- 1Department of Public Health Pharmacology and Toxicology, Faculty of Veterinary Medicine, University of Nairobi, Nairobi, Kenya
- 2Centre for Epidemiological Modelling and Analysis, University of Nairobi, Nairobi, Kenya
- 3Paul G. Allen School for Global Health, Washington State University, Pullman, WA, United States
- 4Animal and Human Health, International Livestock Research Institute, Nairobi, Kenya
- 5Feed the Future Innovation Lab for Animal Health, Washington State University, Pullman, WA, United States
- 6Institute of Tropical and Infectious Diseases, University of Nairobi, Nairobi, Kenya
- 7Department of Clinical Studies, Faculty of Veterinary Medicine, University of Nairobi, Nairobi, Kenya
- 8Institute of Immunology and Infection Research, School of Biological Sciences, University of Edinburgh, Edinburgh, United Kingdom
Introduction: Strengthening global health security relies on adequate protection against infectious diseases through vaccination and treatment. Toll-like receptor (TLR) agonists exhibit properties that can enhance immune responses, making them potential therapeutic agents or vaccine adjuvants.
Methods: We conducted an extensive systematic review to assess the efficacy of TLR agonists as therapeutic agents or vaccine adjuvants for infectious diseases and their safety profile in animals, excluding rodents and cold-blooded animals. We collected qualitative and available quantitative data on the efficacy and safety outcomes of TLR agonists and employed descriptive analysis to summarize the outcomes.
Results: Among 653 screened studies, 51 met the inclusion criteria. In this review, 82% (42/51) of the studies used TLR agonists as adjuvants, while 18% (9/51) applied TLR agonist as therapeutic agents. The predominant TLR agonists utilized in animals against infectious diseases was CpG ODN, acting as a TLR9 agonist in mammals, and TLR21 agonists in chickens. In 90% (46/51) of the studies, TLR agonists were found effective in stimulating specific and robust humoral and cellular immune responses, thereby enhancing the efficacy of vaccines or therapeutics against infectious diseases in animals. Safety outcomes were assessed in 8% (4/51) of the studies, with one reporting adverse effects.
Discussion: Although TLR agonists are efficacious in enhancing immune responses and the protective efficacy of vaccines or therapeutic agents against infectious diseases in animals, a thorough evaluation of their safety is imperative to in-form future clinical applications in animal studies.
Systematic review registration: https://www.crd.york.ac.uk/prospero/display_record.php?RecordID=323122.
1 Introduction
Vaccination is currently the most effective strategy for controlling infectious diseases amidst the rising global concerns over the increasing risk of antimicrobial resistance, especially in low- and middle-income countries with poor pharmaceutical regulatory frameworks (1, 2). On the other hand, there are emerging public health concerns arising from the emergence of new pathogen strains and the re-emergence of many infectious diseases (3). Designing effective vaccines for infectious diseases, especially for emerging pathogens remains challenging. To improve the immune potency of existing vaccines, there is a need to understand host-pathogen immune responses and develop novel vaccines based on this knowledge (4, 5).
Traditionally, vaccines have been developed as live attenuated, live whole organisms, killed, and inactivated toxoids from organisms (6). Live attenuated or live whole cells vaccines, despite concerns over incomplete attenuation and associated risks of disease after vaccination, as in the cases of yellow fever and measles, contain sufficient PAMPs thus inducing adequate immunostimulatory activity (6–8). For example, for Theileria parva, an intracellular pathogen, a live vaccine, the Muguga cocktail vaccine containing three Theileria parva stocks has fairly good protection, albeit accompanied by creating a carrier state in vaccinated cattle and potential field parasite diversity changes and potential for disease introduction in previously naïve populations (9, 10). On the other hand, subunit vaccines containing protein or glycoprotein pieces of a pathogen, despite offering improved safety and prospects for quick development of new vaccines are comparatively poor immunogens (11, 12). Improved immunogenicity is achieved by the addition of adjuvants (13, 14). However, the use of adjuvants maybe accompanied by adverse effects. For instance, water-in-oil immersion adjuvants such as Freund’s complete adjuvant (FCA) and Freund’s incomplete adjuvant (FIA), despite their high potency as immune stimulants, induce severe adverse effects, including abscess formation, granulomas, inflammation at the site of injection, severe pain, and fever (15). On the other hand, aluminum salts, despite their immunostimulatory boosting activities, weakly induce Th1 immunity, a critical response for intracellular parasites such as Theileria spp. and Leishmania spp., among other pathogens (16, 17). Thus, finding an immune-potent adjuvant with minimal or no side effects is essential.
Presently, there exists a heightened understanding of the dynamics in host-pathogen interactions, resulting in the identification of alternative adjuvant formulations and therapeutics against various microbes (6). The discovery of Pathogen Associated Molecular Patterns (PAMPs) and their role in immunomodulation has been a ripe area for research (18, 19). PAMPs are conserved highly expressed functional microbial components, recognized by receptors in humans and animals (20). In responding to infections, the receptors that bind to PAMPs, known as Pattern Recognition Receptors (PRRs), recognize these conserved microbial components and initiate immune cascades, producing proinflammatory and antimicrobial responses and chemotactic factors (21). Toll-like receptors (TLRs), a type PRRs, are located on both the cell surface and within the endosomes. They identify PAMPs, including carbohydrates, nucleic acids, lipids, and proteins (20). The TLRs are expressed on and in cells of the innate immune system of mammals, such as dendritic cells, macrophages, monocytes, and cells of the adaptive immune system such as B cells (22). TLRs found on the cell surfaces recognize surface-associated PAMPs, while those in the endosomes detect nucleic acids. There are 10 TLRs (TLR 1 to TLR 10) in mammals, with distinct functions in the innate immune system, apart from mice, which have 13 (23). In the avian species, chicken TLRs are the most studied, having slightly different TLRs from mammals and mice, including TLR1La, TLR1Lb, TLR2a, TLR2b, TLR3, TLR4, TLR5, TLR7, TLR21, and TLR15 (24). TLRs detect a variety of PAMPs: TLR1/TLR2 binds peptidoglycans from Gram-positive bacteria; TLR2/TLR6 binds to diacylated lipopeptides; TLR3 binds to dsRNA, TLR4 binds to lipopolysaccharide on Gram-negative bacteria outer membrane; TLR5 binds to flagellin of motile bacteria; TLR7/8 binds to ssRNA; TLR9 recognizes unmethylated juxtaposed cytosine and guanine nucleotides (CpG) of bacterial DNA (25, 26). The binding of TLR ligands on antigen-presenting cells (APCs) promotes innate inflammatory responses that induce adaptive immunity, rendering TLRs ideal targets for developing effective therapeutic agents and vaccine adjuvants (24).
With the growing evidence of the active involvement of TLRs in the immune response of animals to infection, TLR agonists gained significant interest in development of vaccines and therapeutics in animals (27). Activation of the immune system by some TLR agonists has been documented to lead to detrimental side effects linked to unintended expansion of the adaptive immune cells, resulting in susceptibility to an infection or reduced efficacy of vaccines (28). It is crucial, therefore, to comprehensively underscore the adverse effects of TLR agonists, a vital consideration in their selection as therapeutics or vaccine adjuvants (28, 29). Thus, we conducted a systematic review to assess the efficacy and safety of TLR agonists as therapeutic agents and vaccine adjuvants for infectious diseases in animals to answer the following questions: (i) are TLR agonists efficacious therapeutic agents or vaccine adjuvants for infectious diseases in animals? (ii) Are TLR agonists safe for animal use as therapeutic agents and vaccine adjuvants?
2 Methodology
This systematic review used the Preferred Reporting Items for Systematic Reviews and Meta-Analysis (PRISMA) guidelines. The review protocol is registered at the International Prospective Register of Systematic Reviews (PROSPERO); protocol registration number CRD42023323122 (30).
2.1 Database sources and search strategy
A systematic search was conducted on January 25, 2023, and followed by an additional search on April 29, 2024 to ensure the search was current and up-to-date. The aim was to identify all the potentially relevant peer-reviewed articles from major electronic databases including PubMed, Embase, and Google Scholar. The systematic search was based on PICO (Population, Intervention, Control, and Outcome) framework and medical subject headings (MeSH) to identify keywords and index terms in which the Boolean operators (“AND,” “OR,” and “NOT”) were utilized to connect the keywords. The keywords used for the general search include: “(tlr or toll-like receptor) and (ligand or agonist) and (vaccine or therapeutic or prophylactic) and (efficacy or safety) and (infection or infectious agent or infectious disease) and (animal or livestock or veterinary) NOT (human or mice or mouse).” The terms for the measures of outcome, efficacy and safety were not included in the search to prevent limitation in the database searches (31). A general search was conducted directly from PubMed and Embase databases, and using Publish or Perish software for the Google Scholar database. Reference lists were retrieved and saved in Microsoft Excel spreadsheets in Comma Separated Value format (CSV) (Table 1).
2.2 Eligibility criteria
The published studies were screened by titles, abstracts, and full-text reviews to determine their eligibility. The studies included in this review must have been peer-reviewed and published, animal studies investigating TLR agonists as a therapeutic agent or vaccine adjuvant, investigating infectious agents or diseases, and conducted at any year, and in any part of the world. Studies were excluded if they were not peer-reviewed and published, involved either human participants or rodents, ex vivo and in vitro studies or involved in vivo studies, studies involving investigation of non-infectious agents or diseases, and studies that lacked full-text availability. Rodent studies were excluded from this review due to significant anatomical, pharmacological, and pathophysiological differences from larger animals, which would limit the translational applicability of their outcomes (32). Additionally, ex vivo, in vitro, and in vivo studies were excluded because of the complexities of correlating their outcomes to those observed in animals (33).
2.3 Selection of studies
Initially, duplicates were removed, and the screening questions were developed according to the inclusion and exclusion criteria (as described above). The screening was conducted HO and VR on the Rayyan QCRI platform: Initially an assessment of titles and abstracts and selection of eligible articles using the inclusion and exclusion criteria questions was conducted by HO and VR and subsequently, a full-text review of the selected articles to scrutinize and assess the studies’ eligibility according to the inclusion and exclusion criteria. Any conflicts on the eligibility of any article were resolved by an independent reviewer (AL).
2.4 Data extraction, analysis, and presentation
A data collection tool was prepared on Microsoft Excel Version 16.77 (23091003) and relevant data was extracted from the selected articles and cross-checked by two reviewers (HO and VR). Any disagreement by the reviewers on the relevance of the collected data was resolved by involving a third party (AL) to reach a consensus. The data variables extracted are shown in Table 2. Data on study characteristics, efficacy, and safety of Toll-like receptor (TLR) agonists were analyzed qualitatively using R statistical software version 4.1.2 (2021-11-01) and Microsoft Excel version 16.77 (23091003) and summarized using tables and figures.
2.5 Risk of bias assessment
A Risk of Bias (RoB) assessment for all the studies included in the review was conducted by HO and VR. The Systematic Review Centre for Laboratory Animal Experimentation (SYRCLE) risk of bias (RoB) tool was used to assess the risk of bias across 10 domains (34): sequence generation, baseline characteristics, allocation concealment, random housing, performance blinding, random outcome assessment, detection blinding, incomplete outcome data, selective outcome reporting, and other bias sources. The overall bias was assessed using screening questions and judgment, described in detail elsewhere (34). Reviewer conflicts on identifying the source of risk were resolved by an independent reviewer (AL).
3 Results
3.1 Study selection
A total of 711 potentially relevant studies were identified in our initial database search. Once duplicates were removed, titles and abstracts from 536 articles were screened, out of which 472 studies that did not meet the inclusion criteria were excluded based on their titles and abstracts. The full-text review was conducted on 64 studies; ultimately, 51 studies met the inclusion criteria and thus were included in this review (Figure 1).
3.2 Study characteristics
This review included studies published without limitation on the publication year, spanning from 2007 to April 2024. The years 2016, 2019, and 2021 marked the highest number of publications, of six publications, in the respective years (Figure 2). The average age of the publications from the year of publication of the studies to the time we conducted this review was approximately 6 years.
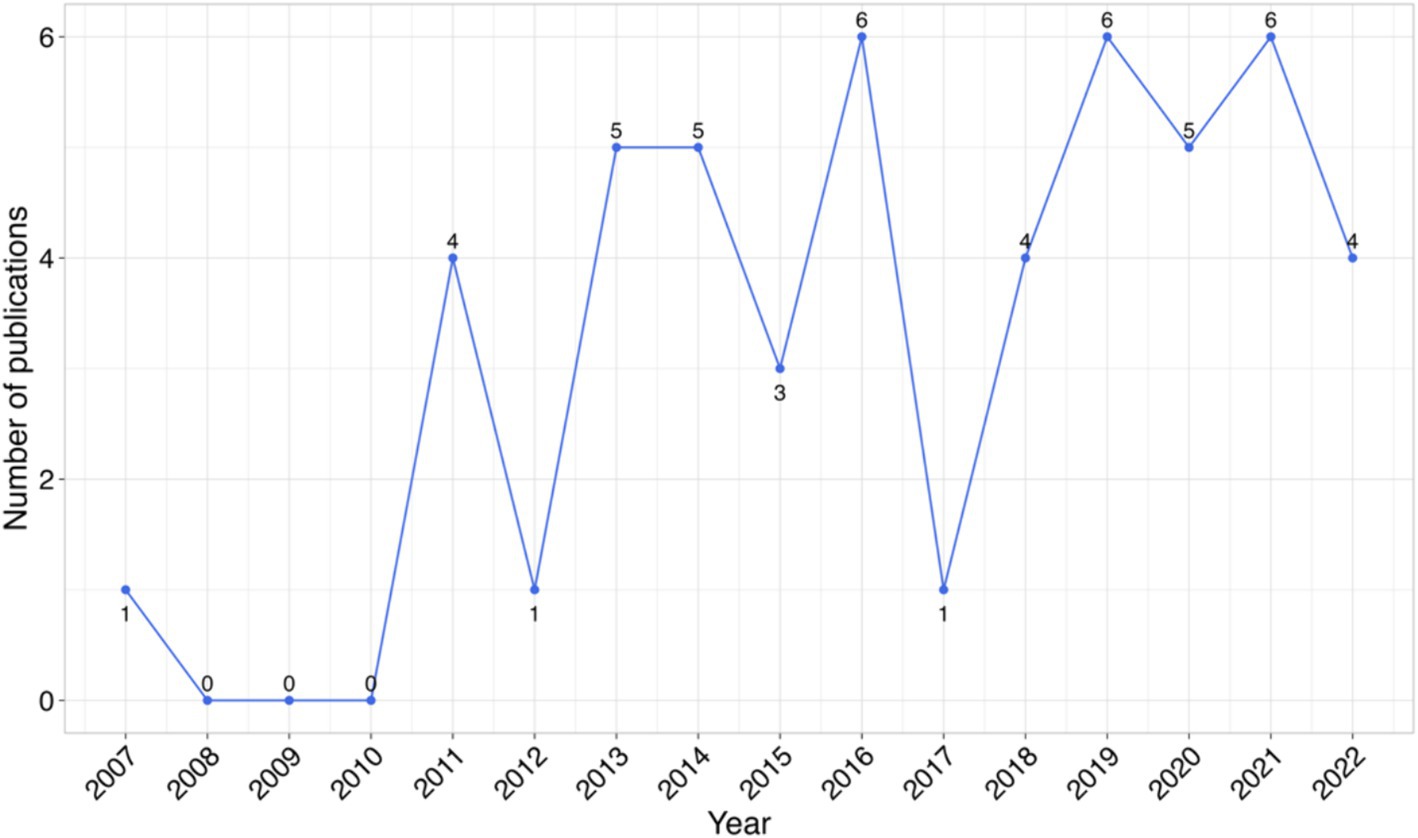
Figure 2. Studies published annually on use of TLR agonists as therapeutic agents and vaccine adjuvants for infectious diseases in animals.
Considering that there was no geographical limitation in the selection of the studies, most of the TLR agonist animal studies for infectious diseases were conducted in the United States (37%) and Canada (26%). Nonetheless, we did not identify any studies conducted in countries across South America, with only one study conducted in Africa, specifically in Tanzania (Figure 3).
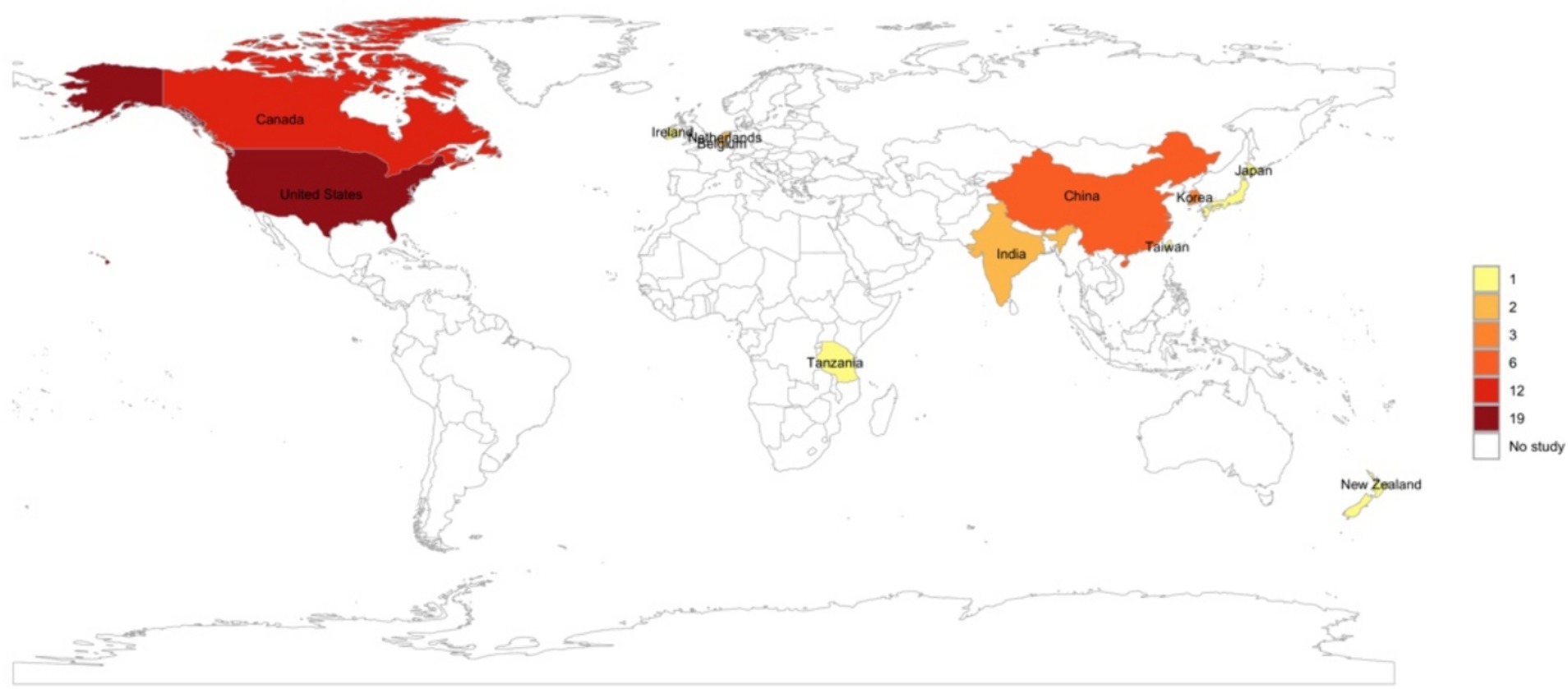
Figure 3. World map created using R statistical software showing the geographical distribution of studies included in the review.
Eighty-two percent (42 out of 51) of the studies employed TLR agonists as vaccine adjuvants, while 18% (9 out of 51) utilized TLR agonists for therapeutic purposes. The diseases addressed in the reviewed studies encompassed viral diseases (76%), bacterial diseases (12%), protozoal diseases (6%), and parasitic infections (6%). Inactivated vaccines (41%) and subunit vaccines (33%) emerged as the most frequently utilized types in our review. The studies employed four types of animals, including primates, cattle, swine, and chickens, with non-primates (41%) being the most commonly used animal across the studies reviewed (Table 3). Across the studies, TLR agonists were administered through various routes, such as intra-air sac, intradermal, intramuscular, intranasal, intra-tracheal, oral, and subcutaneous. Given that some studies evaluated the administration of TLR agonists through multiple routes, notably, the intramuscular route (50%) was the most common method of TLR agonist delivery (Table 3).
3.3 Application of TLR agonists as adjuvants and drugs
Several TLR agonist were evaluated, including TLR1/2, TLR2, TLR3, TLR4, TLR 2/4, TLR5, TLR7, TLR7/8, and TLR9 agonist. Among these, CpG-ODN, a TLR9 agonist in mammals and a TLR21 agonist in chickens emerged as the predominantly used in 37% (19/51) of the studies (Table 4).
3.4 Description of efficacy outcomes
Of the studies reviewed, 92% (47/51) assessed the efficacy and immunogenicity of TLR agonists, whereas 8% (4/51) assessed the safety and immunogenicity of TLR agonists. The outcomes of the application of TLR agonists in the studies reviewed were measured by assessment of humoral and cellular responses. 90% (46/51) of the studies reported enhanced antigen-specific humoral and cellular immune responses using TLR agonists compared to control groups. Within the control groups, 45% (23/51) received placebo, 22% (11/51) were administered either antigen without adjuvant, 16% (8/51) were left unvaccinated, while 12% (6/51) received adjuvant without antigen or were administered as a drug, and 4% (2/51) received a vehicle without either antigen or adjuvant. The incorporation of TLR agonists resulted in enhanced vaccine or therapeutic efficacy, as reported in 90% (46/51) of the studies (Table 5). Three studies (6%, 3/51) of the studies quantified the efficacy of vaccines that utilized TLR agonists either as adjuvants or therapeutic agents, including 57, 70, and 100% (35–37). Forty-one percent (21/51) of the studies reviewed investigated the utilization of multiple TLR agonists either in combination or as individual adjuvants. Among these, 43% (9/21) explored the synergistic effects of TLR agonists observing enhanced humoral and/or cellular immune responses with combined TLR agonists compared to singular TLR agonist application (Tables 5, 6). In summary, specific beneficial outcomes of using TLR agonists included reduced viremia, increased survival rate of animals, enhanced protection against diseases, and reduced clinical symptoms of the various infectious diseases evaluated (Figure 4).
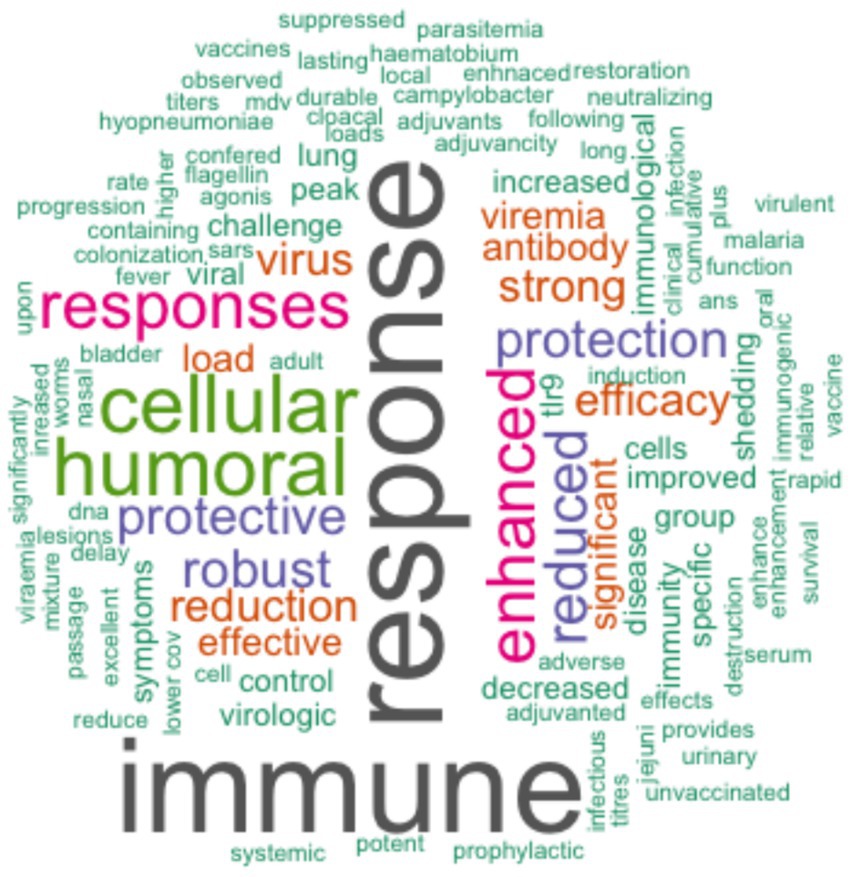
Figure 4. Word cloud created using R statistical software showing a summary of specific outcomes from the reviewed studies on TLR agonists. The size of each word corresponds to the frequency of occurrence of the respective outcome across the studies that had the specified outcome.
3.5 Description of safety outcomes
The safety outcomes in the studies we reviewed were qualitatively assessed. Eight percent (4/51) of the studies evaluated the adverse effects of the TLR agonists as vaccine adjuvants. One of the studies, reported adverse effects, including fever, chronic inflammation, and granuloma in the use of TLR1/2, TLR9, and TLR8 agonists combination administered intramuscularly in swine (38) (Table 7).
3.6 Quality of the studies
In approximately 59% (30/51) of the studies, randomization was reported, while 41% (21/51) of studies did not. According to the Systematic Review Centre for Laboratory Animal Experimentation (SYRCLE) risk of bias assessment, all the studies showed a low risk of bias from baseline characteristics, incomplete outcome data, and selective outcome (Figure 5). All the studies failed to report on allocation concealment, random housing, performance blinding, and detection binding, with 60% (31/51) reporting on sequence generation (Figure 5). All the studies clearly stated objectives and methodology, including types of data collected and the location of the study. Approximately 98% of the studies reported the sample size and the study groups. However, none of the studies indicated a statistical sample size calculation method.
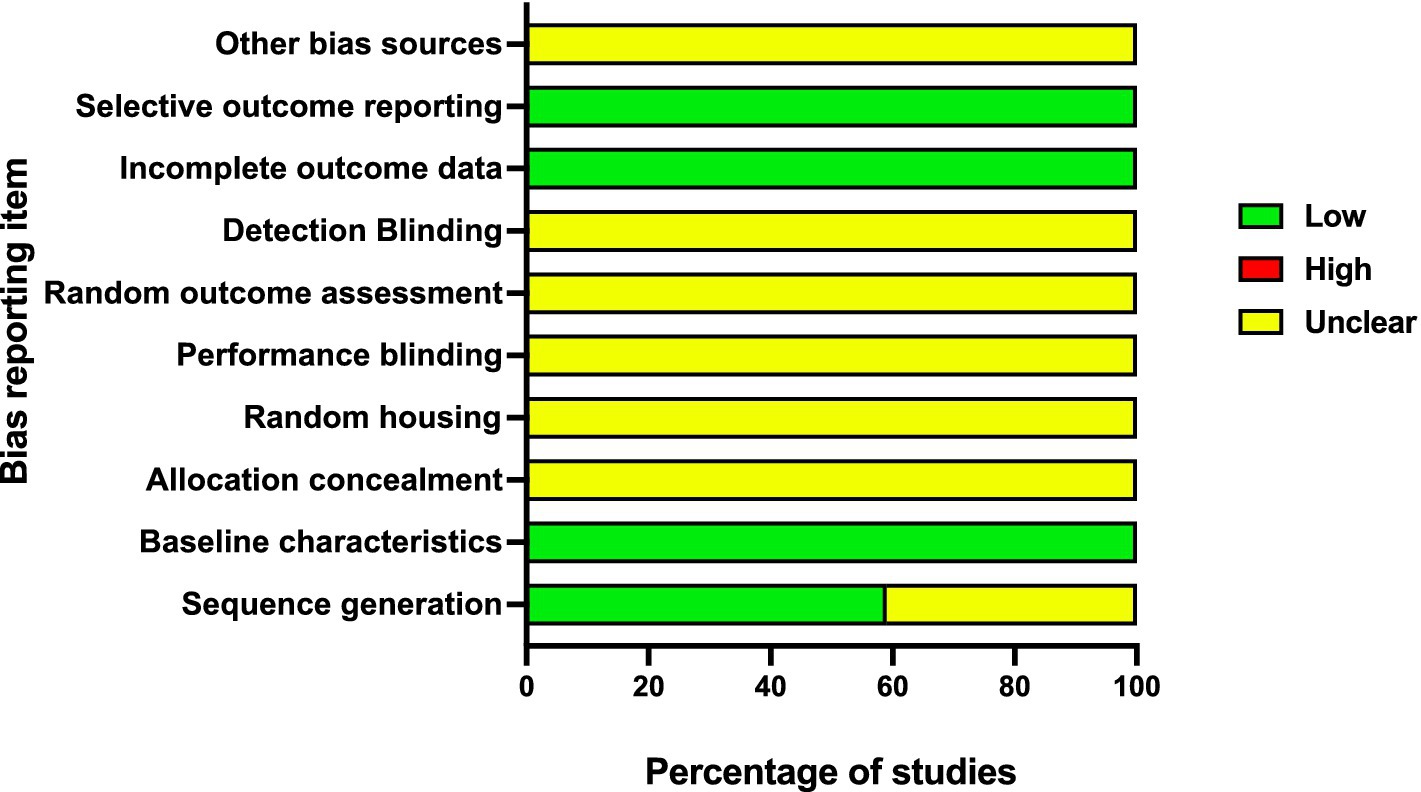
Figure 5. Summary of risk of bias score from all the studies in each domain according to SYRCLE protocol.
4 Discussion
The review assessed the efficacy and safety of TLR agonists as therapeutic agents and vaccine adjuvants for infectious diseases. This study covers 51 studies. Our review shows TLR agonists are used more as vaccine adjuvants than therapeutic agents against infectious diseases in animals. As adjuvants, most TLR agonists effectively enhance immune response. They increased the efficacy of vaccines against infectious diseases, including viral, bacterial, protozoal, and parasitic infections, with viral infections attributing to 70% of the diseases assessed. A combination of TLR agonists resulted in synergistic effects with robust humoral and cellular immune responses that enhanced vaccine efficacy. Most of the studies did not evaluate the safety profile of the TLR agonists. Only 8% of the studies assessed adverse effects of specific TLR agonists. Adverse effects, including fever, chronic inflammation, and granuloma, were reported using a combination of TLR1/2, TLR9, and TLR8 agonists in swine.
TLRs play a crucial role in initiating innate immune responses and linkage to adaptive immune responses, rendering them promising targets for enhancing the efficacy of animal vaccines and therapeutics (18). The ability of TLRs to detect and respond to pathogens has highlighted their potential role as therapeutic agents in both infectious and noninfectious diseases. Targeting key processes in innate immunity could be explored for the prevention of infectious diseases in animals (39). By enhancing the host’s immune system, TLR agonists can mitigate the likelihood of infections. With the increasing global concern over antimicrobial resistance, there is a surge in identifying alternative therapeutics, and TLR agonists have been gaining popularity in this context over the years (1). In veterinary medicine, the growing risk of antimicrobial resistance necessitates the exploration of such alternatives. The strategic use of TLR agonists could potentially offer a means to combat infections while minimizing the reliance on traditional antibiotics, thus contributing to the global effort to manage and reduce antimicrobial resistance.
Recent developments in vaccinology have shown a surge in the utilization of non-pathogenic alternatives to traditional vaccines, including subunit vaccines, DNA vaccines, and mRNA vaccines. While these alternatives have gained demonstrated immunogenicity in murine studies, their efficacy in large animals remains limited. Consequently, there is a need to incorporate potent adjuvants to boost their immunogenicity (40, 41). Adjuvants act as immunostimulatory agents, and some act as vaccine delivery carriers, impacting the efficacy and safety of vaccine antigens (42). In the current review, TLR 9 agonists emerged as a popular TLR agonist for the infectious diseases reviewed. Other studies have reported high adjuvanticity in CpG ODN, a TLR 9 agonist, for diseases such as malaria, hepatitis B, HIV, anthrax, and COVID-19 (43). The wide application of TLR9 in this review indicates that it is effective in enhancing targeted immune responses in animals either as vaccine adjuvants or therapeutic agents against infectious diseases. This finding is consistent with other studies that have demonstrated the efficiency and effectiveness of TLR 9 and TLR 7 to clear viral pathogens (44, 45).
The synergistic effects of TLR agonists can be observed when they are used in combination with other adjuvants or therapeutic agents. For example, the combination of TLR3 and TLR9 agonists has been shown to significantly reduce clinical signs of canine herpesvirus infection (46). Similarly, the co-administration of vaccines with TLR agonists in combination with other adjuvants such as saponins has been shown to enhance protective immunity in livestock against bacterial and viral pathogens (47). Covalently linked TLR agonists confined in a particle format have also been shown to increase immune stimulation (48). These combinations leverage different aspects of the immune response, resulting in a more comprehensive defense against infectious agents. In the review, it was observed that combining TLR agonists resulted in a stronger immune response compared to using a single TLR agonist. Importantly, TLR agonists associated with T-cell responses are particularly desirable for therapeutics and vaccine efficacy against many infectious diseases in animals. TLR2, TLR3, TLR7, and TLR9 agonists have been shown to elicit CD8+ T cell responses and confer protective immunity against livestock disease pathogens, including Theileria spp., babesia spp., toxoplasma spp., Trypanosoma spp., and leishmania spp. (49).
Activation of TLRs by their ligands leads to a signaling cascade specific to the type of ligand, interacting receptors, and the adaptor molecules engaged in the signaling (50). However, pro-inflammatory cytokines and chemokines targeting pathogen clearance can result in excessive inflammatory reactions that can be harmful to the host (51). Furthermore, other studies have highlighted adverse effects in human trials related to formulations of TLR4, TLR 5, and TLR 9 agonists (52, 53). Generally, TLR agonists have been documented to have good safety profiles in cancer therapeutics in human trials. However, this review demonstrates the deficiency in establishing the safety profile of TLR agonists in animals in vivo, despite TLR agonists having a broad spectrum of pathogen-derived compounds and the complexity of species immunopathological responses, which may influence the side effects of TLR adjuvants (54, 55). In this review, non-human primates comprise the largest proportion of experimental animals, surpassing chickens, cattle, and swine. The popularity of non-human primates encountered in this review shapes the scope of infectious diseases investigated. Consequently, in this review, there was a significant scarcity of studies involving large animals such as cattle and pigs, leading to a bias in the infectious diseases covered in this review. Additional studies are essential to ascertain the safety profiles of TLR agonists in animals, as this factor profoundly impacts the efficacy outcomes of TLR agonists and, consequently, the efficacy of associated vaccines and therapeutics. Furthermore, the safety and efficacy of TLR agonists are affected by the route of administration, which is informed by their therapeutic purpose (56–58). In this review, the intramuscular and subcutaneous routes were the most common routes for TLR agonist administration. Local administration, such as the subcutaneous route, is more convenient than the systemic, intravenous route, and it has been shown to yield greater bioavailability than the oral route (56, 59, 60).
The application of TLR agonists shows great potential as prophylactic and therapeutic agents, as well as adjuvants, in veterinary research and medicine. This review demonstrates their effectiveness as potent adjuvants that can be incorporated into veterinary vaccines for infectious diseases to enhance efficacy and durability. Although the application of TLR agonists as therapeutics is currently limited, the reviewed studies highlight their potential as stand-alone therapeutics to boost immune responses in animals already infected with pathogens. The growing issue of antimicrobial resistance further emphasizes the need to develop alternative strategies, such as TLR agonists, to manage infectious diseases in animals.
The methodological limitations identified in this review highlight the ongoing challenges in the quality of methodology reporting in animal experiments, generally indicating potential reporting bias. Some studies did not mention randomization, which could introduce selection bias and confounding factors into the experiments (34, 57). Additionally, the failure to report allocation concealment, random housing, blinding, and detection blinding in all studies suggests potential selection bias and performance bias, which could compromise the validity and reliability of the findings (34). The absence of techniques for sampling and determination of sample sizes could lead to selection bias and affect the statistical power of the study, potentially resulting in inconclusive outcomes and ethical issues related to unnecessarily large or too small sample sizes (61). Addressing these methodological shortcomings in future animal research is essential to enhance the validity of research outcomes.
Moreover, this systematic review is subject to certain limitations that should be considered when interpreting its findings. The safety profile of TLR agonists presented in this study is not exhaustive. Given the rapid rise in the use of TLR agonists in veterinary medicine, evaluating and documenting any side effects associated with their use is crucial, no matter how mild they may seem. Murine-related studies, which comprise the majority of animal experimental studies, were excluded due to differences in physiological and pathological systems between large animals and murine models. These differences can significantly impact disease development and impede translational medicine efforts (62).
Future research should focus on assessing the safety profile of TLR agonists in large animals individually, rather than as part of a vaccine. Dose titration studies should be conducted to determine the appropriate dosages of TLR agonists for various animals, particularly when used as therapeutic agents. This could lead to improvements in their formulation and delivery methods, thereby maximizing their efficacy. Understanding the specific interactions between different TLR agonists and their safety profiles could enhance their therapeutic and adjuvant properties, targeting both synergy and the therapeutic potential of their antagonistic effects against specific infectious diseases in animals. Additionally, futute studies should incorporate a wider variety of animal models and large-scale field trials. These are necessary to validate the effectiveness of TLR agonists across diverse animal populations and environmental conditions.
5 Conclusion
Toll-like receptor agonists are efficacious immune response enhancers and thus have great potential in animal health interventions. Their synergistic effects when combining two or more TLR agonists or with other immune-modulating agents offer a powerful approach to improving disease outcomes both as vaccines and therapeutic agents. With the rising global concerns over antimicrobial resistance (AMR), the application of TLR agonists as alternative therapeutics could be revolutionary in veterinary medicine. Apart from mitigating AMR, these agents have the potential to be not only more cost-effective but also more efficient, as therapeutics like antibiotics are dosage-dependent on weight.
More effort should be devoted to collecting comprehensive data on the safety profile of TLR agonists. Understanding these safety profiles is crucial for identifying appropriate candidates for use across different animal species, considering variability in immunopathological responses, and informing the selection of safe and efficacious adjuvants.
Data availability statement
The original contributions presented in the study are included in the article/Supplementary material, further inquiries can be directed to the corresponding author.
Author contributions
HO: Conceptualization, Data curation, Formal analysis, Investigation, Methodology, Project administration, Software, Validation, Visualization, Writing – original draft, Writing – review & editing. VR: Writing – review & editing. MN: Formal analysis, Visualization, Writing – review & editing. GO: Writing – review & editing. AL: Funding acquisition, Supervision, Writing – review & editing. NG: Writing – review & editing. VN: Funding acquisition, Supervision, Writing – review & editing. GA: Supervision, Writing – review & editing. ST: Funding acquisition, Resources, Supervision, Writing – review & editing.
Funding
The author(s) declare that financial support was received for the research, authorship, and/or publication of this article. This work was funded by the United States Agency for International Development (USAID) Bureau for Resilience and Food Security under Agreement # 7200AA20CA00022 as part of Feed the Future Innovation Lab for Animal Health. Any opinions, findings, conclusions, or recommendations expressed here are those of the authors alone.
Acknowledgments
We would like to acknowledge the use of ChatGPT-4, an AI language model developed by OpenAI (https://openai.com/chatgpt/), for providing assistance in refining textual content during the research process.
Conflict of interest
The authors declare that the research was conducted in the absence of any commercial or financial relationships that could be construed as a potential conflict of interest.
Publisher’s note
All claims expressed in this article are solely those of the authors and do not necessarily represent those of their affiliated organizations, or those of the publisher, the editors and the reviewers. Any product that may be evaluated in this article, or claim that may be made by its manufacturer, is not guaranteed or endorsed by the publisher.
Supplementary material
The Supplementary material for this article can be found online at: https://www.frontiersin.org/articles/10.3389/fvets.2024.1428713/full#supplementary-material
References
1. Asokan, GV, and Kasimanickam, RK. Emerging infectious diseases, antimicrobial resistance and millennium development goals: resolving the challenges through one health. Cent Asian J Glob Health. (2014) 2:76. doi: 10.5195/cajgh.2013.76
2. Rosini, R, Nicchi, S, Pizza, M, and Rappuoli, R. Vaccines against antimicrobial resistance. Front Immunol. (2020) 11:1048. doi: 10.3389/fimmu.2020.01048
3. Ellwanger, JH, Veiga, ABGD, Kaminski, VDL, Valverde-Villegas, JM, Freitas, AWQD, and Chies, JAB. Control and prevention of infectious diseases from a one health perspective. Genet Mol Biol. (2021) 44:e20200256. doi: 10.1590/1678-4685-gmb-2020-0256
4. Cleaveland, S, Laurenson, MK, and Taylor, LH. Diseases of humans and their domestic mammals: pathogen characteristics, host range and the risk of emergence. Philos Trans R Soc Lond Ser B Biol Sci. (2001) 356:991–9. doi: 10.1098/rstb.2001.0889
5. Taylor, LH, Latham, SM, and Woolhouse, MEJ. Risk factors for human disease emergence. Philos Trans R Soc Lond Ser B Biol Sci. (2001) 356:983–9. doi: 10.1098/rstb.2001.0888
6. Pasquale, A, Preiss, S, Silva, F, and Garçon, N. Vaccine adjuvants: from 1920 to 2015 and beyond. Vaccine. (2015) 3:320–43. doi: 10.3390/vaccines3020320
7. Liljeqvist, S, and Ståhl, S. Production of recombinant subunit vaccines: protein immunogens, live delivery systems and nucleic acid vaccines. J Biotechnol. (1999) 73:1–33. doi: 10.1016/S0168-1656(99)00107-8
8. Minor, PD. Live attenuated vaccines: historical successes and current challenges. Virology. (2015) 479-480:379–92. doi: 10.1016/j.virol.2015.03.032
9. De Deken, R, Martin, V, Saido, A, Madder, M, Brandt, J, and Geysen, D. An outbreak of East coast fever on the comoros: a consequence of the import of immunised cattle from Tanzania? Vet Parasitol. (2007) 143:245–53. doi: 10.1016/j.vetpar.2006.08.018
10. Magulu, E, Kindoro, F, Mwega, E, Kimera, S, Shirima, G, and Gwakisa, P. Detection of carrier state and genetic diversity of Theileria parva in ECF-vaccinated and naturally exposed cattle in Tanzania. Vet Parasitol Reg Stud Rep. (2019) 17:100312. doi: 10.1016/j.vprsr.2019.100312
11. Moyle, PM, and Toth, I. Modern subunit vaccines: development, components, and research opportunities. ChemMedChem. (2013) 8:360–76. doi: 10.1002/cmdc.201200487
12. Skwarczynski, M, and Toth, I. (eds.). Micro- and nanotechnology in vaccine development – introduction In: Micro- and nanotechnology in vaccine development. Kidlington, Oxford, United Kingdom: Elsevier Science (2017). XVII–XVIII.
13. Marciani, DJ. Vaccine adjuvants: role and mechanisms of action in vaccine immunogenicity. Drug Discov Today. (2003) 8:934–43. doi: 10.1016/S1359-6446(03)02864-2
14. Vogel, FR. Improving vaccine performance with adjuvants. Clin Infect Dis. (2000) 30:S266–70. doi: 10.1086/313883
15. Gupta, RK, Relyveld, EH, Lindblad, EB, Bizzini, B, Ben-Efraim, S, and Gupta, CK. Adjuvants—a balance between toxicity and adjuvanticity. Vaccine. (1993) 11:293–306. doi: 10.1016/0264-410X(93)90190-9
16. Marrack, P, McKee, AS, and Munks, MW. Towards an understanding of the adjuvant action of aluminium. Nat Rev Immunol. (2009) 9:287–93. doi: 10.1038/nri2510
17. Singh, M, Ugozzoli, M, Kazzaz, J, Chesko, J, Soenawan, E, Mannucci, D, et al. A preliminary evaluation of alternative adjuvants to alum using a range of established and new generation vaccine antigens. Vaccine. (2006) 24:1680–6. doi: 10.1016/j.vaccine.2005.09.046
18. Janeway, CA, and Medzhitov, R. Innate immune recognition. Annu Rev Immunol. (2002) 20:197–216. doi: 10.1146/annurev.immunol.20.083001.084359
19. Levitz, SM, and Golenbock, DT. Beyond empiricism: informing vaccine development through innate immunity research. Cell. (2012) 148:1284–92. doi: 10.1016/j.cell.2012.02.012
20. Ghosh, D, and Stumhofer, J. Do you see what I see: recognition of protozoan parasites by toll-like receptors. Curr Immunol Rev. (2014) 9:129–40. doi: 10.2174/1573395509666131203225929
21. Yarovinsky, F, and Sher, A. Toll-like receptor recognition of toxoplasma gondii. Int J Parasitol. (2006) 36:255–9. doi: 10.1016/j.ijpara.2005.12.003
22. Kawai, T, and Akira, S. Innate immune recognition of viral infection. Nat Immunol. (2006) 7:131–7. doi: 10.1038/ni1303
23. Nie, L, Cai, SY, Shao, JZ, and Chen, J. Toll-like receptors, associated biological roles, and signaling networks in non-mammals. Front Immunol. (2018) 9:1523. doi: 10.3389/fimmu.2018.01523
24. Brownlie, R, and Allan, B. Avian toll-like receptors. Cell Tissue Res. (2011) 343:121–30. doi: 10.1007/s00441-010-1026-0
25. Medvedev, AE. Toll-like receptor polymorphisms, inflammatory and infectious diseases, allergies, and cancer. J Interf Cytokine Res. (2013) 33:467–84. doi: 10.1089/jir.2012.0140
26. Pandey, S, Kawai, T, and Akira, S. Microbial sensing by toll-like receptors and intracellular nucleic acid sensors. Cold Spring Harb Perspect Biol. (2015) 7:a016246. doi: 10.1101/cshperspect.a016246
27. Heegaard, PMH, Dedieu, L, Johnson, N, Le Potier, MF, Mockey, M, Mutinelli, F, et al. Adjuvants and delivery systems in veterinary vaccinology: current state and future developments. Arch Virol. (2011) 156:183–202. doi: 10.1007/s00705-010-0863-1
28. Owen, AM, Fults, JB, Patil, NK, Hernandez, A, and Bohannon, JK. TLR agonists as mediators of trained immunity: mechanistic insight and immunotherapeutic potential to combat infection. Front Immunol. (2021) 11:622614. doi: 10.3389/fimmu.2020.622614
29. Pashine, A, Valiante, NM, and Ulmer, JB. Targeting the innate immune response with improved vaccine adjuvants. Nat Med. (2005) 11:S63–8. doi: 10.1038/nm1210
30. Oboge, H, Mwangi, T, Nene, V, Oluga, G, Riitho, V, Nyamai, M, et al. Immunogenicity, efficacy, and safety of TLR agonists as therapeutic agents and adjuvants TargetingAnimal models of infectious diseases: a systematic review. PROSPERO 2023 CRD42023323122. Available at: https://www.crd.york.ac.uk/prospero/display_record.php?ID=CRD42023323122 (Accessed Feburary 20, 2024).
31. Tawfik, GM, Dila, KAS, Mohamed, MYF, Tam, DNH, Kien, ND, Ahmed, AM, et al. A step by step guide for conducting a systematic review and meta-analysis with simulation data. Trop Med Health. (2019) 47:46. doi: 10.1186/s41182-019-0165-6
32. Hartung, T. Thoughts on limitations of animal models. Parkinsonism Relat Disord. (2008) 14:S81–3. doi: 10.1016/j.parkreldis.2008.04.003
33. Steger-Hartmann, T, and Raschke, M. Translating in vitro to in vivo and animal to human. Curr Opin Toxicol. (2020) 23-24:6–10. doi: 10.1016/j.cotox.2020.02.003
34. Hooijmans, CR, Rovers, MM, De Vries, RB, Leenaars, M, Ritskes-Hoitinga, M, and Langendam, MW. SYRCLE’s risk of bias tool for animal studies. BMC Med Res Methodol. (2014) 14:43. doi: 10.1186/1471-2288-14-43
35. Johnson, RF, Kurup, D, Hagen, KR, Fisher, C, Keshwara, R, Papaneri, A, et al. An inactivated rabies virus-based Ebola vaccine, FILORAB1, adjuvanted with glucopyranosyl lipid a in stable emulsion confers complete protection in nonhuman primate challenge models. J Infect Dis. (2016) 214:S342–54. doi: 10.1093/infdis/jiw231
36. Khatri, V, Chauhan, N, Vishnoi, K, von Gegerfelt, A, Gittens, C, and Kalyanasundaram, R. Prospects of developing a prophylactic vaccine against human lymphatic, filariasis – evaluation of protection in non-human primates. Int J Parasitol. (2018) 48:773–83. doi: 10.1016/j.ijpara.2018.04.002
37. Walker-Sperling, VEK, Mercado, NB, Chandrashekar, A, Borducchi, EN, Liu, J, Nkolola, JP, et al. Therapeutic efficacy of combined active and passive immunization in ART-suppressed, SHIV-infected rhesus macaques. Nat Commun. (2022) 13:3463. doi: 10.1038/s41467-022-31196-5
38. Matthijs, AMF, Auray, G, Jakob, V, García-Nicolás, O, Braun, RO, Keller, I, et al. Systems immunology characterization of novel vaccine formulations for Mycoplasma hyopneumoniae bacterins. Front Immunol. (2019) 10:1087. doi: 10.3389/fimmu.2019.01087
39. Hennessy, EJ, Parker, AE, and O’Neill, LAJ. Targeting toll-like receptors: emerging therapeutics? Nat Rev Drug Discov. (2010) 9:293–307. doi: 10.1038/nrd3203
40. Bill, RM. Recombinant protein subunit vaccine synthesis in microbes: a role for yeast? J Pharm Pharmacol. (2015) 67:319–28. doi: 10.1111/jphp.12353
41. Coffman, RL, Sher, A, and Seder, RA. Vaccine adjuvants: putting innate immunity to work. Immunity. (2010) 33:492–503. doi: 10.1016/j.immuni.2010.10.002
42. Fan, J, Jin, S, Gilmartin, L, Toth, I, Hussein, WM, and Stephenson, RJ. Advances in infectious disease vaccine adjuvants. Vaccine. (2022) 10:1120. doi: 10.3390/vaccines10071120
43. Yang, JX, Tseng, JC, Yu, GY, Luo, Y, Huang, CYF, Hong, YR, et al. Recent advances in the development of toll-like receptor agonist-based vaccine adjuvants for infectious diseases. Pharmaceutics. (2022) 14:423. doi: 10.3390/pharmaceutics14020423
44. Bezemer, GFG, and Garssen, J. TLR9 and COVID-19: a multidisciplinary theory of a multifaceted therapeutic target. Front Pharmacol. (2021) 11:601685. doi: 10.3389/fphar.2020.601685
45. Martinsen, JT, Gunst, JD, Højen, JF, Tolstrup, M, and Søgaard, OS. The use of toll-like receptor agonists in HIV-1 cure strategies. Front Immunol. (2020) 11:1112. doi: 10.3389/fimmu.2020.01112
46. Wheat, W, Chow, L, Kuzmik, A, Soontararak, S, Kurihara, J, Lappin, M, et al. Local immune and microbiological responses to mucosal administration of a liposome-TLR agonist immunotherapeutic in dogs. BMC Vet Res. (2019) 15:330. doi: 10.1186/s12917-019-2073-8
47. Choi, JH, You, SH, Ko, MK, Jo, HE, Shin, SH, Jo, H, et al. Improved immune responses and safety of foot-and-mouth disease vaccine containing immunostimulating components in pigs. J Vet Sci. (2020) 21:e74. doi: 10.4142/jvs.2020.21.e74
48. Albin, TJ, Tom, JK, Manna, S, Gilkes, AP, Stetkevich, SA, Katz, BB, et al. Linked toll-like receptor triagonists stimulate distinct, combination-dependent innate immune responses. ACS Cent Sci. (2019) 5:1137–45. doi: 10.1021/acscentsci.8b00823
49. Gurung, P, and Kanneganti, TD. Immune responses against protozoan parasites: a focus on the emerging role of nod-like receptors. Cell Mol Life Sci. (2016) 73:3035–51. doi: 10.1007/s00018-016-2212-3
50. Sameer, AS, and Nissar, S. Toll-like receptors (TLRs): structure, functions, signaling, and role of their polymorphisms in colorectal cancer susceptibility. Biomed Res Int. (2021) 2021:1–14. doi: 10.1155/2021/1157023
51. West, AP, Koblansky, AA, and Ghosh, S. Recognition and signaling by toll-like receptors. Annu Rev Cell Dev Biol. (2006) 22:409–37. doi: 10.1146/annurev.cellbio.21.122303.115827
52. Adams, S. Toll-like receptor agonists in cancer therapy. Immunotherapy. (2009) 1:949–64. doi: 10.2217/imt.09.70
53. Rolfo, C, Giovannetti, E, Martinez, P, McCue, S, and Naing, A. Applications and clinical trial landscape using toll-like receptor agonists to reduce the toll of cancer. Npj Precis Oncol. (2023) 7:26. doi: 10.1038/s41698-023-00364-1
54. Petrovsky, N. Comparative safety of vaccine adjuvants: a summary of current evidence and future needs. Drug Saf. (2015) 38:1059–74. doi: 10.1007/s40264-015-0350-4
55. Schroder, K, Irvine, KM, Taylor, MS, Bokil, NJ, Le Cao, KA, Masterman, KA, et al. Conservation and divergence in toll-like receptor 4-regulated gene expression in primary human versus mouse macrophages. Proc Natl Acad Sci. (2012) 109:E944–53. doi: 10.1073/pnas.1110156109
56. Engel, AL, Holt, GE, and Lu, H. The pharmacokinetics of toll-like receptor agonists and the impact on the immune system. Expert Rev Clin Pharmacol. (2011) 4:275–89. doi: 10.1586/ecp.11.5
57. Nierkens, S, Den Brok, MH, Roelofsen, T, Wagenaars, JAL, Figdor, CG, Ruers, TJ, et al. Route of administration of the TLR9 agonist CpG critically determines the efficacy of cancer immunotherapy in mice. PLoS One. (2009) 4:e8368. doi: 10.1371/journal.pone.0008368
58. Wan, D, Que, H, Chen, L, Lan, T, Hong, W, He, C, et al. Lymph-node-targeted cholesterolized TLR7 agonist liposomes provoke a safe and durable antitumor response. Nano Lett. (2021) 21:7960–9. doi: 10.1021/acs.nanolett.1c01968
59. Fletcher, S, Steffy, K, and Averett, D. Masked oral prodrugs of toll-like receptor 7 agonists: a new approach for the treatment of infectious disease. Curr Opin Investig Drugs. (2006) 7:702–8.
60. Harrison, LI, Astry, C, Kumar, S, and Yunis, C. Pharmacokinetics of 852A, an imidazoquinoline toll-like receptor 7-specific agonist, following intravenous, subcutaneous, and oral administrations in humans. J Clin Pharmacol. (2007) 47:962–9. doi: 10.1177/0091270007303766
61. Andrade, C. Sample size and its importance in research. Indian J Psychol Med. (2020) 42:102–3. doi: 10.4103/IJPSYM.IJPSYM_504_19
62. Gerdts, V, Wilson, HL, Meurens, F, Littel, VD, Van Den Hurk, S, Wilson, D, et al. Large animal models for vaccine development and testing. ILAR J. (2015) 56:53–62. doi: 10.1093/ilar/ilv009
63. Ahmad, G, Zhang, W, Torben, W, Ahrorov, A, Damian, R, Wolf, R, et al. Preclinical prophylactic efficacy testing of Sm-p80-based vaccine in a nonhuman primate model of Schistosoma mansoni infection and immunoglobulin G and E responses to Sm-p80 in human serum samples from an area where schistosomiasis is endemic. J Infect Dis. (2011) 204:1437–49. doi: 10.1093/infdis/jir545
64. Alqazlan, N, Astill, J, Taha-Abdelaziz, K, Nagy, É, Bridle, B, and Sharif, S. Probiotic lactobacilli enhance immunogenicity of an inactivated H9N2 influenza virus vaccine in chickens. Viral Immunol. (2021) 34:86–95. doi: 10.1089/vim.2020.0209
65. Barjesteh, N, Shojadoost, B, Brisbin, J, Emam, M, Hodgins, D, Nagy, E, et al. Reduction of avian influenza virus shedding by administration of toll-like receptor ligands to chickens. Vaccine. (2015) 33:4843–9. doi: 10.1016/j.vaccine.2015.07.070
66. Dhakal, S, Ghimire, S, Renu, S, Ross, KA, Lakshmanappa, YS, Hogshead, BT, et al. Evaluation of CpG-ODN-adjuvanted polyanhydride-based intranasal influenza nanovaccine in pigs. Vet Microbiol. (2019) 237:108401. doi: 10.1016/j.vetmic.2019.108401
67. Kwissa, M, Amara, R, Robinson, H, Moss, B, Alkan, S, Jabbar, A, et al. Adjuvanting a DNA vaccine with a TLR9 ligand plus Flt3 ligand results in enhanced cellular immunity against the simian immunodeficiency virus. J Exp Med. (2007) 204:2733–46. doi: 10.1084/jem.20071211
68. Li, C, Huang, X, Cai, J, Lu, A, Hao, S, Zhang, Z, et al. The immunomodulatory functions of various CpG oligodeoxynucleotideson CEF cells and H9N2 subtype avian influenza virus vaccination. Vaccine. (2022) 10:616. doi: 10.3390/vaccines10040616
69. Lin, X, Mohsin, M, Abbas, R, Li, L, Chen, H, Huang, C, et al. Evaluation of immunogenicity and protective efficacy of eimeria maxima immune mapped protein 1 with EDA adjuvant in chicken. Pak Vet J. (2020) 40:209–13. doi: 10.29261/pakvetj/2020.043
70. Matthijs, A, Auray, G, Boyen, F, Schoos, A, Michiels, A, Garcia-Nicolas, O, et al. Efficacy of three innovative bacterin vaccines against experimental infection with Mycoplasma hyopneumoniae. Vet Res. (2019) 50:91. doi: 10.1186/s13567-019-0709-0
71. Parvizi, P, Abdul-Careem, MF, Mallick, AI, Haq, K, Haghighi, HR, Orouji, S, et al. The effects of administration of ligands for toll-like receptor 4 and 21 against Marek’s disease in chickens. Vaccine. (2014) 32:1932–8. doi: 10.1016/j.vaccine.2014.01.082
72. Singh, SM, Alkie, TN, Hodgins, DC, Nagy, É, Shojadoost, B, and Sharif, S. Systemic immune responses to an inactivated, whole H9N2 avian influenza virus vaccine using class B CpG oligonucleotides in chickens. Vaccine. (2015) 33:3947–52. doi: 10.1016/j.vaccine.2015.06.043
73. Singh, SM, Alkie, TN, Nagy, É, Kulkarni, RR, Hodgins, DC, and Sharif, S. Delivery of an inactivated avian influenza virus vaccine adjuvanted with poly(D,L-lactic-co-glycolic acid) encapsulated CpG ODN induces protective immune responses in chickens. Vaccine. (2016) 34:4807–13. doi: 10.1016/j.vaccine.2016.08.009
74. St Paul, M, Barjesteh, N, Brisbin, J, Villaneueva, A, Read, L, Hodgins, D, et al. Effects of ligands for toll-like receptors 3, 4, and 21 as adjuvants on the immunogenicity of an avian influenza vaccine in chickens. Viral Immunol. (2014) 27:167–73. doi: 10.1089/vim.2013.0124
75. Taha-Abdelaziz, K, Astill, J, Shojadoost, B, Borrelli, S, Monteiro, M, and Sharif, S. Campylobacter-derived ligands induce cytokine and chemokine expression in chicken macrophages and cecal tonsil mononuclear cells. Vet Microbiol. (2020) 246:108732. doi: 10.1016/j.vetmic.2020.108732
76. Tougan, T, Aoshi, T, Coban, C, Katakai, Y, Kai, C, Yasutomi, Y, et al. TLR9 adjuvants enhance immunogenicity and protective efficacy of the SE36/AHG malaria vaccine in nonhuman primate models. Hum Vaccin Immunother. (2013) 9:283–90. doi: 10.4161/hv.22950
77. Vreman, S, McCaffrey, J, Popma-de Graaf, D, Nauwynck, H, Savelkoul, H, Moore, A, et al. Toll-like receptor agonists as adjuvants for inactivated porcine reproductive and respiratory syndrome virus (PRRSV) vaccine. Vet Immunol Immunopathol. (2019) 212:27–37. doi: 10.1016/j.vetimm.2019.04.008
78. Vreman, S, McCaffrey, J, Rebel, A, Moore, A, and Stockhofe-Zurwieden, N. Local immune responses after skin vaccination in neonatal and adult pigs with different toll-like-receptor agonists as adjuvant. J Comp Pathol. (2020) 174:149. doi: 10.1016/j.jcpa.2019.10.028
79. Vreman, S, Stockhofe-Zurwieden, N, Popma-de Graaf, DJ, Savelkoul, HFJ, Barnier-Quer, C, Collin, N, et al. Immune responses induced by inactivated porcine reproductive and respiratory syndrome virus (PRRSV) vaccine in neonatal pigs using different adjuvants. Vet Immunol Immunopathol. (2021) 232:110170. doi: 10.1016/j.vetimm.2020.110170
80. Garrido, C, Curtis, AD, Dennis, M, Pathak, SH, Gao, H, Montefiori, D, et al. SARS-CoV-2 vaccines elicit durable immune responses in infant rhesus macaques. Sci Immunol. (2021) 6:eabj3684. doi: 10.1126/sciimmunol.abj3684
81. Holbrook, B, Kim, J, Blevins, L, Jorgensen, M, Kock, N, D’Agostino, R, et al. A novel R848-conjugated inactivated influenza virus vaccine is efficacious and safe in a neonate nonhuman primate model. J Immunol. (2016) 197:555–64. doi: 10.4049/jimmunol.1600497
82. Pino, M, Abid, T, Pereira Ribeiro, S, Edara, VV, Floyd, K, Smith, JC, et al. A yeast expressed RBD-based SARS-CoV-2 vaccine formulated with 3M-052-alum adjuvant promotes protective efficacy in non-human primates. Sci Immunol. (2021) 6:eabh3634. doi: 10.1126/sciimmunol.abh3634
83. Tang, Y, Lu, J, Wu, P, Liu, Z, Tian, Z, Zha, G, et al. Inactivated vaccine with adjuvants consisting of pattern recognition receptor agonists confers protection against avian influenza viruses in chickens. Vet Microbiol. (2014) 172:120–8. doi: 10.1016/j.vetmic.2014.05.007
84. Iyer, S, Gangadhara, S, Victor, B, Shen, X, Chen, X, Nabi, R, et al. Virus-like particles displaying trimeric simian immunodeficiency virus (SIV) envelope gp160 enhance the breadth of DNA/modified vaccinia virus Ankara SIV vaccine-induced antibody responses in Rhesus macaques. J Virol. (2016) 90:8842–54. doi: 10.1128/JVI.01163-16
85. Karmakar, S, Zhang, W, Ahmad, G, Torben, W, Alam, MU, Le, L, et al. Cross-species protection: Schistosoma mansoni Sm-p80 vaccine confers protection against Schistosoma haematobium in hamsters and baboons. Vaccine. (2014) 32:1296–303. doi: 10.1016/j.vaccine.2013.12.057
86. Lumsden, JM, Pichyangkul, S, Srichairatanakul, U, Yongvanitchit, K, Limsalakpetch, A, Nurmukhambetova, S, et al. Evaluation of the safety and immunogenicity in rhesus monkeys of a recombinant malaria vaccine for plasmodium vivax with a synthetic toll-like receptor 4 agonist formulated in an emulsion. Infect Immun. (2011) 79:3492–500. doi: 10.1128/IAI.05257-11
87. Singh, S, Ramirez-Salazar, E, Doueiri, R, Valentin, A, Rosati, M, Hu, X, et al. Control of heterologous simian immunodeficiency virus SIVsmE660 infection by DNA and protein coimmunization regimens combined with different toll-like-receptor-4-based adjuvants in macaques. J Virol. (2018) 92:e00281-18. doi: 10.1128/JVI.00281-18
88. Zurawski, G, Zurawski, S, Flamar, AL, Richert, L, Wagner, R, Tomaras, GD, et al. Targeting HIV-1 Env gp140 to LOX-1 elicits immune responses in Rhesus macaques. PLoS One. (2016) 11:e0153484. doi: 10.1371/journal.pone.0153484
89. Bashir, K, Kappala, D, Singh, Y, Dar, J, Mariappan, A, Kumar, A, et al. Combination of TLR2 and TLR3 agonists derepress infectious bursal disease virus vaccine-induced immunosuppression in the chicken. Sci Rep. (2019) 9:8197. doi: 10.1038/s41598-019-44578-5
90. Kannaki, T, Priyanka, E, Abhilash, M, and Haunshi, S. Co-administration of toll-like receptor (TLR)-3 agonist poly I:C with different infectious bursal disease (IBD) vaccines improves IBD specific immune response in chicken. Vet Res Commun. (2021) 45:285–92. doi: 10.1007/s11259-021-09809-z
91. Park, H, Adamson, L, Ha, T, Mullen, K, Hagen, SI, Nogueron, A, et al. Polyinosinic-polycytidylic acid is the most effective TLR adjuvant for SIV gag protein-induced T cell responses in nonhuman primates. J Immunol. (2013) 190:4103–15. doi: 10.4049/jimmunol.1202958
92. Parvizi, P, Mallick, A, Haq, K, Haghighi, H, Orouji, S, Thanthrige-Don, N, et al. A toll-like receptor 3 ligand enhances protective effects of vaccination against Marek’s disease virus and hinders tumor development in chickens. Viral Immunol. (2012) 25:394–401. doi: 10.1089/vim.2012.0033
93. Sariol, CA, Martínez, MI, Rivera, F, Rodríguez, IV, Pantoja, P, Abel, K, et al. Decreased dengue replication and an increased anti-viral humoral response with the use of combined toll-like receptor 3 and 7/8 agonists in macaques. PLoS One. (2011) 6:e19323. doi: 10.1371/journal.pone.0019323
94. Bekerman, E, Hesselgesser, J, Carr, B, Nagel, M, Hung, M, Wang, A, et al. PD-1 blockade and TLR7 activation lack therapeutic benefit in chronic simian immunodeficiency virus-infected macaques on antiretroviral therapy. Antimicrob Agents Chemother. (2019) 63:e01163–19. doi: 10.1128/AAC.01163-19
95. Bricker, KM, Obregon-Perko, V, Uddin, F, Williams, B, Uffman, EA, Garrido, C, et al. Therapeutic vaccination of SIV-infected, ART-treated infant rhesus macaques using Ad48/MVA in combination with TLR-7 stimulation. PLoS Pathog. (2020) 16:e1008954. doi: 10.1371/journal.ppat.1008954
96. Huang, Q, Yang, H, Yang, D, Hao, Y, Yu, S, Guo, Z, et al. A synthetic toll-like receptor 7 agonist inhibits porcine reproductive and respiratory syndrome virus replication in piglets. Vet Microbiol. (2022) 271:109475. doi: 10.1016/j.vetmic.2022.109475
97. Lanford, RE, Guerra, B, Chavez, D, Giavedoni, L, Hodara, VL, Brasky, KM, et al. GS-9620, an oral agonist of toll-like receptor-7, induces prolonged suppression of hepatitis B virus in chronically infected chimpanzees. Gastroenterology. (2013) 144:1508–1517.e10. doi: 10.1053/j.gastro.2013.02.003
98. Ventura, JD, Nkolola, JP, Chandrashekar, A, Borducchi, EN, Liu, J, Mercado, NB, et al. Therapeutic efficacy of an Ad26/MVA vaccine with SIV gp140 protein and vesatolimod in ART-suppressed rhesus macaques. Npj Vaccines. (2022) 7:53. doi: 10.1038/s41541-022-00477-x
99. Mokhtar, H, Biffar, L, Somavarapu, S, Frossard, JP, McGowan, S, Pedrera, M, et al. Evaluation of hydrophobic chitosan-based particulate formulations of porcine reproductive and respiratory syndrome virus vaccine candidate T cell antigens. Vet Microbiol. (2017) 209:66–74. doi: 10.1016/j.vetmic.2017.01.037
100. Hajam, IA, Kim, JH, and Lee, JH. Incorporation of membrane-anchored flagellin into Salmonella Gallinarum bacterial ghosts induces early immune responses and protection against fowl typhoid in young layer chickens. Vet Immunol Immunopathol. (2018) 199:61–9. doi: 10.1016/j.vetimm.2018.03.011
101. Lankester, F, Lugelo, A, Werling, D, Mnyambwa, N, Keyyu, J, Kazwala, R, et al. The efficacy of alcelaphine herpesvirus-1 (AlHV-1) immunization with the adjuvants Emulsigen® and the monomeric TLR5 ligand FliC in zebu cattle against AlHV-1 malignant catarrhal fever induced by experimental virus challenge. Vet Microbiol. (2016) 195:144–53. doi: 10.1016/j.vetmic.2016.09.019
102. Lu, H, Zhou, X, Wu, Z, Zhang, X, Zhu, L, Guo, X, et al. Comparison of the mucosal adjuvanticities of two toll-like receptor ligands for recombinant adenovirus-delivered African swine fever virus fusion antigens. Vet Immunol Immunopathol. (2021) 239:110307. doi: 10.1016/j.vetimm.2021.110307
103. Senevirathne, A, Hewawaduge, C, Park, J, Park, S, and Lee, J. Parenteral immunization of Salmonella Typhimurium ghosts with surface-displayed Escherichia coli flagellin enhancesTLR-5 mediated activation of immune responses that protect the chicken against Salmonella infection. Microb Pathog. (2020) 147:104252. doi: 10.1016/j.micpath.2020.104252
104. Yin, G, Qin, M, Liu, X, Suo, J, Tang, X, Tao, G, et al. An Eimeria vaccine candidate based on Eimeria tenella immune mapped protein 1 and the TLR-5 agonist Salmonella typhimurium FliC flagellin. Biochem Biophys Res Commun. (2013) 440:437–42. doi: 10.1016/j.bbrc.2013.09.088
105. St Paul, M, Brisbin, J, Barjesteh, N, Villaneueva, A, Parvizi, P, Read, L, et al. Avian influenza virus vaccines containing toll-like receptors 2 and 5 ligand adjuvants promote protective immune responses in chickens. Viral Immunol. (2014) 27:160–6. doi: 10.1089/vim.2013.0129
106. Wedlock, DN, Aldwell, FE, Vordermeier, HM, Hewinson, RG, and Buddle, BM. Protection against bovine tuberculosis induced by oral vaccination of cattle with Mycobacterium bovis BCG is not enhanced by co-administration of mycobacterial protein vaccines. Vet Immunol Immunopathol. (2011) 144:220–7. doi: 10.1016/j.vetimm.2011.09.005
107. Park, C, Lee, M, Baek, J, Cho, S, Hyun, B, You, S, et al. Intradermal co-inoculation of codon pair deoptimization (CPD)-attenuated chimeric porcine reproductive and respiratory syndrome virus (PRRSV) with toll like receptor (TLR) agonists enhanced the protective effects in pigs against heterologous challenge. Vet Microbiol. (2021) 256:109048. doi: 10.1016/j.vetmic.2021.109048
Keywords: toll-like receptor agonists, efficacy, safety, therapeutics, adjuvants, vaccines, infectious diseases, animals
Citation: Oboge H, Riitho V, Nyamai M, Omondi GP, Lacasta A, Githaka N, Nene V, Aboge G and Thumbi SM (2024) Safety and efficacy of toll-like receptor agonists as therapeutic agents and vaccine adjuvants for infectious diseases in animals: a systematic review. Front. Vet. Sci. 11:1428713. doi: 10.3389/fvets.2024.1428713
Edited by:
Michael Kogut, United States Department of Agriculture, United StatesReviewed by:
Sandra Barroso-Arévalo, VISAVET Health Surveillance Centre (UCM), SpainBasavaraj S. Mathapati, Indian Council of Medical Research (ICMR), India
Copyright © 2024 Oboge, Riitho, Nyamai, Omondi, Lacasta, Githaka, Nene, Aboge and Thumbi. This is an open-access article distributed under the terms of the Creative Commons Attribution License (CC BY). The use, distribution or reproduction in other forums is permitted, provided the original author(s) and the copyright owner(s) are credited and that the original publication in this journal is cited, in accordance with accepted academic practice. No use, distribution or reproduction is permitted which does not comply with these terms.
*Correspondence: Harriet Oboge, b2JvZ2VoYXJyaWV0QGdtYWlsLmNvbQ==