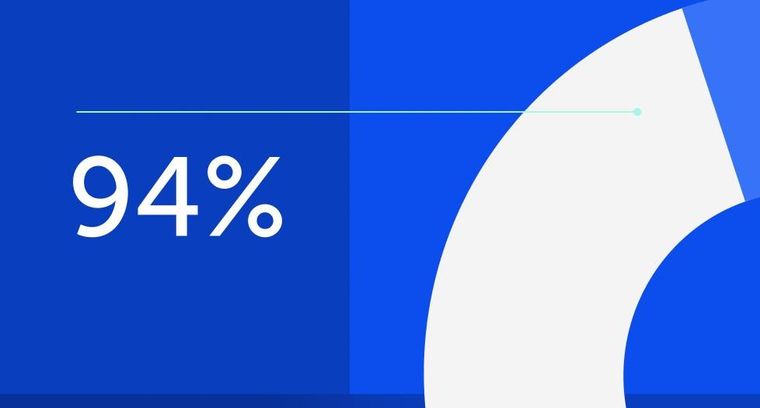
94% of researchers rate our articles as excellent or good
Learn more about the work of our research integrity team to safeguard the quality of each article we publish.
Find out more
ORIGINAL RESEARCH article
Front. Vet. Sci., 01 March 2024
Sec. Parasitology
Volume 11 - 2024 | https://doi.org/10.3389/fvets.2024.1361552
This article is part of the Research TopicParasites in One Health InterfaceView all 18 articles
The aim of this study was to investigate the anti-Eimeria tenella mechanism of Qingchang Compound (QCC) and provide a basis for its clinical application. The active ingredients, active ingredient-disease intersection targets, and possible pathways of QCC for the treatment of chicken coccidiosis were analyzed, the binding ability of pharmacodynamic components and target proteins was determined by network pharmacology and the molecular docking, and a model of infection with coccidiosis was constructed to verify and analyze the mechanism of action of QCC against coccidiosis. Among the 57 components that met the screening conditions, the main bioactive components were quercetin, dichroine, and artemisinin, with IL-1β, IL-6, IL-10, IFN-γ, and IL-8 as the core targets. Simultaneously, the KEGG signaling pathway of QCC anti-coccidiosis in chickens was enriched, including cytokine-cytokine receptor interactions. The results showed that the main pharmacodynamic components of QCC and the core targets could bind well; artemisinin and alpine possessed the largest negative binding energies and presented the most stable binding states. In addition, in vivo studies showed that QCC reduced blood stool in chickens with coccidiosis, restored cecal injury, and significantly reduced the mRNA and protein expression levels of IL-1β, IL-10, and IFN-γ in ceca (p < 0.01). Our results suggest that the main active ingredients of QCC are artemisinin and alpine and its mechanism of action against coccidiosis may be related to the reduction of the inflammatory response by acting on specific cytokines.
Chicken coccidiosis is a protozoan disease caused by coccidian parasites belonging to the phylum Apicomplexa, class Sporozoa, order Eucoccidiorida, family Eimeriidae, and genus Eimeria. The main causative agents of chicken coccidiosis are seven species of Eimeria, namely Eimeria tenella, E. necatrix, E. brunetti, E. maxima, E. acervulina, E. mitis, and E. praecox, among which E. tenella is the most pathogenic. This disease primarily affects young and juvenile chickens (1–4). It has been reported that the annual economic loss to China’s chicken industry due to coccidiosis is up to 5 billion yuan, and drug expenditure alone is approximately 1.56–1.95 billion yuan (5).
Although, the chemotherapeutic drugs (Diclazuril and Sulfonamides) were mainly agents, it had a severe resistance in coccidia isolated from chicken farms, manifesting as broad, crossover, or multidrug resistance (6). Therefore, researchers have conducted in multiple directions, including live vaccines, recombinant vaccines, and medicinal plants (7–9). However, live vaccines have problems of poor stability, easy virulence regression, and uneven immunity, thus the application of live vaccines for coccidia needs further improvement. At present, the development and clinical application of recombinant proteins targeting coccidia require a long time. Traditional Chinese medicine is widely used in veterinary clinics because it is difficult for microorganisms to develop drug resistance to these widely sourced herbal prescriptions. Hence, the research and development of veterinary traditional Chinese medicine anti-coccidial drugs aligns with the market demand and national policy.
The main characteristic of veterinary Chinese medicinal compounds is the combination of multiple components and targets; however, traditional research of compound therapeutics has mainly involved a single target and pathway. Research on the mechanisms of traditional Chinese medicines against parasite has mainly focused on the direct effects of drugs on the activation of the host’s immune function. The direct drug effects include recognition, movement, adhesion, invasion, and intracellular development, whereas the activation of the host’s immune function includes both specific and non-specific immunity, making it difficult to fully explain the pharmacological effects and molecular mechanisms of Chinese veterinary drug compounds. In recent years, network pharmacology has been widely used to reveal the pharmacodynamic components, action of signaling channels, and compatibility connotations of Chinese veterinary drugs with the overall research mode of “multi-component-multi-target disease” (10–12). Currently, research on the anti-coccidiosis effect of QCC in chickens is mostly limited to pharmacodynamic studies, and there are few reports on the anti-coccidiosis mechanism(s) of action of this herb. The traditional methods for studying the possible mechanism of action of veterinary Chinese medicine against coccidiosis are tedious, time consuming, and expensive. In contrast, the use of network pharmacology and molecular docking in this regard presents significant advantages (efficient, accurate, and comprehensive) (13). In this study, the main pharmacodynamic components, core targets, and enriched signaling pathways of QCC were analyzed using modern Chinese medicine network pharmacology and molecular docking, the core targets of the drug were verified through animal studies, and the anti-coccidiosis mechanism of action of QCC was determined.
The filtering conditions for the TCMSP database were Oral Bioavailability (OB) ≥ 30% and Drug-likeness (DL) ≥ 0.18. Through literature review, we identified the active components of four Chinese herbs—Artemisiae annuae herba, Dichroae radix, Agrimonia pilosa, and Sanguisorbae radix—and supplemented or cross-verified the data retrieved. Target information for these active components was downloaded from the TCMSP database. Component target proteins and gene names were matched using UniProt.1 The drugs, active components, and potential targets were imported into Cytoscape 3.8.2 software (Bloomage BioTechnology Corporation Limited, China) to construct a drug-active component-target network to identify important candidate components.
In the GeneCards,2 OMIM,3 and NCBI4 databases, we conducted keyword searches using the terms “Avian Coccidiosis,” “Coccidiosis in Chicken,” and “Chicken coccidia” to obtain targets related to coccidiosis in chickens. Species filtering was then performed using UniProt, and a Venn diagram was generated online.5 The targets of Artemisiae annuae herba, Dichroae radix, Agrimonia pilosa, Sanguisorbae radix, and chicken coccidiosis were imported separately. The intersection of these sets was used to identify common targets, which serve as targets for treating chicken coccidiosis with QCC. The intersecting targets obtained from the Venn diagram were imported into the String database.6 The species “Gallus” was selected, and a minimum interaction score of “medium confidence (0.400)” was chosen. After the protein–protein interaction (PPI) network was obtained, a TSV-format file was downloaded. This file was imported into Cytoscape 3.8.2 to construct a PPI network. The “Analyze Network” function was used to perform network analysis, calculating the degree values for all nodes in the network. Targets that ranked the highest based on their degree values were selected and used for subsequent molecular docking verification.
Gene Ontology (GO) and Kyoto Encyclopedia of Genes and Genomes (KEGG) pathway enrichment analyses were performed on the potential target genes through the DAVID website.7 The input for potential targets was restricted to chicken species. The resulting GO analysis was filtered based on a p-value of less than 0.05, and the KEGG analysis was filtered based on a p-value of less than 0.01. The results were sorted according to the number of enriched genes, and the top 20 entries were selected for both. Bubble charts and bar graphs were created to identify the main biological processes (BP), primary cellular components (CC), and principal molecular functions (MF) involved, as well as key signaling pathways implicated.
The 3D structures of the components of Artemisiae annuae herba, Dichroae radix, Agrimonia pilosa, and Sanguisorbae radix were obtained from the PubChem database and converted to the moL2 format using OpenBabel. They were then imported into PyMOL for addition of hydrogen, charging, and other treatments. The protein conformations were screened using the RCSB PDB database.8 The selection criteria included having a well-defined protein structure obtained through X-ray crystallography with a crystal resolution smaller than 3A. These proteins were then processed to remove water and small molecules and treated with hydrogen addition, charge addition, and non-polar hydrogen merging in Autodock Tools 1.5.6. Docking calculations between large and small molecules were performed using AutoDock Tools. Receptor–ligand pairs were sorted and screened based on binding free energy (affinity in kcal/mol). A compound is considered to spontaneously bind and interact with a protein if its binding energy is <0 kcal/mol, with lower-energy conformations being more stable. Generally, a binding energy of <−1.2 kcal/mol indicates good binding efficiency (14). Visualization was performed using PyMOL 2.3.2 software.
A precise amount of QCC (Artemisiae annuae herba, Dichroae radix, Agrimonia pilosa, and Radix Sanguisorbae = 5:2:2:1) was weighed and soaked in distilled water for 0.5 h, followed by extraction in 75% ethanol for 1 h, and subsequent water extraction for 1 h. The extracts from both processes were combined and concentrated to yield a solution with a concentration of 1.0 g/mL of raw medicine, which was then set aside for later use.
Tender E. oocysts were obtained from the Chongqing Academy of Animal Science. Gavage feeding of sporulated oocysts was performed in chickens to revitalize the oocysts. Fecal samples from the chickens were collected upon the appearance of bloody feces to isolate the oocysts. Harvested E. oocysts were cultured in 2.5% potassium dichromate solution at 28°C for 3 days (10). During this period, oocyst status was monitored. Culturing was halted when sporulation and hatching rates exceeded 90%, and the sporulated oocysts were collected and set aside.
One-day-old Ross broiler chicks purchased from a poultry farm in Chongqing were allowed ad libitum access to food and water. The feed did not contain any additives. After reaching 16 days of age, the chicks were transferred to flame-sterilized cages.
Thirty 16 days-old Ross broiler chicks were randomly divided into three groups: Control, Model, and QCC. Except for the Control group, the other two groups were orally gavaged with 1 × 104 sporulated oocysts per chicken. Five days post inoculation, the QCC group was orally administered QCC extract at a dose of 2.4 g/kg. An equivalent volume of physiological saline was administered to the Control and Model groups. The treatment was administered once daily for a continuous period of 7 days. All chickens had ad libitum access to food and water, and the feed provided did not contain any additives.
During this study, the clinical manifestations of the chickens were observed and recorded, including their mental state, feeding, fur, movement, and fecal state. At the end of the study, all chickens were dissected, and the ceca was collected, fixed with 4% paraformaldehyde, embedded in paraffin, sliced, and stained with hematoxylin and eosin. Pathological changes in the tissues were observed under a microscope, and a microscopic imaging system was used to capture images.
At the end of the experiment, eight chickens from each group were randomly euthanized. The cecal tissue samples were collected aseptically and immediately stored in liquid nitrogen until further use. Total RNA was extracted from the cecal tissue following the protocol provided in the TRIzol reagent kit. Subsequently, cDNA was synthesized by reverse transcription and used as a template for real-time quantitative PCR (qPCR) analysis. The qPCR products were visualized and photographed using a gel imaging system. The density of the target bands was quantified using image analysis software, and the relative mRNA expression levels of the target genes were calculated using the 2−ΔΔCt method. The primers were designed and synthesized according to gallus IL-1β (NM_204524.1), IL-6 (NM_204628.1), IL-10 (NM_001004414), IL-8 (DQ393272), and IFN-γ (NM_205149.1) gene sequences published in GenBank, all of which were synthesized by Bioengineering (Shanghai) Co., Ltd. The details of the primers used for real-time qPCR amplification are presented in Table 1.
All data were analyzed with SPSS 25.0 statistical software and are presented as means ± SD. One-way analysis of variance (ANOVA) was used to analyze data between groups, and the LSD method was used for pairwise comparisons. Statistical significance was set at p < 0.05 and 0.01. The letters a, b, and c indicate p < 0.05, and A, B, and C indicate p < 0.01.
After screening through the TCMSP database, 57 compounds were identified that met the criteria of OB ≥30% and DL ≥0.18. Among them, 22 were from Artemisiae annuae herba, 17 were from Dichroae radix, and an additional compound, dichroine, was included because of its importance, resulting in 18 compounds from Dichroae radix. Additionally, five compounds were identified from Agrimonia pilosa and 13 from Sanguisorbae radix. After deduplication, a total of 51 unique compounds were identified. Artemisia annua’s active components corresponded to 210 target sites; Dichroae radix’s active components corresponded to 52 target sites; Agrimonia pilosa’s active components corresponded to 178 target sites; and Sanguisorbae radix’s active components corresponded to 182 target sites. After eliminating the duplicates, 228 unique target sites were identified.
Following searches in the GeneCards, OMIM, and NCBI databases, 101 disease-target sites were identified. After species-specific filtering, 89 unique disease target sites were identified. Using Venn diagram analyses, 14 intersecting target sites were identified among the active constituents of Artemisiae annuae herba, Dichroae radix, Agrimonia pilosa, and Sanguisorbae radix and disease-specific targets for chicken coccidiosis (Figure 1A). A pharmacological network of drug-active component targets was constructed for Artemisiae annuae herba, Dichroae radix, Agrimonia pilosa, and Sanguisorbae radix. In this network, blue nodes represent potential target sites, pink nodes represent effective compounds, and yellow nodes represent drugs. The graph incorporates 51 compound nodes and 228 potential target nodes. Based on their prominence in the network, the top five compounds were quercetin, kaempferol, luteolin, artemisinin, and berberine. From the perspective of individual compound-target networks and based on the theory of network pharmacology, these five compounds could potentially account for the majority of the therapeutic effects of QCC and may represent the primary active substances in Artemisiae annuae herba, Dichroae radix, Agrimonia pilosa, and Sanguisorbae radix (Figure 1B). An analysis was conducted on the intersecting target sites obtained from both the constituent and disease-specific targets using Venn diagrams on the STRING website, resulting in a PPI network (Figure 1C). The network comprises 12 nodes and 46 edges. The size of each node symbolizes its degree value, whereas the color gradient from light to dark represents betweenness centrality. The thickness and color of the edges indicate the edge betweenness and combined score, respectively. Topological analysis of the PPI network identified hub proteins within this network. Based on their degree values, the top five proteins were IL-1β, IL-6, IL-10, IFN-γ, and IL-8. GO enrichment analysis was performed on potential target genes using DAVID. A total of 31 GO terms were identified, including 27 related to BP, one related to CC, and three related to MF (Figure 1D). The top 10 terms in each category are shown in Figure 1E. KEGG pathway analysis yielded a total of 11 enriched pathways.
Figure 1. Network pharmacology analysis. (A) Venn diagram of intersection of QCC and anti-coccidiosis targets; (B) active ingredients-target network of QCC; (C) protein–protein interaction (PPI) network within the common targets; (D) enrichment analysis of GO biological processes; (E) enrichment analysis of pathway signaling.
Significant compounds such as quercetin, kaempferol, luteolin, artemisinin, and berberine were docked with the core target proteins IL-1β, IL-6, IL-10, IFN-γ, and IL-8. The docking results are listed in Table 2. All docking binding energies were less than −1.2 kcal/mol, indicating that the active components artemisinin, berberine, quercetin, kaempferol, and luteolin from Artemisiae annuae herba, Dichroae radix, Agrimonia pilosa, and Sanguisorbae radix could spontaneously bind with core targets (Table 3). Docking results were visualized using PyMOL software, and detailed docking interactions were observed under magnification (Figure 2). These findings suggested that QCC could exert its anti-coccidial effects through components like artemisinin, berberine, quercetin, kaempferol, and luteolin targeting IL-1β, IL-6, IL-10, IFN-γ, and IL-8.
Figure 2. Molecular docking 3D diagram of core active ingredients in QCC with core anti-coccidiosis targets. (A) Quercetin-IFN-γ; (B) Quercetin-IL-1β; (C) Dichroine-IL-6; (D) Kaempferol IL-8; (E) Artemetin-IL1β; (F) Luteolin-IL-10.
Apart from the control group, chickens in both the model and drug treatment groups began to display symptoms of coccidiosis on the 4th day post-inoculation with sporulated oocysts. Symptoms included bloody feces, poor mental state, dull feather coat, decreased food intake, and blood in the cecal cavity. After administering QCC, clinical symptoms improved. The feces became more formed, and only trace amounts of blood were present (Figure 3). The mental state of the chickens improved, food intake returned to normal, and blood in the cecal cavity was not evident.
Figure 3. Effect of QCC on clinical manifestations of chickens with coccidiosis. (A) The red box indicates that there is a large amount of blood in the feces. (B) The red box indicates that the cecum is dark red with clots. (C) The red box indicates that a small amount of blood could be found in feces. (D) The red box indicates that the cecum is smooth, no swelling, with a smooth outer wall and no obvious blood was found.
The cecal tissue structure of chickens in the control group was normal, the cells were neatly arranged and there was no obvious histopathological damage. Cecal tissue from the model group showed a large range of inflammatory cell infiltration, and coccidia bodies were observed in epithelial cells. Further, the mucosal layer was severely damaged. In the QCC group, no coccidiosis was found in the cecum epithelial cells; however, a small area of inflammatory cell infiltration and epithelial cell shedding was observed (Figure 4).
Figure 4. Effect of QCC on Cecal Tissue of Coccidiosis Chickens. (A) Control group, HE, Bar = 100 μm, 100×; (B) model group, HE, Bar = 100 μm, 100×. A large number of inflammatory cells infiltrate (black arrow), and numerous parasite bodies are seen in glandular epithelial cells (green arrow); (C) QCC group, HE, Bar = 100 μm, 100×. Inflammatory cell infiltration (black arrow), glandular epithelial cells detached from the basal portion (yellow arrow).
At the end of the study, there was no significant difference in the mRNA expression of IL-6 and IL-8 (p > 0.05) in cecum tissues. The mRNA expression levels of IL-1β, IL-10, and IFN-γ in the model group were high, and the difference was significant compared with that in the control group (p < 0.01). The mRNA expression levels of IL-1β, IL-10, and IFN-γ in the QCC group were reduced, and the difference was significant compared with that in the model group (p < 0.01) (Figure 5).
Figure 5. The mRNA expression of coccidiosis after QCC treated in caecum. The mRNA expresses of IL-1β (A), IL-10 (B), IFN-γ (C), IL-6 (D), IL-8 (E). Different lower-case letters on the column mark indicate significant difference (p < 0.05), and different capital letters on the superscript indicate extremely significant difference (p < 0.01).
At the end of the study, there was no significant difference in the protein expression of IL-6 and IL-8 in the cecum tissues from all the groups (p > 0.05). Protein expression of IL-1β and IFN-γ in the QCC group was significantly lower than in the model group (p < 0.01). Compared to the control group, the protein expression of IL-10 in the cecum of the model and drug groups increased significantly (p < 0.01), but there was no significant difference between the model and QCC groups (p > 0.05) (Figure 6).
Figure 6. The protein expression of coccidiosis after QCC treated in caecum. The western bolt bar (A) and protein expression (B) of IL-1β, IL-10, IFN-γ, IL-6, and IL-8. Different lower-case letters on the column mark indicate significant difference (p < 0.05), and different capital letters on the superscript indicate extremely significant difference (p < 0.01).
Chicken coccidiosis is an acute inflammatory intestinal protozoal disease with high incidence rate and mortality, which is globally prevalent and non-seasonal (15, 16). The use of anticoccidial chemicals, coccidiocides, coccidiostats, and ionophores has been a common practice in modern poultry production for controlling avian coccidiosis. However, the emergence of drug resistance and increasing consumer demand for meat free of residues has prompted the development of alternative control methods (17). In recent years, research has found that some traditional Chinese veterinary medicine, plant extracts, essential oils and probiotics, have significant anti coccidiosis activity with minimal side effects, and low susceptibility to drug resistance, such as Artemisia brevifolia Extract could enhancing the immunomodulatory potential against coccidiosis (18), herbal formula extract highly reduced the coccidian multiplication rate and reduced the severity of intestinal lesions (19). Citrus sinensis Essential Oil and Star Anise (Illicium verum) Essential Oil supplementation lowered oocysts shedding in faeces and faecal score (20, 21). Among these alternative insecticides, veterinary traditional Chinese medicine has a relatively complete medication theory and history. According to historical experience, some TCMs with “cold taste and bitter” properties, such as Artemisiae annuae herba and Dichroae radix, have antiparasitic effects. Qingchang Compound (QCC) is a traditional Chinese medicine for veterinary use with efficacy against chicken coccidiosis, which has undergone early stage clinical trials alongside Artemisiae annuae herba, Dichroae radix, Agrimonia pilosa, and Sanguisorbae radix (22). Our study preliminarily predicted and validated the activity of the top five active ingredients (quercetin, kaempferol, luteolin, artemisinin, and dichroine) in QCC and their affinity with five core targets (IL-1β, IL-6, IL-10, IFN-γ, and IL-8). Further study revealed that QCC could improve the clinical symptoms of cecal coccidiosis in chickens, reduce cecal tissue injuries, and downregulate the expression of IL-1β, IL-10, and INF-γ.
In this study, the main components of QCC with anti-coccidiosis efficacy in chickens were analyzed by network pharmacology, and the main bioactive components (artemisinin, dichroine, quercetin, kaempferol, and luteolin) exhibit strong anti-parasitic activity. Previous studies have confirmed that the Artemisia annua and Atractylodes lancea has significant anti-coccidial effects (23). Artemisinin, the main active component of the traditional Chinese medicine Artemisia annua, can induce apoptosis of second-generation merozoites by reducing mitochondrial membrane potential, leading to a remarkable suppression of the upregulated mRNA levels of NF-κB and interleukin-17A in the ceca during Eimeria tenella infection (23, 24). In addition, artemisinin can bind to membrane proteins (ATP transporters) in the asexual reproductive stage of plasmodium falciparum to reduce the infectivity of plasmodium malaria parasites (25, 26), and then promote the functional recovery by regulating the body’s inflammatory response. The natural product dichroine and its synthetic derivatives has been proved that can disrupt the activity of synthetase by regulating the gene encoding gluprolyl-tRNA synthetase in plasmodium falciparum (27). The anti-parasitic effects of quercetin, kaempferol, and luteolin are primarily manifested in their ability to inhibit the inflammatory response induced by parasites (28–30). Therefore, we speculate that the anti-coccidiosis effect of QCC is mainly related to artemisinin, dichroine, quercetin, kaempferol, and luteolin, which may be related to their direct inhibition of parasite reproduction and reduction of excessive inflammatory responses.
Molecular docking is an important method for studying the interactions between ligands and receptors, predicting their binding modes and affinities, and searching for candidate compounds based on the docking results (31). We determined that the core targets (IL-1β, IL-6, IL-10, IFN-γ, and IL-8) of QCC can effectively bind with the core pharmacological components, among which artemisinin and alpine showed the largest negative binding energies and most stable binding abilities. And those of IL-1β and IFN-γ to each major component were low, indicating that it could bind spontaneously with the main component. When infected with coccidia, T lymphocytes can secrete various cytokines (the main is IFN-γ, IL-1β), which in turn play a role in anti-infection and immune protection (32). IFN-γ plays a crucial role in resisting intracellular parasitic protozoa, and directly kills parasites or inhibit their growth in the host (33). IL-1β is an important pro-inflammatory factor in the body, which can enhance the host’s resistance to coccidian infection, but can also exacerbate intestinal damage and dysfunction (34). Therefore, it is speculated that the therapeutic substances of QCC can regulate the synthesis and secretion of IL-1β, IFN-γ, and IL-8 through the aforementioned interactions, thereby modulating intestinal inflammation and achieving therapeutic effects against nematode infections. In addition, KEGG analysis showed that these targets are mainly focused on the cytokine receptor interaction and influenza a pathway, indicating that future research should focus on these pathways.
In vivo study, IL-1β, INF-γ, and IL-10 mRNA were significantly increased in chicken ceca after infection with coccidiosis, the results were consistent with those of previous studies (35, 36). This paper found that QCC can reduce the expression levels of IL-1β, INF-γ, and IL-10. IL-1β is one of the important pro-inflammatory factors involved in intestinal inflammation, which promotes the production of inflammatory transmitters, the multiplication and differentiation of B cells, and stimulates the expression of immune molecules in body cells, thereby improving the level of humoral and cellular immunity (37). INF-γ is an important active substance that regulates the balance of the Th1/Th2 immune responses and plays an important role in immune regulation (38), which has been reported to be the most important cytokine in the fight against coccidiosis infection (39). IL-10 reduces the degree of intestinal inflammation by inhibiting the release of IL-1, IL-6, and IL-8 (40). Combine with our results, we believe that after treatment with QCC, the body is in a state of low inflammatory response and a low degree of infection, which may be related to the decrease in bloody stool and alleviation of intestinal tissue damage. In the next study, we will verify the effects of the effective ingredients of QCC on the body and investigate whether there is synergy among artemisinin, dichroine, quercetin, kaempferol, and luteolin.
QCC can reduce blood content in feces and intestinal damage in coccidiosis infected chickens by regulating inflammation-related cytokines, reducing the intestinal inflammatory response, and preventing the development of parasites in intestinal epithelial cells, thereby possessing anti-coccidiosis activity.
The datasets presented in this study can be found in online repositories. The names of the repository/repositories and accession number(s) can be found in the article/supplementary material.
The animal studies were approved by the ethics approval reference number for the use of animals was XKY-20230420, which approved by the Chongqing Academy of Animal Science Animal Ethics Committee. The studies were conducted in accordance with the local legislation and institutional requirements. Written informed consent was obtained from the owners for the participation of their animals in this study.
ZY: Writing – original draft. CC: Data curation, Writing – review & editing. SZ: Formal analysis, Investigation, Writing – original draft. HT: Resources, Writing – review & editing. MZ: Methodology, Writing – original draft. YY: Writing – original draft. HZ: Writing – original draft.
The author(s) declare financial support was received for the research, authorship, and/or publication of this article. The experimental materials and reagents of this project are all funded by Chongqing Natural Science Foundation Chongqing Natural Science Foundation Project (cstc2019jcyj-msxmX0061).
The authors declare that the research was conducted in the absence of any commercial or financial relationships that could be construed as a potential conflict of interest.
All claims expressed in this article are solely those of the authors and do not necessarily represent those of their affiliated organizations, or those of the publisher, the editors and the reviewers. Any product that may be evaluated in this article, or claim that may be made by its manufacturer, is not guaranteed or endorsed by the publisher.
2. ^ https://www.genecards.org/
4. ^ https://www.ncbi.nlm.nih.gov/
5. ^ http://www.bioinformatics.com.cn/
1. Blake, DP , Knox, J , Dehaeck, B , Huntington, B , Rathinam, T , Ravipati, V, et al. Re-calculating the cost of coccidiosis in chickens. Vet Res. (2020) 51:115. doi: 10.1186/s13567-020-00837-2
2. Bortoluzzi, C , Perez-Calvo, E , Olsen, PB , van der Vaart, S , van Eerden, E , Schmeisser, J, et al. Effect of microbial muramidase supplementation in diets formulated with different fiber profiles for broiler chickens raised under various coccidiosis management programs. Poult Sci. (2023) 102:102955. doi: 10.1016/j.psj.2023.102955
3. Gulzar, N , Andleeb, S , Raza, A , Ali, S , Liaqat, I , Raja, SA, et al. Acute toxicity, anti-diabetic, and anti-cancerous potential of Trillium govanianum-conjugated silver nanoparticles in Balb/c mice. Curr Pharm Biotechnol. (2023) 24:124025. doi: 10.2174/1389201024666230818124025
4. Bremner, A , Kim, S , Morris, KM , Nolan, MJ , Borowska, D , Wu, Z, et al. Kinetics of the cellular and transcriptomic response to Eimeria maxima in relatively resistant and susceptible chicken lines. Front Immunol. (2021) 12:653085. doi: 10.3389/fimmu.2021.653085
5. Mao, X , Dou, Y , Fan, X , Yu, B , He, J , Zheng, P, et al. The effect of dietary Yucca schidigera extract supplementation on productive performance, egg quality, and gut health in laying hens with Clostridium perfringens and coccidia challenge. Poult Sci. (2023) 102:102822. doi: 10.1016/j.psj.2023.102822
6. Chapman, HD , and Rathinam, T . Focused review: the role of drug combinations for the control of coccidiosis in commercially reared chickens. Int J Parasitol Drugs Drug Resist. (2022) 18:32–42. doi: 10.1016/j.ijpddr.2022.01.001
7. Lakho, S , Haseeb, M , Huang, J , Hasan, M , Khand, F , Leghari, A, et al. Eimeria tenella 14-kDa phosphohistidine phosphatase stimulates maturation of chicken dendritic cells and mediates DC-induced T cell priming in a Th1 cytokine interface. Res Vet Sci. (2022) 152:61–71. doi: 10.1016/j.rvsc.2022.07.022
8. Haseeb, M , Huang, J , Lakho, S , Yang, Z , Hasan, M , Ehsan, M, et al. Em14-3-3 delivered by PLGA and chitosan nanoparticles conferred improved protection in chicken against Eimeria maxima. Parasitol Res. (2022) 121:675–89. doi: 10.1007/s00436-021-07420-4
9. Jamil, M , Aleem, M , Shaukat, A , Khan, A , Mohsin, M , Rehman, T, et al. Medicinal plants as an alternative to control poultry parasitic diseases. Life. (2022) 12:449. doi: 10.3390/life12030449
10. Yan, Z , Chen, Q , Fu, L , Fu, W , Zheng, H , Tang, H, et al. Effect of Qing Chang oral liquid on the treatment of artificially infected chicken coccidiosis and the cellular immunity. Vet Med Sci. (2022) 8:2504–10. doi: 10.1002/vms3.922
11. Luo, Y , Yang, S , Wen, M , Wang, B , Liu, J , Li, S, et al. Insights into the mechanisms of triptolide nephrotoxicity through network pharmacology-based analysis and RNA-seq. Front Plant Sci. (2023) 14:1144583. doi: 10.3389/fpls.2023.1144583
12. Yang, W , Zhang, Y , Gao, J , Hu, P , Yang, Y , and Xu, X . A meta-analysis of the Zilongjin tablets for non-small cell lung cancer and its network pharmacology of action against NSCLC and COVID-19. Front Med. (2023) 10:1080121. doi: 10.3389/fmed.2023.1080121
13. Zhang, P , Zhang, D , Zhou, W , Wang, L , Wang, B , Zhang, T, et al. Network pharmacology: towards the artificial intelligence-based precision traditional Chinese medicine. Brief Bioinform. (2023) 25:518. doi: 10.1093/bib/bbad518
14. Liang, X , Xie, H , Yu, L , Ouyang, J , Peng, Q , Chen, K, et al. Study on the effects and mechanisms of Wenzhong Bushen formula in improving ovarian reserve decline in mice based on network pharmacology. J Ethnopharmacol. (2024) 324:117756. doi: 10.1016/j.jep.2024.117756
15. Mesa-Pineda, C , Navarro-Ruíz, JL , López-Osorio, S , Chaparro-Gutiérrez, JJ , and Gómez-Osorio, LM . Chicken coccidiosis: from the parasite lifecycle to control of the disease. Front Vet Sci. (2021) 8:787653. doi: 10.3389/fvets.2021.787653
16. Fatoba, AJ , and Adeleke, MA . Diagnosis and control of chicken coccidiosis. A recent update. J Parasit Dis. (2018) 42:483–93. doi: 10.1007/s12639-018-1048-1
17. Muthamilselvan, T , Kuo, T , Wu, Y , and Yang, W . Herbal remedies for coccidiosis control. A review of plants, compounds, and anticoccidial actions. Evid Based Complement Alternat Med. (2016) 2016:2657981. doi: 10.1155/2016/2657981
18. Kashif, H , Asghar, A , Hamdan, A , Abdulrahman, M , Ahmad, A , Atif, R, et al. Immunomodulatory effects of Artemisia brevifolia extract against experimentally induced coccidiosis in broiler chicken. Pak Vet J. (2023) 43:333–8. doi: 10.29261/pakvetj/2023.026
19. Pop, L , Varga, E , Coroian, M , Nedian, M , Mircean, V , Dumitrache, M, et al. Efficacy of a commercial herbal formula in chicken experimental coccidiosis. Parasites Vect. (2019) 12:343. doi: 10.1186/s13071-019-3595-4
20. Aqsa, I , and Abdullah, A . Anticoccidial efficacy of Citrus sinensis essential oil in broiler chicken. Pak Vet J. (2022) 42:461–6. doi: 10.29261/pakvetj/2022.082
21. Nawal, A , Khalid, M , Zohaib, S , Kostadin, K , Junaid, A , Muhammad, A, et al. Anticoccidial activity of star Anise (Illicium verum) essential oil in broiler chicks. Pak Vet J. (2023) 43:553–8. doi: 10.29261/pakvetj/2023.050
22. Yan, Z , Xu, D , Fu, W , Chen, C , Tang, H , Zhu, M, et al. Screening of traditional Chinese medicine prescription against chicken coccidiosis and its pathological effects on cecal tissue of sick chicken. J Southern Agric. (2019) 2019:2322–8. doi: 10.3969/j.issn
23. Jiao, J , Yang, Y , Liu, M , Li, J , Cui, Y , Yin, S, et al. Artemisinin and Artemisia annua leaves alleviate Eimeria tenella infection by facilitating apoptosis of host cells and suppressing inflammatory response. Vet Parasitol. (2018) 254:172–7. doi: 10.1016/j.vetpar.2018.03.017
24. Mo, P , Ma, Q , Zhao, X , Cheng, N , Tao, J , and Li, J . Apoptotic effects of antimalarial artemisinin on the second generation merozoites of Eimeria tenella and parasitized host cells. Vet Parasitol. (2014) 206:297–303. doi: 10.1016/j.vetpar.2014.09.025
25. Ismail, HM , Barton, V , Phanchana, M , Charoensutthivarakul, S , Wong, MHL , Hemingway, J, et al. Artemisinin activity-based probes identify multiple molecular targets within the asexual stage of the malaria parasites plasmodium falciparum 3D7. Proc Natl Acad Sci USA. (2016) 113:2080–5. doi: 10.1073/pnas.1600459113
26. Sun, L , Chen, Z , Ni, Y , and He, Z . Network pharmacology-based approach to explore the underlying mechanism of sinomenine on sepsis-induced myocardial injury in rats. Front Pharmacol. (2023) 14:1138858. doi: 10.3389/fphar.2023.1138858
27. Herman, JD , Pepper, LR , Cortese, JF , Estiu, G , Galinsky, K , Zuzarte-Luis, V, et al. The cytoplasmic prolyl-tRNA synthetase of the malaria parasite is a dual-stage target of febrifugine and its analogs. Sci Transl Med. (2015) 7:288ra77. doi: 10.1126/scitranslmed.aaa3575
28. Sabya, S , Amit, K , Priya, R , Shubhankar, K , and Sandeep, K . Therapeutic potential of quercetin-loaded Nanoemulsion against experimental visceral Leishmaniasis: in vitro/ ex vivo studies and mechanistic insights. Mol Pharm. (2022) 19:3367–84. doi: 10.1021/acs.molpharmaceut.2c00492
29. Hanif, H , Abdollahi, V , Javani Jouni, F , Nikoukar, M , Rahimi Esboei, B , Shams, E, et al. Quercetin nano phytosome: as a novel anti-leishmania and anti-malarial natural product. J Parasit Dis. (2023) 47:257–64. doi: 10.1007/s12639-022-01561-8
30. Abo, M , Metwally, K , El-Menyawy, H , Hegab, F , and El-Wakil, E . Assessment of the potential prophylactic and therapeutic effects of kaempferol on experimental Trichinella spiralis infection. J Helminthol. (2023) 97:e36. doi: 10.1017/S0022149X23000184
31. Siham, O , Nuha, A , and Yassir, A . Immunoinformatics, molecular docking and dynamics simulation approaches unveil a multi epitope-based potent peptide vaccine candidate against avian leukosis virus. Sci Rep. (2024) 14:2870. doi: 10.1038/s41598-024-53048-6
32. Li, J , Yang, X , Jia, Z , Ma, C , Pan, X , and Ma, D . Activation of ChTLR15/ChNF-κB-ChNLRP3/ChIL-1β signaling transduction pathway mediated inflammatory responses to E. tenella infection. Vet Res. (2021) 52:15. doi: 10.1186/s13567-020-00885-8
33. Pu, X , Pan, Y , Xiang, Q , Lu, M , Xu, L , Yan, R, et al. Inhibitory effect of Eimeria maxima IFN-γ inhibitory molecules on the immune function of T cell subsets in chickens. Poult Sci. (2023) 102:103098. doi: 10.1016/j.psj.2023.103098
34. Bai, B , Liu, Q , Kong, R , Jia, Z , Chen, H , Zhi, W, et al. Role of Nrf2/HO-1 pathway on inhibiting activation of ChTLR15/ChNLRP3 inflammatory pathway stimulated by E.Tenella sporozoites. Poult Sci. (2024) 103:103445. doi: 10.1016/j.psj.2024.103445
35. Min, W , Lillehoj, H , Burnside, J , Weining, K , Staeheli, P , and Zhu, J . Adjuvant effects of IL-1beta, IL-2, IL-8, IL-15, IFN-alpha, IFN-gamma TGF-beta4 and lymphotactin on DNA vaccination against Eimeria acervulina. Vaccine. (2001) 20:267–74. doi: 10.1016/s0264-410x(01)00270-5
36. Hong, Y , Lillehoj, H , Lee, S , Dalloul, R , and Lillehoj, E . Analysis of chicken cytokine and chemokine gene expression following Eimeria acervulina and Eimeria tenella infections. Vet Immunol Immunopathol. (2006) 114:209–23. doi: 10.1016/j.vetimm.2006.07.007
37. Casillas-Ramírez, A , Micó-Carnero, M , Sánchez-González, A , Maroto-Serrat, C , Trostchansky, A , and Peralta, C . NO-IL-6/10-IL-1β axis: a new pathway in steatotic and non-steatotic liver grafts from brain-dead donor rats. Front Immunol. (2023) 14:1178909. doi: 10.3389/fimmu.2023.1178909
38. Jiang, S , Sun, B , Zhang, Y , Han, J , Zhou, Y , Pan, C, et al. The immediate adverse drug reactions induced by ShenMai injection are mediated by thymus-derived T cells and associated with RhoA/ROCK signaling pathway. Front Immunol. (2023) 14:1135701. doi: 10.3389/fimmu.2023.1135701
39. Liu, J , Liu, L , Li, L , Tian, D , Li, W , Xu, L, et al. Protective immunity induced by Eimeria common antigen 14-3-3 against Eimeria tenella, Eimeria acervulina and Eimeria maxima. BMC Vet Res. (2018) 14:337. doi: 10.1186/s12917-018-1665-z
Keywords: Qingchang compound, network pharmacology, molecular docking, experimental verification, main components, core target
Citation: Yan Z, Chen C, Zhai S, Tang H, Zhu M, Yu Y and Zheng H (2024) Mechanism of Qingchang compound against coccidiosis based on network pharmacology-molecular docking. Front. Vet. Sci. 11:1361552. doi: 10.3389/fvets.2024.1361552
Received: 26 December 2023; Accepted: 22 February 2024;
Published: 01 March 2024.
Edited by:
Hongbin Yan, Chinese Academy of Agricultural Sciences, ChinaReviewed by:
Muhammad Tahir Aleem, Nanjing Agricultural University, ChinaCopyright © 2024 Yan, Chen, Zhai, Tang, Zhu, Yu and Zheng. This is an open-access article distributed under the terms of the Creative Commons Attribution License (CC BY). The use, distribution or reproduction in other forums is permitted, provided the original author(s) and the copyright owner(s) are credited and that the original publication in this journal is cited, in accordance with accepted academic practice. No use, distribution or reproduction is permitted which does not comply with these terms.
*Correspondence: Zhiqiang Yan, eWFuemhpcWlhbmcyMDIwMTJAMTYzLmNvbQ==; Yuandi Yu, eXV5ZEBjcWFhLmNu; Hua Zheng, emhlbmdfMTIxXzE2QDE2My5jb20=
†These authors have contributed equally to this work
Disclaimer: All claims expressed in this article are solely those of the authors and do not necessarily represent those of their affiliated organizations, or those of the publisher, the editors and the reviewers. Any product that may be evaluated in this article or claim that may be made by its manufacturer is not guaranteed or endorsed by the publisher.
Research integrity at Frontiers
Learn more about the work of our research integrity team to safeguard the quality of each article we publish.