- 1Department of Biology, Faculty of Sciences, University of Hafr Al Batin, Hafr Al Batin, Saudi Arabia
- 2Department of Pharmacy Practice, Faculty of Pharmacy, King Abdulaziz University, Jeddah, Saudi Arabia
- 3Pharmacy Program, Batterjee Medical College, Jeddah, Saudi Arabia
- 4Faculty of Medicine, Al-Azhar University, Assiut, Egypt
- 5Faculty of Medicine, Alexandria University, Alexandria, Egypt
- 6Pharmacology Department, Faculty of Veterinary Medicine, Suez Canal University, Ismailia, Egypt
- 7Department of Medicine and Infectious Diseases, Faculty of Veterinary Medicine, Cairo University, Giza, Egypt
- 8Department of Chemistry, Preparatory Year Program, Batterjee Medical College, Jeddah, Saudi Arabia
- 9Pharmacy Practice Department, Pharmacy Program, Batterjee Medical College, Jeddah, Saudi Arabia
Chlorpyrifos (CPF) is one of the most commonly used organophosphosphate-based (OP) insecticides. Its wide use has led to higher morbidity and mortality, especially in developing countries. Moringa seed extracts (MSE) have shown neuroprotective activity, antioxidant, anti-inflammatory, and antibacterial features. The literature lacks data investigating the role of MSE against CPF-induced cerebral and ocular toxicity in mice. Therefore, we aim to investigate this concern. A total of 40 mature male Wistar Albino mice were randomly distributed to five groups. Initially, they underwent a one-week adaptation period, followed by a one-week treatment regimen. The groups included a control group that received saline, MSE 100 mg/kg, CPF 12 mg/kg, CPF-MSE 50 mg/kg, and CPF-MSE 100 mg/kg. After the treatment phase, analyses were conducted on serum, ocular, and cerebral tissues. MSE100 and CPF-MSE100 normalized the pro-inflammatory markers (interleukin-1β (IL-1β), interleukin-6 (IL-6), and tumor necrosis factor-alpha (TNF-α)) and AChE serum levels. CPF-MSE50 significantly enhanced these serum levels compared to CPF; however, it showed higher levels compared to the control. Moreover, the tissue analysis showed a significant decrease in oxidative stress (malondialdehyde (MDA) and nitric oxide (NO)) and an increase in antioxidant markers (glutathione (GSH), glutathione peroxidase (GSH-PX)), superoxide dismutase (SOD), and catalase (CAT) in the treated groups compared to CPF. Importantly, the significance of these effects was found to be dose-dependent, particularly evident in the CPF-MSE100 group. We conclude that MSE has a promising therapeutic effect in the cerebral and ocular tissues of CPF-intoxicated mice, providing a potential solution for OP public health issues.
1 Introduction
Organophosphosphate (OP)—based insecticides have been commonly employed in agricultural and household settings. Chlorpyrifos (CPF) [O, O-diethyl-o-(3, 5, 6-trichloro-2-pyridyl) phosphorothionate] is one of the most common OP-based insecticides, which is widely used due to its broad spectrum insecticidal activity (1–4). Its wide use has led to higher morbidity and mortality, especially in developing countries (5–7).
Chlorpyrifos affects many tissues, including neurological, ocular, cardiac, hepatic, and renal. It inhibits the acetylcholinesterase (AChE) enzyme in the neuronal synaptic clefts, which is essential for eliminating acetylcholine. AChE inhibition leads to acetylcholine (ACh) accumulation in those synaptic areas, causing toxic effects. Accumulated ACh causes neuronal, behavioral, and cognitive dysfunction, impaired memory, delayed neural development, and death (8–10). Moreover, CPF has been reported to interfere with the mitochondrial electron transport chain (ETC), increasing the production of reactive oxygen species (ROS). In addition, it interferes with the process important for eliminating ROS. CPF limits the antioxidant activities of certain enzymes, such as glutathione peroxidase (GPx), superoxide dismutase (SOD), and catalase (CAT). This in turn leads to oxidative stress, lipid peroxidation, and damage to the affected tissues (11, 12).
The use of plant extracts in medicine has been described for centuries (13). They provide a rich source of bioactive agents with notable effects (14–19). The Moringaceae family is a family of drought-resistant trees. Moringa oleifera (M. oleifera) is the most popular member of this family (15, 20, 21). This plant is characterized by containing various bioactive components in its seeds and leaves. It comprises several vitamins, minerals, and proteins such as vitamins A and C and iron, calcium, and potassium (22–25). M. oleifera extracts have shown neuroprotective activity, antioxidant, anti-inflammatory, and antibacterial features (25–28). Those features are attributed to containing polyphenols, terpenoids, and flavonoids, which regulate the antioxidant and detoxification activities (25, 26, 29).
Thereby M. oleifera seed extracts (MSE) have potential promising therapeutic effects against oxidative damage and OP toxicity (24). Although the known therapeutic effects of MSE, there is a shortage in the literature regarding its role in CPF intoxication. Hence, in this study, we aim to investigate the role of MSE on the CPF-intoxicated cerebral and ocular tissues of mice.
2 Methods
2.1 Chemical supplies
Pure moringa seed extract (MSE) and chlorpyrifos (CPF) were purchased from moringa sales at the National Research Centre (NRC), Egypt. The dried seeds were ground into fine powders and sieved. The enzyme-linked immunosorbent assay (ELISA) kits for pro-inflammatory cytokines, including interleukin-6 (IL-6), IL-1β, and tumor necrosis factor-alpha (TNF-α), were obtained from R&D (Mannheim, Germany) (Catalog # ARY005B). The AChE kit was obtained from Jiancheng Bioengineering Institute (Nanjing, China) (Catalog # A024). Kits for oxidative stress indicators and antioxidant status were purchased from Biodiagnostics Co. (Cairo, Egypt).
2.2 The study animals and design of the experiment
All procedures of our experiments were revised and approved by the Ethical Committee of the Faculty of Veterinary Medicine, Suez Canal University, Ismailia, Egypt (2023018).
A total of 40 mature male Wistar Albino mice (weighted 190 ± 10 g) were included in our experiments. They were obtained from the Egyptian Organization of Biological Products and Vaccines. Mice were cared for in laboratory chambers with suitable ventilation and temperature (25 ± 2°C) with a relatively humid atmosphere between 40 to 50% and under a 12-h cycle of light and dark. Additionally, they were supplied with enough food and water. For one week before the experiment, the mice were acclimated under these conditions.
After the one-week adaptation, mice were randomly distributed to one of five groups (eight mice in each group); the first group (control group) received saline as a control, group (2) received 100 mg/kg moringa seed extract (MSE), group (3) were administered 12 mg/kg chlorpyrifos (CPF) via oral gavage for 7 days, group (4) received 12 mg/kg CPF and 50 mg/kg MSE for 7 days and group (5) received 12 mg/kg CPF and 100 mg/kg MSE daily for 7 days.
2.3 Collection of blood samples and preparation of tissues
Blood samples were obtained from the retro-orbital venous plexus on the 15th day of the experiment and were centrifuged at 3000 g for 15 min. Then the obtained serum was kept at −20°C for further testing. All mice were sacrificed using isoflurane after blood sample collection. The cerebral and ocular tissue samples were obtained from all animals, cleaned of blood clots with saline and purified water then traditionally manually dissected. Then, all dissected samples were homogenized in 5–10 mL of ice-cold buffer per gram of tissue. After that, the tissues were centrifuged at 5000 rpm for 30 min. The formed supernatant was collected in tubes and stored at −80°C for spectrophotometry.
2.4 Assessment of AChE levels
The AChE levels were measured using colorimetric kits that use a specific enzymatic reaction to measure AChE levels in serum samples. Detailed assay procedures were strictly followed according to the manufacturer’s instructions. Specifically, the assays were guided by the Lowry assay (30) for protein concentration measurement.
2.5 Assessment of the pro-inflammatory mediators
We used the ELISA kits to assess the pro-inflammatory cytokines, including IL-6, IL-1β, and TNF-α, following the protocol provided by R&D (Mannheim, Germany).
2.6 Statues of tissue antioxidant and oxidative stress markers
Nitric oxide (NO) and malondialdehyde (MDA) were the markers for lipid peroxidation and were assessed using spectrophotometry techniques. NO was assessed as described by Green et al. (31) while MDA was estimated as described by Mihara et al. (32). Tissue anti-oxidant markers included glutathione (GSH), GSH peroxidase (GSH-Px), catalase (CAT), and superoxide dismutase (SOD) and were assessed using methods described by Beutler et al. (33), Paglia et al. (34), Aebi (35), and Nishikimi et al. (36) respectively.
2.7 Statistical analysis
Statistical analysis was done using the Statistical Package for social sciences (SPSS) 26.0. The Shapiro–Wilk test was employed to assess the normal distribution of the data. The statistical significance of the results was determined using the one-way analysis of variance (ANOVA) test. Tukey’s multiple range test was used for individual comparisons. Data were presented as mean and standard error (SE). Data were considered significant if the p-value was <0.05.
3 Results
3.1 Role of moringa seed extract on serum pro-inflammatory cytokines and acetylcholinesterase in CPF-intoxicated mice
The control group showed significantly lower levels than the CPF group regarding the IL-1β, IL-6, and TNF-α (33.7, 28.2, and 28.3%, respectively). Similarly, the MSE100 group showed significantly lower levels of IL-1β, IL-6, and TNF-α than the CPF group (31, 27.1, and 27.2%, respectively). Moreover, compared with the CPF group, the CPF-MSE50, and CPF-MSE100 showed significantly lower levels of IL-1β (63.2 and 37.5%, respectively), IL-6 (60 and 33.2%, respectively) and TNF-α (52.4 and 30.5%, respectively). However, the levels of the inflammatory markers in the MSE100 and CPF-MSE100 did not significantly differ from the control group. Additionally, the CPF-MSE50 showed significantly higher cytokine levels than the control group.
Regarding acetylcholinesterase (AChE), the control group showed significantly higher levels than the CPF group (3.3%). The CPF group also showed significantly lower levels than the other groups (control; 3.3%, MSE100; 3.4%, CPF-MSE50; 2.3%, and CPF-MSE100; 3%). However, there was no significant difference between MSE100, CPF-MSE100, and control groups (Table 1; Figure 1).
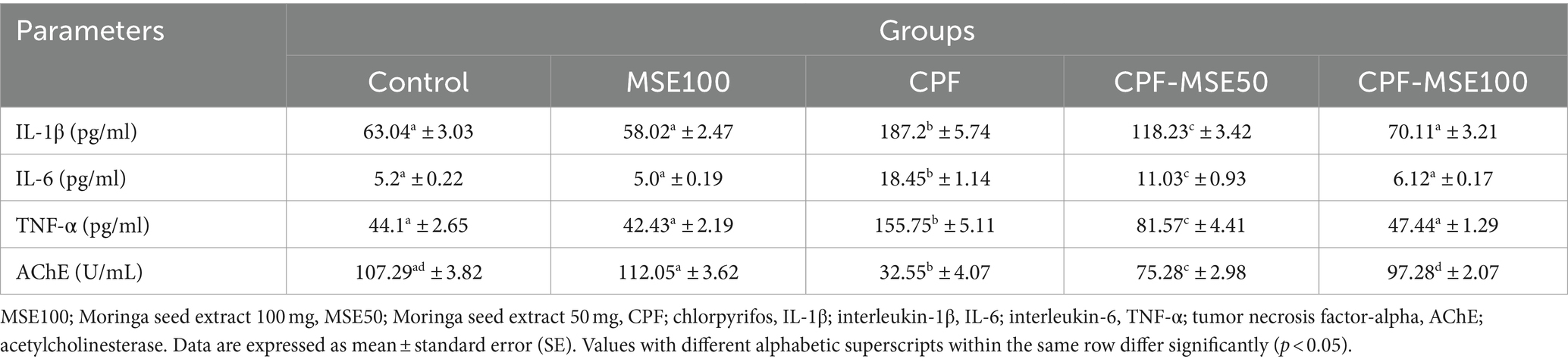
Table 1. Role of MSE on serum pro-inflammatory cytokines and acetylcholinesterase in CPF-intoxicated mice.
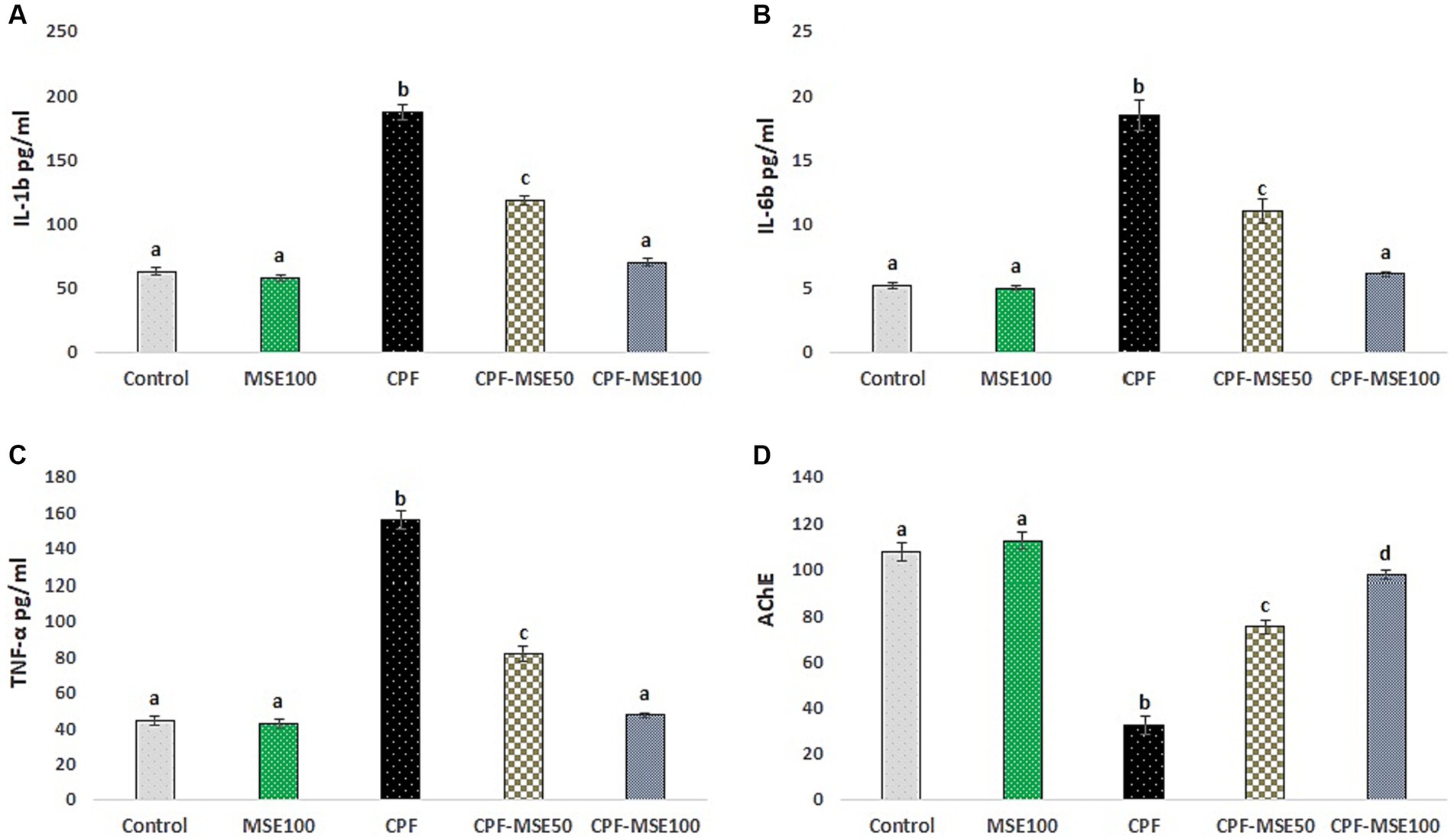
Figure 1. Effect of MSE on serum levels in CPF-intoxicated mice. This figure depicts the impact of Moringa seed extract (MSE) on serum levels in mice intoxicated with Chlorpyrifos (CPF). (A) refers to interleukin-1β (IL-1β); (B) refers to interleukin-6 (IL-6); (C) refers to tumor necrosis factor-alpha (TNF-α); (D) refers to acetylcholinesterase (AChE). Serum levels of pro-inflammatory markers (IL-1β, IL-6, TNF-α, and AChE) were assessed in five experimental groups: Control negative, MSE 100 mg/kg, CPF 12 mg/kg, CPF-MSE 50 mg/kg, and CPF-MSE 100 mg/kg. Values having different alphabetic superscripts are significantly different (p < 0.05).
3.2 Effect of MSE on ocular tissue oxidative stress and antioxidant levels in CPF-intoxicated mice
Compared with the CPF group, the MSE100, CPF-MSE50, and CPF-MSE100 showed significantly lower levels of MDA (46.5, 72.1, and 52.1%, respectively) and NO (53.3, 77.1, and 56.5%). On the other hand, the MSE100, CPF-MSE50, and CPF-MSE100 showed significantly higher levels of GSH (50.8, 64, 53%), GSH-PX (47, 64.3, and 50.2%, respectively), SOD (31.7, 55.4, and 34.7%, respectively), and CAT (40.1%, 72.4, and 46.3%, respectively) when compared with CPF group (Table 2; Figure 2). However, MSE100, CPF-MSE100, and the control group significantly differ regarding all oxidative stress and antioxidant parameters except for CAT. The CAT level was significantly higher in the control group compared with the CPF-MSE100 group.
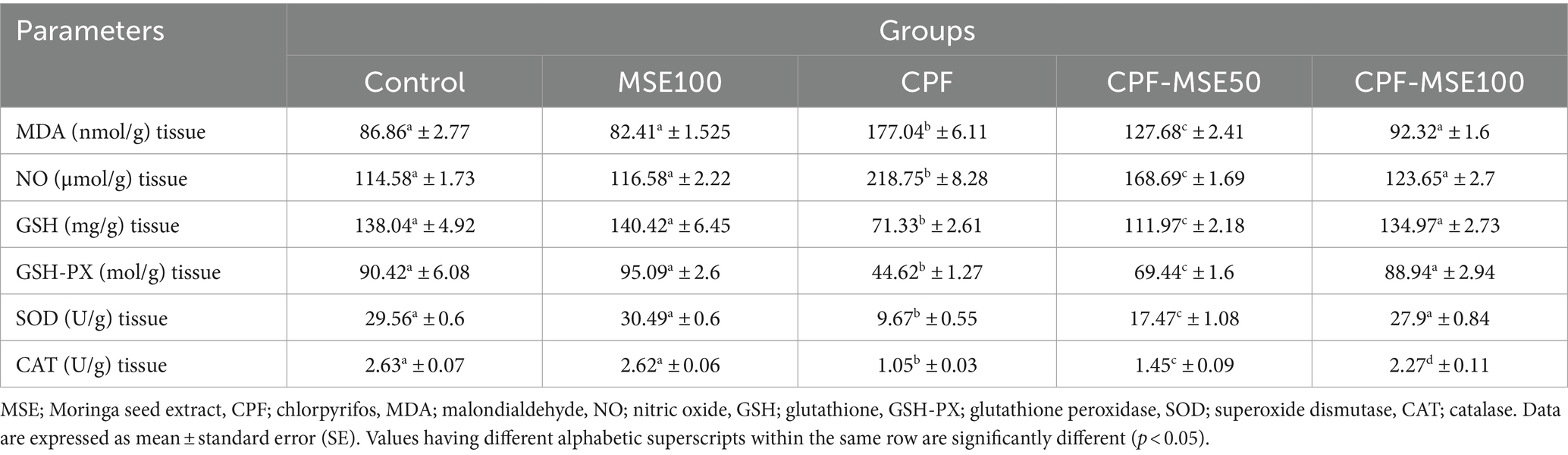
Table 2. Effect of MSE on ocular tissue oxidative stress and antioxidant levels in CPF-intoxicated mice.
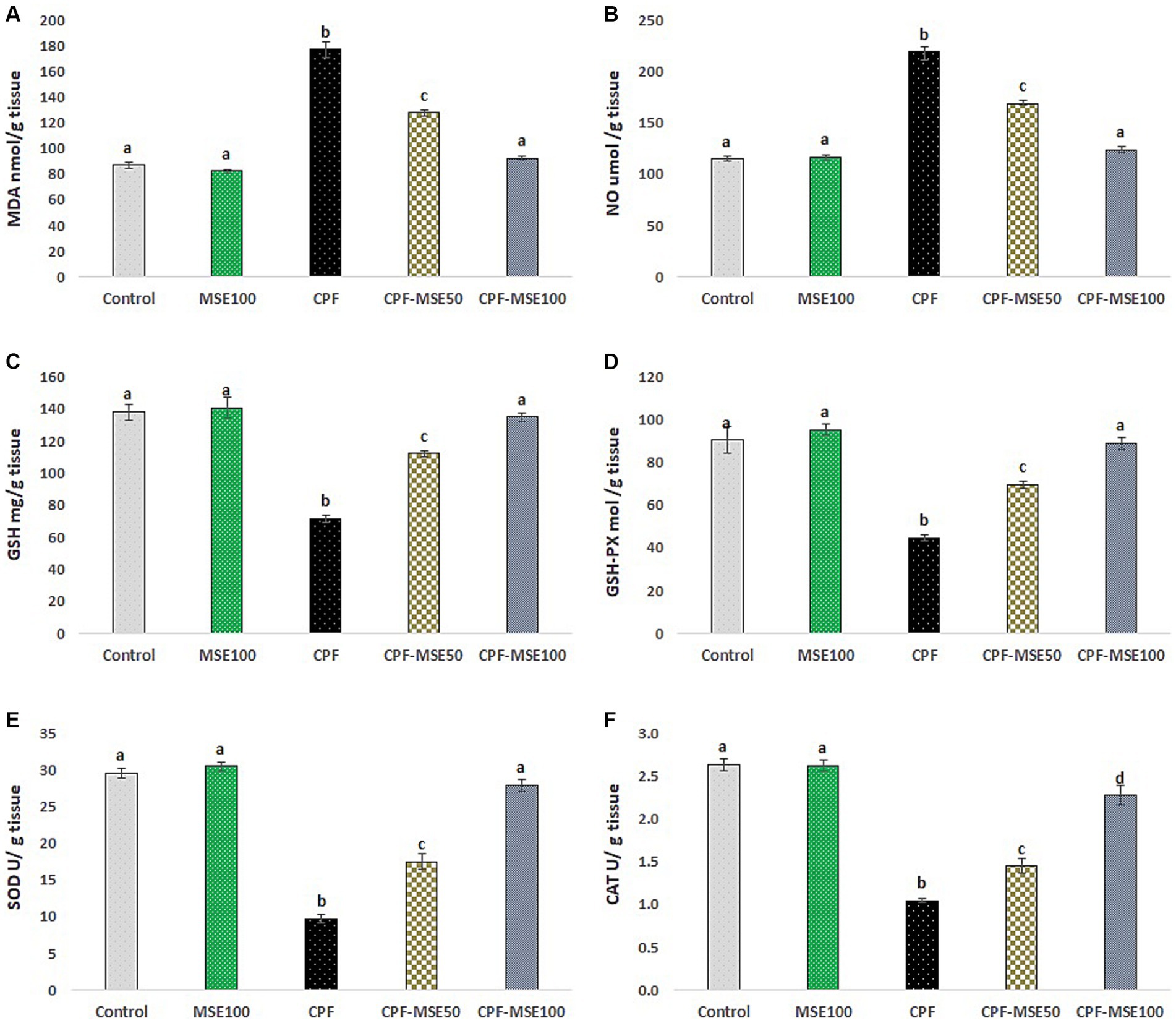
Figure 2. Effect of MSE on ocular tissue oxidative stress and antioxidants levels in CPF-intoxicated mice. (A) refers to malondialdehyde (MDA); (B) refers to nitric oxide (NO); (C) refers to glutathione; (D) refers to glutathione peroxidase (GSH-PX); (E) refers to superoxide dismutase (SOD), and (F) refers to catalase (CAT). This figure presents the impact of Moringa Seed Extract (MSE) on oxidative stress (MDA and NO) and antioxidant levels (GSH-PX, SOD and CAT) in the ocular tissues of mice exposed to Chlorpyrifos (CPF). Values having different alphabetic superscripts are significantly different (p < 0.05).
3.3 Effect of MSE on cerebral tissue oxidative stress and antioxidant levels in CPF-intoxicated mice
Compared with the CPF group, the MSE100, CPF-MSE50, and CPF-MSE100 groups showed significantly lower levels of MDA (40.3, 75, and 44%, respectively), and NO (44.6, 76, and 53% respectively). On the other hand, the MSE100, CPF-MSE50, and CPF-MSE100 showed significantly higher levels of GSH (45.3, 53.3, and 43%, respectively), GSH-PX (31.6, 45.2, and 37% respectively), SOD (33.7, 61, and 39% respectively), and CAT (48.1, 70.1, and 54.2% respectively) compared with CPF group (Table 3; Figure 3). On the other hand, MSE100 and CPF-MSE100 did not significantly differ regarding all oxidative stress markers and antioxidants. In addition, MSE100 and CPF-MSE100 showed significantly better results compared with CPF-MSE 50 (Figure 4).
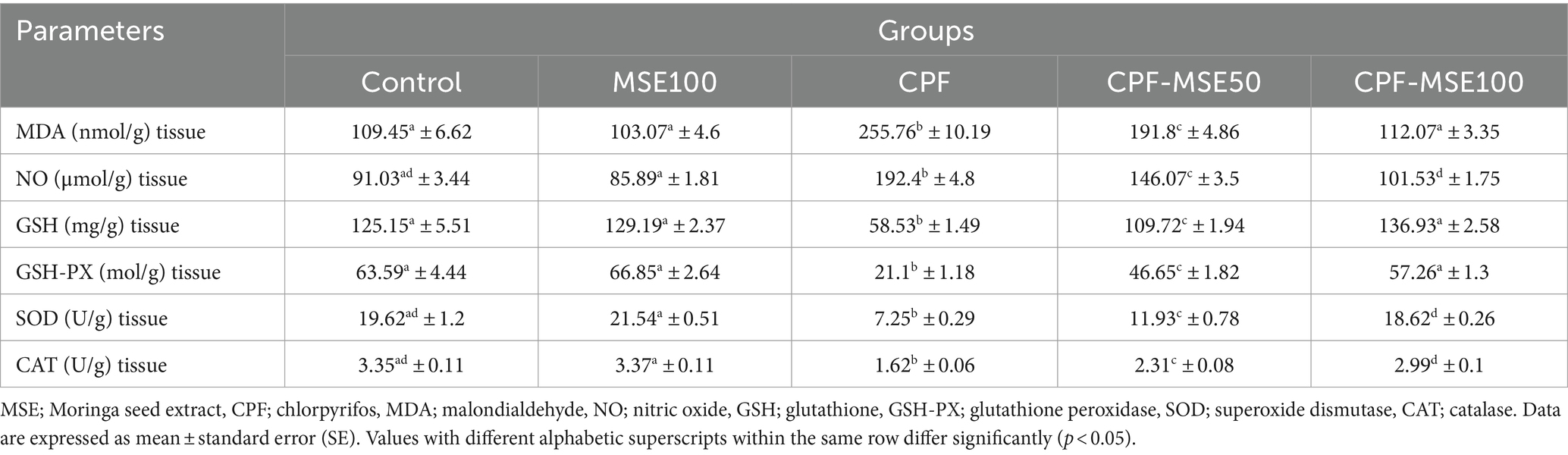
Table 3. Effect of MSE on cerebral tissue oxidative stress and antioxidant levels in CPF-intoxicated mice.
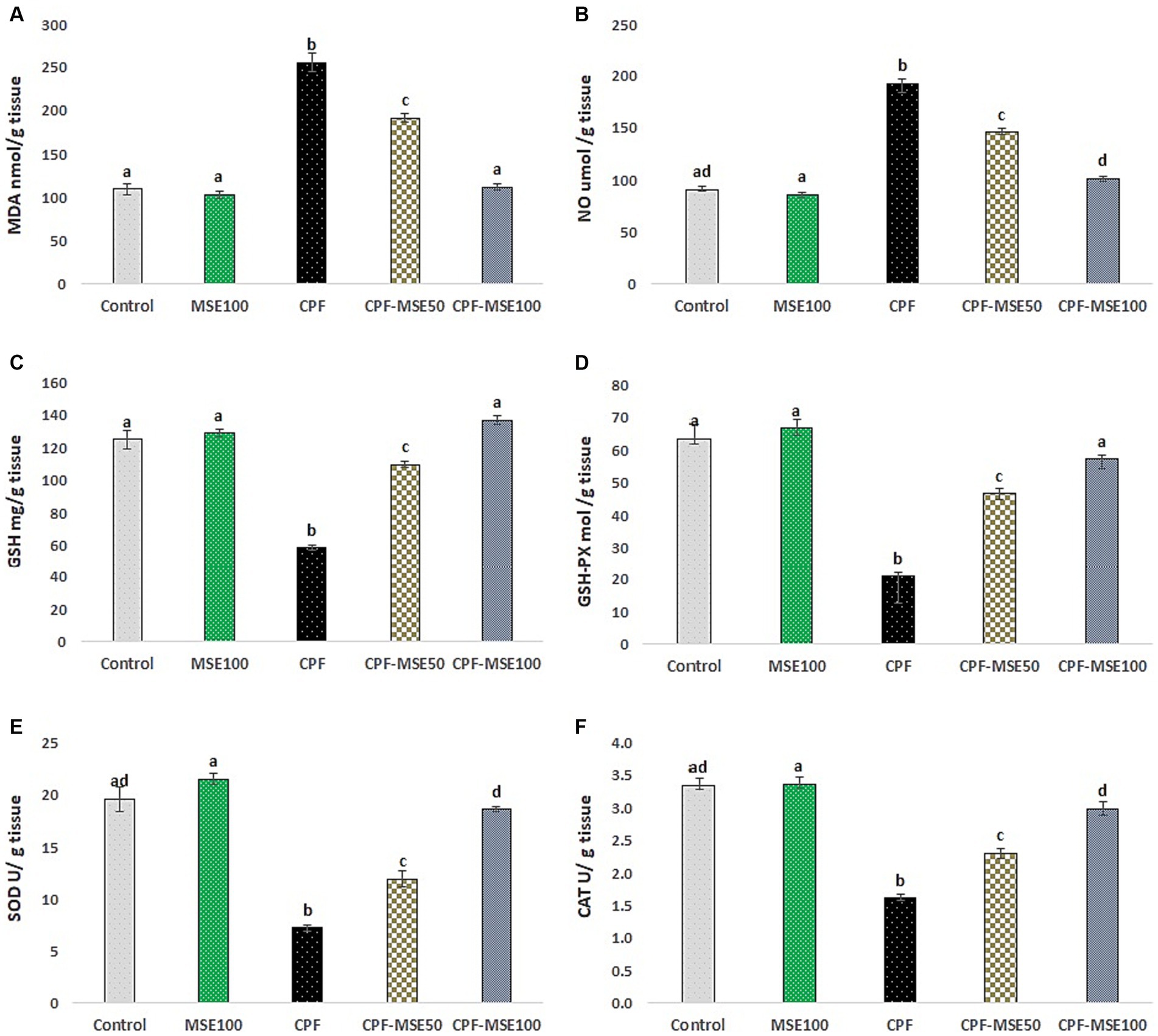
Figure 3. Effect of MSE on cerebral tissue oxidative stress and antioxidant levels in CPF-intoxicated mice. (A) refers to malondialdehyde (MDA); (B) refers to nitric oxide (NO); (C) refers to glutathione; (D) refers to glutathione peroxidase (GSH-PX); (E) refers to superoxide dismutase (SOD), and (F) refers to catalase (CAT). This figure presents the impact of Moringa Seed Extract (MSE) on oxidative stress (MDA and NO) and antioxidant levels (GSH, GSH-PX, SOD and CAT) in the cerebral tissue of mice exposed to Chlorpyrifos (CPF). Values having different alphabetic superscripts are significantly different (p < 0.05).
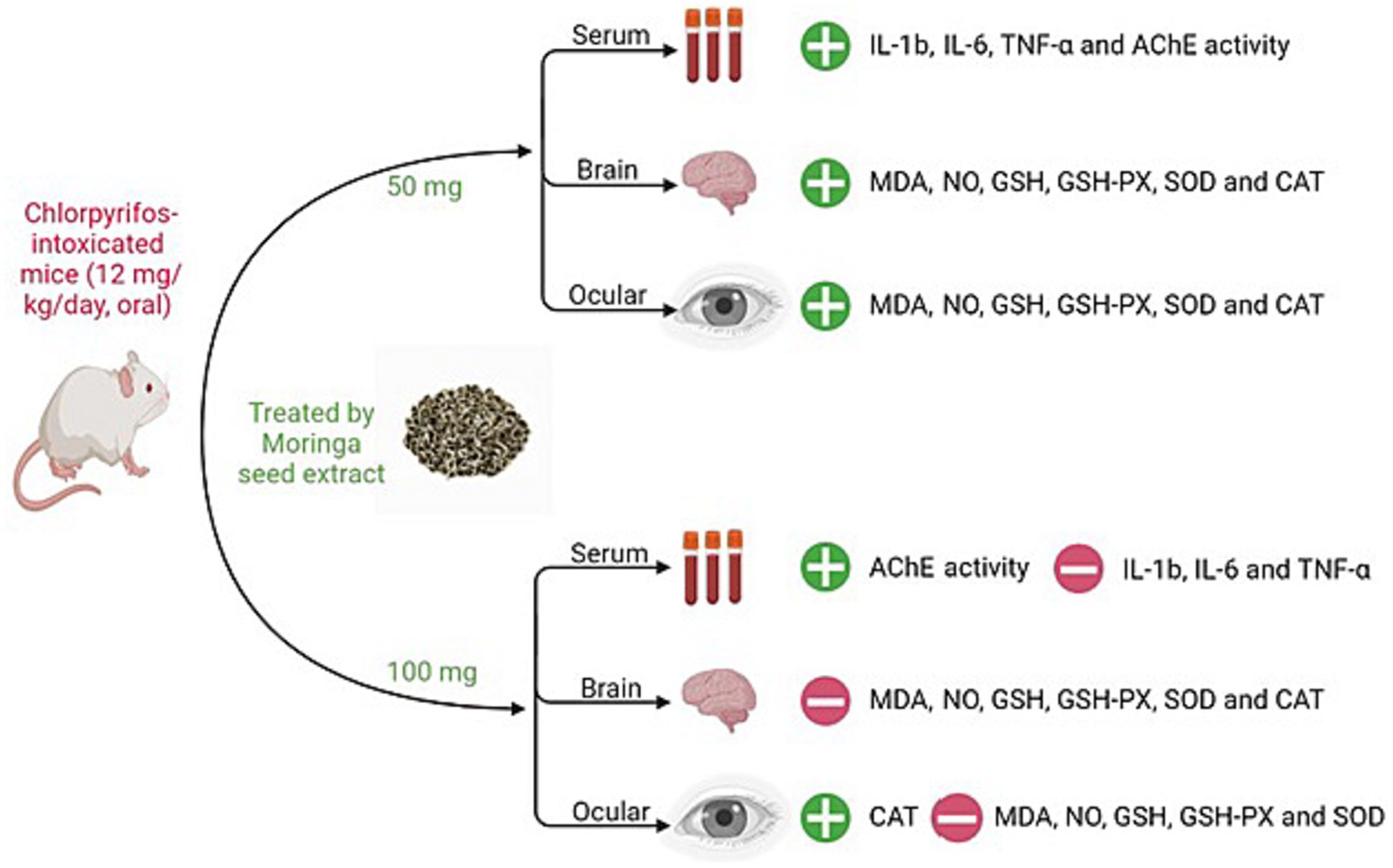
Figure 4. Summary of MSE effect on cerebral and ocular toxicity induced by CPF in mice. IL-1β; interleukin-1β, IL-6; interleukin-6, TNF-α; tumor necrosis factor-alpha, AChE; acetylcholinesterase, MDA; malondialdehyde, NO; nitric oxide, GSH; glutathione, GSH-PX; glutathione peroxidase, SOD; superoxide dismutase, CAT; catalase.
4 Discussion
In this study, we aimed to investigate the protective role of MSE against CPF toxicity. We found that MSE has a promising therapeutic effect in the cerebral and ocular tissues of CPF-intoxicated mice. Compared with the CPF group, the MSE 100, CPF-MSE50, and CPF-MSE100 groups significantly elevated the AChE levels. However, the MSE100 and CPF-MSE100 AChE levels did not significantly differ from the values of the control group. But they were still significantly better than the CPF group. Moreover, MSE, MSE 100, CPF-MSE50, and CPF-MSE100 groups showed a significant reduction in the serum levels of the inflammatory mediators compared to the CPF group. In this study, MSE decreased the oxidative stress markers (MDA and NO) levels and elevated the levels of antioxidant agents (GSH, GSH-PX, SOD, and CAT) in the ocular and cerebral tissues of mice. MSE50, MSE100, and CPF-MSE 100 groups significantly enhanced oxidative stress compared with the CPF group. However, MSE100 and CPF-MSE100 showed significantly better results compared with CPF-MSE 50.
Notably, the values of oxidative stress markers were higher in the cerebral tissue compared with the ocular tissue. In addition, cerebral tissue showed lower levels of antioxidants, indicating their consumption by the cerebral tissue more than the ocular tissue. This points to the significant oxidative stress the brain tissue endures. Cerebral tissue is characterized by higher liability to oxidative damage induced by ROS (8). This is attributed to higher energy demand and lower anti-oxidant levels such as SOD and CAT, in addition to, higher content of polyunsaturated fatty acids (PUFA), proteins, and nucleic acids (24, 37).
Given the findings indicating decreased serum levels of inflammation and markers of oxidative stress, it is important to mention the fundamental mechanisms through which MSE exerts its protective effects. MSE’s compounds demonstrate anti-inflammatory effects by inhibiting pro-inflammatory enzymes such as cyclooxygenase and lipoxygenase, as observed with quercetin and kaempferol. Additionally, MSE regulates cytokine production by modulating signaling pathways like nuclear factor-kappa B (NF-kappa B), thereby decreasing the production of pro-inflammatory cytokines such as TNF-α and IL-1β. Furthermore, MSE contains flavonoids and polyphenols known for their antioxidant properties, which contribute to reducing oxidative stress and inflammation (38, 39).
Similarly, it has been reported that chronic OP insecticide exposures lead to chronic ocular damage through OP-induced retinal apoptosis (40–44). Retina easily suffers from CPF-induced damage. Normally, it is subjected to severe oxidative stress resulting from the high metabolic activities stimulated by light exposure. Moreover, retinal oxidative stress has been related to a variety of retinal pathological conditions. However, the effect of CPF on eye structures other than the retina needs further investigation (44, 45).
CPF as one of the OP compounds interferes with macromolecule production such as DNA, RNA, and proteins. In addition, it impairs normal neuronal development and interferes with the normal process of neurotransmission cascade through the affection of several enzymes important for signal transduction (8, 46, 47). In addition, CPF inhibits the AChE enzyme and has been linked to oxidative damage of human and animal tissues (9–12, 48).
Oxidative tissue damage is attributed to the accumulation of reactive oxygen species (ROS); the metabolic products of the energy production cascade. Under normal circumstances, it can be controlled by the scavenger action of antioxidant enzymes like GSH, GSH-PX, SOD, and CAT. The imbalance between ROS and anti-oxidants results in the accumulation of harmful ROS leading to significant tissue damage and cellular apoptosis (49, 50).
Our findings point to the role of oxidative damage and reactive oxygen species (ROS) in cerebral and ocular tissues of CPF-intoxicated mice. This is evidenced by the significantly higher levels of oxidative stress markers and the decreased level of the antioxidant agents in the CPF-intoxicated groups. As a result of ROS activity, the antioxidant enzymes were significantly consumed with significantly higher values of MDA and NO in the CPF group compared to the control, MSE, and CPF-MSE 100 groups.
Our results also indicate a probable dose-dependent pattern of MSE. This is evidenced by the significant reduction of the inflammatory mediators, oxidative stress marker, and the significant elevation of AChE, and the antioxidant agents in the MSE100 and CPF-MSE100 compared with the CPF-MSE50 group. Additionally, MSE100 and CPF-MSE100 showed similarity to the baseline values of the control group with no statistically significant difference. This points to the significant role of MSE that achieves tissue homeostasis even in CPF-intoxicated cerebral and ocular tissues of mice. Furthermore, our results confirm what has been previously reported in the literature regarding its anti-oxidant (51–55), anti-inflammatory (24, 56, 57), and AChE-enhancing features (24, 28, 58). However, the latter is debatable.
The therapeutic properties of MSE are ascribed to the potent constituents of its seeds and leaves, which include flavonoids, phenolics, carotenoids, and vitamins A and C, alongside essential minerals such as iron, calcium, and potassium (15, 23, 25, 59, 60). Additionally, MSE is rich in bioactive phytochemicals like niazirin, niazimicin, β-sitosterol, and 4(alpha-L-rhamnosyloxy) benzyl isothiocyanate, each contributing to its therapeutic potential (61). For instance, niazirin is recognized for its strong antioxidant activity (62); niazimicin has neuroprotective features (63); β-sitosterol exhibits anti-adipogenic activities (64); and the α rhamnosyloxy has been reported to attenuate spinal cord injury-associated damage (65). However, the exact mechanism of its protective role has not been established yet. In previous studies, the anti-oxidant power of MSE was assessed by defining the total amounts of phenols, flavonoids, and tannin contents in addition to defining the scavenging and reducing powers of the diphenyl picrylhydrazyl (DPPH), azinobis-ethylbenzothiazoline-sulfonic acid (ABTS), and nitric oxide (NO) (53). The DPPH, ABTS, and NO scavenging activities were attributed to the phenolic and flavonoid contents of the moringa seed water extracts (53, 66).
Considering our results alongside prior research, MSE exhibits several protective and therapeutic effects against OP toxicity through its antioxidant, anti-inflammatory, and AChE inhibitory actions. These findings indicate a possible avenue for addressing OP-related public health concerns. Consequently, we recommend further exploration into the protective potential of MSE in human populations.
5 Conclusion
Our study findings highlight the promising therapeutic effects of MSE in ameliorating CPF toxicity in mice. MSE administration resulted in significant enhancements in AChE activity and antioxidant functions while concurrently reducing levels of inflammatory mediators and oxidative stress markers in both cerebral and ocular tissues of CPF-intoxicated mice.
In conclusion, our study highlights MSE as a promising natural intervention for mitigating CPF toxicity, offering insights into its therapeutic mechanisms and suggesting avenues for future research. By embracing these insights and addressing the identified research gaps, we can advance our understanding of MSE’s potential in alleviating pesticide-induced toxicity and contribute to the development of effective therapeutic interventions based on natural compounds like MSE.
As a limitation of the current study, specific molecular pathways should be deeply involved in its preventive and therapeutic effects, and assess its safety and efficacy in clinical settings.
Data availability statement
The original contributions presented in the study are included in the article/supplementary material, further inquiries can be directed to the corresponding author.
Ethics statement
The animal study was approved by all procedures of our experiments were revised and approved by the Ethical Committee of the Faculty of Veterinary Medicine, Suez Canal University, Ismailia, Egypt (2023018). The study was conducted in accordance with the local legislation and institutional requirements.
Author contributions
IA: Formal analysis, Writing – review & editing, Conceptualization, Investigation, Methodology, Project administration, Software, Validation, Writing – original draft, Funding acquisition, Supervision, Visualization. AA: Conceptualization, Formal analysis, Funding acquisition, Investigation, Methodology, Project administration, Supervision, Validation, Visualization, Writing – review & editing. MZ: Formal analysis, Investigation, Methodology, Visualization, Data curation, Software, Writing – original draft. AE: Data curation, Formal analysis, Investigation, Methodology, Software, Visualization, Writing – original draft, Project administration. A-FA-F: Data curation, Methodology, Software, Visualization, Writing – original draft, Validation. MK: Data curation, Methodology, Software, Visualization, Writing – original draft. MA: Software, Writing – original draft, Conceptualization, Investigation, Project administration, Validation. NG: Conceptualization, Formal analysis, Investigation, Methodology, Project administration, Supervision, Validation, Visualization, Writing – review & editing.
Funding
The author(s) declare that financial support was received for the research, authorship, and/or publication of this article.
Acknowledgments
The authors extend their appreciation to the Deanship of Scientific Research, University of Hafr Al Batin for funding this work through the research group project no 0066-1443-S.
Conflict of interest
The authors declare that the research was conducted in the absence of any commercial or financial relationships that could be construed as a potential conflict of interest.
Publisher’s note
All claims expressed in this article are solely those of the authors and do not necessarily represent those of their affiliated organizations, or those of the publisher, the editors and the reviewers. Any product that may be evaluated in this article, or claim that may be made by its manufacturer, is not guaranteed or endorsed by the publisher.
References
1. Abdel-Daim, MM, Dawood, MAO, Elbadawy, M, Aleya, L, and Alkahtani, S. Spirulina platensis reduced oxidative damage induced by Chlorpyrifos toxicity in Nile Tilapia (Oreochromis niloticus). Animals (Basel). (2020) 10:473. doi: 10.3390/ani10030473
2. Atsdr. Agency for Toxic Substances and Disease Registry. Toxicological profile for chlorpyrifos. Atlanta, Ga: U.S., Department of Health and Human Services, Public Health Service (1997).
3. Clark, S. Chlorpyrifos In: SJ Enna and DB Bylund, editors. xPharm: The comprehensive pharmacology reference. New York: Elsevier (2007)
4. Koshlukova, SE, and Reed, NR. Chlorpyrifos In: P Wexler, editor. Encyclopedia of toxicology (third edition). Oxford: Academic Press (2014)
5. Alkahtane, AA, Ghanem, E, Bungau, SG, Alarifi, S, Ali, D, Albasher, G, et al. Carnosic acid alleviates chlorpyrifos-induced oxidative stress and inflammation in mice cerebral and ocular tissues. Environ Sci Pollut Res Int. (2020) 27:11663–70. doi: 10.1007/s11356-020-07736-1
6. Eddleston, M, Karalliedde, L, Buckley, N, Fernando, R, Hutchinson, G, Isbister, G, et al. Pesticide poisoning in the developing world—a minimum pesticides list. Lancet. (2002) 360:1163–7. doi: 10.1016/S0140-6736(02)11204-9
7. Mali, H, Shah, C, Raghunandan, BH, Prajapati, AS, Patel, DH, Trivedi, U, et al. Organophosphate pesticides an emerging environmental contaminant: pollution, toxicity, bioremediation progress, and remaining challenges. J Environ Sci. (2023) 127:234–50. doi: 10.1016/j.jes.2022.04.023
8. Aboubakr, M, Elshafae, SM, Abdelhiee, EY, Fadl, SE, Soliman, A, Abdelkader, A, et al. Antioxidant and anti-inflammatory potential of Thymoquinone and lycopene mitigate the Chlorpyrifos-induced toxic neuropathy. Pharmaceuticals (Basel). (2021) 14:940. doi: 10.3390/ph14090940
9. Meyer, A, Seidler, FJ, and Slotkin, TA. Developmental effects of chlorpyrifos extend beyond neurotoxicity: critical periods for immediate and delayed-onset effects on cardiac and hepatic cell signaling. Environ Health Perspect. (2004) 112:170–8. doi: 10.1289/ehp.6690
10. Qiao, D, Seidler, FJ, Padilla, S, and Slotkin, TA. Developmental neurotoxicity of chlorpyrifos: what is the vulnerable period? Environ Health Perspect. (2002) 110:1097–103. doi: 10.1289/ehp.021101097
11. Singh, N, Lawana, V, Luo, J, Phong, P, Abdalla, A, Palanisamy, B, et al. Organophosphate pesticide chlorpyrifos impairs Stat1 signaling to induce dopaminergic neurotoxicity: implications for mitochondria mediated oxidative stress signaling events. Neurobiol Dis. (2018) 117:82–113. doi: 10.1016/j.nbd.2018.05.019
12. Turton, N, Heaton, RA, Ismail, F, Roberts, S, Nelder, S, Phillips, S, et al. The effect of organophosphate exposure on neuronal cell coenzyme Q(10) status. Neurochem Res. (2021) 46:131–9. doi: 10.1007/s11064-020-03033-y
13. El-Saber Batiha, G, Magdy Beshbishy, A, El-Mleeh, A, Abdel-Daim, M, and Prasad Devkota, H. Traditional uses, bioactive chemical constituents, and pharmacological and toxicological activities of Glycyrrhiza glabra L. (Fabaceae). Biomolecules. (2020) 10:352. doi: 10.3390/biom10030352
14. Abdel-Daim, MM, Eissa, IAM, Abdeen, A, Abdel-Latif, HMR, Ismail, M, Dawood, MAO, et al. Lycopene and resveratrol ameliorate zinc oxide nanoparticles-induced oxidative stress in Nile tilapia, Oreochromis niloticus. Environ Toxicol Pharmacol. (2019) 69:44–50. doi: 10.1016/j.etap.2019.03.016
15. Abdel-Latif, HMR, Abdel-Daim, MM, Shukry, M, Nowosad, J, and Kucharczyk, D. Benefits and applications of Moringa oleifera as a plant protein source in Aquafeed: a review. Aquaculture. (2022) 547:737369. doi: 10.1016/j.aquaculture.2021.737369
16. El-Shemy, HA, Aboul-Enein, AM, Aboul-Enein, KM, and Fujita, K. Willow leaves' extracts contain anti-tumor agents effective against three cell types. PLoS One. (2007) 2:e178. doi: 10.1371/journal.pone.0000178
17. Grewal, AK, Singh, TG, Sharma, D, Sharma, V, Singh, M, Rahman, MH, et al. Mechanistic insights and perspectives involved in neuroprotective action of quercetin. Biomed Pharmacother. (2021) 140:111729. doi: 10.1016/j.biopha.2021.111729
18. Kabir, MT, Rahman, MH, Shah, M, Jamiruddin, MR, Basak, D, Al-Harrasi, A, et al. Therapeutic promise of carotenoids as antioxidants and anti-inflammatory agents in neurodegenerative disorders. Biomed Pharmacother. (2022) 146:112610. doi: 10.1016/j.biopha.2021.112610
19. Sermakkani, M, and Thangapandian, V. Gc-Ms analysis of Cassia italica leaf methanol extract. Asian J Pharm Clin Res. (2012) 5:90–4. Available at: https://innovareacademics.in/journal/ajpcr/Vol5Issue2/840.pdf
20. Abdel-Daim, MM, Alkahtani, S, Almeer, R, and Albasher, G. Alleviation of lead acetate-induced nephrotoxicity by Moringa oleifera extract in rats: highlighting the antioxidant, anti-inflammatory, and anti-apoptotic activities. Environ Sci Pollut Res Int. (2020) 27:33723–31. doi: 10.1007/s11356-020-09643-x
21. Kuete, V. Chapter 22—Moringa oleifera In: V Kuete, editor. Medicinal spices and vegetables from Africa. San Francisco, CA, USA: Academic Press (2017).
22. Abdel-Daim, MM, Khalil, SR, Awad, A, Abu Zeid, EH, El-Aziz, RA, and El-Serehy, HA. Ethanolic extract of Moringa oleifera leaves influences Nf-κB signaling pathway to restore kidney tissue from cobalt-mediated oxidative injury and inflammation in rats. Nutrients. (2020) 12:1031. doi: 10.3390/nu12041031
23. Bennett, RN, Mellon, FA, Foidl, N, Pratt, JH, Dupont, MS, Perkins, L, et al. Profiling glucosinolates and phenolics in vegetative and reproductive tissues of the multi-purpose trees Moringa oleifera L. (horseradish tree) and Moringa stenopetala L. J Agric Food Chem. (2003) 51:3546–53. doi: 10.1021/jf0211480
24. Kandeil, MA, Mohammed, ET, Hashem, KS, Aleya, L, and Abdel-Daim, MM. Moringa seed extract alleviates titanium oxide nanoparticles (TiO2-Nps)-induced cerebral oxidative damage, and increases cerebral mitochondrial viability. Environ Sci Pollut Res Int. (2020) 27:19169–84. doi: 10.1007/s11356-019-05514-2
25. Mbikay, M. Therapeutic potential of Moringa oleifera leaves in chronic hyperglycemia and dyslipidemia: a review. Front Pharmacol. (2012) 3:24. doi: 10.3389/fphar.2012.00024
26. Arora, S, and Arora, S. Nutritional significance and therapeutic potential of Moringa oleifera: the wonder plant. J Food Biochem. (2021) 45:e13933. doi: 10.1111/jfbc.13933
27. Fard, MT, Arulselvan, P, Karthivashan, G, Adam, SK, and Fakurazi, S. Bioactive extract from Moringa oleifera inhibits the pro-inflammatory mediators in lipopolysaccharide stimulated macrophages. Pharmacogn Mag. (2015) 11:S556–63. doi: 10.4103/0973-1296.172961
28. Zhou, J, Yang, WS, Suo, DQ, Li, Y, Peng, L, Xu, LX, et al. Moringa oleifera seed extract alleviates scopolamine-induced learning and memory impairment in mice. Front Pharmacol. (2018) 9:389. doi: 10.3389/fphar.2018.00389
29. El-Seedi, HR, El-Shabasy, RM, Khalifa, SA, Saeed, A, Shah, A, Shah, R, et al. Metal nanoparticles fabricated by green chemistry using natural extracts: biosynthesis, mechanisms, and applications. RSC Adv. (2019) 9:24539–59. doi: 10.1039/C9RA02225B
30. Lowry, OH. Protein measurement with the Folin phenol reagent. J Biol Chem. (1951) 193:265–75. doi: 10.1016/S0021-9258(19)52451-6
31. Green, LC, Wagner, DA, Glogowski, J, Skipper, PL, Wishnok, JS, and Tannenbaum, SR. Analysis of nitrate, nitrite, and [15N]nitrate in biological fluids. Anal Biochem. (1982) 126:131–8. doi: 10.1016/0003-2697(82)90118-X
32. Mihara, M, and Uchiyama, M. Determination of malonaldehyde precursor in tissues by thiobarbituric acid test. Anal Biochem. (1978) 86:271–8. doi: 10.1016/0003-2697(78)90342-1
33. Beutler, E, Duron, O, and Kelly, BM. Improved method for the determination of blood glutathione. J Lab Clin Med. (1963) 61:882–8.
34. Paglia, DE, and Valentine, WN. Studies on the quantitative and qualitative characterization of erythrocyte glutathione peroxidase. J Lab Clin Med. (1967) 70:158–69.
35. Aebi, H. Catalase in vitro. Methods Enzymol. (1984) 105:121–6. doi: 10.1016/S0076-6879(84)05016-3
36. Nishikimi, M, Appaji, N, and Yagi, K. The occurrence of superoxide anion in the reaction of reduced phenazine methosulfate and molecular oxygen. Biochem Biophys Res Commun. (1972) 46:849–54. doi: 10.1016/S0006-291X(72)80218-3
37. Huerta-García, E, Pérez-Arizti, JA, Márquez-Ramírez, SG, Delgado-Buenrostro, NL, Chirino, YI, Iglesias, GG, et al. Titanium dioxide nanoparticles induce strong oxidative stress and mitochondrial damage in glial cells. Free Radic Biol Med. (2014) 73:84–94. doi: 10.1016/j.freeradbiomed.2014.04.026
38. ChiȘ, A, Noubissi, PA, Pop, O-L, MureȘan, CI, Fokam Tagne, MA, Kamgang, R, et al. Bioactive compounds in Moringa oleifera: mechanisms of action, focus on Their Anti-Inflammatory Properties. Plants. (2024) 13:20. doi: 10.3390/plants13010020
39. Flora, SJ, and Pachauri, V. Moringa (Moringa oleifera) seed extract and the prevention of oxidative stress In: Nuts and seeds in health and disease prevention. eds. V. R. Preedy, R. R. Watson, and V. B. Patel. Cambridge, MA, USA: Elsevier (2011).
40. Boyes, WK, Padilla, S, Tandon, P, and Barone, S Jr. Effects of organophosphates on the visual system of rats. J Appl Toxicol. (1994) 14:135–43. doi: 10.1002/jat.2550140216
41. Dementi, B. Ocular effects of organophosphates: a historical perspective of Saku disease. J Appl Toxicol. (1994) 14:119–29. doi: 10.1002/jat.2550140214
42. Geller, AM, Sutton, LD, Marshall, RS, Hunter, DL, Madden, V, and Peiffer, RL. Repeated spike exposure to the insecticide chlorpyrifos interferes with the recovery of visual sensitivity in rats. Doc Ophthalmol. (2005) 110:79–90. doi: 10.1007/s10633-005-7347-8
43. Tandon, P, Padilla, S, Barone, S, Pope, C, and Tilson, H. Fenthion produces a persistent decrease in muscarinic receptor function in the adult rat retina. Toxicol Appl Pharmacol. (1994) 125:271–80. doi: 10.1006/taap.1994.1073
44. Yu, F, Wang, Z, Ju, B, Wang, Y, Wang, J, and Bai, D. Apoptotic effect of organophosphorus insecticide chlorpyrifos on mouse retina in vivo via oxidative stress and protection of combination of vitamins C and E. Exp Toxicol Pathol. (2008) 59:415–23. doi: 10.1016/j.etp.2007.11.007
45. Yu, L, Kelly, U, Ebright, JN, Malek, G, Saloupis, P, Rickman, DW, et al. Oxidative stress-induced expression and modulation of phosphatase of regenerating Liver-1 (Prl-1) in mammalian retina. Biochim Biophys Acta. (2007) 1773:1473–82. doi: 10.1016/j.bbamcr.2007.06.005
46. Eaton, DL, Daroff, RB, Autrup, H, Bridges, J, Buffler, P, Costa, LG, et al. Review of the toxicology of chlorpyrifos with an emphasis on human exposure and neurodevelopment. Crit Rev Toxicol. (2008) 38:1–125. doi: 10.1080/10408440802272158
47. Ubaid ur Rahman, H, Asghar, W, Nazir, W, Sandhu, MA, Ahmed, A, and Khalid, N. A comprehensive review on chlorpyrifos toxicity with special reference to endocrine disruption: evidence of mechanisms, exposures and mitigation strategies. Sci Total Environ. (2021) 755:142649. doi: 10.1016/j.scitotenv.2020.142649
48. Saulsbury, MD, Heyliger, SO, Wang, K, and Johnson, DJ. Chlorpyrifos induces oxidative stress in oligodendrocyte progenitor cells. Toxicology. (2009) 259:1–9. doi: 10.1016/j.tox.2008.12.026
49. Binmahfouz, LS, Hassanein, EHM, Bagher, AM, Hareeri, RH, Alamri, ZZ, Algandaby, MM, et al. Berberine alleviates chlorpyrifos-induced nephrotoxicity in rats via modulation of Nrf2/ho-1 axis. Heliyon. (2024) 10:e25233. doi: 10.1016/j.heliyon.2024.e25233
50. Sharma, P, Jha, AB, Dubey, RS, and Pessarakli, M. Reactive oxygen species, oxidative damage, and Antioxidative defense mechanism in plants under stressful conditions. J Bot. (2012) 2012:217037. doi: 10.1155/2012/217037
51. Abou-Zeid, SM, Ahmed, AI, Awad, A, Mohammed, WA, Metwally, MMM, Almeer, R, et al. Moringa oleifera ethanolic extract attenuates tilmicosin-induced renal damage in male rats via suppression of oxidative stress, inflammatory injury, and intermediate filament proteins mrna expression. Biomed Pharmacother. (2021) 133:110997. doi: 10.1016/j.biopha.2020.110997
52. Chumark, P, Khunawat, P, Sanvarinda, Y, Phornchirasilp, S, Morales, NP, Phivthong-Ngam, L, et al. The in vitro and ex vivo antioxidant properties, hypolipidaemic and antiatherosclerotic activities of water extract of Moringa oleifera lam. Leaves. J Ethnopharmacol. (2008) 116:439–46. doi: 10.1016/j.jep.2007.12.010
53. Jahan, IA, Hossain, MH, Ahmed, KS, Sultana, Z, Biswas, PK, and Nada, K. Antioxidant activity of Moringa oleifera seed extracts. Orient Pharm Exp Med. (2018) 18:299–307. doi: 10.1007/s13596-018-0333-y
54. Moyo, B, Oyedemi, S, Masika, PJ, and Muchenje, V. Polyphenolic content and antioxidant properties of Moringa oleifera leaf extracts and enzymatic activity of liver from goats supplemented with Moringa oleifera leaves/sunflower seed cake. Meat Sci. (2012) 91:441–7. doi: 10.1016/j.meatsci.2012.02.029
55. Sreelatha, S, and Padma, PR. Antioxidant activity and total phenolic content of Moringa oleifera leaves in two stages of maturity. Plant Foods Hum Nutr. (2009) 64:303–11. doi: 10.1007/s11130-009-0141-0
56. Arulselvan, P, Tan, WS, Gothai, S, Muniandy, K, Fakurazi, S, Esa, NM, et al. Anti-inflammatory potential of ethyl acetate fraction of Moringa oleifera in downregulating the Nf-κB signaling pathway in lipopolysaccharide-stimulated macrophages. Molecules. (2016) 21:1452. doi: 10.3390/molecules21111452
57. Kooltheat, N, Sranujit, RP, Chumark, P, Potup, P, Laytragoon-Lewin, N, and Usuwanthim, K. An ethyl acetate fraction of Moringa oleifera lam. Inhibits human macrophage cytokine production induced by cigarette smoke. Nutrients. (2014) 6:697–710. doi: 10.3390/nu6020697
58. Oyeleye, SI, Ojo, OR, and Oboh, G. Moringa oleifera leaf and seed inclusive diets influenced the restoration of biochemicals associated with erectile dysfunction in the penile tissue of Stz-induced diabetic male rats treated with/without Acarbose drug. J Food Biochem. (2021) 45:e13323. doi: 10.1111/jfbc.13323
59. Alhakmani, F, Kumar, S, and Khan, SA. Estimation of total phenolic content, in-vitro antioxidant and anti-inflammatory activity of flowers of Moringa oleifera. Asian Pac J Trop Biomed. (2013) 3:623–7; discussion 626–7. doi: 10.1016/S2221-1691(13)60126-4
60. Vongsak, B, Sithisarn, P, and Gritsanapan, W. Simultaneous Hplc quantitative analysis of active compounds in leaves of Moringa oleifera lam. J Chromatogr Sci. (2014) 52:641–5. doi: 10.1093/chromsci/bmt093
61. Fahey, JW. Moringa oleifera: a review of the medical evidence for its nutritional, therapeutic, and prophylactic properties. Part 1. Trees Life J. (2005) 1:1–15. doi: 10.1201/9781420039078.ch12
62. Wang, F, Bao, Y, Shen, X, Zengin, G, Lyu, Y, Xiao, J, et al. Niazirin from Moringa oleifera lam. Attenuates high glucose-induced oxidative stress through Pkcζ/Nox4 pathway. Phytomedicine. (2021) 86:153066. doi: 10.1016/j.phymed.2019.153066
63. Abdelsayed, EM, Medhat, D, Mandour, YM, Hanafi, RS, and Motaal, AA. Niazimicin: a thiocarbamate glycoside from Moringa oleifera lam. Seeds with a novel neuroprotective activity. J Food Biochem. (2021) 45:e13992. doi: 10.1111/jfbc.13992
64. Vasanth, K, Minakshi, GC, Velu, K, Priya, T, Kumar, RM, Kaliappan, I, et al. Anti-adipogenic β-sitosterol and lupeol from Moringa oleifera suppress adipocyte differentiation through regulation of cell cycle progression. J Food Biochem. (2022) 46:e14170. doi: 10.1111/jfbc.14170
65. Giacoppo, S, Galuppo, M, De Nicola, GR, Iori, R, Bramanti, P, and Mazzon, E. 4(α-l-rhamnosyloxy)-benzyl isothiocyanate, a bioactive phytochemical that attenuates secondary damage in an experimental model of spinal cord injury. Bioorg Med Chem. (2015) 23:80–8. doi: 10.1016/j.bmc.2014.11.022
Keywords: moringa, chlorpyrifos, brain, eye, antioxidant, oxidative stress, organophosphate, insecticide
Citation: Alanazi IS, Altyar AE, Zaazouee MS, Elshanbary AA, Abdel-Fattah A-FM, Kamel M, Albaik M and Ghaboura N (2024) Effect of moringa seed extract in chlorpyrifos-induced cerebral and ocular toxicity in mice. Front. Vet. Sci. 11:1381428. doi: 10.3389/fvets.2024.1381428
Edited by:
Mohamed Elbadawy, Benha University, EgyptReviewed by:
Mohamed A. Saleh, University of Sharjah, United Arab EmiratesMariam Alamoudi, Prince Sattam Bin Abdulaziz University, Saudi Arabia
Zeinab Mahasneh, The University of Jordan, Jordan
Copyright © 2024 Alanazi, Altyar, Zaazouee, Elshanbary, Abdel-Fattah, Kamel, Albaik and Ghaboura. This is an open-access article distributed under the terms of the Creative Commons Attribution License (CC BY). The use, distribution or reproduction in other forums is permitted, provided the original author(s) and the copyright owner(s) are credited and that the original publication in this journal is cited, in accordance with accepted academic practice. No use, distribution or reproduction is permitted which does not comply with these terms.
*Correspondence: Ibtesam S. Alanazi, ZXNhbGFuYXp5QHVoYi5lZHUuc2E=; Mai Albaik, bWFpLmFsYmFpa0BibWMuZWR1LnNh