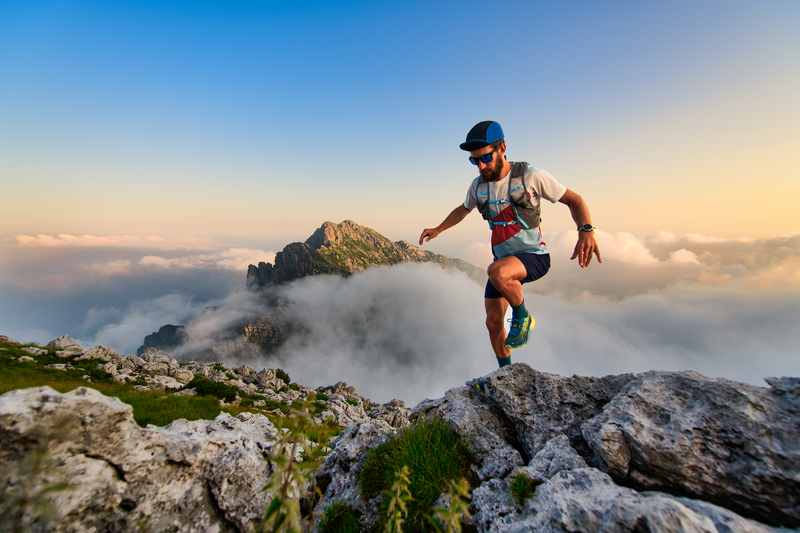
95% of researchers rate our articles as excellent or good
Learn more about the work of our research integrity team to safeguard the quality of each article we publish.
Find out more
ORIGINAL RESEARCH article
Front. Vet. Sci. , 21 October 2024
Sec. Veterinary Infectious Diseases
Volume 11 - 2024 | https://doi.org/10.3389/fvets.2024.1458069
Introduction: Enterococcus are considered an important genus in terms of Hospital-Acquired Infections (HAIs), which means that their characterization regarding resistance and virulence profiles in the hospital environment is of extreme importance. This article addresses this issue through the characterization of enterococci collected from a Veterinary Biological Isolation and Containment Unit (BICU).
Methods: A total of 73 isolates, collected from different surfaces of a Veterinary BICU, were identified as Enterococcus through PCR at species level, after which 34 isolates were selected as representatives using (GTG)5 fingerprinting. These isolates were further characterized phenotypically in terms of antimicrobial resistance through disk diffusion and of virulence factors’ expression.
Results: The majority of the enterococci isolated presented resistance to erythromycin (79.4%), ampicillin (73.5%), amoxicillin-clavulanic acid (70.6%), tetracycline (67.6%), ciprofloxacin (58.8%) and levofloxacin (50.0%), and were able to produce hemolysin (88.2%) and biofilm (82.3%). Furthermore, in terms of pathogenicity, three isolates (8.8%) were classified as high threats and two (5.9%) as moderate threats.
Discussion: The degree of resistance, production of virulence factors and the percentage of isolates classified as moderate or high threat means that a constant vigilance of such strains in veterinary units, but also in clinics and hospitals in general, is an important tool in terms of infection prevention and consequent reduction of HAIs.
Enterococci, particularly Enterococcus faecalis and E. faecium, have gained significant attention due to their emerging role in Hospital-Acquired Infections (HAIs) within human and veterinary settings. According to the last European Centre for Disease Prevention and Control (ECDC) report, enterococci accounted for 18.9% of intensive care unit (ICU)-acquired bloodstream infections and 23.0% of ICU-acquired urinary tract infections in patients admitted to ICUs in 2020, being the second most prevalent bacteria in both cases (1). Additionally, another report by the ECDC referred that, between 2018 and 2020, 17.6% of surgical site infections were cause by Enterococcus spp., only surpassed by Staphylococcus spp. (29.2%) (2).
Not being highly virulent organisms, their capacity to cause infection mainly derives from: (i) their ability to linger in the environment for long periods of time due to their biofilm-forming capacity (3); (ii) their reduced susceptibility to many antimicrobial agents such as β-lactams, aminoglycosides, and glycopeptides (3–6); (iii) and the plasticity of their genome, which allows them to easily acquire, conserve and disseminate genetic traits, not only among enterococci but also to other Gram-positive bacteria (3, 7–10). Therefore, preventing the existence and permanence of these microorganisms in the hospital’s and ICU’s environment is an essential practice to reduce the number of HAIs (11).
In veterinary practice, Biological Isolation and Containment Units (BICUs) correspond to the hospital areas where animals are placed to mitigate the spread of infections to other animals or humans (zoonosis). These units are preferably located in a distinct area of the hospital, usually with a separate entrance, properly identified and with restricted access only to staff essential for the treatment of the hospitalized animals. These areas should always have their own standard operation procedures (SOPs), available to all personnel (12). If possible, they should also be under negative pressure (<2.5 Pa), with 6 to 12 air changes per hour, to contain all pathogenic organisms present in the air, exhausting them directly to the exterior; if not, a high-efficiency particulate air (HEPA) filter should be installed (13, 14). Other procedures, such as limitation of owner visits, adequate use of personal protection equipment (PPEs), and rigorous cleaning and disinfection protocols should also be applied in those settings (12, 15).
In this study, Enterococcus isolates were collected from the surfaces of a BICU belonging to a Veterinary Teaching Hospital in two distinct periods of time, after which they were identified and their antimicrobial susceptibility and virulence profiles determined, aiming to evaluate a high-risk transmission scenario. Taking into account enterococci’s environmental permanence and their importance in terms of HAIs, combined with their intrinsic resistance to many antimicrobials, these bacteria could become an important source of infection to animals in a BICU, and their presence should consequently be assessed, not only in these units but also in veterinary hospitals and clinics.
The BICU of the Veterinary Teaching Hospital (Faculty of Veterinary Medicine, University of Lisbon) is located in a building separate from the hospital, with its own entrance and restricted access. It receives companion animals suspected or confirmed of having an infectious disease, most commonly upper respiratory infections (URIs), FeLV, FIV, and panleukopenia in the case of cats (16), and parvovirus, leptospirosis, multi-drug resistant (MDR) infections, and distemper in the case of dogs (17).
The unit is divided into a total of four hospitalization rooms: one for cats, two for dogs, and one for intermedium cat patients, i.e., for those suspected but not confirmed of having an infectious disease due to the lack of vaccination or testing, or for cats infected with retrovirus but with no other concomitant diseases. There is also a room that works as a reception and consulting room for the animals that are admitted to the BICU, a work room for medical personnel and students, a preparatory room, and a storage room.
This unit has implemented several infection control measures. These include the presence of a ventilation system equipped with a HEPA filter that maintains a stable temperature and negative pressure, the availability of several SOPs that, amongst others, give indications on how to manipulate patients (including utilization of PPEs), on hand hygiene and also on cleaning and disinfecting protocols for different BICU areas, including daily cleaning and disinfection of the floors and surfaces, weekly cleaning and disinfection of each room and a yearly fumigation of the entire unit, independently of the number of animals housed in the area.
All hospitalization rooms are equipped with cages and examination tables made of stainless steel, a cabinet for material storage needed for patient examination (each room has its own materials), and a sink.
A total of 200 samples were obtained between February and July of 2022 from different high-critical surfaces of the BICU: cages (n = 80), cabinets (n = 41), examination tables (n = 39), effusion pumps (n = 18), door handles (n = 10), sinks (n = 8), incubator (n = 2), and of the plastic box used to storage examination material (stethoscope, thermometer, among others) (n = 2) (Figure 1).
Samples were collected using a swab embedded in a saline solution. Cages were sampled when fully disinfected and ready to receive new patients, but also when the disinfection process was still underway, since these surfaces could serve as a source of cross-contamination. A total of 52 samples were collected from fully disinfected cages, while 9 samples were taken on the first 24 h (first day), 12 on between 24 and 48 h (second day) and 7 between 48 and 72 h (third day) after disinfection.
Collected swabs were immediately transported to the Laboratory of Bacteriology of the same Faculty. After vortex homogenization, 100 μL of each sample were inoculated in Slanetz Bartley agar (AppliChem, Darmstadt, Germany) with the aid of sterile glass beads, and incubated at 36°C (±1°C) for 48 h. Subsequently, four colonies with macroscopic characteristics compatible with Enterococcus spp., i.e., red, brown or pink colonies, were collected and further characterized through Gram staining, catalase reaction, and the bile esculin test (18). Finally, the presumptive identification of all enterococci was confirmed through PCR.
An additional 23 presumptive Enterococcus isolates collected from surfaces of the BICU in 2019 by Verdial et al. (19), and previously reported in another study (20), were also added to this collection and also identified by PCR.
Genus identification was made using primers and adapted conditions as described by Ke et al. (21), in mixtures containing a total of 25 μL composed of: 12.5 μL of Supreme NZYTaq II 2x Green Master Mix (NZYTech, Lisbon, Portugal), 1 μL of DNA and 1 μM of each Ent1 and Ent2 primer (Table 1). Positive (E. faecalis ATCC® 29212) and negative (sterile PCR water) controls were included in all reactions. Thermocycler conditions were as follows: an initial step of 94°C for 3 min, 35 cycles with 1 min at 94°C, 1 min at 48°C, and 1 min at 72°C, followed by a final step at 72°C for 5 min. PCR products were evaluated by agarose gel electrophoresis (1.3%, w/v) in 1x TBE buffer (NZYTech, Lisbon, Portugal) supplemented with GreenSafe Premium (NZYTech, Lisbon, Portugal) at 90 V for 1 h, and photographed with the ChemiDoc™ Gel Imaging System (Bio-Rad, California, United States).
Species identification was made through a multiplex PCR for E. faecium, using primeirs FM1 and FM2, and E. faecalis, using primers FL1 and FL2, and for E. hirae, using primers HI1 and HI2, and E. durans, using primers DU1 and DU2, according to protocols adapted from Jackson et al. (22) (Table 1). Positive (E. faecalis ATCC® 29212, E. faecium CCUG® 36804, E. hirae ATCC® 10541, E. durans DSM® 20633) and negative controls (sterile PCR water) were included in all reactions, and independent replicas (10%) were also performed to guarantee the validity and reproducibility of the results.
For E. faecium and E. faecalis identification, PCR mixtures containing 12.5 μL of Supreme NZYTaq II 2x Green Master Mix (NZYTech, Lisbon, Portugal), 1 μL of DNA and 1.25 μM of each primer were prepared. Thermocycler conditions were as follows: an initial step of 95°C for 5 min, 35 cycles with 1 min at 95°C, 1 min at 54°C, and 1 min at 72°C, followed by a final step at 72°C for 10 min.
For E. hirae and E. durans identification, PCR mixtures containing 12.5 μL of Supreme NZYTaq II 2x Green Master Mix (NZYTech, Lisbon, Portugal), 1 μL of DNA and 0.75 μM of each primer were prepared. Thermocycler conditions were as follows: an initial step of 95°C for 5 min, 30 cycles with 30 s at 95°C, 1 min at 55°C and 1 min at 72°C, followed by a final step at 72°C for 7 min.
PCR products were evaluated as described before.
The protocol used for genomic typing by fingerprinting was adapted from the one described by Semedo-Lemsaddek et al. (23), using primer (GTG)5 in mixtures containing: 1x reaction buffer, 3 μM MgCl2, 0.2 μM of each deoxynucleotide triphosphate (dNTP), 2 μM of Primer, 0.06 U of Taq (Invitrogen, Massachusetts, United States) and 100 ng of DNA. A negative control (sterile PCR water) was included in all reactions, and independent replicas (5%) were also performed to validate and evaluate the reproducibility of the results. Thermocycler conditions were as follows: an initial step of 94°C for 4 min, 40 cycles with 1 min at 94°C, 2 min at 40°C, and 2 min at 72°C, followed by a final step at 72°C for 10 min. PCR products were evaluated by agarose gel electrophoresis (1.5%, w/v) in 1x TBE buffer (NZYTech, Lisbon, Portugal) supplemented with GreenSafe Premium (NZYTech, Lisbon, Portugal) at 90 V for 70 min, and photographed with ChemiDoc™ Gel Imaging System (Bio-Rad Laboratories, California, United States).
The profiles were compared by BioNumerics® 6.6 (Applied Maths, Kortrijk, Belgium), with a hierarchical numerical process based on the Pearson correlation coefficient (optimization 0.5) and the unweighted pair group method with arithmetic average (UPGMA) as the agglomerative clustering. The reproducibility value was determined as the average value for duplicates, and a cut off of 70% was established.
Isolates that had a similarity above 70% but were from different years (2019 or 2022) were maintained for further testing.
The characterization of the susceptibility profile of all isolates under study was performed through the disk diffusion method, made accordingly to the Clinical and Laboratory Standards Institute (CLSI) guidelines (24, 25). Control strains (Staphylococcus aureus ATCC® 25923 and E. faecalis ATCC® 29212) were also tested, as recommended by CLSI, and independent replicates (10%) were performed to assure the reproducibility of the results obtained.
The antibiotics to be tested were chosen according to their frequent use as a treatment option against enterococcal infections in both human and veterinary medicine: ampicillin (10 μg), amoxicillin and clavulanic acid (30 μg), ciprofloxacin (5 μg), levofloxacin (5 μg), tetracycline (30 μg), doxycycline (30 μg), erythromycin (15 μg), chloramphenicol (30 μg), linezolid (30 μg), vancomycin (30 μg), teicoplanin (30 μg), high-level gentamycin (120 μg) and high-level streptomycin (300 μg) (Oxoid Limited®, Hampshire, United Kingdom). High-level aminoglycoside testing in enterococci is performed to assess whether aminoglycosides can be used effectively in combination with penicillin or glycopeptides to treat infections caused by these bacteria. Additionally, if high-level resistance to both aminoglycosides is detected, it generally indicates that the bacteria are resistant to all aminoglycosides (24, 25).
Bacterial suspensions with turbidity equivalent to 0.5 in the McFarland scale (approximately 1.5 × 108 CFU/mL) were prepared for all isolates using a Densimat® (bioMérieux, Lisbon, Portugal). These suspensions were then inoculated using the lawn technique on Mueller–Hinton agar (Oxoid Limited®, Hampshire, United Kingdom) plates, followed by placement of the antibiotic disc on the agar surface and incubation at 36°C (±1°C) for 18 h, or for 24 h in the specific case of vancomycin. The diameter of the zones of inhibition formed around the disc was measured and results were interpreted according to the CLSI guidelines M100 (24), VET09 (25), and M31-A3 (26).
The Multiple Antibiotic Resistance (MAR) index for each isolate was calculated according to Singh et al. (27): the number of antimicrobials to which isolates were resistant divided by the number of antimicrobials tested.
Hemolysin, gelatinase, biofilm, DNAse, proteinase, and lecithinase production were evaluated phenotypically in different agar media, using the incubation conditions described by Fernandes et al. (28), using both positive and negative controls, as well as performing independent replicas (10%).
Hemolysin was evaluated on Columbia agar medium supplemented with 5% sheep blood (bioMérieux, Lisbon, Portugal). The formation of a transparent or greenish halo around the colonies was considered a positive reaction.
Gelatinase was evaluated in nutrient gelatin agar (Oxoid, Basingstoke, United Kingdom), using Pseudomonas aeruginosa ATCC® 27853 as a positive control and Escherichia coli ATCC® 25922 as a negative control. A liquefaction of the medium was classified as positive, after a 30-min refrigeration at approximately 4°C.
Biofilm was evaluated in Brain Heart Infusion agar (VWR, Leuven, Belgium) supplemented with Congo Red as an indicator (Sigma-Aldrich, St. Louis, United States) and sucrose (Merck KGaA, Darmstadt, Germany), using E. faecium ATCC® 29212 as a positive control and Escherichia coli ATCC® 25922 as a negative control. The formation of black colonies with a glossy surface was classified as positive.
DNAse was evaluated in DNAse agar (VWR, Leuven, Belgium) supplemented with toluidine blue (Merck KGaA, Darmstadt, Germany), using S. aureus ATCC® 25923 as a positive control and Escherichia coli ATCC® 25922 as a negative control. The formation of a pink halo around the colonies was considered a positive reaction.
Proteinase was evaluated in Skim Milk agar (VWR, Leuven, Belgium), using P. aeruginosa ATCC® 27853 as a positive control and S. aureus ATCC® 29213 as a negative control. The formation of a transparent halo around the colonies was considered a positive reaction.
Lecitinase was evaluated in Tryptic Soy Agar (VWR, Leuven, Belgium) supplemented with an egg yolk emulsion at 10% (VWR, Leuven, Belgium), using P. aeruginosa ATCC® 27853 as a positive control and Escherichia coli ATCC® 25922 as a negative control. The formation of a white precipitation halo around the colonies was considered a positive reaction.
Firstly, due to the lack of agreement regarding the ideal glucose supplementation value required to evaluate biofilm-forming ability by enterococci (29), a protocol was performed aiming to optimize glucose concentration, adapted from El-Zamkan and Mohamed (30) and Hashem et al. (29). Bacterial suspensions of approximately 1.5 × 108 CFU/mL were prepared for three randomly chosen Enterococcus isolates, in 2 mL of Tryptic Soy Broth (TSB) (VWR, Leuven, Belgium) supplemented with various glucose concentrations (0.25, 0.5, 1.0 and 1.5%). This bacterial suspension was then further diluted 1:100 in equally supplemented TSB, after which 200 μL were inoculated onto 3 different wells of a 96-well plate. The same was performed for negative controls, which corresponded to solutions of the four mentioned different glucose concentrations without bacteria, and then the plate was incubated at 37°C (±1°C) during 48 h. After incubation, the contents of the wells were discarded, and the wells were washed three times with 150 μL of phosphate-buffered saline. The cells were then heat-fixed at 60°C for 1 h. After, 200 μL of crystal violet (Merck, Darmstadt, Germany) at 2% were added to each well, followed by a 15 min incubation at room temperature. Next, the non-adhered dye was removed by inverting the plate and then washing it with water. Finally, 200 μL of ethanol at 95% were added to each well, followed by a 30 min incubation at room temperature to resolubilize the dye. Optic density (OD) of each well was measured at 570 nm using the FLUOstar OPTIMA microtiter-plate reader (BMG Labtech, Ortenberg, Germany). This assay was repeated two more times in independent days.
Isolates were evaluated on their ability to form biofilm according to the OD value obtained as follows: strains with OD < ODc (average OD of negative controls) were considered as non-biofilm producers; those with ODc < OD < 2ODc were considered as weak biofilm producers; strains with 2ODc < OD < 4ODc were considered as moderate biofilm producers; and those with 4ODc < OD were considered as strong biofilm producers.
Biofilm production determination for all isolates was conducted using the same protocol, with a fixed glucose supplementation of 0.5% (final concentration of 0.75%).
The virulence index of each isolate was calculated as the sum of all positive virulence phenotypes exhibited by each isolate divided by the total number of virulence factors tested (27). Regarding biofilm formation capacity, only the results obtained by crystal violet staining were considered, since this is described as a more accurate method in comparison to Congo Red agar plate evaluation (29).
Fisher’s exact test and Pearson’s chi-squared were employed to investigate whether there were statistically significant differences in antibiotic resistance and the presence or absence of virulence factors between the isolates collected in the years 2019 and 2022. The p-values were calculated to assess the significance of the results, using a significance level of 0.05. RStudio (Integrated Development for R, Boston, United States of America), an integrated development environment for the R programming language, was utilized for performing the statistical analyses, using relevant packages (“dplyr,” “tidyr,” “reshape,” “tidyverse” and “ggplot2”) and functions as needed.
Considering all samples collected (n = 200), only 8% (n = 16/200) were positive for enterococcal presence. Infusion pumps were the surface that originated the highest relative number of positive samples (n = 4/18, 22.2%), followed by cages (n = 9/80, 11.3%), door handles (n = 1/10, 10.0%), and cabinets (n = 2/41, 4.9%); all other locations were negative for the presence of Enterococcus spp. (Figure 2). There were also more positive samples collected from dogs’ isolation rooms (n = 10/16, 62.5%) than cats’ isolation rooms (n = 6/16, 37.5%). No isolates were collected from cat cages during the disinfection process or from fully disinfected cages. However, it was possible to obtain 4 positive samples from fully disinfected dog cages, 1 from a cage on the first day of disinfection, 3 on the second day and 1 on the third day. Sampling procedure allowed to obtain a total of 50 presumptive enterococci.
A total of 73 isolates, 23 from 2019 and 50 from 2022, were submitted to PCR for isolates identification at genus and species levels. All isolates were confirmed as belonging to the Enterococcus genus (Figure 3), with 60 (82.2%) being identified as E. faecium, 9 (12.3%) as E. hirae, and 4 (5.5%) as E. faecalis.
Fingerprinting allowed for the selection of 34 representative isolates, 27 belonging to the species E. faecium, 4 to E. hirae and 3 to E. faecalis, which were further characterized. The gels and dendrogram used for selection can be found in Supplementary material 1. As seen in Figure 4, after this selection, it was possible to find isolates with a high similarity from samples collected in different years, more specifically E6 and EN21, E12 and EN31, E3 and EN32, and E17 and EN16.
Figure 4. Dendrograms of representative Enterococcus faecium (A), Enterococcus faecalis (B) and Enterococcus hirae (C) isolates, obtained using the (GTG), primer. Isolates from 2019 are represented by an E followed by a number and isolates from 2024 are represented by an EN followed by a number. The second row, following identification, represents place of collection for each isolate - C1: dogs’ hospitalization room 1; C2: dogs’ hospitalization room 2; G-INT: cats’ hospitalization room for intermediate patients; GINF: cats’ hospitalization room for infected patients.
Antimicrobial susceptibility testing was accomplished through the disk diffusion method, allowing to classify isolates into three distinct phenotypes, according to the diameter of the inhibition halo produced: resistant, intermediate, and susceptible (Table 2).
Table 2. Results from the antimicrobial susceptibility tests of all isolates under study (n = 34), with corresponding susceptibility and resistance rates for each antibiotic tested.
As observed, erythromycin was the antibiotic with a higher degree of resistance (n = 27/34, 79.4%), followed by ampicillin (n = 25/34, 73.5%), amoxicillin-clavulanic acid (n = 24/34, 70.6%), tetracycline (n = 23/34, 67.6%), ciprofloxacin (n = 20/34, 58.8%), levofloxacin (n = 17/34, 50.0%), streptomycin (n = 5/34, 14.7%), doxycycline (n = 4/34, 11.8%) and gentamicin (n = 4/34, 11.8%). All isolates were susceptible to chloramphenicol, linezolid and teicoplanin and no isolate was resistant to vancomycin.
Although resistance to high-level gentamicin and high-level streptomycin were similar, no isolates were resistant to both antibiotics simultaneously.
Moreover, 70.6% of the isolates were considered as MDR, i.e., resistant to one or more agents from three or more antimicrobial categories, excluding intrinsic resistances (31). Intermediate classifications were considered as susceptible for this characterization.
As seen in Table 3, the majority of the enterococci isolated were capable of producing biofilm (n = 33/34, 97.1%) in Congo Red agar, and of producing hemolysin (n = 30/34, 88.2%). A total of 7 isolates (20.6%) were able to produce proteinase, and only 1 isolate (2.9%), identified as E. faecalis, was capable of producing gelatinase. No isolates produced either DNAse or lecithinase.
In terms of the optimization of glucose concentrations to be used in the biofilm quantification assay through crystal violet, the highest average OD values obtained for the 0.5% supplementation and the 1% supplementation were very similar for two isolates, with differences of 0.013 and 0.090. The third isolate, however, presented a higher difference (0.249), yielding higher OD values at a 0.5% supplementation. Additionally, for the first two isolates mentioned, the standard deviation was much lower in the assays using a 0.5% supplementation, associated with more consistent results among replicates. Therefore, the 0.5% supplementation was chosen for the remaining assays.
Regarding biofilm formation, evaluated through the crystal violet microtiter protocol, a total of 6 isolates (17.7%) were classified as non-producers, 22 (64.7%) as weak producers, 5 (14.7%) as moderate producers, and 1 (2.9%) as a strong producer.
Results regarding both the percentage of glucose supplementation optimization and crystal violet biofilm assays can be found in Supplementary material 2.
The MAR and virulence indexes for the isolates evaluated in this study are presented in Table 4.
The mean MAR index for all isolates was 0.34 (±0.17), while for isolates from 2019 was 0.31 (±0.20), and for isolates from 2022 was 0.36 (±0.15). The mean virulence index for all isolates was 0.32 (±0.10), while for isolates from 2019 was 0.35 (±0.12), and for isolates from 2022 was 0.31 (±0.08).
As described by Singh et al. (27), MAR and virulence indexes can be collectively used to classify the pathogenicity potential of the different isolates into four different threat categories: (i) high threat, if MAR index ≥0.30 and virulence index ≥0.50; (ii) moderate threat, if MAR index <0.30 and virulence index ≥0.50; (iii) low threat, if MAR index ≥0.30 and virulence index <0.50 and (iv) no threat, if MAR index <0.30 and virulence index <0.50. Based on this classification, from the totality of isolates presented in this study, 3 isolates (8.8%) were classified as high threats, 2 (5.9%) as moderate threats, 21 (61.8%) as low threats, and 8 (23.5%) as no threat.
The statistical analysis, detailed in Supplementary material 3, did not reveal any significant associations or differences between the variables under study. The majority of the Pearson’s chi-squared tests and Fisher’s exact tests originated high p-values (p-values >0.5), suggesting that the susceptibility and virulence patterns of the isolates remained consistent over time.
For individual antibiotics, no significant alterations were observed in resistance frequencies between isolates from Year 1 and Year 2. Similarly, the combined analysis of all antibiotics showed no overall change in the susceptibility patterns of the isolates. The analysis of virulence factors, both individually and combined, also indicated no significant differences between the 2 years. Additionally, the frequency of multidrug resistance (MDR) remained unchanged.
These results suggest a stable pattern in antibiotic susceptibility and virulence profiles of the isolates over the study period, with no strong relationship or dependency on the year of sampling. However, a larger sample size or more sensitive methods could potentially detect subtler changes or trends.
Enterococci are regarded as bacteria of great importance in terms of antimicrobial resistance, with vancomycin-resistant Enterococcus faecium being considered by the World Health Organization (WHO) as a high-priority pathogen for the development of new antibiotics (32). These bacteria are also recognized for their high environmental permanence ability, presenting a noteworthy resistance to numerous adverse conditions, and a significant capacity for genome plasticity (3–6). All these characteristics make them important pathogens in terms of HAIs, so it is important to evaluate enterococcal presence and characterization in areas of high-risk transmission, such as hospitals (6).
The proportion of positive enterococcal samples obtained in this study in 2022 (8.0%) was similar to the one obtained in 2012 by Hamilton et al. (33) (9.5%) in the United States (US); however, it was much lower than the one obtained in 2023 by Singaravelu et al., (34) (27.4%) in Ireland. All three studies report samples obtained from veterinary hospitals; however, our samples were the only ones obtained strictly from an isolation unit. This lower proportion of positive samples was expected due to surface disinfection being a part of the strict protocols implemented for infection control in the BICU. However, when taking into account that a great proportion of animals, mainly dogs, admitted to this unit present some kind of gastrointestinal disease, as stated by Machado et al. (17), and that they are usually under antibiotic pressure, it is safe to admit that both these factors could lead to the selection of resistant bacteria (35–39), especially Enterococcus strains, since they present intrinsic resistance to many of the antibiotics regularly used in these treatments (4–6, 40).
Gastrointestinal diseases are usually associated with diarrhea, which consequently means that the cages that contain these patients are frequently contaminated with a higher degree of organic matter, rendering them harder to clean and disinfect. This hypothesis is supported by the fact that the second surface with a higher proportion of positive samples were dog cages. The higher proportion of positive samples in fully disinfected cages could be associated with the fact that enterococci are ubiquitous in the environment and could be present in materials used to clean these cages or could be vehiculated by the personnel responsible for cleaning or transporting them.
The higher proportion of positive samples collected from infusion pumps in this study could be due to the fact that, when samples were collected, these pumps were allocated to animals and sometimes, by lapse, may circumvent the disinfection process and serve as a source of cross-contamination.
In terms of ubiquitous bacteria such as Enterococcus, a simple routine evaluation of the proportion of positive/negative samples is not enough to determine the degree of concern that these surfaces represent in terms of infection control, and that further procedures, such as susceptibility tests, should be considered.
In regards to species prevalence, only Hamilton et al. (33) presented comparable results, with E. faecium also being the species with a higher percentage of representation in the samples analyzed by those authors. However, they also indicated that the second most prevalent species was E. faecalis, followed by E. durans, with no other species present. On the other hand, our study revealed a higher prevalence of E. hirae, followed by E. faecalis. In terms of isolates collected from cats and dogs, E. faecium and E. faecalis are usually the most prevalent species found, with some studies describing a higher percentage of E. faecium while others revealing a higher percentage of E. faecalis (41–47). Although some of these studies do not report the presence of E. hirae isolates in samples collected from animals, other studies, including the present one, describe a higher prevalence of this species in comparison to either E. faecium or E. faecalis (44, 47). Kataoka et al. (45) indicated a higher proportion of E. hirae in animals with no previous antibiotic exposure (i.e., less than 4 months old), whereas Ghosh et al. (48) and Jackson et al. (44) denoted that this could be the predominant species in cats. Contrarily to these, the majority of the isolates under study were collected from dog’s isolation rooms that frequently contain animals going through antibiotic treatment. However, if we consider that, in Kataoka et al.’s (45) study, the presence of E. hirae is associated with age rather than antibiotic exposure, the higher proportion of this species could be linked to the fact that the average age of dogs at the UICB is around 8 months (17).
The fingerprinting dendrogram obtained prior to the selection of the representative isolates, available in Supplementary material 1, shows that many isolates with high similarity were collected from the same room of the BICU. This is particularly evident for E. hirae and E. faecalis isolates, which all but one E. hirae and one E. faecalis isolate were originated from samples taken in the same room. However, exceptions exist, such as isolates E1 and E3, which, despite having a 91.4% similarity, were collected from different hospitalization rooms of the BICU. Similar cases include EN15 and EN23 (96.9% similarity), EN32 and EN40 (95.7%), and E6 and E15 (91.9%). Notably, E6 was collected from a hospitalization room for dogs, while E15 was originated from a hospitalization room for cats. This pattern could suggest potential cross-contamination between rooms. Given the high positivity rate of infusion pumps, which are one of the few items shared between all rooms, they could serve as a possible source of cross-contamination despite thorough disinfection.
Enterococci are known for their intrinsic and extrinsic resistance to a number of antibiotics (3–6). This is the reason why it is important to evaluate the antibiotic susceptibility of strains present in different critical environments such as isolation units, where they could possibly cause nosocomial infections in immune-suppressed animals (5).
The analyzed isolates presented a resistance rate of 73.5% to ampicillin, mainly represented by resistant E. faecium isolates (88.9%) (Table 2). This higher resistance ratio was also observed in a study performed in 2011 by Ghosh et al. (48) in the US, in which this species presented a 96.5% resistance to this antibiotic. These results do not come as a surprise, as it is well-known that E. faecium are usually more prone to β-lactam resistance (43, 47, 48), since they are known to frequently present the pbp5 gene, which is the one most frequently associated with penicillin and cephalosporin resistance in Enterococcus (11, 49). On the other hand, when comparing the resistance rate observed in this study with others that evaluated isolates from veterinary clinics or hospitals’ surfaces or animals (cats and dogs), the value observed in this study is the second highest, surpassed only by Ghosh et al. (48). Other percentages found for total resistance are much lower (0.05–39.0%) (33, 41–43, 45, 47, 50, 51), with Iseppi et al. (46) being the only describing a resistance rate higher than 50% (56.5%). The resistance to amoxicillin-clavulanic acid (70.6%) found closely mirrored the one observed for ampicillin (73.5%), a finding consistent with the results reported by Awosile et al. (51). This similarity in resistance aligns with the understanding that β-lactamase production is rarely a mechanism associated with β-lactam resistance in enterococci (11). Both these resistance rates could be associated with the fact that amoxicillin-clavulanic acid is possibly the most frequent antibiotic used in the BICU.
Resistance to tetracycline (67.6%) was very similar to other studies performed in Portugal with isolates from animal origin (67.0–67.3%) (42, 50). Higher rates of tetracycline resistance in enterococci are not a novelty, as reported by various studies, which described values ranging between 43.0 and 97.4% (33, 34, 48, 50). Likewise, high rates of erythromycin resistance in enterococci have also been frequently reported elsewhere (41, 43, 46, 47).
Furthermore, resistance to both ciprofloxacin (58.8%) and levofloxacin (50.0%) were higher compared to findings in other studies, which reported resistance rates ranging from 7.8 to 57.7% for ciprofloxacin, and from 17.0 to 41.8% for levofloxacin (33, 43, 46, 47, 50), with the exception of those reported for ciprofloxacin by Rodrigues et al. (41) (73.1%) and Jackson et al. (44) (10–90%). Even though both compounds are not antibiotics used in the BICU, this higher resistance could be associated with the frequent use of other fluoroquinolones, such as enrofloxacin and marbofloxacin. Contrastingly, resistance to doxycycline, an antibiotic frequently used in veterinary medicine and in the BICU, and resistance to high-level gentamicin (11.8%) and high-level streptomycin (14.7%), were among the lowest reported (42, 44–48). Additionally, no isolate presented high-level resistance to both aminoglycosides.
No isolate presented vancomycin, linezolid or teicoplanin resistance, which has already been described in similar studies on veterinary settings (33, 41–43, 45, 46, 48). On the other hand, the fact that no isolates were resistant to chloramphenicol was not expected, as the majority of studies reported isolates resistant to this antibiotic (42, 44–46, 50–52); however, this lack of resistance could be explained by the fact that chloramphenicol is not routinely used in this isolation unit. The absence of isolates resistant to these compounds can be considered as positive, since vancomycin-resistant enterococci have been a rising threat in today’s medicine (32), and chloramphenicol has been effectively used in human medicine, in association with other antibiotics, against infections promoted by these bacteria (53, 54).
Virulence factors represent an advantageous feature of bacteria in terms of environmental survival. For example, they can be associated with adherence to a variety of surfaces and biofilm formation (11), which could impair the disinfection process (55, 56). This becomes especially problematic considering that the vast majority of the isolates from this study were found to be positive for biofilm production, according to both the Congo Red agar (97.1%) and Crystal Violet (82.3%) methodologies. The occurrence of this virulence factor in high rates in Enterococcus isolates has been reported in other studies (29, 30, 57, 58), and may explain the detection of isolates with similar fingerprinting profiles in samples collected 3 years apart (Figure 4). Notably, seven of those eight isolates (excluding E12) demonstrated biofilm-forming ability in crystal violet assays. Furthermore, it is concerning that this group of similar isolates from different years also includes all three isolates characterized as high threats (E3, E6 and EN21), as they could potentially serve as sources for the dissemination of resistance and virulence determinants.
Similarly, hemolysin production ability was also frequently detected in the isolates under study (88.2%). Contrarily to biofilm formation, the prevalence of this virulence factor reported in different studies is more variable. Analogously to our study, Iseppi et al. (46) reported hemolysin production rates of 63.5% while Poeta et al. (59) reported approximately 22% of positivity for hemolysin production. Cunha et al. (58) reported between 56 and 75%, however in this study α-haemolysis was considered as a negative result, contrarily to the present report and the other mentioned studies (46, 59). This variation could be associated with isolate’s origin, since this study was the only one that analyzed isolates from samples majorly collected from surfaces associated with non-healthy animals, notwithstanding possible human-origin contaminations.
On the other hand, the presence of gelatinase-positive isolates was lower in the present study (2.9%) in comparison to others (8.7–46.9%) (46, 58–60). The only gelatinase-positive isolate found was identified as E. faecalis, which seems to be the most frequent species associated with this virulence factor (46, 59, 60). Taking this into account, the lower percentage of gelatinase-positive isolates observed could be associated with the low proportion of representativity of this species in the totality of the isolates’ collection under study.
Considering the work developed by Verdial et al. (19), a new bacteriological control plan with optimized disinfection protocols was implemented between both sampling periods mentioned in this study. As such, this study also aimed to evaluate the statistical significance between the variations of the resistance profiles of the isolates collected in 2019 and 2022. This analysis aimed to determine any possible correlations between variation in disinfection protocols and antibiotic resistance, however, it was not possible to detect any significant associations or differences between the variables, indicating that there may not be a strong relationship or dependency between isolates’ resistance profiles and the year of the sampling, and, therefore, with the disinfection protocol under practice. However, it is important to note that further research with larger sample sizes or alternative analytical approaches could be valuable to confirm these findings.
In the 2022 samples, the surface associated with isolates with a higher MAR index (Table 4) were cages sampled on the third day of the disinfection procedure (0.46), followed by cabinets (0.43), door handles (0.38), infusion pumps (0.37), disinfected cages (0.35), cages sampled on the first day of the disinfection procedure (0.27), and finally cages sampled on the second day of the disinfection (0.21). VIR indexes were similar for isolates from every surface (approximately 0.33), except for disinfected cages and infusion pumps, which were slightly lower (0.25). The only isolate presenting a high pathogenetic potential was obtained from a cage sampled on the first day of disinfection. Higher index rates for cabinets and door handles are of concern, since these could be perceived as higher risk for cross contamination, and consequently of higher risk for resistant determinants dissemination. The higher index for isolates from disinfected cages compared to those obtained from cages sampled on the first and second days of disinfection is also worrisome, since these cages are considered ready for receiving new patients.
One of the limitations of this article is associated with the high variability inherent in the fingerprinting technique. While this method can be used to effectively reduce the number of isolates in analysis, it can only be indicative of other information such as potential cross-contaminations between rooms, which remain speculative without further validation. Since confirming the occurrence of cross-contaminations was not the primary focus of this study, no additional verification methods were employed. Moreover, the virulence profile of each isolate was determined using phenotypic methods, which could be further validated and enhanced through molecular techniques. However, since virulence in enterococci is predominantly linked to E. faecium (11), the least represented species in this study with only three isolates, and given the constraints associated with the development of molecular techniques, no additional testing was conducted.
To the best of our knowledge, this is the first study that describes antibiotic resistance and virulence profiles of Enterococcus collected from a veterinary BICU. The classification of the majority of isolates as MDR, the high percentage of biofilm and hemolysin producers, and the isolation of three high-threat pathogens from this isolation unit, could be associated with the high number of animals with gastrointestinal disease and under antibiotic pressure, leading to a shift in the intestinal bacterial environment and selection of resistant microbial strains. This means that a regular monitorization of this kind of microorganisms, including not only their isolation but also their characterization in terms of resistance and virulence profiles, in these units and in veterinary hospitals and clinics, seems to be imperative in order to prevent the spread of these pathogens and the possible development of hospital associated infections.
The original contributions presented in the study are included in the article/Supplementary material, further inquiries can be directed to the corresponding author/s.
CG: Writing – original draft, Conceptualization, Data curation, Formal analysis, Investigation, Methodology, Validation. CA: Writing – review & editing, Formal analysis, Investigation, Methodology. AP: Writing – review & editing, Formal analysis, Investigation, Methodology. MA: Writing – review & editing, Investigation, Methodology. SC: Writing – review & editing, Data curation, Formal analysis, Methodology, Software. CV: Writing – review & editing, Methodology. EC: Writing – review & editing, Methodology. RA: Writing – review & editing, Methodology. LT: Writing – review & editing, Resources, Validation. LC: Writing – review & editing, Data curation, Formal analysis, Methodology, Resources, Software, Validation. SG: Writing – review & editing, Conceptualization, Methodology, Project administration, Resources, Supervision, Validation. MO: Writing – review & editing, Conceptualization, Funding acquisition, Methodology, Project administration, Resources, Supervision, Validation.
The author(s) declare that financial support was received for the research, authorship, and/or publication of this article. This research was funded by the Portuguese Foundation for Science and Technology (FCT), under projects UIDB/00276/2020 (CIISA) and LA/P/0059/2020 (AL4AnimalS). CG PhD scholarship was funded by the FCT (2021.06552.BD).
The authors declare that the research was conducted in the absence of any commercial or financial relationships that could be construed as a potential conflict of interest.
The author(s) declared that they were an editorial board member of Frontiers, at the time of submission. This had no impact on the peer review process and the final decision.
All claims expressed in this article are solely those of the authors and do not necessarily represent those of their affiliated organizations, or those of the publisher, the editors and the reviewers. Any product that may be evaluated in this article, or claim that may be made by its manufacturer, is not guaranteed or endorsed by the publisher.
The Supplementary material for this article can be found online at: https://www.frontiersin.org/articles/10.3389/fvets.2024.1458069/full#supplementary-material
SUPPLEMENTARY MATERIAL 1 | Fingerprinting gels and corresponding dendrogram.
SUPPLEMENTARY MATERIAL 2 | Glucose optimization protocol results and crystal violet assay results.
SUPPLEMENTARY MATERIAL 3 | Statistical analysis report.
1. ECDC. Healthcare-associated infections acquired in intensive care units - annual epidemiological report for 2020. (2024). Available at: https://www.ecdc.europa.eu/en/publications-data/healthcare-associated-infections-acquired-intensive-care-units-annual (Accessed May 20, 2024).
2. ECDC. Surveillance Report: Healthcare-associated infections: surgical site infections - Annual Epidemiological Report for 2018–2020. (2023). Available at: https://www.ecdc.europa.eu/en/publications-data/healthcare-associated-infections-surgical-site-annual-2018-2020 (Accessed May 20, 2024).
3. García-Solache, M, and Rice, LB. The Enterococcus: a model of adaptability to its environment. Clin Microbiol Rev. (2019) 32:e00058–18. doi: 10.1128/CMR.00058-18
4. Hollenbeck, BL, and Rice, LB. Intrinsic and acquired resistance mechanisms in Enterococcus. Virulence. (2012) 3:421–569. doi: 10.4161/viru.21282
5. Kristich, CJ, Rice, LB, and Arias, CA. Enterococcal infection - treatment and antibiotic resistance. In enterococci: from commensals to leading causes of drug resistant infection In: MS Gilmore, DB Clewell, I Yasuyoshi, and N Shankar, editors. Boston: Eye Ear Infirmary. (2014). 123–84.
6. Torres, C, Alonso, CA, Ruiz-Ripa, L, León-Sampedro, R, del Campo, R, and Coque, TM. Antimicrobial resistance in Enterococcus spp. of animal origin. Microbiol Spectr. (2018) 6:185–227. doi: 10.1128/9781555819804.ch9
7. Weigel, LM, Clewell, DB, Gill, SR, Clark, NC, McDougal, LK, Flannagan, SE, et al. Genetic analysis of a high-level vancomycin-resistant isolate of Staphylococcus aureus. Science. (2003) 302:1569–71. doi: 10.1126/science.1090956
8. de Niederhäusern, S, Sabia, C, Messi, P, Guerrieri, E, Manicardi, G, and Bondi, M. Glycopeptide-resistance transferability from vancomycin-resistant enterococci of human and animal source to Listeria spp. Lett Appl Microbiol. (2004) 39:483–9. doi: 10.1111/j.1472-765X.2004.01598.x
9. Zhu, W, Clark, NC, McDougal, LK, Hageman, J, McDonald, LC, and Patel, JB. Vancomycin-resistant Staphylococcus aureus isolates associated with Inc18-like vanA plasmids in Michigan. Antimicrob Agents Chemother. (2008) 52:452–7. doi: 10.1128/AAC.00908-07
10. Limbago, BM, Kallen, AJ, Zhu, W, Eggers, P, McDougal, LK, and Albrecht, VS. Report of the 13th vancomycin-resistant Staphylococcus aureus isolate from the United States. J Clin Microbiol. (2014) 52:998–1002. doi: 10.1128/JCM.02187-13
11. Geraldes, C, Tavares, L, Gil, S, and Oliveira, M. Enterococcus virulence and resistant traits associated with its permanence in the hospital environment. Antibiotics. (2022) 11:857. doi: 10.3390/antibiotics11070857
12. Australasian Infectious Diseases Advisory Panel. Practical infection control guidelines. (2015). Available at: https://www.ava.com.au/siteassets/resources/fighting-antimicrobial-resistance/aidap-infection-control-guidelines.pdf (Accessed May 29, 2024).
13. Centers for Disease Control and Prevention, Healthcare Infection Control Practices Advisory Committee. Guidelines for Environmental Infection Control in Health-Care Facilities - Recommendations of CDC and the Healthcare Infection Control Practices Advisory Committee (HICPAC). (2019). Available at: https://www.cdc.gov/infectioncontrol/pdf/guidelines/environmental-guidelines-P.pdf (Accessed May 29, 2024).
14. Portner, JA, and Johnson, JA. Guidelines for reducing veterinary hospital pathogens. Compend Contin Educ Vet. (2010) 32:E1–E12.
15. Guptill, L. Patient management. Vet Clin N Am Small Anim Pract. (2015) 45:277–98. doi: 10.1016/j.cvsm.2014.11.010
16. Machado, ICT. Frequência de doenças infeciosas em carnívoros domésticos hospitalizados na unidade de isolamento do hospital escolar da Faculdade de Medicina Veterinária da Universidade de Lisboa de outubro de 2013 a janeiro de 2016 outubro de 2013 a janeiro de 2016 [master's thesis]. Lisbon, PT: FMV-ULisboa (2016).
17. Machado, IC, Nunes, T, Maximino, M, Malato, J, Tavares, L, Almeida, V, et al. Epidemiologic factors supporting triage of infected dog patients admitted to a Veterinary Hospital Biological Isolation and Containment Unit. Vet Sci. (2023) 10:186. doi: 10.3390/vetsci10030186
18. Oliveira, M, Tavares, M, Gomes, D, Touret, T, São Braz, B, Tavares, L, et al. Virulence traits and antibiotic resistance among enterococci isolated from dogs with periodontal disease. Comp Immunol Microbiol Infect Dis. (2016) 46:27–31. doi: 10.1016/j.cimid.2016.04.002
19. Verdial, C, Carneiro, C, Machado, I, Tavares, L, Almeida, V, Oliveira, M, et al. Controlling bacteriological contamination of environmental surfaces at the Biological Isolation and Containment Unit of a Veterinary Teaching Hospital. Ir Vet J. (2021) 74:18. doi: 10.1186/s13620-021-00197-z
20. Geraldes, C, Verdial, C, Cunha, E, Almeida, V, Tavares, L, Oliveira, M, et al. Evaluation of a Biocide Used in the Biological Isolation and Containment Unit of a Veterinary Teaching Hospital. Antibiotics. (2023) 10:639. doi: 10.3390/antibiotics10060639
21. Ke, D, Picard, FJ, Martineau, F, Ménard, C, Roy, PH, Ouellette, M, et al. Development of a PCR assay for rapid detection of enterococci. J Clin Microbiol. (1999) 37:3497–503. doi: 10.1128/JCM.37.11.3497-3503.1999
22. Jackson, CR, Fedorka-Cray, PJ, and Barrett, JB. Use of a genus- and species-specific multiplex PCR for identification of enterococci. J Clin Microbiol. (2004) 42:3558–65. doi: 10.1128/JCM.42.8.3558-3565.2004
23. Semedo-Lemsaddek, T, Nóbrega, CS, Ribeiro, T, Pedroso, NM, Sales-Luís, T, Lemsaddek, A, et al. Virulence traits and antibiotic resistance among enterococci isolated from Eurasian otter (Lutra lutra). Vet Microbiol. (2013) 163:378–82. doi: 10.1016/j.vetmic.2012.12.032
24. Clinical and Laboratory Standards Institute. CLSI M100: Performance Standards for Antimicrobial Susceptibility Testing. (2024). 34th edition.
25. Clinical and Laboratory Standards Institute. CLSI VET09: Performance Standards for Antimicrobial Disk and Dilution Susceptibility Tests for Bacteria Isolated From Animals. (2024). 2nd edition.
26. Clinical and Laboratory Standards Institute. CLSI M31-A3: Performance Standards For Antimicrobial Disk And Dilution Susceptibility Tests For Bacteria Isolated From Animals; Approved Standard. (2008). 3rd edition.
27. Singh, SK, Ekka, R, Mishra, M, and Mohapatra, H. Association study of multiple antibiotic resistance and virulence: a strategy to assess the extent of risk posed by bacterial population in aquatic environment. Environ Monit Assess. (2017) 189:320. doi: 10.1007/s10661-017-6005-4
28. Fernandes, M, Grilo, ML, Carneiro, C, Cunha, E, Tavares, L, Patino-Martinez, J, et al. Antibiotic resistance and virulence profiles of gram-negative bacteria isolated from Loggerhead Sea turtles (Caretta caretta) of the island of Maio, Cape Verde. Antibiotics. (2021) 10:771. doi: 10.3390/antibiotics10070771
29. Hashem, YA, Amin, HM, Essam, TM, Yassin, AS, and Aziz, RK. Biofilm formation in enterococci: genotype-phenotype correlations and inhibition by vancomycin. Sci Rep. (2017) 7:5733. doi: 10.1038/s41598-017-05901-0
30. El-Zamkan, MA, and Mohamed, HMA. Antimicrobial resistance, virulence genes and biofilm formation in Enterococcus species isolated from milk of sheep and goat with subclinical mastitis. PLoS One. (2021) 16:e0259584. doi: 10.1371/journal.pone.0259584
31. Magiorakos, AP, Srinivasan, A, Carey, RB, Carmeli, Y, Falagas, ME, Giske, CG, et al. Multidrug-resistant, extensively drug-resistant and pandrug-resistant bacteria: an international expert proposal for interim standard definitions for acquired resistance. Clin Microbiol Infect. (2012) 18:268–81. doi: 10.1111/j.1469-0691.2011.03570.x
32. World Health Organization. Bacterial pathogens of public health importance to guide research, development and strategies to prevent and control antimicrobial resistance. (2024). Available at: https://iris.who.int/bitstream/handle/10665/376776/9789240093461-eng.pdf?sequence=1 (Accessed May 17, 2024).
33. Hamilton, E, Kaneene, JB, May, KJ, Kruger, JM, Schall, W, Beal, MW, et al. Prevalence and antimicrobial resistance of Enterococcus spp and Staphylococcus spp isolated from surfaces in a veterinary teaching hospital. J Am Vet Med Assoc. (2012) 240:1463–73. doi: 10.2460/javma.240.12.1463
34. Singaravelu, A, Leggett, B, and Leonard, FC. Improving infection control in a veterinary hospital: a detailed study on patterns of faecal contamination to inform changes in practice. Ir Vet J. (2023) 76:4. doi: 10.1186/s13620-023-00229-w
35. Donskey, CJ. The role of the intestinal tract as a reservoir and source for transmission of nosocomial pathogens. Clin Infect Dis. (2004) 39:219–26. doi: 10.1086/422002
36. Donskey, CJ, Helfand, MS, Pultz, NJ, and Rice, LB. Effect of parenteral fluoroquinolone administration on persistence of vancomycin-resistant Enterococcus faecium in the mouse gastrointestinal tract. Antimicrob Agents Chemother. (2004) 48:326–8. doi: 10.1128/AAC.48.1.326-328.2004
37. Brandl, K, Plitas, G, Mihu, CN, Ubeda, C, Jia, T, Fleisher, M, et al. Vancomycin-resistant enterococci exploit antibiotic-induced innate immune deficits. Nature. (2008) 455:804–7. doi: 10.1038/nature07250
38. Jakobsson, HE, Jernberg, C, Andersson, AF, Sjölund-Karlsson, M, Jansson, JK, and Engstrand, L. Short-term antibiotic treatment has differing long-term impacts on the human throat and gut microbiome. PLoS One. (2010) 5:e9836. doi: 10.1371/journal.pone.0009836
39. Ubeda, C, Taur, Y, Jenq, RR, Equinda, MJ, Son, T, Samstein, M, et al. Vancomycin-resistant Enterococcus domination of intestinal microbiota is enabled by antibiotic treatment in mice and precedes bloodstream invasion in humans. J Clin Invest. (2010) 120:4332–41. doi: 10.1172/JCI43918
40. Miller, WR, Munita, JM, and Arias, CA. Mechanisms of antibiotic resistance in enterococci. Expert Rev Anti-Infect Ther. (2014) 12:1221–36. doi: 10.1586/14787210.2014.956092
41. Rodrigues, J, Poeta, P, Martins, A, and Costa, D. The importance of pets as reservoirs of resistant Enterococcus strains, with special reference to vancomycin. J Vet Med B Infect Dis Vet Public Health. (2002) 49:278–80. doi: 10.1046/j.1439-0450.2002.00561.x
42. Delgado, M, Neto, I, Correia, JH, and Pomba, C. Antimicrobial resistance and evaluation of susceptibility testing among pathogenic enterococci isolated from dogs and cats. Int J Antimicrob Agents. (2007) 30:98–100. doi: 10.1016/j.ijantimicag.2007.03.007
43. Ossiprandi, MC, Bottarelli, E, Cattabiani, F, and Bianchi, E. Susceptibility to vancomycin and other antibiotics of 165 Enterococcus strains isolated from dogs in Italy. Comp Immunol Microbiol Infect Dis. (2008) 31:1–9. doi: 10.1016/j.cimid.2007.08.003
44. Jackson, CR, Fedorka-Cray, PJ, Davis, JA, Barrett, JB, and Frye, JG. Prevalence, species distribution and antimicrobial resistance of enterococci isolated from dogs and cats in the United States. J Appl Microbiol. (2009) 107:1269–78. doi: 10.1111/j.1365-2672.2009.04310.x
45. Kataoka, Y, Ito, C, Kawashima, A, Ishii, M, Yamashiro, S, and Harada, K. Identification and antimicrobial susceptibility of enterococci isolated from dogs and cats subjected to differing antibiotic pressures. J Vet Med Sci. (2013) 75:749–53. doi: 10.1292/jvms.12-0243
46. Iseppi, R, Messi, P, Anacarso, I, Bondi, M, Sabia, C, Condò, C, et al. Antimicrobial resistance and virulence traits in Enterococcus strains isolated from dogs and cats. New Microbiol. (2015) 38:369–78.
47. Yuan, TY, Liang, B, Jiang, BW, Sun, SW, Zhou, YF, Zhu, LW, et al. Virulence genes and antimicrobial resistance in Enterococcus strains isolated from dogs and cats in Northeast China. J Vet Med Sci. (2023) 85:371–8. doi: 10.1292/jvms.22-0410
48. Ghosh, A, Kukanich, K, Brown, CE, and Zurek, L. Resident cats in small animal veterinary hospitals carry multi-drug resistant enterococci and are likely involved in cross-contamination of the hospital environment. Front Microbiol. (2012) 3:62. doi: 10.3389/fmicb.2012.00062
49. Sifaoui, F, Arthur, M, Rice, L, and Gutmann, L. Role of penicillin-binding protein 5 in expression of ampicillin resistance and peptidoglycan structure in Enterococcus faecium. Antimicrob Agents Chemother. (2001) 45:2594–7. doi: 10.1128/AAC.45.9.2594-2597.2001
50. Leite-Martins, L, Mahú, MI, Costa, AL, Bessa, LJ, Vaz-Pires, P, Loureiro, L, et al. Prevalence of antimicrobial resistance in faecal enterococci from vet-visiting pets and assessment of risk factors. Vet Rec. (2015) 176:674. doi: 10.1136/vr.102888
51. Awosile, BB, McClure, JT, Saab, ME, and Heider, LC. Antimicrobial resistance in bacteria isolated from cats and dogs from the Atlantic provinces, Canada from 1994-2013. Can Vet J. (2018) 59:885–93.
52. Kerek, Á, Sterczer, Á, Somogyi, Z, Kovács, D, and Jerzsele, Á. Investigation of the environmental presence of multidrug-resistant bacteria at small animal hospitals in Hungary. Acta Vet Hung. (2021) 68:387–92. doi: 10.1556/004.2020.00055
53. Lagatolla, C, Milic, J, Imperi, F, Cervoni, M, Bressan, R, Luzzati, R, et al. Synergistic activity of fosfomycin and chloramphenicol against vancomycin-resistant Enterococcus faecium (VREfm) isolates from bloodstream infections. Diagn Microbiol Infect Dis. (2021) 99:115241. doi: 10.1016/j.diagmicrobio.2020.115241
54. Shah, S, McManus, D, and Topal, JE. Combination therapy of chloramphenicol and Daptomycin for the treatment of infective endocarditis secondary to multidrug resistant Enterococcus faecium. Hosp Pharm. (2022) 57:345–8. doi: 10.1177/00185787211032364
55. Smith, K, and Hunter, IS. Efficacy of common hospital biocides with biofilms of multi-drug resistant clinical isolates. J Med Microbiol. (2008) 57:966–73. doi: 10.1099/jmm.0.47668-0
56. Wong, HS, Townsend, KM, Fenwick, SG, Trengove, RD, and O’Handley, RM. Comparative susceptibility of planktonic and 3-day-old Salmonella Typhimurium biofilms to disinfectants. J Appl Microbiol. (2010) 108:2222–8. doi: 10.1111/j.1365-2672.2009.04630.x
57. Ngbede, EO, Raji, MA, Kwanashie, CN, and Kwaga, JKP. Antimicrobial resistance and virulence profile of enterococci isolated from poultry and cattle sources in Nigeria. Trop Anim Health Prod. (2017) 49:451–8. doi: 10.1007/s11250-016-1212-5
58. Cunha, E, Ferreira, AF, Valente, S, Matos, A, Carreira, LM, Videira, M, et al. In vivo effect of a Nisin-biogel on the antimicrobial and virulence signatures of canine Oral enterococci. Antibiotics. (2023) 12:468. doi: 10.3390/antibiotics12030468
59. Poeta, P, Costa, D, Klibi, N, Rodrigues, J, and Torres, C. Phenotypic and genotypic study of gelatinase and beta-haemolysis activities in faecal enterococci of poultry in Portugal. J Vet Med B Infect Dis Vet Public Health. (2006) 53:203–8. doi: 10.1111/j.1439-0450.2006.00941.x
Keywords: Enterococcus , hospital-acquired infections, antibiotic resistance, virulence, veterinary, Biological Isolation and Containment Unit
Citation: Geraldes C, Araújo C, Pinheiro AC, Afonso M, Carapeto S, Verdial C, Cunha E, Abreu R, Tavares L, Chambel L, Gil S and Oliveira M (2024) Pathogenicity potential of enterococci isolated from a Veterinary Biological Isolation and Containment Unit. Front. Vet. Sci. 11:1458069. doi: 10.3389/fvets.2024.1458069
Received: 01 July 2024; Accepted: 20 September 2024;
Published: 21 October 2024.
Edited by:
Fabrizio Bertelloni, University of Pisa, ItalyReviewed by:
Piera Anna Martino, University of Milan, ItalyCopyright © 2024 Geraldes, Araújo, Pinheiro, Afonso, Carapeto, Verdial, Cunha, Abreu, Tavares, Chambel, Gil and Oliveira. This is an open-access article distributed under the terms of the Creative Commons Attribution License (CC BY). The use, distribution or reproduction in other forums is permitted, provided the original author(s) and the copyright owner(s) are credited and that the original publication in this journal is cited, in accordance with accepted academic practice. No use, distribution or reproduction is permitted which does not comply with these terms.
*Correspondence: Catarina Geraldes, Y2dlcmFsZGVzQGZtdi51bGlzYm9hLnB0
†Present address: Sandra Carapeto, University of Copenhagen, Copenhagen, Denmark
Disclaimer: All claims expressed in this article are solely those of the authors and do not necessarily represent those of their affiliated organizations, or those of the publisher, the editors and the reviewers. Any product that may be evaluated in this article or claim that may be made by its manufacturer is not guaranteed or endorsed by the publisher.
Research integrity at Frontiers
Learn more about the work of our research integrity team to safeguard the quality of each article we publish.