, Houszka, M, and Kamel, C. Influence of diet type on the inclusion of plant origin active substances on morphological and histochemical characteristics of the stomach and jejunum walls in chicken. J Anim Physiol Anim Nutr (Berl). (2006) 90:255–68. doi: 10.1111/J.1439-0396.2005.00603.X
178. Yang, C, Rehman, MA, Yin, X, Carrillo, CD, Wang, Q, Yang, C, et al. Antimicrobial resistance phenotypes and genotypes of Escherichia coli isolates from broiler chickens fed encapsulated Cinnamaldehyde and Citral. J Food Prot. (2021) 84:1385–99. doi: 10.4315/JFP-21-033
179. Tvrzicka, E, Kremmyda, LS, Stankova, B, and Zak, A. Fatty acids as biocompounds: their role in human metabolism, health and disease--a review. Part 1: classification, dietary sources and biological functions. Biomed Pap Med Fac Univ Palacky Olomouc Czech Repub. (2011) 155:117–30. doi: 10.5507/BP.2011.038
180. Guo, Y, Chen, S, Xia, Z, and Yuan, J. Effects of different types of polyunsaturated fatty acids on immune function and PGE2 synthesis by peripheral blood leukocytes of laying hens. Anim Feed Sci Technol. (2004) 116:249–58. doi: 10.1016/J.ANIFEEDSCI.2004.07.011
181. Akbari, R, Kakhki, M, Ma, DWL, Price, KR, Moats, J, Karrow, NA, et al. Impact of feeding n-3 fatty acids to layer breeders and their offspring on concentration of antibody titres against infectious bronchitis, and Newcastle diseases and plasma fatty acids in the offspring. Br Poult Sci. (2021) 62:270–7. doi: 10.1080/00071668.2020.1847254
182. Thanabalan, A, and Kiarie, EG. Influence of feeding Omega-3 polyunsaturated fatty acids to broiler breeders on indices of Immunocompetence, gastrointestinal, and skeletal development in broiler chickens. Front Vet Sci. (2021) 8:653152. doi: 10.3389/FVETS.2021.653152/BIBTEX
183. Neijat, M, Habtewold, J, Li, S, Jing, M, and House, JD. Effect of dietary n-3 polyunsaturated fatty acids on the composition of cecal microbiome of Lohmann hens. Prostaglandins Leukot Essent Fatty Acids. (2020) 162:102182. doi: 10.1016/J.PLEFA.2020.102182
184. Maina, AN, Lewis, E, and Kiarie, EG. Egg production, egg quality, and fatty acids profiles in eggs and tissues in Lohmann LSL lite hens fed algal oils rich in docosahexaenoic acid (DHA). Poult Sci. (2023) 102:102921. doi: 10.1016/J.PSJ.2023.102921
185. Li, S, Jing, M, Mohamed, N, Rey-Dubois, C, Zhao, S, Aukema, HM, et al. The effect of increasing concentrations of Omega-3 fatty acids from either flaxseed oil or preformed docosahexaenoic acid on fatty acid composition, plasma Oxylipin, and immune response of laying hens. J Nutr. (2023) 153:2105–16. doi: 10.1016/J.TJNUT.2023.05.017
186. Wang, YW, Field, CJ, and Sim, JS. Dietary polyunsaturated fatty acids alter lymphocyte subset proportion and proliferation, serum immunoglobulin G concentration, and immune tissue development in chicks. Poult Sci. (2000) 79:1741–8. doi: 10.1093/PS/79.12.1741
187. Parker, EM, Parker, AJ, Short, G, O’Connor, AM, and Wittum, TE. Salmonella detection in commercially prepared livestock feed and the raw ingredients and equipment used to manufacture the feed: a systematic review and meta-analysis. Prev Vet Med. (2022) 198:105546. doi: 10.1016/J.PREVETMED.2021.105546
188. Christensen, H, Bachmeier, J, and Bisgaard, M. New strategies to prevent and control avian pathogenic Escherichia coli (APEC). Avian Pathol. (2021) 50:370–81. doi: 10.1080/03079457.2020.1845300
189. Zumbrun, SD, Melton-Celsa, AR, Smith, MA, Gilbreath, JJ, Merrell, DS, and O’Brien, AD. Dietary choice affects Shiga toxin-producing Escherichia coli (STEC) O157:H7 colonization and disease. Proc Natl Acad Sci U S A. (2013) 110:E2126–33. doi: 10.1073/PNAS.1222014110/SUPPL_FILE/SD01.XLSX
190. Sethiya, NK. Review on natural growth promoters available for improving gut health of poultry: An alternative to antibiotic growth promoters. Asian J Poult Sci. (2016) 10:1–29. doi: 10.3923/AJPSAJ.2016.1.29
191. Rychen, G, Aquilina, G, Azimonti, G, Bampidis, V, de Bastos, ML, Bories, G, et al. Guidance on the characterization of microorganisms used as feed additives or as production organisms. EFSA J. (2018) 16:e05206. doi: 10.2903/J.EFSA.2018.5206
192. Nilsson, O, Börjesson, S, Landén, A, and Bengtsson, B. Vertical transmission of Escherichia coli carrying plasmid-mediated AmpC (pAmpC) through the broiler production pyramid. J Antimicrob Chemother. (2014) 69:1497–500. doi: 10.1093/JAC/DKU030
193. Aleksandrowicz, A, Khan, MM, Sidorczuk, K, Noszka, M, and Kolenda, R. Whatever makes them stick – Adhesins of avian pathogenic Escherichia coli. Vet Microbiol. (2021) 257:109095. doi: 10.1016/J.VETMIC.2021.109095
194. Wang, S, Shi, Z, Xia, Y, Li, H, Kou, Y, Bao, Y, et al. IbeB is involved in the invasion and pathogenicity of avian pathogenic Escherichia coli. Vet Microbiol. (2012) 159:411–9. doi: 10.1016/J.VETMIC.2012.04.015
195. Wang, S, Bao, Y, Meng, Q, Xia, Y, Zhao, Y, Wang, Y, et al. IbeR facilitates stress-resistance, invasion and pathogenicity of avian pathogenic Escherichia coli. PLoS One. (2015) 10:e0119698. doi: 10.1371/JOURNAL.PONE.0119698
196. Pilatti, L, De Paiva, JB, Rojas, TCG, Leite, JL, Conceição, RA, Nakazato, G, et al. The virulence factor ychO has a pleiotropic action in an avian pathogenic Escherichia coli (APEC) strain. BMC Microbiol. (2016) 16:1–11. doi: 10.1186/S12866-016-0654-2/TABLES/2
197. Wallace, AJ, Stillman, TJ, Atkins, A, Jamieson, SJ, Bullough, PA, Green, J, et al. E. coli Hemolysin E (HlyE, ClyA, SheA): X-Ray Crystal Structure of the Toxin and observation of membrane pores by electron microscopy. Cell. 100:265–76. doi: 10.1016/s0092-8674(00)81564-0
198. Murase, K, Martin, P, Porcheron, G, Houle, S, Helloin, E, Pénary, M, et al. HlyF produced by Extraintestinal pathogenic Escherichia coli is a virulence factor that regulates outer membrane vesicle biogenesis. J Infect Dis. (2016) 213:856–65. doi: 10.1093/INFDIS/JIV506
199. Díaz, JM, Dozois, CM, Avelar-González, FJ, Hernández-Cuellar, E, Pokharel, P, de Santiago, AS, et al. The vacuolating autotransporter toxin (vat) of Escherichia coli causes cell cytoskeleton changes and produces non-lysosomal vacuole formation in bladder epithelial cells. Front Cell Infect Microbiol. (2020) 10:511051. doi: 10.3389/FCIMB.2020.00299/BIBTEX
200. Pons, BJ, Vignard, J, and Mirey, G. Cytolethal distending toxin subunit B: a review of structure–function relationship. Toxins. (2019) 11:595. doi: 10.3390/TOXINS11100595
201. Wilczyński, J, Stępień-Pyśniak, D, Wystalska, D, and Wernicki, A. Molecular and serological characteristics of avian pathogenic Escherichia coli isolated from various clinical cases of poultry Colibacillosis in Poland. Animals. (2022) 12:1090. doi: 10.3390/ANI12091090/S1
202. Gill, A, Dussault, F, McMahon, T, Petronella, N, Wang, X, Cebelinski, E, et al. Characterization of atypical Shiga toxin gene sequences and description of Stx2j, a new subtype. J Clin Microbiol. (2022) 60:e0222921. doi: 10.1128/jcm.02229-21
203. Montenegro, MA, Bitter-Suermann, D, Timmis, JK, Agüero, ME, Cabello, FC, and Sanyal, SC. traT gene sequences, serum resistance and pathogenicity-related factors in clinical isolates of Escherichia coli and other gram-negative bacteria. J Gen Microbiol. (1985) 131:1511–21. doi: 10.1099/00221287-131-6-1511/CITE/REFWORKS
204. Silveira, F, Maluta, RP, Tiba, MR, de Paiva, JB, Guastalli, EAL, and da Silveira, WD. Comparison between avian pathogenic (APEC) and avian faecal (AFEC) Escherichia coli isolated from different regions in Brazil. Vet J. (2016) 217:65–7. doi: 10.1016/J.TVJL.2016.06.007
205. Rodriguez-Siek, KE, Giddings, CW, Doetkott, C, Johnson, TJ, and Nolan, LK. Characterizing the APEC pathotype. Vet Res. (2005) 36:241–56. doi: 10.1051/vetres:2004057
206. Han, Y, Han, X, Wang, S, Meng, Q, Zhang, Y, Ding, C, et al. The waaL gene is involved in lipopolysaccharide synthesis and plays a role on the bacterial pathogenesis of avian pathogenic Escherichia coli. Vet Microbiol. (2014) 172:486–91. doi: 10.1016/j.vetmic.2014.05.029
207. Zuo, J, Tu, C, Wang, Y, Qi, K, Hu, J, Wang, Z, et al. The role of the wzy gene in lipopolysaccharide biosynthesis and pathogenesis of avian pathogenic Escherichia coli. Microb Pathog. (2019) 127:296–303. doi: 10.1016/J.MICPATH.2018.12.021
208. Johnson, TJ, Johnson, SJ, and Nolan, LK. Complete DNA sequence of a ColBM plasmid from avian pathogenic Escherichia coli suggests that it evolved from closely related ColV virulence plasmids. J Bacteriol. (2006) 188:5975–83. doi: 10.1128/JB.00204-06/SUPPL_FILE/JOHNSON_ET_AL_SUPP_TABLE.ZIP
209. Ling, J, Pan, H, Gao, Q, Xiong, L, Zhou, Y, Zhang, D, et al. Aerobactin synthesis genes iucA and iucC contribute to the pathogenicity of avian pathogenic Escherichia coli O2 strain E058. PLoS One. (2013) 8:e57794. doi: 10.1371/JOURNAL.PONE.0057794
210. Ovi, F, Zhang, L, Nabors, H, Jia, L, and Adhikari, P. A compilation of virulence-associated genes that are frequently reported in avian pathogenic Escherichia coli (APEC) compared to other E. coli. J Appl Microbiol. (2023) 134:14. doi: 10.1093/JAMBIO/LXAD014
211. Datsenko, KA, and Wanner, BL. One-step inactivation of chromosomal genes in Escherichia coli K-12 using PCR products. Proc Natl Acad Sci USA. (2000) 97:6640–5. doi: 10.1073/PNAS.120163297/ASSET/103C1B8D-D302-4337-8E67-9F9084156407/ASSETS/GRAPHIC/PQ1201632006.JPEG
212. De Pace, F, Nakazato, G, Pacheco, A, De Paiva, JB, Sperandio, V, and Da Silveira, WD. The type VI secretion system plays a role in type 1 Fimbria expression and pathogenesis of an avian pathogenic Escherichia coli strain. Infect Immun. (2010) 78:4990. doi: 10.1128/IAI.00531-10
213. Fu, D, Shao, Y, Li, J, Wu, J, Wu, X, Song, X, et al. LuxR family transcriptional repressor YjjQ modulates the biofilm formation and motility of avian pathogenic Escherichia coli. Res Vet Sci. (2022) 152:10–9. doi: 10.1016/J.RVSC.2022.07.011
214. Wickramasuriya, SS, Park, I, Lee, K, Lee, Y, Kim, WH, Nam, H, et al. Role of physiology, immunity, microbiota, and infectious diseases in the gut health of poultry. Vaccine. (2022) 10:172. doi: 10.3390/VACCINES10020172
215. Larsonneur, F, Martin, FA, Mallet, A, Martinez-Gil, M, Semetey, V, Ghigo, JM, et al. Functional analysis of Escherichia coli Yad fimbriae reveals their potential role in environmental persistence. Environ Microbiol. (2016) 18:5228–48. doi: 10.1111/1462-2920.13559
216. Freire, CA, Silva, RM, Ruiz, RC, Pimenta, DC, Bryant, JA, Henderson, IR, et al. Secreted autotransporter toxin (sat) mediates innate immune system evasion. Front Immunol. (2022) 13:844878. doi: 10.3389/FIMMU.2022.844878/BIBTEX
217. Pokharel, P, Habouria, H, Bessaiah, H, and Dozois, CM. Serine protease autotransporters of the Enterobacteriaceae (SPATEs): out and about and chopping it up. Microorganisms. (2019) 7:594. doi: 10.3390/MICROORGANISMS7120594
218. Navarro-García, F, Canizalez-Roman, A, Sui, BQ, Nataro, JP, and Azamar, Y. The serine protease motif of EspC from enteropathogenic Escherichia coli produces epithelial damage by a mechanism different from that of pet toxin from enteroaggregative E. coli. Infect Immun. (2004) 72:3609–21. doi: 10.1128/IAI.72.6.3609-3621.2004/ASSET/DC0FD42B-B599-432A-B462-1B936450E54F/ASSETS/GRAPHIC/ZII0060439570009.JPEG
219. Sozcu, A, and Ipek, A. The effects of lignocellulose supplementation on laying performance, egg quality parameters, aerobic bacterial load of eggshell, serum biochemical parameters, and jejunal histomorphological traits of laying hens. Poult Sci. (2020) 99:3179–87. doi: 10.1016/J.PSJ.2020.01.024
220. Ghasemian, M, and Jahanian, R. Dietary mannan-oligosaccharides supplementation could affect performance, immunocompetence, serum lipid metabolites, intestinal bacterial populations, and ileal nutrient digestibility in aged laying hens. Anim Feed Sci Technol. (2016) 213:81–9. doi: 10.1016/J.ANIFEEDSCI.2015.12.012
221. Bassolé, IHN, and Juliani, HR. Essential oils in combination and their antimicrobial properties. Molecules. (2012) 17:3989–4006. doi: 10.3390/MOLECULES17043989
222. De Reu, K, Grijspeerdt, K, Heyndrickx, M, Zoons, J, De Baere, K, Uyttendaele, M, et al. Bacterial eggshell contamination in conventional cages, furnished cages and aviary housing systems for laying hens. Br Poult Sci. (2005) 46:149–55. doi: 10.1080/00071660500065359
223. Singh, R, Cheng, KM, and Silversides, FG. Production performance and egg quality of four strains of laying hens kept in conventional cages and floor pens. Poult Sci. (2009) 88:256–64. doi: 10.3382/PS.2008-00237
224. Sharma, MK, McDaniel, CD, Kiess, AS, Loar, RE, and Adhikari, P. Effect of housing environment and hen strain on egg production and egg quality as well as cloacal and eggshell microbiology in laying hens. Poult Sci. (2022) 101:101595. doi: 10.1016/J.PSJ.2021.101595
225. Vlčková, J, Tůmová, E, Míková, K, Englmaierová, M, Okrouhlá, M, and Chodová, D. Changes in the quality of eggs during storage depending on the housing system and the age of hens. Poult Sci. (2019) 98:6187–93. doi: 10.3382/PS/PEZ401
226. Samiullah, RJR, and Chousalkar, KK. Effect of production system and flock age on egg quality and total bacterial load in commercial laying hens. J Appl Poult Res. (2014) 23:59–70. doi: 10.3382/JAPR.2013-00805
227. Philippe, FX, Mahmoudi, Y, Cinq-Mars, D, Lefrançois, M, Moula, N, Palacios, J, et al. Comparison of egg production, quality and composition in three production systems for laying hens. Livest Sci. (2020) 232:103917. doi: 10.1016/J.LIVSCI.2020.103917
Keywords: avian pathogenic Escherichia coli, colibacillosis, antibiotics, feed additives, pullets, layers
Citation: Waliaula PK, Kiarie EG and Diarra MS (2024) Predisposition factors and control strategies of avian pathogenic Escherichia coli in laying hens. Front. Vet. Sci. 11:1474549. doi: 10.3389/fvets.2024.1474549
Edited by:
Roswitha Merle, Free University of Berlin, GermanyReviewed by:
Christi L. Swaggerty, Agricultural Research Service (USDA), United StatesJorge Rivera-Gomis, Scotland’s Rural College (SRUC), United Kingdom
Copyright © 2024 Elijah G. Kiarie and, His Majesty the King in Right of Canada, as represented by the Minister of Agriculture and Agri-Food Canada for the contribution of Paul K. Waliaula and Moussa S. Diarra. This is an open-access article distributed under the terms of the Creative Commons Attribution License (CC BY). The use, distribution or reproduction in other forums is permitted, provided the original author(s) and the copyright owner(s) are credited and that the original publication in this journal is cited, in accordance with accepted academic practice. No use, distribution or reproduction is permitted which does not comply with these terms.
*Correspondence: Moussa S. Diarra, bW91c3NhLmRpYXJyYUBhZ3IuZ2MuY2E=