- 1Department of Eco-friendly Livestock Science, Institute of Green Bio Science and Technology, Seoul National University, Pyeongchang, Republic of Korea
- 2Graduate School of International Agricultural Technology, Seoul National University, Pyeongchang, Republic of Korea
- 3Department of Geography, McGill University, Montreal, QC, Canada
- 4University of Maryland Center for Environmental Science, Frostburg, MD, United States
- 5National Institute of Animal Sciences, Rural Development Administration, Cheonan, Republic of Korea
- 6National Institute of Animal Sciences, Rural Development Administration, Hamyang, Republic of Korea
- 7Agroécologie, INRAE, Institut Agro, Université de Bourgogne, Université Bourgogne Franche-Comté, Dijon, France
Two in vivo experiments were conducted to evaluate the potential of Pharbitis nil seeds (PA) as an anti-methanogenic additive to ruminant feed. In experiment 1, six Hanwoo steers (459.0 ± 25.8 kg) were fed either a total mixed ration (TMR; 32-d period) or TMR supplemented with PA at 5% dry matter (DM) intake (TMR-PA; 45-d period) for two consecutive periods. Fecal and urine outputs were measured in an apparent digestibility trial in both periods. Methane (CH4) yield and heat energy (HE) were measured using respiratory chambers equipped with gas analyzers. In experiment 2, five rumen cannulated Holstein steers (744 ± 35 kg) were fed the same TMR or TMR-PA diets for 40 days; rumen samples were collected at 0, 1.5, and 3 h after feeding on the last day of the feeding period. In experiment 1, although there were no differences (p > 0.05) in nutrients or gross energy intake (GEI) between the groups, an increase (p < 0.05) in the apparent digestibility of DM (9.1%) and neutral detergent fiber (22.9%) was observed in the TMR-PA fed Hanwoo steers. Pronounced decreases (p < 0.05) in CH4 (g/Kg DM; 17.1%) and urinary N excretion (% N intake; 7.6%) were observed in the TMR-PA group, leading to a 14.7% increase in metabolizable energy intake (% GEI). However, only a numerical increase (p > 0.05) in retained energy was observed due to the increase in HE loss. In experiment 2, a drastic decrease (p < 0.05) in rumen ammonia concentration (56.3%) associated with an increased (p = 0.091) rumen short-chain fatty acid concentration 1.5 h after feeding were observed in TMR-PA fed Holstein steers. A 26.6% increase (p < 0.05) in the propionate proportion during the treatment period clearly reflected a shift in the ruminal H2 sink after 3 h of feeding. A 40% reduction (p = 0.067) in the relative abundance of rumen protozoa Entodinium caudatum was also observed. It was concluded that PA could be a natural feed additive for CH4 and N emission abatement.
1 Introduction
In addition to their environmental footprints, methane (CH4) and nitrogen (N) emissions represent losses in livestock dietary energy and N utilization (1, 2). The total farm-gate greenhouse gas emissions from Korea were 19.3 MtCO2 eq/year in 2021; CH4 and nitrous oxide (N2O) from livestock farming (enteric fermentation and manure management) contributed 6.59 and 1.05 MtCO2 eq/year, respectively (3). The recent update of the nationally determined contribution of Korea includes a target to reduce the total absolute greenhouse gas emissions by 24.4% from their 2017 level (709.1 MtCO2eq; i.e. reducing national greenhouse gas emissions to 536 MtCO2eq) by the year 2030 (4). Furthermore, Korea has pledged to become carbon neutral by 2050 with a focus on low-carbon agricultural technologies targeting the development of low-methane feeds that improve livestock enteric fermentation and reduce CH4 emissions (5). However, the substantial increase in the total cattle population, with a record of 3.35 million heads in the first quarter of 2024 (6), has raised concerns regarding their associated CH4 and N2O emissions.
Several dietary interventions have been proposed to mitigate the emissions of CH4 and noxious N-containing compounds from ruminant husbandry (7, 8). The implementation of these interventions while avoiding changes to nutrient and energy utilization is a major goal in animal nutrition. Large-scale identification of plant materials rich in plant secondary metabolites, such as tannins and saponins, for CH4 and N abatement in the livestock industry has been achieved in recent years (9, 10). Plant materials rich in bioactive lipid compounds and other unsaturated fatty acids (FAs) have been extensively studied to elucidate these effects (7, 8). However, most relevant results have been obtained by in vitro trials; only few plant materials such as hazel leaves (11, 12), tannin rich legumes (13, 14), and conventional or unconventional oil seeds (15–17) have been studied in vivo to determine their nutritional value and effects on CH4 production and N utilization. Moreover, inconsistent results (18, 19) and adverse effects on nutrient digestibility (20) have encouraged ruminant nutritionists to explore novel feed additives with high nutritional value.
Pharbitis nil (Convolvulaceae) is an annual climbing herb widely distributed throughout Korea, Japan, and China, and are reported to be enriched in resin glycosides (21), chlorogenic acid derivatives (22), and other phenolic substances (23). Seeds of Pharbitis nil (PA) were also used as a purgative agent and in treating digestive disorders (24). Our previous in vitro and in sacco studies identified and evaluated the potential of PA for mitigating ruminal CH4 and NH3 production with improved nutrient digestibility at a range of 4.5%−9% dry matter (DM) supplementation (10, 25). We also found that PA is rich in nutrients such as neutral detergent fiber (NDF), crude protein (CP), and fat (25). Our previous studies demonstrated that the abundance of flavonoids and unsaturated FAs in PA probably had detrimental effects on ciliate protozoa (25), which are generally regarded as H2 producers and are detrimental for ruminal N metabolism (8, 26). Therefore, the inclusion of PA in ruminant diets would be an effective strategy to prevent inefficient N utilization and CH4 emission. However, in vivo experiments may be more appropriate for evaluating the nutritive value of PA because a drastic change in the Bacteroides: Firmicutes ratio was observed after PA incubation in rumen due to a probable defaunation effect (25).
Therefore, the present study investigated the effects of supplementing PA in the diet of Hanwoo steers at 5% DM intake on digestibility, CH4 emissions, energy and N balance, and rumen protozoal diversity via reverse transcription quantitative polymerase chain reaction (RT-qPCR) analysis. We hypothesized that PA supplementation to ruminants would enhance nutrient digestibility and decrease protozoal abundance, followed by reductions in N and CH4 emissions, thereby increasing dietary metabolizability and allowing spare energy to be used for productivity gains.
2 Materials and methods
2.1 Animal ethics statement
All experimental animals were obtained from the animal farm of Seoul National University. The methods and protocols for all experiments involving animals were approved by the Committee for the Institutional Animal Care and Use of Seoul National University (SNU-210615-3), and all experiments were performed in accordance with relevant guidelines and regulations.
2.2 Experiment 1: effects of PA on CH4 production, energy balance, and productivity of Hanwoo steers
In experiment 1, six Hanwoo steers with an initial body weight (BW) of 459.0 ± 25.8 kg were randomly allocated to pens equipped with automatic Calan doors. All steers were offered a commercial total mixed ration (TMR; Table 1) as a basal diet for 21 days and allowed to adapt to the Calan doors to consume their individual feeds prior to commencement of experimental work. The experiment included two successive periods where the animals were first fed a basal diet for 32 days (TMR), followed by 45 days of PA supplementation at 5% DM (TMR-PA) of the basal diet. The TMR period included 21 days for diet adaptation, 3 days for respiratory chamber adaptation, 3 days for gas sampling, and 4 days for total feces and urine collection. The TMR-PA period included 35 days for diet adaptation, 3 days for respiratory chamber adaptation, 3 days for gas sampling, and 4 days for total feces and urine collection. Because only three steers could be housed in the respiratory chambers at a given time, steers began the experiment in groups of three, staggered by 1 week. The TMR diet was prepared every 14 days during the experimental period by a local feed mill. The prepared TMR diet was then packed in vinyl bags (20 kg per bag) and stored for feeding to the animals. The diet was provided at 2% initial BW twice daily (at 09:00 and 18:00), and the animals had unrestricted access to water. PA was purchased from the local market in Dongdaemun-gu, Seoul, Republic of Korea, ground to pass through a 1-mm screen (Model 4, Thomas Scientific, Chadds Ford Township, PA, USA), and mixed with the diet during feeding. The BWs of the animals were measured both at the beginning and end of the period to calculate the average daily gain (ADG). Feed refusals were recorded both in the pen and respiratory chambers to determine the DM intake and feed conversion ratio. Samples of feed and PA were collected at the beginning of each period, whereas samples of refusals were collected each day when the animals were in respiratory chambers. All samples were stored at −20°C until further analysis.
2.2.1 Respiratory chamber description and gas sampling for methane and heat production measurements
Gas sampling was performed for three consecutive days, beginning from days 27 and 40 during the TMR and TMR-PA periods, respectively. Animals were placed in the chamber; the CH4, CO2, and oxygen (O2) concentrations were measured using three indirect open-circuit whole-body respiratory chambers composed of steel frames and covered by detachable, clear polyvinyl chloride (PVC) sheets to enable visual communication between animals. The design and operating procedures of the respiratory chambers were developed based on those previously reported in Cesar and Garry (27). The outer dimensions of each chamber were 137 (width) × 356 (depth) × 200 (height) cm, resulting in a chamber volume of 9.7 m3. Each chamber was equipped with a feeder and water bowl; the chamber floor was layered with a rubber mat containing a metal grated opening (80 × 80 cm) in the middle. Each chamber was fixed to the floor; the gap between the floor and the bottom of the chamber (36 cm) was sealed by covering the middle opening with a movable steel container for urine collection. All chambers were designed to maintain ambient temperature and relative humidity at 21°C and 60%, respectively, using an independent regulation system that contained an air refrigeration unit (model DK-C-150E; Dryer Korea) with a recirculating fan (model ALFFIZ-WBCAI-015H; Busung, Korea) and an air filter section. This unit was integrated into the chamber through 10-cm-diameter flexible thermo-insulating tubes from the ceiling. For recirculation, air left the chamber (0.6 m/s) through openings in the middle of the chamber ceiling; the dehumidified air was then recycled into the chamber (0.1 m/s) through openings at the back end of the chamber ceiling. The speed of air circulation was fixed to avoid loss of gases and maximize their recovery. Condensed water from the refrigeration unit was drained into the chamber through a capillary tube attached to the water traps.
Streams of ambient air from the barn were drawn through a 10-cm-diameter opening in the back door of each chamber by a sealed rotary pump connected to a flow meter (model LS-3D; Teledyne Technologies, Thousand Oaks, CA, USA) that provided a constant wet ventilation rate (wet VR) of 450 L/min. Each chamber was fitted with an air outlet containing a filter box in the front section of the ceiling, and the air was continuously drawn out next to the control room through a 10-cm-diameter polyvinyl chloride (PVC) pipe. Air outflow from each chamber was sampled from the middle of a PVC pipe between the chamber and the exhaust pump, through a gas tube connected to the analysis system.
All three chambers shared a common gas analysis system that consisted of a multiplexer (Metabolic Controller, B.S. Technolab, Seoul, Korea) connected to a gas sampling pump (Columbus Instruments, Columbus, OH, USA) and a gas analyzer (model VA-3000, Horiba Ltd., Tokyo, Japan) containing non-dispersive infrared CH4 (0–2,000 ppm) and CO2 (0–10,000 ppm) gas sensors, as well as a paramagnetic O2 (0–25%) sensor. The multiplexer had a gas switching system with eight measuring channels that controlled the three chambers and background air samples; it delivered only one sample stream (from one of the chambers or background) to the analyzer set through a sample flow that was set to 0.6 L/min by the pump. The sample used to determine background gas concentrations was acquired near the inlet of each chamber. Before each air sample entered the analysis system, it was filtered and dried in cylindrical plastic columns filled with desiccants and an indicator (Mesh size 8, Drierite, Xenia, OH, USA). The sample stream was then sequentially delivered to the CH4, CO2, and O2 analyzers. Calibrations of gas sensors (zero and span) were performed before the beginning of each measurement using standard gas mixtures with concentrations of 807 μmol/mol CH4, 7,996 μmol/mol CO2, and 19.99 %mol/mol O2 in N (Air Korea, Seoul, Korea).
Data acquisition and analysis software (Metabolic System, SciTech, Seoul, Korea) was used to record the CH4, CO2, and O2 concentrations, as well as the wet VR, during the measurement period. The time interval of multiplexer channel switching was also defined by the operator in the data acquisition software. Based on the stabilities of gas concentrations and numbers of operating chambers, the air was sampled every 10.5 min for each chamber, with background air and chamber air sampling sequences of 90 and 120 s, respectively. The average value of the final 20 s of sampling time was considered for calculations.
Before animal placement in respiratory chambers, the recovery rate of each chamber was tested in each period using a standard CH4 gas mixture (25% mol/mol balance N2; Air Korea). Briefly, a fixed volume of CH4 (50 ml/min) was injected into each chamber through a gas tube connected to a portable mass flow meter. The gas tube was placed near the feeder to accurately simulate animal emissions during feeding. The gas was allowed to mix for 10 min to achieve an equilibrium state with the inlet and outlet air passages closed. After 10 min, the inlet and outlet air passages were opened; inlet and outlet gases were sampled as described above. The difference in CH4 concentration between outlet and inlet gases (DCH4 ppm), known ventilation rate (wet VR), and the known injected CH4 concentration (InCH4ml/min) were used to calculate the gas recovery rates of the chamber using the following formula:
During the experiment, the same routine operations were conducted when animals were placed in the chamber, and CH4 emission was calculated using the formula:
The same formula and gas recovery rate (%) were used to determine CO2 emissions by substituting the values of DCH4 ppm with DCO2 ppm. For the determination of O2 consumption, the oxygen DO2% observed during the experiment was normalized and scaled up with the DO2% observed during the recovery test.
2.2.2 Digestibility and N balance trial
Feces and urine collection were performed for four consecutive days beginning on days 29 and 42 of the TMR and TMR-PA periods, respectively, immediately after gas measurements. For collection of total feces and urine, clear PVC sheets were detached from the chamber and the chamber itself was used as a metabolic crate. The animals were housed on rubber mattresses and allowed free access to feed and fresh drinking water. The feces voided daily from each animal on the rubber mat were collected using a shovel and dragged into a stainless-steel box located at the end of each metabolic crate. The daily total feces output was collected at 09:00 each day and recorded. The feces were then homogenized; 10% of the total feces from each animal was sampled and stored at −20°C. The total urine excreted from each animal was also collected daily using a custom-made pyramidal silicone funnel attached to the animal's lower body. The funnel was connected to a rotary pump and the excreted urine drained directly into clean 25-L airtight plastic containers via polypropylene tubing. In each container, 300 ml of 4N H2SO4 were included daily to acidify urine upon entry. The daily total urine output was recorded and homogenized; 10% of the urine volume from each animal was sampled and stored at −20°C. All daily subsamples were mixed; 10% of the total mixed sample was retained and used for further analysis.
2.2.3 Chemical analyses
Feed, refusals, PA, and feces were dried in a forced-air oven at 65°C for 72 h, then ground to pass through a 1-mm screen (Thomas Scientific Model 4, NJ, USA). The ground samples were subjected to DM analysis by drying at 105°C for 3 h. The OM was determined after ashing at 600°C using a Nabertherm LE 14/11/R7 Compact Muffle Furnace (Bahnhofstr, Lilienthal, Germany) for 3 h (28). Ether extract (EE) was determined using an ANKOMXT15 Extractor (ANKOM Technology Corp., Fairport, NY, USA) after a filter bag procedure (29) with petroleum ether as the solvent. NDF and acid detergent fiber were measured using the ANKOM A2000 fiber analyzer (ANKOM Technology Corp.) filter bag technique. NDF content was determined with a heat-stable amylase and expressed exclusive of residual ash (amylase neutral detergent fiber organic matter [aNDFom] (30). Acid detergent fiber was measured using the method of Van Soest (31), and the results were presented excluding residual ash (aNDFom). The N contents of feeds, refusals, PA, feces, and acidified urine were determined using the Kjeldahl method (Kjeltec Auto Sampler System, 8400 Analyzer; Foss, Sweden) as described in AOAC method 981.10; 2016. CP was calculated as 6.25 × N. The gross energies (GEs) of feeds, refusals, PA, dried feces, and acidified urine were determined using an automatic isoperibol calorimeter (6400EF, Parr Instrument Company, Moline, IL, USA). FA concentrations in feed and PA were measured by the direct methylation method (32), using previously described analysis techniques (33).
The ammonium nitrogen (NH3-N) concentration in rumen fluid obtained from experiment 2 (described below) was determined using a modified colorimetric method (34). For determination of volatile fatty acids (VFAs), a 5.0-ml aliquot of rumen fluid was mixed with 1.0 ml 25% HPO3 and 0.2 ml 2% pivalic acid (35); the mixture was analyzed using previously described methods (36).
2.2.4 Calculations
Energy partitioning was determined by subtracting the energy losses in feces, urine, and CH4, as well as daily heat production, from the GE intake as detailed in Sinz et al. (15). Heat energy (HE) was calculated with correction for the assumed CO2 production from microbial fermentation based on the equation given in Chawlibog et al. (37).
2.3 Experiment 2: effect of PA on rumen fermentation and protozoal populations in Holstein steers
In experiment 2, five rumen fistulated Holstein steers with an initial BW of 744.8 ± 15.8 kg (mean ± standard error) were randomly allocated to individual feeders equipped with steel stanchions. All animals were adapted to the same basal diet (Table 1) for 25 days (TMR) then supplemented with PA at 5% DM (TMR-PA) of the basal diet for 38 days. The diet was provided at 2% initial BW twice daily (09:00 and 18:00), and the animals had unrestricted access to water. Approximately 200 ml of ruminal fluid were collected from each animal through the cannula before feeding (0 h), and at 1.5 and 3 h after feeding, on the last day of each period; all fluid was strained through four layers of muslin. Immediately after the pH had been measured with a Seven Easy pH meter (Mettler-Toledo, Schwerzenbach, Switzerland), the ruminal fluid was transferred to a 50-ml centrifuge tube, snap-frozen in liquid N, and stored at −80°C until further analysis.
2.3.1 Quantification of rumen protozoal abundance using RT-qPCR
Rumen liquor collected after 3 h of morning feeding from cannulated Holstein steers was subjected to genomic DNA extraction using previously described methods (36). Real-time PCR assays to determine the relative abundances of major rumen protozoal species such as Entodinium caudatum, Epidinium caudatum, Eudiplodinium maggii, and Polyplastron multivesiculatum were performed using the SYBR Green Real-Time PCR Master Mix (Bioneer, Daejeon, Korea) and the CFX96 Touch™ Real-Time PCR Detection System with previously described analysis methods and PCR conditions (10). All primers (Table 2) were designed using the Primer-BLAST tool based on National Center for Biotechnology Information (NCBI) published sequences (www.ncbi.nlm.nih.gov, accessed on December 22, 2021). All primers were optimized for the annealing temperature with the highest product band intensity, which was determined by multiple gradient PCR assays. The primers targeted the 18S ribosomal region or other species-specific conserved coding region that lacked potential matches with other nucleotide sequences available in the NCBI. The 2−ΔΔCT method was used to determine relative fold-changes (38), and all data were normalized to the abundance of total protozoa.
2.4 Statistical analyses
A Shapiro–Wilk test was performed to check the normality of the data. When data were not normally distributed, they were transformed using the PROC RANK procedure of SAS (version 9.4, SAS Institute, Cary, NC, USA). Data were subjected to mixed-model analysis of variance in SAS, considering the treatment and period as fixed effects and animals as the random effect. Pairwise comparisons among least-squares means were performed by the Tukey-Kramer test. The ruminal fermentation characteristics (pH, VFA, and NH3) were analyzed by repeated-measures analysis of variance. The Akaike information criterion was used to determine which covariance structure had the best fit; based on the results, an “autoregressive (AR1)” analysis was chosen for assessment of fermentation characteristics. Means were calculated using the LSMEANS function, then compared using the PDIFF option in SAS. Differences were considered statistically significant at p < 0.05, and trends were considered at 0.05 ≤ p < 0.10.
3 Results
3.1 Chemical composition of the PA and diet
The proximate, FA composition, and metabolite profile of PA are reported in our previous study (25). Briefly, the analysis of the FA composition of PA revealed enrichment of saturated FAs (SFAs; 3.5 g/kg DM), monounsaturated fatty acids (MUFAs; 2.3 g/kg DM), and PUFAs (5.1 g/kg DM) dominated by linoleic acid (C18:2; 4.5 g/kg DM). Analytes such as caffeic acid, chlorogenate, quercetin, quercetin-3-O-glucoside and dioctyl sulfosuccinate were present in high intensities (25). The actual concentrations of these metabolites are yet to be determined.
The TMR offered to animals in both periods had a similar nutrient composition, except that the EE content of TMR-PA was 8% greater than the EE content of the TMR (Table 1) because of the higher EE concentration in PA (25). This also led to greater polyunsaturated FA and total FA concentrations in the TMR-PA diet (Supplementary Table 1) compared with TMR.
3.2 Effects of PA in Hanwoo steers
3.2.1 Intake, digestibility, and performance
There were no significant differences (p > 0.05) in nutrient intake across dietary treatments (Table 3), but an increase (p < 0.001) in apparent nutrient digestibility was observed with maximum increases of 8.4% and 18.6% in OM and NDF digestibility, respectively in TMR-PA compared with TMR. There were no changes (p > 0.05) in ADG or feed conversion ratio in response to PA supplementation.
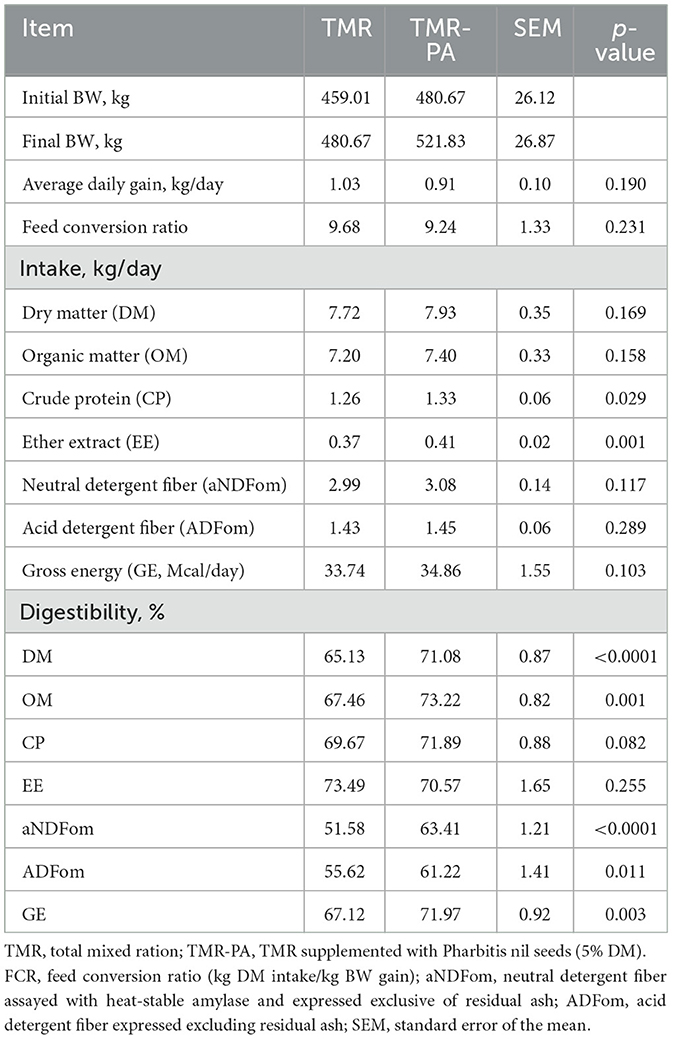
Table 3. Least-squares means of body weight, feed intake, and apparent total tract digestibility of Hanwoo steers (n = 6) supplemented with Pharbitis nil seeds at 5% DM (TMR-PA).
3.2.2 Methane emission
A pronounced decrease (p = 0.079) in daily absolute CH4 production was observed in animals supplemented with PA at 5% DM intake (TMR-PA; Table 4). Relative decreases (p < 0.05) of 17.2% and 17.5% were observed when the results were expressed as CH4 (g/kg OM) and CH4 (g/kg NDF), respectively. The reduction in CH4 yield was particularly pronounced (p < 0.005), with levels of 24.0% and 33.3% expressed for digestible OM and NDF intakes (Table 4). An 18.1% decrease in GE intake lost as CH4 (Ym) was also observed in animals supplemented with PA, relative to animals fed only TMR.
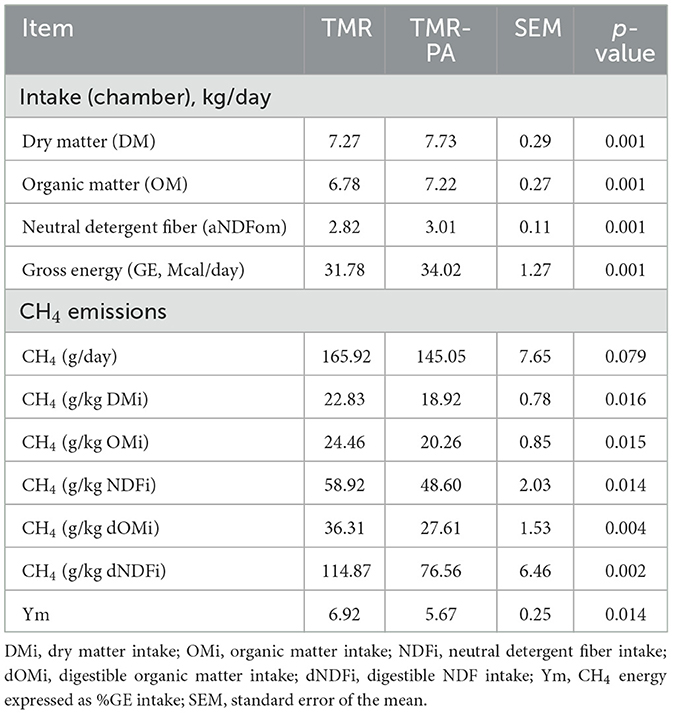
Table 4. Effects of Pharbitis nil seeds supplemented at 5% DM (TMR-PA) on enteric methane emission from Hanwoo steers (n = 6).
3.2.3 N balance
The supplementation of PA at 5% DM intake (TMR-PA) did not affect (p > 0.05) daily fecal or urinary N excretion amount (Table 5). However, when expressed as the proportion of total N intake, a 7.9% and 7.6% decrease (p < 0.05) in fecal and urinary N excretion were noted, respectively. Overall, a 7.7% decrease (p < 0.05) in total N excretion and 30.3% increase (p < 0.05) in N retention in the body were observed in animals fed PA compared with the non-PA-fed group (Table 5).
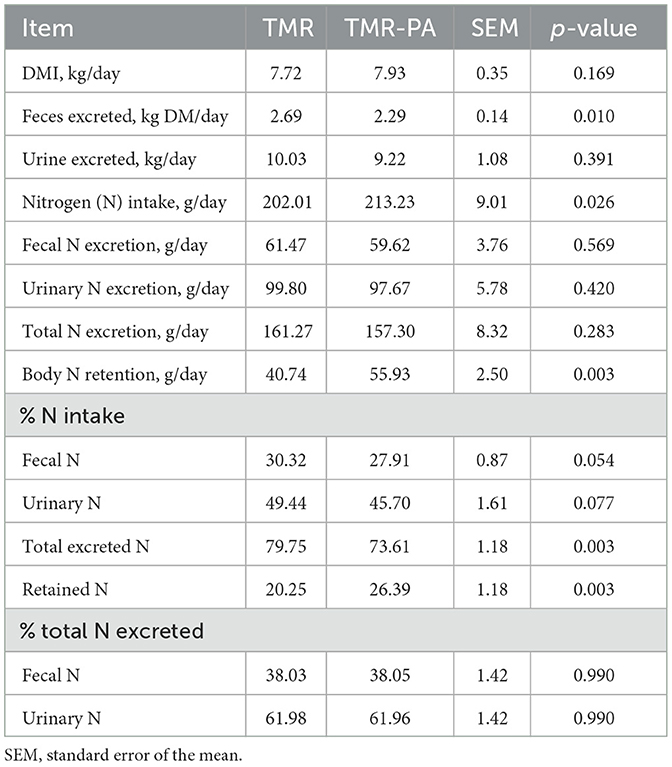
Table 5. Effects of Pharbitis nil seeds supplemented at 5% DM (TMR-PA) on nitrogen balance in Hanwoo steers (n = 6).
3.2.4 Energy balance
No difference (p > 0.05) in CO2 production was observed between the experimental groups (Table 6). The respiratory quotient was observed to be greater (p < 0.05) in TMR fed animals. Although the GE intake did not differ between treatments, the fecal, urine and CH4 energy losses decreased (p < 0.005) when PA was included in the diet, thereby increasing (p < 0.005) the supplies of digestible energy (DE) and metabolizable energy (ME) by 7.2% and 14.8%, respectively, when expressed as % GEI (Table 7). However, only a numerical increase (p > 0.05; Table 7) in retained energy (RE) was observed in animals supplemented with PA. The relationship between ME and DE differed (p < 0.005) between the treatments; correlation coefficients of 0.81 and 0.87 were obtained for the TMR and TMR-PA groups, respectively. The utilization efficiencies of the ME to RE were not significantly different (p > 0.05); they averaged 0.21 and 0.25 for the TMR and TMR-PA groups, respectively (Table 7).
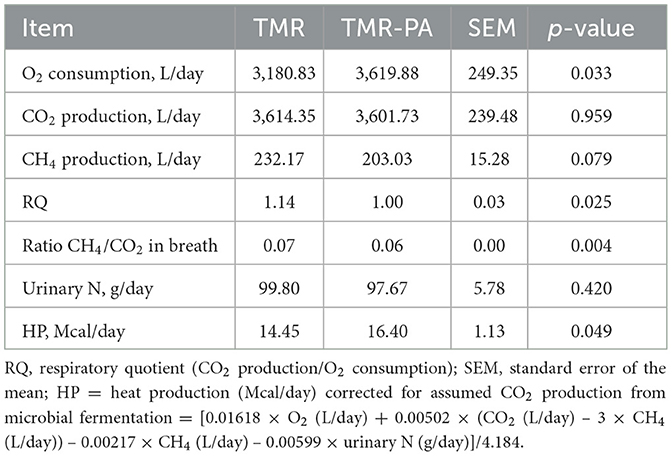
Table 6. Effects of Pharbitis nil seeds supplemented at 5% DM (TMR-PA) on heat production in Hanwoo steers (n = 6).
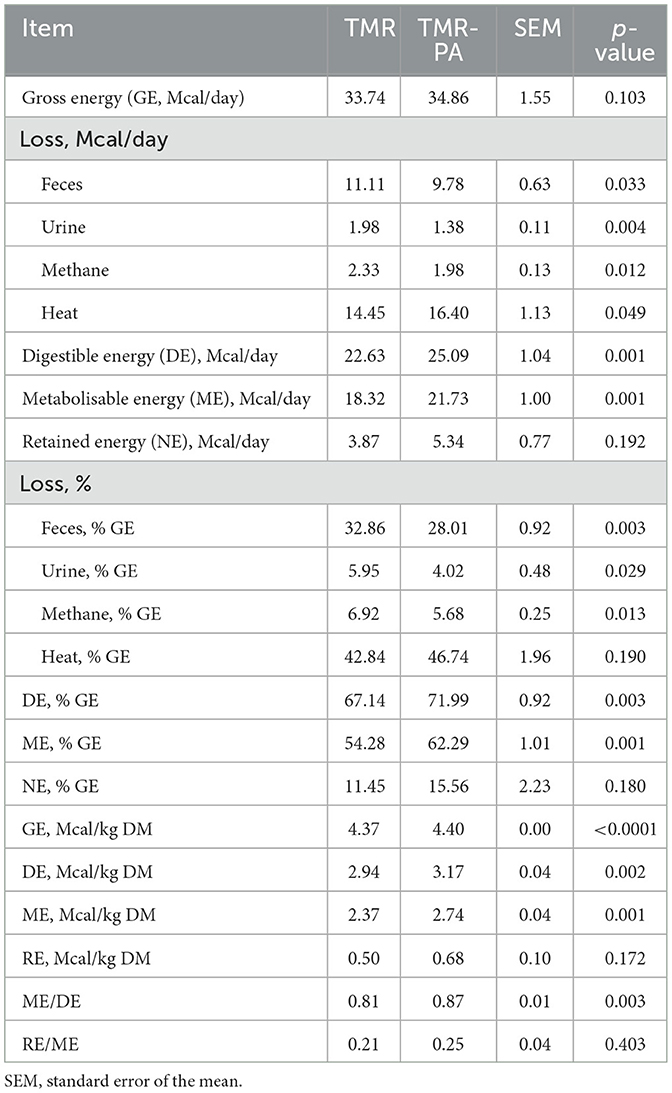
Table 7. Effects of Pharbitis nil seeds supplemented at 5% DM (TMR-PA) on energy balance in Hanwoo steers (n = 6).
3.3 Effects of PA on fermentation parameters and protozoal populations in Holstein steers
The average ruminal pH and NH3-N concentration decreased (p < 0.05) in cannulated Holstein steers supplemented with PA (Table 8). The NH3-N concentration was 56.3% lower in the TMR-PA group than in the TMR group after 1.5h feeding; it continued to remain lower after 3 h feeding. An increase (p = 0.087) in the total VFA concentration after 3 h of feeding, accompanied by a 26.6% increase (p < 0.05) in the propionate proportion, was also observed in the TMR-PA group. This clearly led to a decrease (p < 0.005) in the acetate/propionate ratio. Analysis of rumen protozoal populations revealed a 40% reduction (p = 0.062) in the relative abundance of the protozoal species En. caudatum among animals supplemented with PA (Figure 1). There were no significant differences in the abundances of other protozoal species.
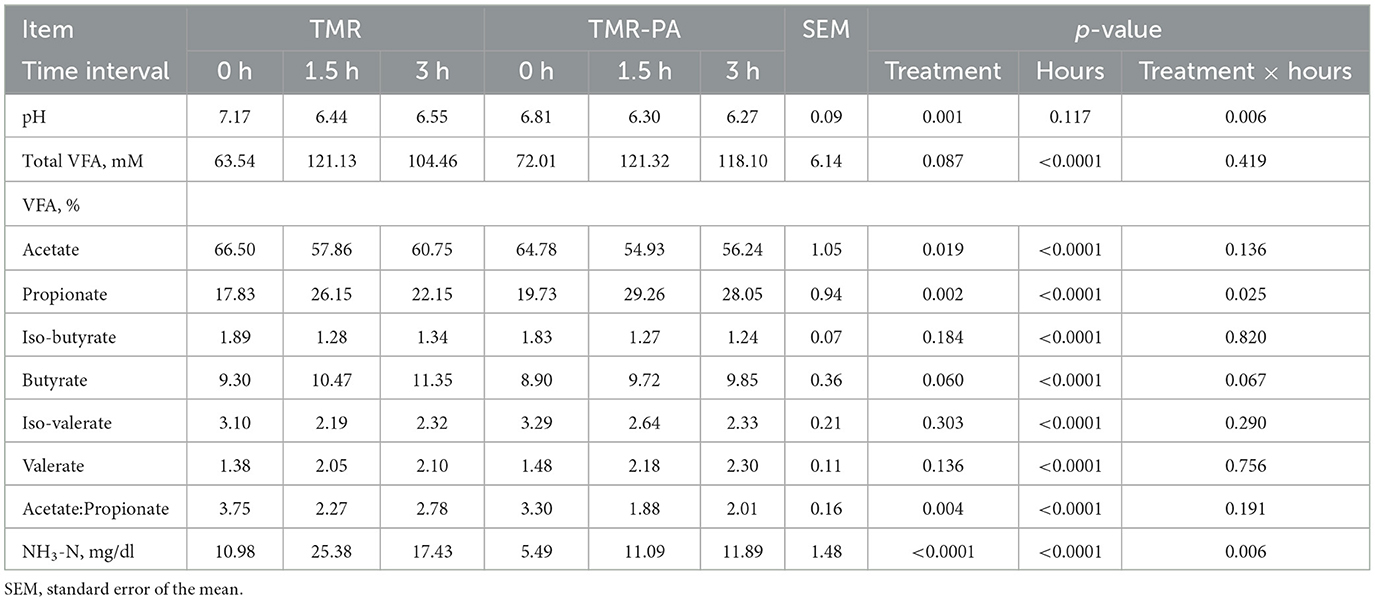
Table 8. Ruminal fermentation characteristics in cannulated Holstein steers supplemented with Pharbitis nil seeds at 5% DM (n = 5).
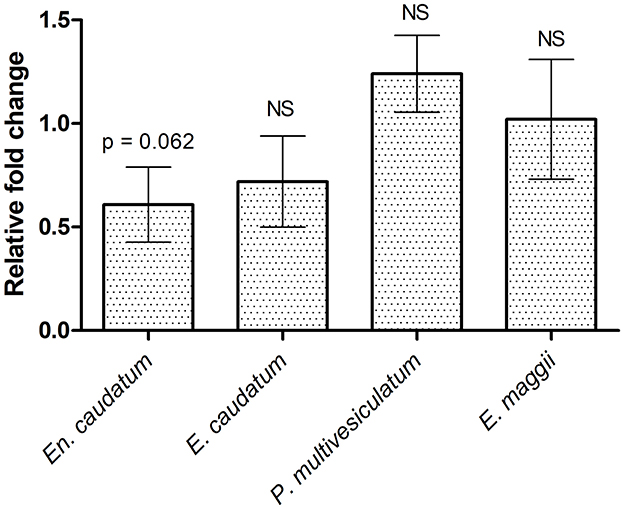
Figure 1. Effect of supplementing Pharbitis nil seeds at 5% DM intake on rumen protozoal abundance in cannulated Holstein steers (n = 5).
4 Discussion
Dietary strategies to mitigate CH4 and N emissions from ruminants must be commercially practical, without negative impacts on digestibility and animal performance. In our previous in vitro evaluations (10) we demonstrated the anti-methanogenic potential of P. nil seeds that are widely distributed in East Asian countries. We also observed increases in the in vitro DM and NDF digestibility with increasing supplementation of PA; the maximum response was recorded at 9% DM supplementation (25). A consistent response was present in the current in vivo trial at a supplemental level of 5% DM; the magnitude of increase in digestibility was comparable with our previous in vitro observation (25). This increased digestibility led to increased DE intake for animals in the treatment period compared with the control period, although there were no differences regarding GE intake. The increase in nutrient digestibility may be partially explained by the laxative effect of PA, which improved intestinal motility (24). There is evidence that altered intestinal motility and contractions create turbulence and convective currents that transport nutrients from the center of the lumen toward the epithelium, eventually improving nutrient absorption (39). Furthermore, dioctyl sulfosuccinate, an anionic surfactant substance traditionally recommended as a laxative and stool softener, was reported to be present in PA in our previous study (25). Another possible explanation is that better synchronization between carbohydrates and N occurred when less ammonia was available to rumen microbes in the treatment period, compared with the control period. This might have increased the growth of cellulolytic bacteria and enhanced fiber digestibility (40). The observed increase and decrease in rumen total VFA and pH in treatment period, respectively, provide further support to this hypothesis. Increases in fibrolytic bacterial populations were also observed in our previous study (25) when PA was incubated in the rumen of Holstein steers. Although the energy balance trial and rumen fermentation trial were performed in two different animal breeds, the basal diet fed was same in the both trials and hence relating their effects can be meaningful.
Greater NDF digestion stimulates acetate fermentation, which may enhance CH4 and CO2 emission (41). In this study, an inverse effect was observed that favored propionate production and decreased CH4 yield, consistent with our previous study (25). This effect led to a decrease in CH4 energy loss for animals in the treatment period, compared with the control period. Furthermore, the observed CH4 yield and Ym from Hanwoo steers fed TMR in the experiment were comparable with previously reported values (42). The proportion of CH4 energy loss to DE intake in the present study (10.3%) was comparable with values from growing Hanwoo steers fed different diets, as reported in our previous study (43). However, the magnitude of CH4 reduction via PA supplementation in the present study was 2.5-fold greater than the magnitude observed in our previous in vitro study with a similar level of PA supplementation (25). There are several possible reasons for this, including differences in culture system (44), animal breed (42), and diet. Furthermore, CH4 reduction in the treatment period may be partially explained by the greater polyunsaturated FA concentration, including dietary linoleic acid (C18:2n6c) from the supplemented PA (25). The role of the dietary FA concentration in ruminal CH4 production is well-known (45). Beauchemin et al. (46) reported that a decrease in CH4 yield (g/kg DM intake) was accompanied by a decrease in the number of rumen protozoa when cows were fed crushed linseed, similar to our results. Defaunation has been associated with lower levels of methanogenesis (26) due to the symbiotic relationship between methanogens and protozoa (47). Dohme et al. (48) also reported the antiprotozoal actions of FAs, including C18:2n6c, which produce considerable decreases in CH4 production. However, in the present study, supplementation with PA alone increased the added FA concentration by 0.4%, which was insufficient to achieve the observed reductions in protozoal population and CH4 (45). This result suggests that other metabolites in PA (e.g., quercetin and quercetin-3-O-glucoside) exhibit antiprotozoal action, as demonstrated in previous studies (49, 50). In our previous studies, we observed a moderate binding of these metabolites to the cyclic guanosine monophosphate-dependent protein kinase of En. Caudatum (25), and also a drastic decrease in total protozoal population in PA treated in vitro rumen buffer (10), further supporting our results. However, the concentrations of these metabolites in PA have not been quantified. Furthermore, Entodinium spp. reportedly constitute only 10–15% of the total protozoal population; they are dominated by Ophryoscolex spp. or other unclassified families (51, 52). Therefore, the decrease in En. caudatum abundance alone could not completely explain the decrease in CH4 production observed in this study. Another possible factor may be the laxative effect of PA, which could have increased the rate of feed passage and requires further investigation. The elimination of rumen protozoa is consistently associated with an increase in the propionate proportion (2), which matches the observed 26.6% increase in propionate proportion during the treatment period in Holstein steers. This finding suggested that propionate synthesis was the major H2 sink, thereby reducing H2 available for CH4 synthesis in animals supplemented with PA (41). Furthermore, defaunation has been related to a decrease in nutrient digestibility (26); such a decrease was not observed in the present study. This was possibly related to the lack of change in the abundances of cellulolytic genera such as Epidinium, Polyplastron, and Eudiplodinium, and a decrease in the abundance of Entodinium, which is weakly hemicellulolytic (53).
The increases in ruminal protein degradation and peptide deamination cause increased production of NH3-N, which is absorbed through the rumen wall and excreted as urea in urine, leading to N losses in a highly volatile form (54). The 56% decrease in the rumen NH3-N concentration in cannulated Holstein steers supplemented with PA could be related to the decreased urinary N excretion and increased N retention in Hanwoo steers in the current experiment. These findings suggest that the ruminal degradability of dietary CP is reduced, which may be related to the overall decrease in protozoa observed in our current and previous studies (25). However, there was no negative effect on the total tract digestibility of CP, which suggested hind gut digestion and CP utilization. Additionally, consistent with the present study, defaunation is associated with lower NH3-N levels in rumen fluid (2), due to a decrease in the microbial grazing of En. caudatum (55), which deaminates the engulfed microbial protein to NH3-N (26). Previous studies (49, 50) have also shown decreases in protozoal ammoniagenesis after the addition of plant extracts that (like PA) are rich in quercetin and quercetin-3-O-glucoside. The minimum required NH3-N concentration for maximum microbial growth in the rumen is 5 mg/dl (56). A previous study showed that levels of 5–8 mg/dl were sufficient to favor fiber digestion, whereas levels of >8 mg/dl did not increase feed degradation in the rumen (57). The NH3-N concentration of 5.5–11.9 mg/dl upon supplementation with PA would therefore meet the minimum requirement for microbial protein synthesis. However, studies on the rumen microbial protein synthesis should be carried out to further support the increased N utilization noted in PA fed animals. The proportion of N retained from N intake for Hanwoo steers fed only a basal diet (18%−20%) observed in this study was bit greater than proportions (16%−18%) observed in other breeds of beef cattle (13, 58, 59).
The decrease in urinary N excretion in animals supplemented with PA also led to a decrease in the urinary energy (UE) content (from 6% GE intake to 4% GE intake) because UE is closely associated with UN due to the higher energy contents of urinary hippuric acids, amino acids, and creatinine (60). The ratio of UE losses to the GE and DE intakes (8.8%) in the present study was much higher than the ratio among Hanwoo steers in the early fattening stage, reported in our previous work (43, 61). This discrepancy may be related to the greater dietary CP content of 16.6% in the present study, compared with the value of 12.5% in the previous studies, which might have increased the UN excretion while enhancing UE loss.
The decreased ratio of energy intake to energy excretion in feces, urine, and enteric CH4 resulted in greater ME intake in animals supplemented with PA compared with animals fed only TMR. The observed DE intake and ME intake for Hanwoo steers in the present study were comparable with the results in our previous work that consolidated many studies involving Hanwoo steers with different BWs and diets (43). Additionally, the DE intake and ME intake values were within the ranges of values reported for other beef cattle breeds (1.8–3.8 and 1.4–3.5 Mcal/kg of DM, respectively) (62). The observed efficiency of converting DE to ME in animals fed a basal diet in the present study (0.81) was similar to the efficiencies recommended by the Agricultural Research Council (63), National Research Council (64), and Commonwealth Scientific and Industrial Research Organization (65). However, it was below the efficiencies within the British feeding system (66) and other recent studies (43, 62, 67), which ranged from 0.86 to 0.90 for several beef cattle breeds (Hanwoo, Friesian, Hereford, Brahman, Thai, Angus, Hereford-Angus, Jersey, MARC II composite, Holstein, Charolais-cross, and Continental-British). Similarly, the utilization efficiency of ME (RE:ME) was 0.21 under 2.4 Mcal/kg DM of ME intake, which was lower than the reported value of 0.29 considering feeds with ME intakes of 2.0 Mcal/kg DM (64). However, this discrepancy may be due to differences in animal breeds and diets between experiments. Moreover, the ME:DE (0.87) and RE:ME (0.25) ratios for animals supplemented with PA were similar to the ratios for suggested values (43, 62, 67), presumably due to increases in DE intake and ME intake upon supplementation with PA.
Increases in ruminant productivity (e.g. ADG) via reduction of enteric CH4 emissions and enhancement of energy conversion efficiency is the desired outcome of a CH4 mitigation strategy. Similarly, Li and Guan (68) reported that increases in N metabolism and utilization contributed to increased feed efficiency in beef cattle. However, decreases in CH4, N emission, and other energy losses in the treatment period did not significantly contribute to the increase in RE, may be due to the increased heat energy loss. This result might also be due to the digestion of metabolites such as phenols and tannins (chlorogenate in high intensities) in PA (25), which may require detoxification with the cost of additional energy (69). Furthermore, CH4 mitigation and urinary N mitigation in the treatment period were insufficient for reduction of the calculated HE because small coefficients for CH4 and urinary N were used in the equation for HE estimation. As a result, RE only increased by 1.3 Mcal/day in the treatment period; which eventually did not contribute much to changes in the ADG and feed conversion efficiency. There were also individual animal variations in heat production that contributed to the lack of statistical significance for RE, suggesting that future studies should be conducted over a longer period with additional animal replicates.
5 Conclusions
Seeds of P. nil demonstrated promise for mitigating CH4 emissions from in vitro rumen fermentation studies. They were very efficient in live animals, leading to a 17.2% decrease in CH4 yield (g/kg OM) when supplemented at 5% DM. The supplemented feed was also palatable, demonstrated high nutritional value and a 7.6% decrease in urinary N loss, and exhibited a >10% increase in nutrient digestibility. Metabolizability (ME/GE) and ME utilization (RE/ME) were also improved, suggesting that PA was a valid alternative to ionophores or other growth promoters. Greater retention of dietary N and E may contribute to improvements in production parameters, thereby reducing age at slaughter, with environmental and economic benefits. However, the increase in heat production was a notable limitation. Future studies should focus on profiling rumen and blood metabolites to identify any potential PA-induced toxicity related to the increased HE. The rumen microbial metagenome should also be evaluated to better understand the beneficial effects of PA on digestibility and energy conversion efficiency.
Data availability statement
The original contributions presented in the study are included in the article/Supplementary material, further inquiries can be directed to the corresponding authors.
Ethics statement
The animal study was approved by Committee for the Institutional Animal Care and Use of Seoul National University (SNU-210615-3). The study was conducted in accordance with the local legislation and institutional requirements.
Author contributions
RB: Conceptualization, Data curation, Investigation, Methodology, Software, Validation, Writing – original draft, Writing – review & editing, Formal analysis, Visualization. PX: Formal analysis, Resources, Writing – review & editing. WH: Formal analysis, Writing – review & editing. TK: Formal analysis, Writing – review & editing. JB: Formal analysis, Writing – review & editing. YL: Formal analysis, Resources, Writing – review & editing. BT: Formal analysis, Writing – review & editing. KK: Conceptualization, Funding acquisition, Project administration, Supervision, Writing – review & editing. RI: Formal analysis, Supervision, Writing – review & editing.
Funding
The author(s) declare financial support was received for the research, authorship, and/or publication of this article. This study was supported by the Korea Institute of Planning and Evaluation for Technology in Food, Agriculture, Forestry and Fisheries (IPET; project no. IP322099).
Acknowledgments
The authors thank Dong Hyeon Lim (Dairy Science Division, National Institute of Animal Sciences, Rural Development Administration, Cheonan 31000, Republic of Korea) and Yongjun Choi (School of Animal Life Convergence Science, Hankyung National University, Anseong 17579, Republic of Korea) for their helpful comments and critical reading of the manuscript.
Conflict of interest
The authors declare that the research was conducted in the absence of any commercial or financial relationships that could be construed as a potential conflict of interest.
Publisher's note
All claims expressed in this article are solely those of the authors and do not necessarily represent those of their affiliated organizations, or those of the publisher, the editors and the reviewers. Any product that may be evaluated in this article, or claim that may be made by its manufacturer, is not guaranteed or endorsed by the publisher.
Supplementary material
The Supplementary Material for this article can be found online at: https://www.frontiersin.org/articles/10.3389/fvets.2024.1467077/full#supplementary-material
References
1. Appuhamy JADRN, France J, Kebreab E. Models for predicting enteric methane emissions from dairy cows in North America, Europe, and Australia and New Zealand. Glob Chang Biol. (2016) 22:3039–56. doi: 10.1111/gcb.13339
2. Dai X, Faciola AP. Evaluating strategies to reduce ruminal protozoa and their impacts on nutrient utilization and animal performance in ruminants – a meta-analysis. Front Microbiol. (2019) 10:2648. doi: 10.3389/fmicb.2019.02648
3. FAOSTAT. Food and Agriculture Organization of the United Nations statistical database. (2021). Available at: http://www.fao.org/faostat/en/#data/GT (accessed July 11, 2023).
4. UNFCCC. The Republic of Korea's Update of its First Nationally Determined Contribution. (2020). Available at: https://www4.unfccc.int/sites/ndcstaging/PublishedDocuments/Republic%20of%20Korea%20First/201230_ROK%27s%20Update%20of%20its%20First%20NDC_editorial%20change.pdf (accessed July 11, 2023).
5. UNFCCC. 2050 carbon neutral strategy of the Republic of Korea. (2020). Available at: https://unfccc.int/sites/default/files/resource/LTS1_RKorea.pdf (accessed July 11, 2023).
6. KOSIS. Korean Statistical Information Service; Number of Farms in households and Animals in heads by type. (2024). Available at: http://kosis.kr/statHtml/statHtml.do?orgId=101&tblId=DT_1EO200&language=en&conn_path=I3 (accessed July 11, 2023).
7. Beauchemin KA, Ungerfeld EM, Eckard RJ, Wang M. Review: fifty years of research on rumen methanogenesis: lessons learned and future challenges for mitigation. Animal. (2020) 14:S2–16 doi: 10.1017/S1751731119003100
8. Hartinger T, Gresner N, Südekum KH. Does intra-ruminal nitrogen recycling waste valuable resources? A review of major players and their manipulation. J Anim Sci Biotechnol. (2018) 9:1–21. doi: 10.1186/s40104-018-0249-x
9. Macheboeuf D, Coudert L, Bergeault R, Lalière G, Niderkorn V. Screening of plants from diversified natural grasslands for their potential to combine high digestibility, and low methane and ammonia production. Animal. (2014) 8:1797–806. doi: 10.1017/S1751731114001785
10. Bharanidharan R, Arokiyaraj S, Baik M, Ibidhi R, Lee SJ, Lee Y, et al. In vitro screening of east Asian plant extracts for potential use in reducing ruminal methane production. Animals. (2021) 11:1020. doi: 10.3390/ani11041020
11. Wang S, Terranova M, Kreuzer M, Marquardt S, Eggerschwiler L, Schwarm A. Supplementation of pelleted hazel (Corylus avellana) leaves decreases methane and urinary nitrogen emissions by sheep at unchanged forage intake. Sci Rep. (2018) 8:1–10. doi: 10.1038/s41598-018-23572-3
12. Terranova M, Eggerschwiler L, Ortmann S, Clauss M, Kreuzer M, Schwarm A. Increasing the proportion of hazel leaves in the diet of dairy cows reduced methane yield and excretion of nitrogen in volatile form, but not milk yield. Anim Feed Sci Technol. (2021) 276:114790. doi: 10.1016/j.anifeedsci.2020.114790
13. Morales SGD, Rosales RB, Vergara DMB, Chirinda N, Arango J. Feeding strategies to increase nitrogen retention and improve rumen fermentation and rumen microbial population in beef steers fed with tropical forages. Sustainability. (2021) 13:10312. doi: 10.3390/su131810312
14. Huyen NT, Desrues O, Alferink SJJ, Zandstra T, Verstegen MWA, Hendriks WH, et al. Inclusion of sainfoin (Onobrychis viciifolia) silage in dairy cow rations affects nutrient digestibility, nitrogen utilization, energy balance, and methane emissions. J Dairy Sci. (2016) 99:3566–77. doi: 10.3168/jds.2015-10583
15. Sinz S, Leparmarai PT, Liesegang A, Ortmann S, Kreuzer M, Marquardt S. Effects of dietary grapeseed extract on performance, energy and nitrogen balance as well as methane and nitrogen losses of lambs and goat kids. Br J Nutr. (2021) 125:26–37. doi: 10.1017/S0007114520002512
16. Kaewpila C, Sommart K, Mitsumori M. Dietary fat sources affect feed intake, digestibility, rumen microbial populations, energy partition and methane emissions in different beef cattle genotypes. Animal. (2018) 12:2529–38. doi: 10.1017/S1751731118000587
17. Wang S, Giller K, Kreuzer M, Ulbrich SE, Braun U, Schwarm A. Contribution of ruminal fungi, archaea, protozoa, and bacteria to the methane suppression caused by oilseed supplemented diets. Front Microbiol. (2017) 8:1864. doi: 10.3389/FMICB.2017.01864/BIBTEX
18. Lagrange SP, Macadam JW, Villalba JJ. The use of temperate tannin containing forage legumes to improve sustainability in forage–livestock production. Agronomy. (2021) 11:2264. doi: 10.3390/agronomy11112264
19. Verma S, Taube F, Malisch CS. Examining the variables leading to apparent incongruity between antimethanogenic potential of tannins and their observed effects in ruminants—a review. Sustainability. (2021) 13:1–24. doi: 10.3390/su13052743
20. Yanza YR, Fitri A, Suwignyo B, Elfahmi, Hidayatik N, Kumalasari NR, et al. The utilisation of tannin extract as a dietary additive in ruminant nutrition: a meta-analysis. Animals. (2021) 11:3317. doi: 10.3390/ani11113317
21. Ono M. Resin glycosides from Convolvulaceae plants. J Nat Med. (2017) 71:591–604. doi: 10.1007/s11418-017-1114-5
22. Saito N, Cheng J, Ichimura M, Yokoi M, Abe Y, Honda T. Flavonoids in the acyanic flowers of Pharbitis nil. Phytochemistry. (1994) 35:687–91. doi: 10.1016/S0031-9422(00)90588-0
23. Kim KH, Ha SK, Choi SU, Kim SY, Lee KR. Bioactive phenolic constituents from the seeds of Pharbitis nil. Chem Pharm Bull. (2011) 59:1425–9. doi: 10.1248/cpb.59.1425
24. Kim YS, Kim JW, Ha NY, Kim J, Ryu HS. Herbal therapies in functional gastrointestinal disorders: a narrative review and clinical implication. Front Psychiatry. (2020) 11:601. doi: 10.3389/fpsyt.2020.00601
25. Bharanidharan R, Thirugnanasambantham K, Ibidhi R, Baik M, Kim TH, Lee Y, et al. Metabolite profile, ruminal methane reduction, and microbiome modulating potential of seeds of Pharbitis nil. Front Microbiol. (2022) 13:1466. doi: 10.3389/fmicb.2022.892605
26. Newbold CJ. De la Fuente G, Belanche A, Ramos-Morales E, McEwan NR. The role of ciliate protozoa in the rumen. Front Microbiol. (2015) 6:1313. doi: 10.3389/fmicb.2015.01313
27. Cesar P, Garry W. Technical manual on respiration chamber designs. (2014). Available at: https://books.google.com/books/about/Technical_Manual_on_Respiration_Chamber.html?id=coa6DAEACAAJ (accessed August 9, 2024).
28. Undersander D, Mertens DR, Thiex N. Forage analysis procedures. Omaha, NE: National Forage Testing Association (1993).
29. Latimer G. Official Methods of Analysis of AOAC International, 21st Edition. AOAC International 2019–2022. (2019). Available at: https://www.aoac.org/official-methods-of-analysis-21st-edition-2019/ (accessed May 11, 2021).
30. Van Soest PJ, Robertson JB, Lewis BA. Methods for dietary fiber, neutral detergent fiber, and nonstarch polysaccharides in relation to animal nutrition. J Dairy Sci. (1991) 74:3583–97. doi: 10.3168/jds.S0022-0302(91)78551-2
31. Van Soest PJ. Collaborative study of acid-detergent fiber and lignin. J AOAC Int. (1973) 56:781–4. doi: 10.1093/jaoac/56.4.781
32. O'Fallon JV, Busboom JR, Nelson ML, Gaskins CT. A direct method for fatty acid methyl ester synthesis: application to wet meat tissues, oils, and feedstuffs. J Anim Sci. (2007) 85:1511–21. doi: 10.2527/jas.2006-491
33. Bharanidharan R, Thirugnanasambantham K, Ibidhi R, Bang G, Jang SS, Baek YC, et al. Effects of dietary protein concentration on lipid metabolism gene expression and fatty acid composition in 18–23-month-old Hanwoo steers. Animals. (2021) 11:3378. doi: 10.3390/ani11123378
34. Chaney AL Marbach EP. Modified reagents for determination of urea and ammonia. Clin Chem. (1962) 8:130–2. doi: 10.1093/clinchem/8.2.130
35. Erwin ES, Marco GJ, Emery EM. Volatile fatty acid analyses of blood and rumen fluid by gas chromatography. J Dairy Sci. (1961) 44:1768–71. doi: 10.3168/JDS.S0022-0302(61)89956-6
36. Bharanidharan R, Arokiyaraj S, Kim EB, Lee CH, Woo YW, Na Y, et al. Ruminal methane emissions, metabolic, and microbial profile of Holstein steers fed forage and concentrate, separately or as a total mixed ration. PLoS ONE. (2018) 13:e0202446. doi: 10.1371/journal.pone.0202446
37. Chwalibog A, Jensen K, Thorbek G. Oxidation of nutrients in bull calves treated with β-adrenergic agonists. Arch Anim Nutr. (1996) 49:255–61. doi: 10.1080/17450399609381888
38. Livak KJ, Schmittgen TD. Analysis of relative gene expression data using real-time quantitative PCR and the 2-ΔΔCT method. Methods. (2001) 25:402–8. doi: 10.1006/meth.2001.1262
39. Müller M, Canfora EE, Blaak EE. Gastrointestinal transit time, glucose homeostasis and metabolic health: modulation by dietary fibers. Nutrients. (2018). 10:275 doi: 10.3390/nu10030275
40. Zhang J, Zheng N, Shen W, Zhao S, Wang J. Synchrony degree of dietary energy and nitrogen release influences microbial community, fermentation, and protein synthesis in a rumen simulation system. Microorganisms. (2020) 8:231. doi: 10.3390/microorganisms8020231
41. Moss AR, Jouany JP, Newbold J. Methane production by ruminants: its contribution to global warming. Anim Res. (2000) 49:231–53. doi: 10.1051/animres:2000119
42. Bharanidharan R, Lee CH, Thirugnanasambantham K, Ibidhi R, Woo YW, Lee HG, et al. Feeding systems and host breeds influence ruminal fermentation, methane production, microbial diversity and metagenomic gene abundance. Front Microbiol. (2021) 12:2038. doi: 10.3389/fmicb.2021.701081
43. Ibidhi R, Bharanidharan R, Kim JG, Hong WH, Nam IS, Baek YC, et al. Developing equations for converting digestible energy to metabolizable energy for korean hanwoo beef cattle. Animals. (2021) 11:1696. doi: 10.3390/ani11061696
44. Yang W. Factors affecting rumen fermentation using batch culture technique. In:AF Jozala, , editor. Fermentation Processes. London: IntechOpen (2017). doi: 10.5772/64207
45. Patra AK. The effect of dietary fats on methane emissions, and its other effects on digestibility, rumen fermentation and lactation performance in cattle: a meta-analysis. Livest Sci. (2013) 155:244–54. doi: 10.1016/j.livsci.2013.05.023
46. Beauchemin KA, McGinn SM, Benchaar C, Holtshausen L. Crushed sunflower, flax, or canola seeds in lactating dairy cow diets: effects on methane production, rumen fermentation, and milk production. J Dairy Sci. (2009) 92:2118–27. doi: 10.3168/jds.2008-1903
47. Levy B, Jami E. Exploring the prokaryotic community associated with the rumen ciliate protozoa population. Front Microbiol. (2018) 9:2526. doi: 10.3389/fmicb.2018.02526
48. Dohme F, Machmüller A, Wasserfallen A, Kreuzer M. Ruminal methanogenesis as influenced by individual fatty acids supplemented to complete ruminant diets. Lett Appl Microbiol. (2008) 32:47–51. doi: 10.1111/j.1472-765x.2001.00863.x
49. Ayemele AG, Ma L, Park T, Xu J, Yu Z, Bu D. Giant milkweed (Calotropis gigantea): a new plant resource to inhibit protozoa and decrease ammoniagenesis of rumen microbiota in vitro without impairing fermentation. Sci Total Environ. (2020) 743:140665. doi: 10.1016/j.scitotenv.2020.140665
50. Ayemele AG, Ma L, Li X, Yang P, Xu J, Yu Z, et al. Identification of bioactive phytochemicals from six plants: mechanistic insights into the inhibition of rumen protozoa, ammoniagenesis, and α-glucosidase. Biology. (2021) 10:1055. doi: 10.3390/biology10101055
51. Bailoni L, Carraro L, Cardin M, Cardazzo B. Active rumen bacterial and protozoal communities revealed by RNA-based amplicon sequencing on dairy cows fed different diets at three physiological stages. Microorganisms. (2021) 9:754. doi: 10.3390/microorganisms9040754
52. Zhang Y, Li F, Chen Y, Wu H, Meng Q, Guan LL. Metatranscriptomic profiling reveals the effect of breed on active rumen eukaryotic composition in beef cattle with varied feed efficiency. Front Microbiol. (2020) 11:367. doi: 10.3389/fmicb.2020.00367
53. Takenaka A, Tajima K, Mitsumori M, Kajikawa H. Fiber digestion by rumen ciliate protozoa. Microbes Environ. (2004) 19:203–10. doi: 10.1264/jsme2.19.203
54. Dijkstra J, Oenema O, van Groenigen JW, Spek JW, van Vuuren AM, Bannink A. Diet effects on urine composition of cattle and N2O emissions. Animal. (2013) 7(Suppl 2):292–302. doi: 10.1017/S1751731113000578
55. Park T, Yu Z. Do Ruminal ciliates select their preys and prokaryotic symbionts? Front Microbiol. (2018) 9:1710. doi: 10.3389/fmicb.2018.01710
56. Satter LD, Slyter LL. Effect of ammonia concentration on rumen microbial protein production in vitro. Br J Nutrit. (1974) 32:199–208. doi: 10.1079/bjn19740073
57. Firkins JL Yu Z, Morrison M. Ruminal nitrogen metabolism: perspectives for integration of microbiology and nutrition for dairy. J Dairy Sci. (2007) 90:E1–E16. doi: 10.3168/jds.2006-518
58. Beltran IE, Calvache I, Cofre R, Salazar F, Keim JP, Morales A, et al. Nitrogen intake and its partition on urine, dung and products of dairy and beef cattle in Chile. Agronomy. (2022) 12:15. doi: 10.3390/agronomy12010015
59. Lee C, Araujo RC, Koenig KM, Beauchemin KA, Croy D, Cushman N, et al. Effects of encapsulated nitrate on enteric methane production and nitrogen and energy utilization in beef heifers. J Anim Sci. (2015) 93:2391–404. doi: 10.2527/JAS.2014-8845
60. Morris DL, Firkins JL, Lee C, Weiss WP, Kononoff PJ. Relationship between urinary energy and urinary nitrogen or carbon excretion in lactating Jersey cows. J Dairy Sci. (2021) 104:6727–38. doi: 10.3168/jds.2020-19684
61. Kim KH, Oh YG, Kim W, Lee SC, Shin KJ, Jeon BT. Determination of energy requirements for maintenance in Hanwoo steers. J Anim Sci Technol. (2004) 46:193–200. doi: 10.5187/jast.2004.46.2.193
62. Galyean ML, Cole NA, Tedeschi LO, Branine ME. Efficiency of converting digestible energy to metabolizable energy and reevaluation of the California net energy System maintenance requirements and equations for predicting dietary net energy values for beef cattle. J Anim Sci. (2016) 94:1329–41. doi: 10.2527/jas.2015-0223
63. Agricultural Research Council. The Nutrient Requirements of Ruminant Livestock. Technical Review by an Agricultural Research Council Working Party. Farnham Royal: Common wealth Agricultural Bureau (1980).
64. National Research Council. Nutrient Requirements of Dairy Cattle: Seventh Revised Edition. Washington, DC: National Research Council, Board on Agriculture and Natural Resources, Committee on Animal Nutrition, Subcommittee on Dairy Cattle Nutrition - Google Libros (2001).
65. The Commonwealth Scientific and Industrial Research Organisation (CSIRO). Nutrient Requirements of Domesticated Ruminants. Collingwood, VIC: CSIRO Publishing (2007).
66. Alderman G, Blake JS. The energy and protein requirements according to AFRC (1993) of high genetic merit dairy cows. BSAP Occas Publ. (1995) 19:99–101. doi: 10.1017/s0263967x00031864
67. Hales KE. Relationships between digestible energy and metabolizable energy in current feedlot diets. Transl Anim Sci. (2019) 3:945–52. doi: 10.1093/tas/txz073
68. Li F, Guan LL. Metatranscriptomic profiling reveals linkages between the active rumen microbiome and feed efficiency in beef cattle. Appl Environ Microbiol. (2017) 83:e00061–17. doi: 10.1128/AEM.00061-17
69. White RG, Lawler JP. Can methane suppression during digestion of woody and leafy browse compensate for energy costs of detoxification of plant secondary compounds? A test with muskoxen fed willows and birch. Comp Biochem Physiol A Mol Integr Physiol. (2002) 133:849–59 doi: 10.1016/S1095-6433(02)00152-6
Keywords: energy partitioning, Hanwoo, methane, nitrogen utilization, Pharbitis nil
Citation: Bharanidharan R, Xaysana P, Hong WH, Kim T, Byun JS, Lee Y, Tomple BM, Kim KH and Ibidhi R (2024) Methane emission, nitrogen excretion, and energy partitioning in Hanwoo steers fed a typical TMR diet supplemented with Pharbitis nil seeds. Front. Vet. Sci. 11:1467077. doi: 10.3389/fvets.2024.1467077
Received: 19 July 2024; Accepted: 13 August 2024;
Published: 24 September 2024.
Edited by:
Moyosore Joseph Adegbeye, University of Africa, NigeriaReviewed by:
Ravikanth Reddy Poonooru, University of Missouri, United StatesTaye Abegunde, Obafemi Awolowo University, Nigeria
Copyright © 2024 Bharanidharan, Xaysana, Hong, Kim, Byun, Lee, Tomple, Kim and Ibidhi. This is an open-access article distributed under the terms of the Creative Commons Attribution License (CC BY). The use, distribution or reproduction in other forums is permitted, provided the original author(s) and the copyright owner(s) are credited and that the original publication in this journal is cited, in accordance with accepted academic practice. No use, distribution or reproduction is permitted which does not comply with these terms.
*Correspondence: Kyoung Hoon Kim, a2hoa2ltJiN4MDAwNDA7c251LmFjLmty; Ridha Ibidhi, cmlkaGEuaWJpZGhpJiN4MDAwNDA7YWdyb3N1cGRpam9uLmZy