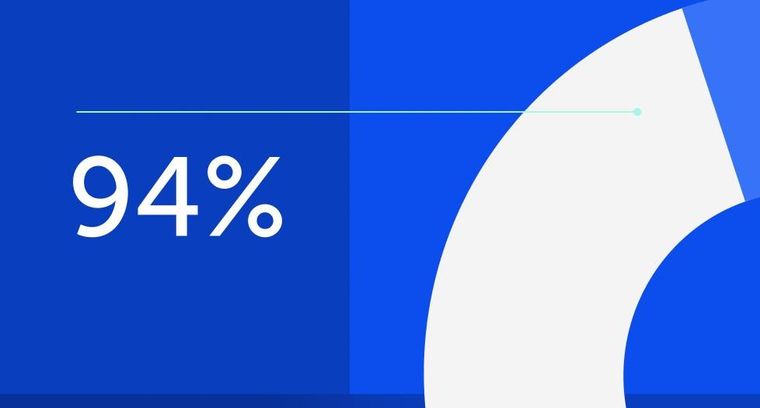
94% of researchers rate our articles as excellent or good
Learn more about the work of our research integrity team to safeguard the quality of each article we publish.
Find out more
REVIEW article
Front. Vet. Sci., 21 February 2024
Sec. Veterinary Dentistry and Oromaxillofacial Surgery
Volume 11 - 2024 | https://doi.org/10.3389/fvets.2024.1325559
Oral and maxillofacial (OMF) defects are not limited to humans and are often encountered in other species. Reconstructing significant tissue defects requires an excellent strategy for efficient and cost-effective treatment. In this regard, tissue engineering comprising stem cells, scaffolds, and signaling molecules is emerging as an innovative approach to treating OMF defects in veterinary patients. This review presents a comprehensive overview of OMF defects and tissue engineering principles to establish proper treatment and achieve both hard and soft tissue regeneration in veterinary practice. Moreover, bench-to-bedside future opportunities and challenges of tissue engineering usage are also addressed in this literature review.
As is the case with other clinical disciplines, oral and maxillofacial (OMF) surgery is constantly evolving, always aiming to improve treatment capability. Veterinary OMF surgeons often encounter technical challenges when reconstructing hard and soft tissue defects, and alternative approaches that can help minimize some of the limitations are necessary (1). In this context, tissue engineering was introduced as a novel approach to tissue regeneration and repair (2), and extensive studies have been conducted aiming to overcome the drawbacks of conventional solutions in OMF surgery. In addition, tissue regeneration implies the involvement of tissue component substitution to the damaged tissue returning to the normal state. While tissue repair involves a “patching” mechanism, with connective tissue deposition such as a scar to provide enough integrity to the injured tissue. However, excessive connective tissue deposition, known as fibrosis, in some tissues may alter the tissue functions due to the inability of tissue remodeling (3–5). As noted by Langer and Vacanti, tissue engineering comprises the use of stem cells, scaffolds, and/or signaling molecules (2). Interestingly, trend of using differentiated or somatic cells can be employed for tissue engineering as well (6). Recently, the trend of scaffold-free approaches have been raised and elaborated in the tissue engineering field. The approaches focus on the overcoming the issues of cell survival and localization, immune reaction, and ECM protection and growth factor storage (7, 8). Among the different types of cells, stem cells have been highlighted because primary cells have limitations in cell resource and risk for disease transmission (9). Stem cells to be used for tissue engineering may be embryonic stem cells (ESCs), adult stem cells such as mesenchymal stem cells (MSCs) and hematopoietic stem cells (HSCs), or induced pluripotent stem cells (iPSCs) (9). However, MSCs have received much attention due to their potential for clinical use in both humans and animals (10). Mesenchymal stem cells can be divided based on the collection site into oral and non-oral tissue origins (11). Scaffolds can be utilized as a carrier for cells and signaling molecules (9). In this review, we elaborate on some of the fundamental concepts underlying tissue engineering and describe current trends directed toward future applications in veterinary OMF surgery. The tissue engineering concept to be translated for OMF regeneration in veterinary practice is illustrated in Figure 1.
Figure 1. Translational concept of oral and maxillofacial tissue engineering in veterinary medicine.
The OMF region is composed of hard and soft tissues. Hard tissues include the teeth and multiple bones, such as the maxillary, incisive, mandibular, nasal, frontal, temporal, zygomatic, and palatine bones, among many others. The mandible articulates with maxillary structures via the temporomandibular joint (TMJ). Associated soft tissues include the TMJ disc and attachment, cartilage and attachment, tongue, oral mucosal surfaces, lymphoid tissue, salivary glands, muscles, nerves, vascular system, and skin (12). Understanding the complex anatomy and functionality of the OMF region is essential when diagnosing associated disorders and implementing proper treatments that can achieve an optimal outcome.
Anatomical defects that affect the functionality of the OMF region are common in both humans and animals. Generally, OMF defects can be classified as congenital or acquired (13). Orofacial clefts represent a relatively prevalent example of a congenital OMF defect of high clinical relevance that requires surgical reconstruction. Conversely, acquired defects are a frequent sequela of oncological surgery and traumatic injuries of the OMF region. Several classifications or grading schemes have been reported in humans based on the specific anatomical location of the OMF defect. Although there is no standardized classification system for animals, OMF traumatic injuries (e.g., vehicular accident, animal bites, blunt force, and others) and congenital defects (clefts of the lip, palate, or both) have been comprehensively documented in dogs (14–17).
Depending on the nature, extent, location, and stage of the OMF disorder, conventional surgical techniques may be contraindicated due to the inability to reconstruct existing or resultant defects (18). Moreover, allogenic organ transplantation and prosthetic rejection due to an adverse immune response may pose an important obstacle (19). Tissue engineering is an interdisciplinary field that combines biology and engineering principles to establish functional substitutes for damaged tissue (2) with the aim of addressing the aforementioned limitations, especially for the application in OMF reconstruction.
Using the current state of the art tissue engineering solutions, current conventional surgical techniques, such as autologous bone graft surgery could be complemented or replaced to address the general surgical concerns of donor site morbidity, poor anatomical match, insufficient graft volume, graft resorption, and rejection (20, 21). To reconstruct orofacial clefts in dogs, soft tissue flaps are typically harvested from the region adjacent to the defect. In severe cases where the cleft is too wide for local tissue flaps, tooth extraction and a staged approach has been described (22). Surgical site morbidity associated with soft tissue harvest sites, tooth extraction, as well as dehiscence rate (23), are the primary complications of this concern. Regarding acquired bone defects that result in functional disturbances, treatments have been variable and span the spectrum of surgical and non-surgical options (24, 25). None of the techniques described are without complication. Alternatively, tissue regeneration is an option that aims to restore and replace damaged tissue that overcomes conventional correction techniques by applying tissue engineering field (26).
As previously mentioned, stem cells represent the main cell source used for tissue regeneration because of their self-renewal, multilineage differentiation and high proliferative potential (19, 26, 27). Mesenchymal stem cells (MSCs) are the primary focus of this review given that they can differentiate into hard and soft tissue cells and be tissue-engineered for repair of different OMF defects. With this in mind, we further divide cell sources according to origin as either oral or non-oral MSCs. To be noted, craniomaxillofacial tissues have not only oral MSCs, but also others including suture-derived MSCs and periosteum-derived stem cells (28, 29). However, this literature review focuses on the oral/mouth-derived MSCs because suture- and periosteum-derived MSCs are still sparsely reported.
Oral derived human stem cells have been previously reported (11, 28, 29). In 2000, dental pulp stem cells (DPSCs) were first isolated from permanent third molar teeth and their stemness properties were demonstrated (30). Successful isolation of DPSCs was followed by stem cells from exfoliated deciduous teeth in 2003 (31), periodontal ligament stem cells (PDLSCs) in 2004 (32), dental follicle stem cells (DFSCs) in 2005 (33), stem cells from apical papilla (SCAP) in 2006 (34), and gingival MSCs (GMSCs) in 2010 (35). It has been reported that oral stem cells are advantageous in terms of collection due to its easiness with least invasive way, convenience, and affordable, cryopreservable, and transportable. Additionally, the cells are able to have interaction with scaffold and signaling molecule (36–38). These previous studies showed that the cells exhibited the expected behavior and potentiality such as high proliferation rate and multilineage differentiation ability. According to the International Society for Cellular Therapy (ISCT), multilineage differentiation ability is that MSCs can be differentiated into osteoblasts, adipocytes, and chondroblasts in vitro (39). Many studies also reported confirmational expression of pluripotency regulators and proliferative marker either as in genes or proteins, e.g., SRY-box transcription factor (TF) 2 (SOX2), REX1 TF (REX1), octamer-binding TF 4 (OCT4), and homeobox TF Nanog (NANOG), and proliferation marker Ki67 antigen (Ki67), respectively (40–42). In addition, this literature review presented the results of MSC surface markers both from flow cytometry and polymerase chain reaction (PCR) results, including CD146 (MSC multipotency), CD44 (cell-matrix interaction), CD29 (cell adhesion), CD73 (cell migration and anti-inflammatory property) CD90 (cell adhesion, migration, homing, proliferation, apoptosis, and differentiation), CD105 (cell migration, proliferation, and differentiation), and CD45 (hematopoietic stem cell (HSC) marker) (43–49).
Stem cells have also been isolated from the oral tissues of non-human mammalian species including canines, felines, equines, chimpanzees, swines, murines, and minipigs (34, 43, 50–53). Among these, canine-derived oral-origin MSCs have been widely studied (41, 43, 54–64). In contrast, feline-derived oral-origin MSCs have only been sparsely reported. Therefore, we have drawn parallels between canine and human oral-origin MSCs. Indeed, one study showed that canine DPSCs exhibited similar stemness properties to human DPSCs including adherence to a plastic surface and fibroblast-like morphology (43). The same study also found that canine DPSCs have a higher proliferation rate than human bone marrow-derived mesenchymal stem cells (BM-MSCs), yet lower than human DPSCs. In addition, human DPSCs have a higher number in colony formation than canine DPSCs (63). Moreover, canine DPSCs showed higher mRNA expression of CD146 and Nanog (43). In addition, previous studies reported that canine DPSCs recapitulate the pluripotency of human DPSCs with the ability to differentiate into osteogenic, adipogenic, and chondrogenic lineages, but not neurogenic lineage (43, 57, 65–67). Apart from that, the osteogenic and adipogenic differentiation potential of canine DPSCs was weaker compared to human DPSCs (43, 63). The RUNX2 expression of human and canine DPSCs on day 7 after osteogenic induction showed ~2.5 and 0.6 times comparing to control group (non-induced group), respectively. For adipogenic differentiation, canine DPSCs showed that fewer formation of intracellular lipid droplets compared to human DPSCs (43, 63). Interestingly, previous studies have shown that human DPSCs can differentiate into insulin-producing cells (IPCs) (68–70). Feline DPSCs have also exhibited MSC properties, with spindle-shaped cells that have the ability to form colonies (71). Like other MSCs, feline DPSCs were also able to differentiate into osteogenic, adipogenic, and chondrogenic. However, the MSC surface marker expression profile of feline DPSCs has not been established.
Similarly, stem cells from human exfoliated deciduous teeth (SHED) have been isolated. One study showed that SHEDs are fibroblast-like shaped multipotent stem cells with proliferative and clonogenic capacity (31). It was noted that the highest number of population doublings was from SHED, followed by human DPSCs and BM-MSCs. Just like other subgroups of MSCs, SHEDs were shown to express CD146 and STRO-1. Additionally, SHEDs can differentiate into odontogenic, osteogenic, adipogenic, and neurogenic lineages in a specific medium. Moreover, odontoblasts were found after SHED transplantation for 8 weeks into immunocompromized mice, being unable to completely regenerate dentin-pulp-like complex, like human DPSCs. Similarly, stem cells derived from canine exfoliated deciduous teeth are termed SCED. One study reported that SCED express CD105 and CD90 but not CD45 (59). Also, SCED were able to differentiate into neurogenic lineage (59). Unfortunately, a literature search did not yield any studies further characterizing SCED. In addition, stem cells from equine and ovine exfoliated deciduous teeth have been reported and showed fibroblastic-like morphology and proliferative property (72, 73). However, stem cells from exfoliated deciduous tooth from feline, porcine, bovine, caprine, non-human primate (NHP), rabbit, rat, and murine have not been reported.
In 1985, progenitor cells that reside in the periodontal ligament of mice were reported (74). The multipotency of PDLSCs was subsequently investigated. In humans, PDLSCs have typical fibroblast-like morphology, can form colonies, and can differentiate into osteoblasts, adipocytes, chondrocytes, and neurogenic (32, 75, 76). Consistent with MSCs, PDLSCs highly expressed CD73, CD90, CD105, and STRO-1 but negatively expressed CD45 (63). Furthermore, an in vivo study of human PDLSCs in combination with hydroxyapatite (HA)/tricalcium phosphate (TCP) showed their ability to differentiate into cementum-like, PDL-like structures and to generate collagen fibers in immunocompromized mice (32). In the canine model, canine PDLSCs exhibited similar morphology and MSCs marker to human PDLSCs. The ability to differentiate into osteogenic, adipogenic, chondrogenic, and neurogenic lineages was also observed in canine PDLSCs (63, 64). However, canine DPSCs have a weaker potential to differentiate into osteoblasts and adipocytes compared to human PDLSCs (64). In addition, canine PDLSCs were reported to have ~2 times lower colony formation than human PDLSCs (63). Otherwise, canine cells combined with HA could still generate cementum-like, PDL-like structures and collagen fibers, similar to human cells (64).
A report of human DFSCs revealed a fibroblast-like morphology. Cells were proliferative and formed colonies. Like with other MSCs, human DFSCs could be induced into osteogenic, adipogenic, and neurogenic lineages (33, 77). Moreover, cells expressed CD29, CD44, CD90, CD105, and CD166, but no hematopoietic markers. In the same study, the authors isolated canine DFSCs and cultured them into cell sheets. However, there are no records regarding the characteristics of canine DFSCs.
Stem cells from apical papilla (SCAP) also exhibited MSC characteristics including fibroblast-like morphology and expression of STRO-1 as well as the other MSCs surface markers, and can differentiate into osteogenic and adipogenic lineages (34). A literature search did not yield any reports of SCAP isolation from canine teeth, however.
Given that GMSCs can be collected relatively easily, human and canine GMSCs were compared for their surface marker expression and osteogenic potential (56). Human GMSCs were found to express CD73, CD90, and CD105 and were able to differentiate into the osteogenic lineage. Conversely, canine GMSCs did not express CD73 or CD105, but did express CD90, and were able to differentiate into the osteogenic lineage. Unlike canine, human GMSCs have been characterized in several studies. One study revealed that human GMSCs are fibroblast-like shaped, and expressing several MSCs surface markers, such as CD44 and CD13, and have the capability to generate osteoblasts, adipocytes, and chondrocytes in vitro (78). Another study reported that human GMSCs proliferation was faster than BM-MSCs without additional growth factors, and cells were found to be clonogenic (79).
Mesenchymal stem cells can be isolated from non-oral tissues, such as bone marrow (BM-MSCs) (61, 65), adipose (AD-MSCs) (61), umbilical cord (UC-MSCs), Wharton's jelly (WJ-MSCs), muscle, and liver. In this review, we focus on BM-MSCs and AD-MSCs.
Collection sites to aspirate BM-MSCs are either the iliac crest, the femoral shaft, or the proximal humerus, both in humans and canines (55, 80–82). As its harvesting protocol is invasive (81, 83), post-operative pain and risk of infection should be well-managed before the procedure is performed (84). Fibroblast-shaped morphology has been observed in human, canine, and feline BM-MSCs (61, 62, 65, 85). Canine BM-MSCs have been shown to express CD73, CD90, and CD146, but not CD45 (61, 62, 65). Humenik et al. demonstrated that canine BM-MSCs also express CD29 (86). Like canine BM-MSCs, human BM-MSCs were shown to express CD73, CD90, CD105, and CD146, but not CD45 (62). In the same study, human and canine BM-MSCs were also found to be clonogenic. Unlike canine and human BM-MSCs, feline BM-MSCs were only expressed CD9, CD44, and MHC1 as MSC surface markers (85). The mRNA expression of SOX2, NANOG, and OCT4 as pluripotency genes was detected in both human and canine BM-MSCs (41, 87). Moreover, we have found that canine, feline, and human BM-MSCs are able to differentiate into many lineages, such as osteogenic, adipogenic, and chondrogenic (41, 55, 61, 65, 85, 88). In addition, both human and feline BM-MSCs are able to differentiate into neurogenic lineage (41, 85). A collective analysis mentioned that BM-MSCs across species have differences and uniqueness of stemness characteristics. To implement this result, dissemination of stemness characteristics is required for further clinical application. This collective analysis of BM-MSCs has been reported in the previous publication (81).
Previous studies have noted that human and canine AD-MSCs exhibit the same fibroblast-like morphology as BM-MSCs (61, 89). Human AD-MSCs can be isolated from neck or abdominal adipose tissues (89). In canines, adipose tissue can be obtained from subcutaneous, omental, and inguinal deposits and even biopsied fat (58, 61). Like BM-MSCs, canine AD-MSCs are able to express MSCs surface markers, such as CD90 and CD73, but not hematopoietic stem cells (HSCs) marker CD45 (61). Moreover, canine AD-MSCs are considered pluripotent based on protein expression of Oct4, Nanog, and Sox2 expressions (58). Like canine AD-MSCs, human AD-MSCs have been shown to express CD90, CD105, CD44, and CD73, but not CD45 (89). The same study found that human AD-MSCs expressed proteins such as Nanog, Sox2, and SSEA4, like canine AD-MSCs. In addition, human and canine AD-MSCs can differentiate into osteogenic, adipogenic, and chondrogenic lineages (58, 61, 89). Other studies have also reported that canine AD-MSCs have the ability to differentiate into IPCs (54, 61). It has been shown that AD-MSCs can also be isolated from felines (90, 91). These studies have noted that cell morphology was similar to other species and were proliferative, and that cells expressed CD9, CD44, CD90, and CD105 and could differentiate into osteogenic, adipogenic, and chondrogenic lineages (90, 91). To date, there is no data to provide collective analysis of AD-MSCs.
Of note, some studies include BM-MSCs and AD-MSCs as MSCs of oral-origin. Indeed, mandibles represent a potential collection site (29). Similarly, MSCs have been collected from neck fat in equines and the corresponding MSC characteristics and wound healing potential have been documented (92). A comprehensive summary of oral and non-oral potential cell sources from human, canine, feline, and equines is presented in Table 1 and livestock and laboratory animals are presented in Tables 2, 3, respectively.
Scaffolds and signaling molecules have been comprehended as other essential components of tissue engineering to regenerate damaged tissues or organs (2). Scaffold is defined as a three-dimensional (3D) biomaterial for supporting the cells to proliferate and differentiate during the process of tissue regeneration (9). Furthermore, signaling molecules are crucial in the involvement of cellular responses (154). Thus, besides stem cells, scaffolds and signaling molecules are suggested to have the potential to be used for OMF reconstruction (155).
Selecting an appropriate scaffold for each specific tissue is an essential factor in tissue engineering. The biomaterial and scaffold are a transport device for cells and signaling molecules to promote tissue regeneration (9, 156, 157). Moreover, the scaffold structure functions as a reservoir for water, nutrients, cytokines, and growth/differentiation factors (27). Therefore, scaffold fabrication should be considered for its functionality, biocompatibility, biodegradability, mechanical properties, and structure characteristics such as pore size, porosity, and interconnectivity (158, 159). Addition, the 3D structural scaffold can be printed to resemble donor tissue architecture (9). Also, the designated material is expected to support cell attachment, migration, proliferation, differentiation, maturation, and ECM production (19, 160).
Scaffold materials can be broadly categorized into natural, and synthetic (157). Biomaterials can be classified into metal, ceramic, polymer, and composite (161). The advantages and disadvantages of each material should be taken into consideration before utilizing them for OMF hard or soft tissue regeneration and based on the desired functionality of the material on a specific tissue.
As for scaffold-free approaches, techniques include self-organization (cell sheet and aggregate engineering) and self-assembly processes. Interestingly, scaffold-free approaches can be applied for OMF regeneration, especially for the indication of skin, TMJ disc, cartilage, bone, and periodontal ligament tissues (7, 8, 162).
Titanium alloy prostheses have been of interest for OMF hard tissue repair applications (163). However, this material often leads to local tissue damage and chronic inflammatory response due to poor biomechanical properties and low biocompatibility (164) and other materials that may exhibit superior qualities as well as practical advantages and disadvantages are available. In this regard, two important properties to consider are the osteoinductive and osteoconductive potential of the chosen scaffold material especially when applied for bone regeneration purpose (83).
Other materials that can be used for bone regeneration include ceramics, synthetic polymers, and natural polymers. Natural polymer-based scaffolds are known to be biologically active and able to enhance cell adhesion and growth. Despite these properties, limitations in fabrication and inferior mechanical characteristics represent mechanical property constraints compared with other scaffold types, resulting in major disadvantages of this type of scaffold for bone regeneration (157, 165, 166). One study reported starch as natural polymer could not be used directly due to its poor stability and mechanical properties. Improvement of mechanical properties was gained by reinforcement with HA as composite (167). Examples of natural polymers employed for bone tissue regeneration include collagen, gelatin, chitosan, alginate, silk proteins, hyaluronic acid, fibrin, and keratin (168). In contrast, synthetic polymers exhibit elastic properties, endless forms, established structures, and comparable characteristics to biological tissues that are predictable and reproducible (169). However, the main disadvantages of synthetic biomaterials are lack of cell adhesion sites, the need to chemically modify them to improve cell adhesion, and some materials produce toxic by-products for surrounding cells and tissues (157, 169). Biodegradable synthetic materials that are popular for bone tissue engineering include polylactic acid (170), polyglycolic acid (PGA), polycaprolactone (PCL), poly(lactic-co-glycolic) acid (PLGA), and polyurethane (PU) (169). Lastly, ceramics are biocompatible and suitable for replacing hard tissues, but their brittleness represents a drawback. Common ceramic scaffolds used for bone regeneration are HA and TCP (171). Some of the disadvantages of ceramic scaffolds can be minimized by fusing one specific type of material with another to generate a hybrid with enhanced regenerative potential (172).
Oral and maxillofacial soft tissue engineering is meant to reconstruct lip, skin, salivary gland, oral mucosa, muscle, ligament, and TMJ-related tissues, or periodontal tissues. Soft tissue engineering facilitates defect reconstruction for soft tissues (173). Unlike hard tissue, scaffold materials for soft tissues vary in their flexibility (9). Since vascularization has been the central issue of soft tissue engineering, a strong blood supply must be achieved for successful soft tissue reconstruction (173). Thus, scaffolds are required to be able to carry blood supply via vascular ingrowth. Additionally, engineered scaffolds should be adjusted to shape and size of the defect and accommodate the appropriate cells, thus sensory and motoric functions of the damaged tissues would be regained (173).
Several materials have been reported for use in OMF soft tissue engineering. Hydrogels (HG) play a prominent role given that they can be generated from various materials, such as chitosan, chitin, hyaluronic acid, gelatin, peptide, PLGA, and PEG. One study mentioned that HG is preferable for soft tissue but not hard tissue reconstruction because its mechanical properties do not support load bearing (174). Despite that, a recent review noted that HG can regenerate periodontal soft and hard tissue as well as dental pulp, dentine, and enamel (175). Another study showed that commercial biodegradable scaffolds made of either a combination of collagen fibers porous matrix and glycosaminoglycan, polyglycin 910, or collagen membrane and collagen I, can generate an oral mucosa equivalent (OME) in vitro (176). Moreover, a silk fibroin/hyaluronic acid-based scaffold has been suggested for soft tissue regeneration (177). Silk fibroin has also been combined with gelatin and chitosan into a mimicked scaffold in vitro using human keratinocyte cells for OMF soft tissue engineering applications (178). In addition, a previous study explored the potential of polyglycolic acid polymer scaffolds for salivary gland tissue engineering (179). To be used in the veterinary medicine field, such materials should meet general consideration from material selection, design and manufacturing, design control and testing, to safety and efficacy before going to the clinical studies to meet the product purpose (180).
In this review, we have included cartilage as a soft tissue pertinent to the OMF region, specifically at the TMJ. Like scaffolds in general, the fabrication of a scaffold for cartilage should take into consideration its composition and biological properties, its architecture, and its mechanical and degradation (181). Materials that have been studied for TMJ regeneration, which are intended to mimic both cartilage and bone tissues, include PLA disc (182), PGA (183), chitosan/alginate (184), fibrin/chitosan (185), biphasic scaffolds of HA/TCP and hyaluronic acid (170), and PGA and PLGA/polyethylene glycol (PEG) (186).
Signaling molecules represent a crucial aspect when developing strategies for OMF tissue engineering. These molecules refer to growth factors, which are proteins produced by the cells (27). Current technologies allow the production of growth factors using recombinant methods. By binding to their receptors, signaling molecules activate intracellular signaling events that can trigger or inhibit cell adhesion, proliferation, migration, and differentiation. Thus, this approach gives rise to regenerating damaged tissue. Moreover, signaling molecules can be applied as a single treatment and adsorbed on a scaffold, as previously mentioned above. This combination enhances the integration between the material and host tissues (27).
Many signaling molecules have been investigated for their therapeutical potential in context of OMF regeneration, and even, some are clinically approved by the Food and Drug Administration (187). Several well-known signaling molecules include bone morphogenetic proteins (BMPs), transforming growth factor-β (TGF-β), platelet-derived growth factor (PDGF), fibroblast growth factor (FGF), vascular endothelial growth factor (VEGF), and insulin-like growth factor (IGF) (188). Among these, BMPs are the most widely studied for bone regeneration, and several types of BMPs have been mentioned to have crucial roles in bone morphogenesis and bone defect repair and regeneration (189, 190). Interestingly, rhBMP-2 and rhBMP-7 have been approved by the Food and Drug Administration (187) for bone regeneration applications (189, 190). In general, BMPs are considered very relevant for OMF regeneration (191), being capable of osteoinductive on MSCs (190). On the other hand, TGF-β has also been proposed as a potent modulator of bone regeneration with the ability to enhancing osteogenesis at a low concentration (192). Paradoxically, TGF-β at a high concentration inhibits osteogenesis. Another molecule called teriparatide has also been of interest to OMF surgeons. Teriparatide is a recombinant parathyroid hormone used to treat osteoporosis (193). Moreover, teriparatide is approved by the FDA for treatment of osteoporosis and has been reported to regenerate jawbone defects (194). Finally, the potential of leptin to enhance oral mucosa regeneration by increasing vascularization has been reported (195).
Animal experiment models have been introduced to study the translational treatment of OMF tissue engineering in clinical practice by mimicking real clinical situations. In this review, we provided most of the in vivo studies employing non-human MSCs and successful treatment in veterinary patients with OMF defects. The data are expected to be used for further translation to treat OMF defects in veterinary patients.
Periodontal tissue regeneration in periodontitis-induced beagles has been reported to using rhBMP-2 adsorbed on PLGA-gelatin sponge carrier material treatment (196). Another report demonstrated that autologous canine DPSCs combined with a commercial xenograft scaffold in a periodontitis-induced canine model had the ability to achieve periodontal tissue regeneration after 8 weeks of transplantation (197).
Another published study performed mandibular osteotomy in beagles to create a bone defect and treated it with a 3D-printed HA scaffold co-cultured with canine BM-MSCs (164). They found that the seeded cells played an essential role in large bone defects by differentiating into osteoblasts, regulating immune response, and providing a microenvironment for tissue regeneration. Also, De kok et al. reported that canine BM-MSCs enhanced bone formation when combined with a HA/TCP scaffold in an alveolar defect canine model (198). In addition, SHEDs have been transplanted with a scaffold in a mandibular defect canine model (199). However, this study showed no significant difference in bone regeneration compared with the control group. Nevertheless, the authors demonstrated no tumorigenesis or severe inflammation after transplantation (182, 200).
Evaluation of maxillary sinus augmentation (MSA) has also been performed in canine by reconstructing it with autologous osteoblasts on a β-TCP scaffold (201). It was shown that the addition of osteoblasts to the scaffold improved maxillary regeneration by the remarkable differences of height and volume of augmented maxillary sinus compared to groups with scaffold only and autogenous bone graft (201).
Allogenic transplantation for pulp regeneration study has been reported using the canine model. After pulpectomy, canine DPSCs transplantation was conducted in dogs with consideration given matched and mismatched dog leukocyte antigen (DLA). The authors revealed that the both DLA matched and mismatched allogenic transplantation are safe and effective for pulp regeneration due to the lack of signs for toxicity and the generation of pulp-like tissues 12 weeks post-transplantation (202). The results are supported by another study revealing canine DPSCs do not express HLA-DR (similar to DLA Class II in dogs) by flow cytometry (49). In addition, one study explored the blood type- and breed-associated immune reaction on allogenic transplantation in equines. The authors revealed that universal blood donor-type of Standardbred has been suggested to be less likely occurred in a study of equine BM-MSCs with low expression of MHC Class II (similar to DLA Class II in dogs) than non-blood donor type and in Thoroughbred. This study implies that breed of donor should be considered with the use of universal blood donor-type (203).
Regarding soft tissue, Qian et al. revealed that combining leptin, silk fibrin, and polydopamine improves oral mucosa healing and triggers blood vessel regeneration in New Zealand rabbits (195). In addition, regeneration of oral mucosa has been previously simulated in nude mice with skin defects implanted with a human gingival endothelial cells (HGECs)-human gingival fibroblasts (HGFs)-vascular endothelial cells (VECs)-acellular vascular matrix (ACVM)-0.25% human-like collagen I (HLC-I) complex (204). In such study, investigators found that the scaffold complex had a regenerative effect based on the presence of epithelioid-like, lamina propria-like, and vascular-like structures observed on histopathological analysis.
As for the salivary gland, one study showed that 3D hyaluronic acid-based hydrogel scaffold implantation in rats was able to adhere around the parotid tissue (205). Interestingly, the implanted scaffold seeded with human salivary cells was able to retain its spheroid structure and express CD44 and receptor for hyaluronan-mediated motility (RHAMM/CD168; a progenitor cell marker). Another study has shown that human salivary cells seeded on unwoven sheets of polyglycolic acid polymers are able to form functional tissues in mice (179). These two studies indicate that both scaffolds have potential for salivary gland regeneration.
In vivo TMJ studies have been reported. Replacement of TMJ disc in New Zealand rabbits with PLA discs containing autologous AD-MSCs was demonstrated (182). The authors presented that the material has the potential for further use yet needs more dissemination. In addition, a porcine urinary bladder matrix (UBM) scaffold was implanted in the TMJ in a dog model (200). They found that the material was inductive and suggested that an extracellular matrix (ECM)-based scaffold can be a solution for TMJ disc replacement. In addition, a study of scaffold-free TMJ implant from costal chondrocytes has been reported in the minipigs as TMJ disc regeneration model. The study found that the implants are safe, able to prevent degeneration of disc thinning, and even, regenerate the defects by refining the osteoarthritis score for the efficacy (162).
Despite the positive outcomes of the cell-based treatment, there has been reports that MSCs provide no clinical improvement in clinical cases. Autologous bone marrow aspirate concentrate, adipose stromal vascular fraction, and allogeneic human umbilical cord tissue-derived MSCs (UC-MSCs) showed no significant difference on beneficial outcomes for osteoarthritis in a phase 3 trial comparing with conventional therapy, corticosteroid injection (CSI) (206). A meta-analysis report also showed that stem cell infusion did not result in clinical improvement for acute myocardial infarction (207). According to the reports, such strategies could be made starting from identifying the possibilities of non-beneficial outcomes, re-designing the experiment, and evaluating the new strategy.
Some of the previously mentioned in vivo studies and corresponding results offer the opportunity to leverage tissue engineering solutions to repair OMF defects in veterinary patients. Tissue engineering is a multidisciplinary field consisting of medicine, material science, engineering, and biology. The prospective tissue-engineered products could be either comprising of cells, signaling molecules, scaffolds, or combination of scaffold with cells, or signaling molecules (208). Thus, according to the medical product classification by FDA, the products are either biological, medical device, or combination of biological and medical device. In addition, to prove the safety and efficacy in the clinical case, such studies should be performed as well. Indeed, several case studies have been reported in the veterinary literature illustrating broad potential for clinical use (209, 210).
In one case reported by Spector and Boudrieau (210), a partial mandibulectomy performed in a cocker spaniel to treat an odontoma resulted in a large 5-cm defect impacted functionality and quality of life. The defect was reconstructed using rhBMP-2 delivered in an absorbable collagen sponge containing HA/TCP granules (compression resistant matric [CRM]) and plate fixation. The results demonstrated that the correction was successful without any significant complications, either in functional or cosmetic aspects, after 36 months. The authors also suggested that rhBMP-2 can be an alternative to reconstructive techniques for dog use, increasing cost efficiency by using a lower dose than in humans. This initial methodology was further described and expanded on. Case series of segmental mandibulectomies and bilateral rostral mandibular reconstruction and chronic, defect union fracture treatment have been reported to be resolved by the use of plate and CRM infused with rhBMP-2 (211–213). Additionally, Tsugawa et al. reported a retrospective study of the promising mentioned methodology for canine acanthomatous ameloblastoma (CAA) (214). The studies showed that margin between implant material and native bone become indistinct at week 4 or later post-operatively without relatively minimum complications. Similarly, autologous and allogeneic mesenchymal stem cells derived from adipose tissue were reported for clinical use in a sample of cats with refractory feline chronic gingivostomatitis (215, 216). These studies suggest that both cell sources are relatively safe and potentially effective even though treatments were not compared to other types of intervention.
An interesting clinical application of a 3D-printed PCL/β-TCP scaffold has also been reported in a maxillary bone defect due to an oral squamous cell carcinoma in a 12-year-old female mixed breed dog (209). Reconstruction was successful based on computed tomography images 2 months after surgery.
Several animal studies were presented with beneficial outcomes of investigated technologies. However, some products are not intended for veterinary patients (179, 195, 196, 199–201, 204, 205). Given the high success rates of OMF tissue engineering reported in experimental animal models and selected clinical cases, tissue engineering for OMF defect repair appears to be a viable alternative in veterinary medicine. To a greater extent, proposed and safety- and efficacy-proven OMF tissue engineering products can be registered for an intellectual property (IP) for veterinary patients.
Despite the immense potential of tissue engineering for OMF defect repair in veterinary medicine, several milestones and challenges lie ahead (Figure 2). Firstly, generating ideas by gathering information on clinically relevant OMF diseases, identifying proper funding mechanisms to conduct pertinent bench and clinical research, and conducting market analysis to explore translational feasibility. Similarly, the potential of stem cells from one specific source and species origin should be further investigated. Indeed, cells need to be more exhaustively characterized. This can be following from the proposed mechanisms of MSCs in differentiation to the targeted tissue, immunomodulation property, and as endocrine secretors (217, 218). Such characterization and in vivo experiments are essential because they will determine cell potency. In vivo studies related to OMF surgery can be performed following the treatment interest since one type of cell may have different potential with another cell in terms of kind of treatment. The topic has been raised by a previous study (219) and also applies to scaffolds and signaling molecules. In addition, tissue engineering as personalized medicine should be considered as well with the 3D printing technologies to adapt with the patients' needs (220). Research on the immune response after transplantation using adequate animal models is also required. Rejection of a transplant may occur due to several factors, such as the source of the transplant (i.e., auto-, allo-, or xenogeneic), dose, and route of administration. Moreover, any pre-clinical experiments need to be performed considering any human resources, facilities, and budget available. Before implementing novel therapies, properly designed clinical trials should be conducted. Since clinical studies require blinded and randomized interventions, sample sizes must be met to ensure robust and reproducible results, which is often a challenge in veterinary medicine. Moreover, any eventual translation of interventions into clinical practice would require building up clients' trust and very likely third-party involvement. Finally, Ivanoska et al. have noted logistical challenges including product transport and cell delivery (219).
Based on the experimental and clinical studies, regenerative tissue engineering is a promising approach with immense veterinary translational potential for the reconstruction of OMF defects in animals. However, the types of tissue engineering components should be chosen appropriately based on past, current, and future research in order to achieve optimal outcomes based on tissue regeneration.
SDP: Writing—original draft. TT: Writing—original draft. MP: Writing—original draft. TH: Writing—original draft. SP: Writing—review & editing. NF: Writing—review & editing. CS: Writing—review & editing. SR: Writing—review & editing.
The author(s) declare that financial support was received for the research, authorship, and/or publication of this article. SR was supported by the Grants for Development of New Faculty Staff, Ratchadaphiseksomphot Fund, Chulalongkorn University, Thailand-Science research and Innovation Fund Chulalongkorn University, and the Research Grant, Faculty of Veterinary Science, Chulalongkorn University.
The authors declare that the research was conducted in the absence of any commercial or financial relationships that could be construed as a potential conflict of interest.
The author(s) declared that they were an editorial board member of Frontiers, at the time of submission. This had no impact on the peer review process and the final decision.
All claims expressed in this article are solely those of the authors and do not necessarily represent those of their affiliated organizations, or those of the publisher, the editors and the reviewers. Any product that may be evaluated in this article, or claim that may be made by its manufacturer, is not guaranteed or endorsed by the publisher.
1. Winer JN, Verstraete FJ, Cissell DD, Lucero S, Athanasiou KA, Arzi B. The application of 3-dimensional printing for preoperative planning in oral and maxillofacial surgery in dogs and cats. Vet Surg. (2017) 46:942–51. doi: 10.1111/vsu.12683
2. Langer R, Vacanti JP. Tissue engineering. Science. (1993) 260:920–6. doi: 10.1126/science.8493529
3. Krafts KP. Tissue repair: the hidden drama. Organogenesis. (2010) 6:225–33. doi: 10.4161/org.6.4.12555
4. Fertala J, Wang ML, Rivlin M, Beredjiklian PK, Abboud J, Arnold WV, et al. Extracellular targets to reduce excessive scarring in response to tissue injury. Biomolecules. (2023) 13:758. doi: 10.3390/biom13050758
5. Oakes S, Kumar V, Abbas A, Aster J. Inflammation and Repair. Robbins and Cotran's Pathologic Basis of Disease. 10th ed. Amsterdam: Elsevier (2020). p. 75–6.
6. Uccioli L. A clinical investigation on the characteristics and outcomes of treating chronic lower extremity wounds using the tissuetech autograft system. Int J Low Extrem Wounds. (2003) 2:140–51. doi: 10.1177/1534734603258480
7. De Pieri A, Rochev Y, Zeugolis DI. Scaffold-free cell-based tissue engineering therapies: advances, shortfalls and forecast. NPJ Regener Med. (2021) 6:18. doi: 10.1038/s41536-021-00133-3
8. DuRaine GD, Brown WE, Hu JC, Athanasiou KA. Emergence of scaffold-free approaches for tissue engineering musculoskeletal cartilages. Ann Biomed Eng. (2015) 43:543–54. doi: 10.1007/s10439-014-1161-y
9. Howard D, Buttery LD, Shakesheff KM, Roberts SJ. Tissue engineering: strategies, stem cells and scaffolds. J Anat. (2008) 213:66–72. doi: 10.1111/j.1469-7580.2008.00878.x
10. Kulus M, Kulus J, Jankowski M, Borowiec B, Jeseta M, Bukowska D, et al. The use of mesenchymal stem cells in veterinary medicine. Med J Cell Biol. (2018) 6:101–7. doi: 10.2478/acb-2018-0016
11. Huang G-J, Gronthos S, Shi S. Mesenchymal stem cells derived from dental tissues vs. those from other sources: their biology and role in regenerative medicine. J Dental Res. (2009) 88:792–806. doi: 10.1177/0022034509340867
12. Gioso MA, Carvalho VG. Oral anatomy of the dog and cat in veterinary dentistry practice. Vet Clin. (2005) 35:763–80. doi: 10.1016/j.cvsm.2004.10.003
13. Gupta AD, Verma A, Islam JI, Agarwal S. Maxillofacial defects and their classification: a review. Int J Adv Res. (2016) 4:6. doi: 10.21474/IJAR01/618
14. De Paolo MH, Arzi B, Pollard RE, Kass PH, Verstraete FJ. Craniomaxillofacial trauma in dogs-part I: fracture location, morphology and etiology. Front Vet Sci. (2020) 7:241. doi: 10.3389/fvets.2020.00241
15. De Paolo MH, Arzi B, Pollard RE, Kass PH, Verstraete FJ. Craniomaxillofacial trauma in dogs-part II: association between fracture location, morphology and etiology. Front Vet Sci. (2020) 7:242. doi: 10.3389/fvets.2020.00242
16. Peralta S, Fiani N, Kan-Rohrer KH, Verstraete FJ. Morphological evaluation of clefts of the lip, palate, or both in dogs. Am J Vet Res. (2017) 78:926–33. doi: 10.2460/ajvr.78.8.926
17. Wolf ZT, Brand HA, Shaffer JR, Leslie EJ, Arzi B, Willet CE, et al. Genome-wide association studies in dogs and humans identify ADAMTS20 as a risk variant for cleft lip and palate. PLoS Genet. (2015) 11:e1005059. doi: 10.1371/journal.pgen.1005059
18. Zacher AM, Marretta SM. Oral and maxillofacial surgery in dogs and cats. Vet Clin. (2013) 43:609–49. doi: 10.1016/j.cvsm.2013.02.010
19. Johnson H, Gallicchio V. Stem cells and tissue engineering: an overview of modern advances and discoveries related to stem cells that contribute to replacement of native tissue. Madridge J Clin Res. (2017) 1:20–6. doi: 10.18689/mjcr-1000104
20. Nkenke E, Neukam FW. Autogenous bone harvesting and grafting in advanced jaw resorption: morbidity, resorption and implant survival. Eur J Oral Implantol. (2014) 7:S203–S17.
21. Tsiklin IL, Shabunin AV, Kolsanov AV, Volova LT. In vivo bone tissue engineering strategies: advances and prospects. Polymers. (2022) 14:3222. doi: 10.3390/polym14153222
22. Peralta S, Nemec A, Fiani N, Verstraete FJ. Staged double-layer closure of palatal defects in 6 dogs. Vet Surg. (2015) 44:423–31. doi: 10.1111/j.1532-950X.2014.12131.x
23. Peralta S, Campbell RD, Fiani N, Kan-Rohrer KH, Verstraete FJ. Outcomes of surgical repair of congenital palatal defects in dogs. J Am Vet Med Assoc. (2018) 253:1445–51. doi: 10.2460/javma.253.11.1445
24. Bar-Am Y, Verstraete FJ. Elastic training for the prevention of mandibular drift following mandibulectomy in dogs: 18 cases (2005-2008). Vet Surg. (2010) 39:574–80. doi: 10.1111/j.1532-950X.2010.00703.x
25. Boudrieau RJ, Mitchell SL, Seeherman H. Mandibular reconstruction of a partial hemimandibulectomy in a dog with severe malocclusion. Vet Surg. (2004) 33:119–30. doi: 10.1111/j.1532-950X.2004.04019.x
26. Suh H. Tissue restoration, tissue engineering and regenerative medicine. Yonsei Med J. (2000) 41:681–4. doi: 10.3349/ymj.2000.41.6.681
27. Melek LN. Tissue engineering in oral and maxillofacial reconstruction. Tanta Dental J. (2015) 12:211–23. doi: 10.1016/j.tdj.2015.05.003
28. Nguyen A, Nguyen V, James AW, Scott MA. Craniomaxillofacial sources of mesenchymal stem cells: a brief review. Int J Orthopaed. (2015) 2:333–40. doi: 10.17554/j.issn.2311-5106.2015.02.85
29. Zhao H, Chai Y. Stem cells in teeth and craniofacial bones. J Dent Res. (2015) 94:1495–501. doi: 10.1177/0022034515603972
30. Gronthos S, Mankani M, Brahim J, Robey PG, Shi S. Postnatal human dental pulp stem cells (DPSCs) in vitro and in vivo. Proc Nat Acad Sci USA. (2000) 97:13625–30. doi: 10.1073/pnas.240309797
31. Miura M, Gronthos S, Zhao M, Lu B, Fisher LW, Robey PG, et al. SHED: stem cells from human exfoliated deciduous teeth. Proc Nat Acad Sci USA. (2003) 100:5807–12. doi: 10.1073/pnas.0937635100
32. Seo B-M, Miura M, Gronthos S, Bartold PM, Batouli S, Brahim J, et al. Investigation of multipotent postnatal stem cells from human periodontal ligament. Lancet. (2004) 364:149–55. doi: 10.1016/S0140-6736(04)16627-0
33. Morsczeck C, Götz W, Schierholz J, Zeilhofer F, Kühn U, Möhl C, et al. Isolation of precursor cells (PCs) from human dental follicle of wisdom teeth. Matrix Biol. (2005) 24:155–65. doi: 10.1016/j.matbio.2004.12.004
34. Sonoyama W, Liu Y, Fang D, Yamaza T, Seo B-M, Zhang C, et al. Mesenchymal stem cell-mediated functional tooth regeneration in swine. PLoS ONE. (2006) 1:e79. doi: 10.1371/journal.pone.0000079
35. Fawzy El-Sayed KM, Dörfer CE. Gingival mesenchymal stem/progenitor cells: a unique tissue engineering gem. Stem Cells Int. (2016) 2016:7154327. doi: 10.1155/2016/7154327
36. Aly LAA. Stem cells: sources, and regenerative therapies in dental research and practice. World J Stem Cells. (2015) 7:1047. doi: 10.4252/wjsc.v7.i7.1047
37. Bansal R, Jain A. Current overview on dental stem cells applications in regenerative dentistry. J Nat Sci Biol Med. (2015) 6:29. doi: 10.4103/0976-9668.149074
38. Sunil P, Manikandhan R, Muthu M, Abraham S. Stem cell therapy in oral and maxillofacial region: an overview. J Oral Maxillof Pathol. (2012) 16:58. doi: 10.4103/0973-029X.92975
39. Dominici M, Le Blanc K, Mueller I, Slaper-Cortenbach I, Marini F, Krause D, et al. Minimal criteria for defining multipotent mesenchymal stromal cells. The International Society for Cellular Therapy position statement. Cytotherapy. (2006) 8:315–7. doi: 10.1080/14653240600855905
40. Bhandari DR, Seo K-W, Roh K-H, Jung J-W, Kang S-K, Kang K-S. REX-1 expression and p38 MAPK activation status can determine proliferation/differentiation fates in human mesenchymal stem cells. PLoS ONE. (2010) 5:e10493. doi: 10.1371/journal.pone.0010493
41. Greco SJ, Liu K, Rameshwar P. Functional similarities among genes regulated by OCT4 in human mesenchymal and embryonic stem cells. Stem Cells. (2007) 25:3143–54. doi: 10.1634/stemcells.2007-0351
42. Wang Y, Tu W, Lou Y, Xie A, Lai X, Guo F, et al. Mesenchymal stem cells regulate the proliferation and differentiation of neural stem cells through Notch signaling. Cell Biol Int. (2009) 33:1173–9. doi: 10.1016/j.cellbi.2009.08.004
43. Dissanayaka WL, Zhu X, Zhang C, Jin L. Characterization of dental pulp stem cells isolated from canine premolars. J Endod. (2011) 37:1074–80. doi: 10.1016/j.joen.2011.04.004
44. Maleki M, Ghanbarvand F, Behvarz MR, Ejtemaei M, Ghadirkhomi E. Comparison of mesenchymal stem cell markers in multiple human adult stem cells. Int J Stem Cells. (2014) 7:118–26. doi: 10.15283/ijsc.2014.7.2.118
45. Moraes DA, Sibov TT, Pavon LF, Alvim PQ, Bonadio RS, Da Silva JR, et al. A reduction in CD90 (THY-1) expression results in increased differentiation of mesenchymal stromal cells. Stem Cell Res Ther. (2016) 7:1–14. doi: 10.1186/s13287-016-0359-3
46. Ode A, Kopf J, Kurtz A, Schmidt-Bleek K, Schrade P, Kolar P, et al. CD73 and CD29 concurrently mediate the mechanically induced decrease of migratory capacity of mesenchymal stromal cells. Eur Cells Mater. (2011) 22:26–42. doi: 10.22203/eCM.v022a03
47. Ogata K, Satoh C, Tachibana M, Hyodo H, Tamura H, Dan K, et al. Identification and hematopoietic potential of CD45– clonal cells with very immature phenotype (CD45– CD34– CD38– Lin–) in patients with myelodysplastic syndromes. Stem Cells. (2005) 23:619–30. doi: 10.1634/stemcells.2004-0280
48. Tan K, Zhu H, Zhang J, Ouyang W, Tang J, Zhang Y, et al. CD73 expression on mesenchymal stem cells dictates the reparative properties via its anti-inflammatory activity. Stem Cells Int. (2019) 2019:8717694. doi: 10.1155/2019/8717694
49. Utumi PH, Fracaro L, Senegaglia AC, Fragoso FYI, Miyasaki DM, Rebelatto CLK, et al. Canine dental pulp and umbilical cord-derived mesenchymal stem cells as alternative sources for cell therapy in dogs. Res Vet Sci. (2021) 140:117–24. doi: 10.1016/j.rvsc.2021.08.006
50. Balic A, Mina M. Characterization of progenitor cells in pulps of murine incisors. J Dent Res. (2010) 89:1287–92. doi: 10.1177/0022034510375828
51. Cheng P-H, Snyder B, Fillos D, Ibegbu CC, Huang AH-C, Chan AW. Postnatal stem/progenitor cells derived from the dental pulp of adult chimpanzee. BMC Cell Biol. (2008) 9:1–11. doi: 10.1186/1471-2121-9-20
52. Zheng Y, Liu Y, Zhang C, Zhang H, Li W, Shi S, et al. Stem cells from deciduous tooth repair mandibular defect in swine. J Dent Res. (2009) 88:249–54. doi: 10.1177/0022034509333804
53. Mensing N, Gasse H, Hambruch N, Haeger J-D, Pfarrer C, Staszyk C. Isolation and characterization of multipotent mesenchymal stromal cells from the gingiva and the periodontal ligament of the horse. BMC Vet Res. (2011) 7:1–13. doi: 10.1186/1746-6148-7-42
54. Dang Le Q, Rodprasert W, Kuncorojakti S, Pavasant P, Osathanon T, Sawangmake C. In vitro generation of transplantable insulin-producing cells from canine adipose-derived mesenchymal stem cells. Sci Rep. (2022) 12:1–18. doi: 10.1038/s41598-022-13114-3
55. Drela K, Stanaszek L, Snioch K, Kuczynska Z, Wrobel M, Sarzynska S, et al. Bone marrow-derived from the human femoral shaft as a new source of mesenchymal stem/stromal cells: an alternative cell material for banking and clinical transplantation. Stem Cell Res Ther. (2020) 11:1–13. doi: 10.1186/s13287-020-01697-5
56. El-Bialy T, Kucharski C, Farid M, Ghaffar K, Fawzi E. Human and dogs' gingival stem cells are different. Cell Stem Cells Regen Med. (2015) 1:103. doi: 10.16966/2472-6990.103
57. Gronthos S, Brahim J, Li W, Fisher L, Cherman N, Boyde A, et al. Stem cell properties of human dental pulp stem cells. J Dent Res. (2002) 81:531–5. doi: 10.1177/154405910208100806
58. Neupane M, Chang C-C, Kiupel M, Yuzbasiyan-Gurkan V. Isolation and characterization of canine adipose-derived mesenchymal stem cells. Tissue Eng A. (2008) 14:1007–15. doi: 10.1089/ten.tea.2007.0207
59. Prado C, Fratini P, Matias GdSS, Bocabello RZ, Monteiro J, dos Santos Jr CJ, et al. Combination of stem cells from deciduous teeth and electroacupuncture for therapy in dogs with chronic spinal cord injury: a pilot study. Res Vet Sci. (2019) 123:247–51. doi: 10.1016/j.rvsc.2019.01.011
60. Purwaningrum M, Giachelli CM, Osathanon T, Rattanapuchpong S, Sawangmake C. Dissecting specific Wnt components governing osteogenic differentiation potential by human periodontal ligament stem cells through interleukin-6. Sci Rep. (2023) 13:9055. doi: 10.1038/s41598-023-35569-8
61. Rodprasert W, Nantavisai S, Pathanachai K, Pavasant P, Osathanon T, Sawangmake C. Tailored generation of insulin producing cells from canine mesenchymal stem cells derived from bone marrow and adipose tissue. Sci Rep. (2021) 11:1–17. doi: 10.1038/s41598-021-91774-3
62. Rozemuller H, Prins H-J, Naaijkens B, Staal J, Bühring H-J, Martens AC. Prospective isolation of mesenchymal stem cells from multiple mammalian species using cross-reacting anti-human monoclonal antibodies. Stem Cells Dev. (2010) 19:1911–21. doi: 10.1089/scd.2009.0510
63. Wang W, Yuan C, Liu Z, Geng T, Li X, Wei L, et al. Characteristic comparison between canine and human dental mesenchymal stem cells for periodontal regeneration research in preclinical animal studies. Tissue Cell. (2020) 67:101405. doi: 10.1016/j.tice.2020.101405
64. Wang WJ, Zhao YM, Lin BC, Yang J, Ge LH. Identification of multipotent stem cells from adult dog periodontal ligament. Eur J Oral Sci. (2012) 120:303–10. doi: 10.1111/j.1600-0722.2012.00975.x
65. Nantavisai S, Pisitkun T, Osathanon T, Pavasant P, Kalpravidh C, Dhitavat S, et al. Systems biology analysis of osteogenic differentiation behavior by canine mesenchymal stem cells derived from bone marrow and dental pulp. Sci Rep. (2020) 10:1–18. doi: 10.1038/s41598-020-77656-0
66. Sawangmake C, Nowwarote N, Pavasant P, Chansiripornchai P, Osathanon T. A feasibility study of an in vitro differentiation potential toward insulin-producing cells by dental tissue-derived mesenchymal stem cells. Biochem Biophys Res Commun. (2014) 452:581–7. doi: 10.1016/j.bbrc.2014.08.121
67. Yu J, He H, Tang C, Zhang G, Li Y, Wang R, et al. Differentiation potential of STRO-1+ dental pulp stem cells changes during cell passaging. BMC Cell Biol. (2010) 11:1–7. doi: 10.1186/1471-2121-11-32
68. Kuncorojakti S, Rodprasert W, Le QD, Osathanon T, Pavasant P, Sawangmake C. In vitro induction of human dental pulp stem cells toward pancreatic lineages. J Visual Exp. (2021) 2021:e62497. doi: 10.3791/62497
69. Kuncorojakti S, Rodprasert W, Yodmuang S, Osathanon T, Pavasant P, Srisuwatanasagul S, et al. Alginate/Pluronic F127-based encapsulation supports viability and functionality of human dental pulp stem cell-derived insulin-producing cells. J Biol Eng. (2020) 14:1–15. doi: 10.1186/s13036-020-00246-1
70. Sawangmake C, Rodprasert W, Osathanon T, Pavasant P. Integrative protocols for an in vitro generation of pancreatic progenitors from human dental pulp stem cells. Biochem Biophys Res Commun. (2020) 530:222–9. doi: 10.1016/j.bbrc.2020.06.145
71. Algorta A, Artigas R, Yaneselli K, Rodellar C, Maisonnave J. Isolation and in vitro characterization of feline dental pulp stem. Cells. (2021) 23:21. doi: 10.1016/j.jcyt.2021.02.064
72. Srionrod N, Bootcha R, Petchdee S. Foal deciduous teeth stem cells enhance wound healing in rabbit wound model. Thai J Vet Med. (2016) 46:155. doi: 10.56808/2985-1130.2708
73. Yong-Ming H, Wei Z, Xiu-Li Z, Jia-Yu L, De-Rong Z. Ectopic osteogenesis of SGDs in goat's muscle pouch. Shanghai J Stomatol. (2012) 21:622–7.
74. McCulloch C. Progenitor cell populations in the periodontal ligament of mice. Anat Rec. (1985) 211:258–62. doi: 10.1002/ar.1092110305
75. Gay IC, Chen S, MacDougall M. Isolation and characterization of multipotent human periodontal ligament stem cells. Orthod Craniofac Res. (2007) 10:149–60. doi: 10.1111/j.1601-6343.2007.00399.x
76. Sawangmake C, Pavasant P, Chansiripornchai P, Osathanon T. High glucose condition suppresses neurosphere formation by human periodontal ligament-derived mesenchymal stem cells. J Cell Biochem. (2014) 115:928–39. doi: 10.1002/jcb.24735
77. Yang H, Li J, Hu Y, Sun J, Guo W, Li H, et al. Treated dentin matrix particles combined with dental follicle cell sheet stimulate periodontal regeneration. Dental Mater. (2019) 35:1238–53. doi: 10.1016/j.dental.2019.05.016
78. Mitrano TI, Grob MS, Carrion F, Nova-Lamperti E, Luz PA, Fierro FS, et al. Culture and characterization of mesenchymal stem cells from human gingival tissue. J Periodontol. (2010) 81:917–25. doi: 10.1902/jop.2010.090566
79. Tomar GB, Srivastava RK, Gupta N, Barhanpurkar AP, Pote ST, Jhaveri HM, et al. Human gingiva-derived mesenchymal stem cells are superior to bone marrow-derived mesenchymal stem cells for cell therapy in regenerative medicine. Biochem Biophys Res Commun. (2010) 393:377–83. doi: 10.1016/j.bbrc.2010.01.126
80. Muench LN, Kia C, Otto A, Mehl J, Baldino JB, Cote MP, et al. The effect of a single consecutive volume aspiration on concentrated bone marrow from the proximal humerus for clinical application. BMC Musculoskelet Disord. (2019) 20:1–8. doi: 10.1186/s12891-019-2924-2
81. Purwaningrum M, Jamilah NS, Purbantoro SD, Sawangmake C, Nantavisai S. Comparative characteristic study from bone marrow-derived mesenchymal stem cells. J Vet Sci. (2021) 22:e74. doi: 10.4142/jvs.2021.22.e74
82. Raskin RE, Messick JB. Bone marrow cytologic and histologic biopsies: indications, technique, and evaluation. Vet Clin. (2012) 42:23–42. doi: 10.1016/j.cvsm.2011.10.001
83. Nantavisai S, Egusa H, Osathanon T, Sawangmake C. Mesenchymal stem cell-based bone tissue engineering for veterinary practice. Heliyon. (2019) 5:e02808. doi: 10.1016/j.heliyon.2019.e02808
84. Vasiliadis AV, Galanis N. Human bone marrow-derived mesenchymal stem cells from different bone sources: a panorama. Stem Cell Investig. (2020) 7:13. doi: 10.21037/sci-2020-013
85. Martin DR, Cox NR, Hathcock TL, Niemeyer GP, Baker HJ. Isolation and characterization of multipotential mesenchymal stem cells from feline bone marrow. Exp Hematol. (2002) 30:879–86. doi: 10.1016/S0301-472X(02)00864-0
86. Humenik F, Cizkova D, Cikos S, Luptakova L, Madari A, Mudronova D, et al. Canine bone marrow-derived mesenchymal stem cells: genomics, proteomics and functional analyses of paracrine factors. Mol Cell Proteom. (2019) 18:1824–35. doi: 10.1074/mcp.RA119.001507
87. Shahsavari A, Weeratunga P, Ovchinnikov DA, Whitworth DJ. Pluripotency and immunomodulatory signatures of canine induced pluripotent stem cell-derived mesenchymal stromal cells are similar to harvested mesenchymal stromal cells. Sci Rep. (2021) 11:1–18. doi: 10.1038/s41598-021-82856-3
88. Maciel BB, Rebelatto CL, Brofman PR, Brito HF, Patricio LF, Cruz MA, et al. Morphology and morphometry of feline bone marrow-derived mesenchymal stem cells in culture. SciELO Brasil. (2014) 16:1127–34. doi: 10.1590/S0100-736X2014001100016
89. Trivanović D, Nikolić S, Krstić J, Jauković A, Mojsilović S, Ilić V, et al. Characteristics of human adipose mesenchymal stem cells isolated from healthy and cancer affected people and their interactions with human breast cancer cell line M CF-7 in vitro. Cell Biol Int. (2014) 38:254–65. doi: 10.1002/cbin.10198
90. Clark KC, Fierro FA, Ko EM, Walker NJ, Arzi B, Tepper CG, et al. Human and feline adipose-derived mesenchymal stem cells have comparable phenotype, immunomodulatory functions, and transcriptome. Stem Cell Res Ther. (2017) 8:1–16. doi: 10.1186/s13287-017-0528-z
91. Lee B-Y, Li Q, Song W-J, Chae H-K, Kweon K, Ahn J-O, et al. Altered properties of feline adipose-derived mesenchymal stem cells during continuous in vitro cultivation. J Vet Med Sci. (2018) 80:930–8. doi: 10.1292/jvms.17-0563
92. Arévalo-Turrubiarte M, Olmeo C, Accornero P, Baratta M, Martignani E. Analysis of mesenchymal cells (MSCs) from bone marrow, synovial fluid and mesenteric, neck and tail adipose tissue sources from equines. Stem Cell Res. (2019) 37:101442. doi: 10.1016/j.scr.2019.101442
93. Ishikawa S, Horinouchi C, Murata D, Matsuzaki S, Misumi K, Iwamoto Y, et al. Isolation and characterization of equine dental pulp stem cells derived from Thoroughbred wolf teeth. J Vet Med Sci. 79:47–51. doi: 10.1292/jvms.16-0131
94. Purbantoro SD, Osathanon T, Nantavisai S, Sawangmake C. Osteogenic growth peptide enhances osteogenic differentiation of human periodontal ligament stem cells. Heliyon. (2022) 2022:e09936. doi: 10.1016/j.heliyon.2022.e09936
95. Mukhtar AH, Alqutub MN. Osteogenic potential of periodontal ligament stem cells cultured in osteogenic and regular growth media: confocal and scanning electron microscope study. J Contemp Dent Pract. (2020) 21:776–80. doi: 10.5005/jp-journals-10024-2822
96. Pöschke A, Krähling B, Failing K, Staszyk C. Molecular characteristics of the equine periodontal ligament. Front Vet Sci. (2018) 4:235. doi: 10.3389/fvets.2017.00235
97. Warhonowicz M, Staszyk C, Rohn K, Gasse H. The equine periodontium as a continuously remodeling system: morphometrical analysis of cell proliferation. Arch Oral Biol. (2006) 51:1141–9. doi: 10.1016/j.archoralbio.2006.05.013
98. Vidal MA, Kilroy GE, Lopez MJ, Johnson JR, Moore RM, Gimble JM. Characterization of equine adipose tissue-derived stromal cells: adipogenic and osteogenic capacity and comparison with bone marrow-derived mesenchymal stromal cells. Vet Surg. (2007) 36:613–22. doi: 10.1111/j.1532-950X.2007.00313.x
99. Violini S, Ramelli P, Pisani LF, Gorni C, Mariani P. Horse bone marrow mesenchymal stem cells express embryo stem cell markers and show the ability for tenogenic differentiation by in vitro exposure to BMP-12. BMC Cell Biol. (2009) 10:1–10. doi: 10.1186/1471-2121-10-29
100. Worster AA, Nixon AJ, Brower-Toland BD, Williams J. Effect of transforming growth factor β1 on chondrogenic differentiation of cultured equine mesenchymal stem cells. Am J Vet Res. (2000) 61:1003–10. doi: 10.2460/ajvr.2000.61.1003
101. Alipour F, Parham A, Mehrjerdi HK, Dehghani H. Equine adipose-derived mesenchymal stem cells: phenotype and growth characteristics, gene expression profile and differentiation potentials. Cell J. (2015) 16:456. doi: 10.22074/cellj.2015.491
102. Braun J, Hack A, Weis-Klemm M, Conrad S, Treml S, Kohler K, et al. Evaluation of the osteogenic and chondrogenic differentiation capacities of equine adipose tissue-derived mesenchymal stem cells. Am J Vet Res. (2010) 71:1228–36. doi: 10.2460/ajvr.71.10.1228
103. Reed SA, Johnson SE. Equine umbilical cord blood contains a population of stem cells that express Oct4 and differentiate into mesodermal and endodermal cell types. J Cell Physiol. (2008) 215:329–36. doi: 10.1002/jcp.21312
104. Iohara K, Zheng L, Ito M, Tomokiyo A, Matsushita K, Nakashima M, et al. population cells isolated from porcine dental pulp tissue with self-renewal and multipotency for dentinogenesis, chondrogenesis, adipogenesis, and neurogenesis. Stem Cells. (2006) 24:2493–503. doi: 10.1634/stemcells.2006-0161
105. Ding G, Liu Y, Wang W, Wei F, Liu D, Fan Z, et al. Allogeneic periodontal ligament stem cell therapy for periodontitis in swine. Stem Cells. (2010) 28:1829–38. doi: 10.1002/stem.512
106. Liu Y, Zheng Y, Ding G, Fang D, Zhang C, Bartold PM, et al. Periodontal ligament stem cell-mediated treatment for periodontitis in miniature swine. Stem Cells. (2008) 26:1065–73. doi: 10.1634/stemcells.2007-0734
107. Wasi S, Otsuka K, Yao K-L, Tung PS, Aubin JE, Sodek J, et al. An osteonectinlike protein in porcine periodontal ligament and its synthesis by periodontal ligament fibroblasts. Can J Biochem Cell Biol. (1984) 62:470–8. doi: 10.1139/o84-064
108. Nohutcu R, McCauley LK, Shigeyama Y, Somerman MJ. Expression of mineral-associated proteins by periodontal ligament cells: in vitro vs. ex vivo. J Periodontal Res. (1996) 31:369–72. doi: 10.1111/j.1600-0765.1996.tb00505.x
109. Gronthos S, Mrozik K, Shi S, Bartold P. Ovine periodontal ligament stem cells: isolation, characterization, and differentiation potential. Calcif Tissue Int. (2006) 79:310–7. doi: 10.1007/s00223-006-0040-4
110. Tsuchiya S, Ohshima S, Yamakoshi Y, Simmer JP, Honda MJ. Osteogenic differentiation capacity of porcine dental follicle progenitor cells. Connect Tissue Res. (2010) 51:197–207. doi: 10.3109/03008200903267542
111. Abay N, Gurel Pekozer G, Ramazanoglu M, Kose GT. Bone formation from porcine dental germ stem cells on surface modified polybutylene succinate scaffolds. Stem Cells Int. (2016) 2016:8792191. doi: 10.1155/2016/8792191
112. Handa K, Saito M, Yamauchi M, Kiyono T, Sato S, Teranaka T, et al. Cementum matrix formation in vivo by cultured dental follicle cells. Bone. (2002) 31:606–11. doi: 10.1016/S8756-3282(02)00868-2
113. Bosnakovski D, Mizuno M, Kim G, Takagi S, Okumura M, Fujinaga T. Isolation and multilineage differentiation of bovine bone marrow mesenchymal stem cells. Cell Tissue Res. (2005) 319:243–53. doi: 10.1007/s00441-004-1012-5
114. McCarty RC, Gronthos S, Zannettino AC, Foster BK, Xian CJ. Characterisation and developmental potential of ovine bone marrow derived mesenchymal stem cells. J Cell Physiol. (2009) 219:324–33. doi: 10.1002/jcp.21670
115. Rentsch C, Hess R, Rentsch B, Hofmann A, Manthey S, Scharnweber D, et al. Ovine bone marrow mesenchymal stem cells: isolation and characterization of the cells and their osteogenic differentiation potential on embroidered and surface-modified polycaprolactone-co-lactide scaffolds. In Vitro Cell Dev Biol Anim. (2010) 46:624–34. doi: 10.1007/s11626-010-9316-0
116. Arrizabalaga JH, Nollert MU. Properties of porcine adipose-derived stem cells and their applications in preclinical models. Adipocyte. (2017) 6:217–23. doi: 10.1080/21623945.2017.1312040
117. Brückner S, Tautenhahn H-M, Winkler S, Stock P, Dollinger M, Christ B, et al. fat option for the pig: hepatocytic differentiated mesenchymal stem cells for translational research. Exp Cell Res. (2014) 321:267–75. doi: 10.1016/j.yexcr.2013.10.018
118. Stock P, Brückner S, Ebensing S, Hempel M, Dollinger MM, Christ B. The generation of hepatocytes from mesenchymal stem cells and engraftment into murine liver. Nat Protoc. (2010) 5:617–27. doi: 10.1038/nprot.2010.7
119. Wang KH, Kao AP, Wangchen H, Wang FY, Chang CH, Chang CC, et al. Optimizing proliferation and characterization of multipotent stem cells from porcine adipose tissue. Biotechnol Appl Biochem. (2008) 51:159–66. doi: 10.1042/BA20070201
120. Huang T, He D, Kleiner G, Kuluz JT. Neuron-like differentiation of adipose-derived stem cells from infant piglets in vitro. J Spinal Cord Med. (2007) 30:S35–40. doi: 10.1080/10790268.2007.11753967
121. Liu H-Y, Chen C-C, Lin Y-Y, Chen Y-J, Liu B-H, Wong S-C, et al. Chitosan-assisted differentiation of porcine adipose tissue-derived stem cells into glucose-responsive insulin-secreting clusters. PLoS ONE. (2017) 12:e0172922. doi: 10.1371/journal.pone.0172922
122. Casado JG, Gomez-Mauricio G, Alvarez V, Mijares J, Tarazona R, Bernad A, et al. Comparative phenotypic and molecular characterization of porcine mesenchymal stem cells from different sources for translational studies in a large animal model. Vet Immunol Immunopathol. (2012) 147:104–12. doi: 10.1016/j.vetimm.2012.03.015
123. Arrigoni E, Lopa S, De Girolamo L, Stanco D, Brini AT. Isolation, characterization and osteogenic differentiation of adipose-derived stem cells: from small to large animal models. Cell Tissue Res. (2009) 338:401–11. doi: 10.1007/s00441-009-0883-x
124. Bionaz M, Monaco E, Wheeler MB. Transcription adaptation during in vitro adipogenesis and osteogenesis of porcine mesenchymal stem cells: dynamics of pathways, biological processes, up-stream regulators, and gene networks. PLoS ONE. (2015) 10:e0137644. doi: 10.1371/journal.pone.0137644
125. Lu T, Xiong H, Wang K, Wang S, Ma Y, Guan W. Isolation and characterization of adipose-derived mesenchymal stem cells (ADSCs) from cattle. Appl Biochem Biotechnol. (2014) 174:719–28. doi: 10.1007/s12010-014-1128-3
126. Grzesiak J, Krzysztof M, Karol W, Joanna C. Isolation and morphological characterisation of ovine adipose-derived mesenchymal stem cells in culture. Int J Stem Cells. (2011) 4:99. doi: 10.15283/ijsc.2011.4.2.99
127. Dar ER, Gugjoo MB, Javaid M, Hussain S, Fazili MR, Dhama K, et al. Adipose tissue-and bone marrow-derived mesenchymal stem cells from sheep: culture characteristics. Animals. (2021) 11:2153. doi: 10.3390/ani11082153
128. Ling LE, Feng L, Liu HC, Wang DS, Shi ZP, Wang JC, et al. The effect of calcium phosphate composite scaffolds on the osteogenic differentiation of rabbit dental pulp stem cells. J Biomed Mater Res A. (2015) 103:1732–45. doi: 10.1002/jbm.a.35303
129. Liu H-C, Wang D-S, Su F, Wu X, Shi Z-P. Reconstruction of alveolar bone defects using bone morphogenetic protein 2 mediated rabbit dental pulp stem cells seeded on nano-hydroxyapatite/collagen/poly (L-lactide). Tissue Eng A. (2011) 17:2417–33. doi: 10.1089/ten.tea.2010.0620
130. El-Backly RM, Massoud AG, El-Badry AM, Sherif RA, Marei MK. Regeneration of dentine/pulp-like tissue using a dental pulp stem cell/poly (lactic-co-glycolic) acid scaffold construct in New Zealand white rabbits. Austr Endodont J. (2008) 34:52–67. doi: 10.1111/j.1747-4477.2008.00139.x
131. Yang X, Walboomers XF, van den Beucken JJ, Bian Z, Fan M, Jansen JA. Hard tissue formation of STRO-1-selected rat dental pulp stem cells in vivo. Tissue Eng A. (2009) 15:367–75. doi: 10.1089/ten.tea.2008.0133
132. Ellis KM, O'Carroll DC, Lewis MD, Rychkov GY, Koblar SA. Neurogenic potential of dental pulp stem cells isolated from murine incisors. Stem Cell Res Ther. (2014) 5:1–13. doi: 10.1186/scrt419
133. Emerton K, Drapeau S, Prasad H, Rohrer M, Roffe P, Hopper K, et al. Regeneration of periodontal tissues in non-human primates with rhGDF-5 and beta-tricalcium phosphate. J Dent Res. (2011) 90:1416–21. doi: 10.1177/0022034511423665
134. Guo S, Guo W, Ding Y, Gong J, Zou Q, Xie D, et al. Comparative study of human dental follicle cell sheets and periodontal ligament cell sheets for periodontal tissue regeneration. Cell Transplant. (2013) 22:1061–73. doi: 10.3727/096368912X656036
135. Techawattanawisal W, Nakahama K, Komaki M, Abe M, Takagi Y, Morita I. Isolation of multipotent stem cells from adult rat periodontal ligament by neurosphere-forming culture system. Biochem Biophys Res Commun. (2007) 357:917–23. doi: 10.1016/j.bbrc.2007.04.031
136. Hong H, Chen X, Li K, Wang N, Li M, Yang B, et al. Dental follicle stem cells rescue the regenerative capacity of inflamed rat dental pulp through a paracrine pathway. Stem Cell Res Ther. (2020) 11:1–16. doi: 10.1186/s13287-020-01841-1
137. Guo W, He Y, Zhang X, Lu W, Wang C, Yu H, et al. The use of dentin matrix scaffold and dental follicle cells for dentin regeneration. Biomaterials. (2009) 30:6708–23. doi: 10.1016/j.biomaterials.2009.08.034
138. Luan X, Ito Y, Dangaria S, Diekwisch TG. Dental follicle progenitor cell heterogeneity in the developing mouse periodontium. Stem Cells Dev. (2006) 15:595–608. doi: 10.1089/scd.2006.15.595
139. Nugraha AP, Rantam FA, Narmada IB, Ernawati DS, Ihsan IS. Gingival-derived mesenchymal stem cell from rabbit (Oryctolagus cuniculus): isolation, culture, and characterization. Eur J Dent. (2021) 15:332–9. doi: 10.1055/s-0040-1719213
140. Nugraha AP, Narmada IB, Ernawati DS, Widodo ADW, Lestari P, Dinaryanti A, et al. Gingival mesenchymal stem cells from Wistar Rat's Gingiva (Rattus Novergicus)-isolation and characterization (in vitro study). J Int Dental Med Res. (2018) 11:694–9. Available online at: https://www.semanticscholar.org/paper/Gingival-Mesenchymal-Stem-Cells-from-Wistar-Rat-%E2%80%99-s-Nugraha-Narmada/d79bb92688b8ee015deefff723c8261fd055fbe9
141. Sun Q, Nakata H, Kasugai S, Kuroda S. Isolation and characteristics of mouse gingival mesenchymal stem cells. J Bio-Integrat. (2019) 9:21–8. doi: 10.32176/biointeg.9.1_21
142. Iizuka T, Takahashi T, Ushijima N. Characteristics and properties of gingival mesenchymal stem cells. Hokkaido Den J. (2017) 38:40–6. Available online at: https://eprints.lib.hokudai.ac.jp/dspace/handle/2115/67335
143. Izadpanah R, Joswig T, Tsien F, Dufour J, Kirijan JC, Bunnell BA. Characterization of multipotent mesenchymal stem cells from the bone marrow of rhesus macaques. Stem Cells Dev. (2005) 14:440–51. doi: 10.1089/scd.2005.14.440
144. Zhang W, Zhang F, Shi H, Tan R, Han S, Ye G, et al. Comparisons of rabbit bone marrow mesenchymal stem cell isolation and culture methods in vitro. PLoS ONE. (2014) 9:e88794. doi: 10.1371/journal.pone.0088794
145. Liu H, Wei LK, Jian XF, Huang J, Zou H, Zhang SZ, et al. Isolation, culture and induced differentiation of rabbit mesenchymal stem cells into osteoblasts. Exp Ther Med. (2018) 15:3715–24. doi: 10.3892/etm.2018.5894
146. Caroti CM, Ahn H, Salazar HF, Joseph G, Sankar SB, Willett NJ, et al. A novel technique for accelerated culture of murine mesenchymal stem cells that allows for sustained multipotency. Sci Rep. (2017) 7:1–14. doi: 10.1038/s41598-017-13477-y
147. Soleimani M, Nadri S. A protocol for isolation and culture of mesenchymal stem cells from mouse bone marrow. Nat Protoc. (2009) 4:102–6. doi: 10.1038/nprot.2008.221
148. Jiang J-L, Li T, Bi X, Wu Z-X, Hou K-Y, Chen Z. Induced differentiation of macaque adipose-derived stem cells in vitro. Chin Med J. (2021) 134:2379–81. doi: 10.1097/CM9.0000000000001486
149. Zomer HD, Roballo KC, Lessa TB, Bressan FF, Goncalves NN, Meirelles FV, et al. Distinct features of rabbit and human adipose-derived mesenchymal stem cells: implications for biotechnology and translational research. Stem Cells Cloning. (2018) 11:43. doi: 10.2147/SCCAA.S175749
150. Gayathri V, Harikrishnan V, Mohanan PV. Integration of rabbit adipose derived mesenchymal stem cells to hydroxyapatite burr hole button device for bone interface regeneration. Int J Biomater. (2016) 2016:1067857. doi: 10.1155/2016/1067857
151. Sun J, Liu WH, Deng FM, Luo YH, Wen K, Zhang H, et al. Differentiation of rat adipose-derived mesenchymal stem cells into corneal-like epithelial cells driven by PAX6. Exp Ther Med. (2018) 15:1424–32. doi: 10.3892/etm.2017.5576
152. He Q, Ye Z, Zhou Y, Tan W-S. Comparative study of mesenchymal stem cells from rat bone marrow and adipose tissue. Turk J Biol. (2018) 42:477–89. doi: 10.3906/biy-1802-52
153. Kilroy G, Dietrich M, Wu X, Gimble JM, Floyd ZE. Isolation of murine adipose-derived stromal/stem cells for adipogenic differentiation or flow cytometry-based analysis. Adipose Derived Stem Cells. (2018) 11:137–46. doi: 10.1007/978-1-4939-7799-4_11
155. Rai R, Raval R, Khandeparker RVS, Chidrawar SK, Khan AA, Ganpat MS. Tissue engineering: step ahead in maxillofacial reconstruction. J Int Oral Health. (2015) 7:138.
156. Ikada Y. Challenges in tissue engineering. J Royal Soc Interface. (2006) 3:589–601. doi: 10.1098/rsif.2006.0124
157. O'brien FJ. Biomaterials & scaffolds for tissue engineering. Mater Today. (2011) 14:88–95. doi: 10.1016/S1369-7021(11)70058-X
158. Velasco MA, Narváez-Tovar CA, Garzón-Alvarado DA. Design, materials, and mechanobiology of biodegradable scaffolds for bone tissue engineering. BioMed Res Int. (2015) 2015:729076. doi: 10.1155/2015/729076
159. Kumar A, Kargozar S, Baino F, Han SS. Additive manufacturing methods for producing hydroxyapatite and hydroxyapatite-based composite scaffolds: a review. Front Mater. (2019) 6:313. doi: 10.3389/fmats.2019.00313
160. Caddeo S, Boffito M, Sartori S. Tissue engineering approaches in the design of healthy and pathological in vitro tissue models. Front Bioeng Biotechnol. (2017) 5:40. doi: 10.3389/fbioe.2017.00040
161. Murugan R, Ramakrishna S. Development of nanocomposites for bone grafting. Compos Sci Technol. (2005) 65:2385–406. doi: 10.1016/j.compscitech.2005.07.022
162. Vapniarsky N, Huwe LW, Arzi B, Houghton MK, Wong ME, Wilson JW, et al. Tissue engineering toward temporomandibular joint disc regeneration. Sci Transl Med. (2018) 10:eaaq1802. doi: 10.1126/scitranslmed.aaq1802
163. Elias C, Lima J, Valiev R, Meyers M. Biomedical applications of titanium and its alloys. JOM. (2008) 60:46–9. doi: 10.1007/s11837-008-0031-1
164. Zhang L, Tang J, Sun L, Zheng T, Pu X, Chen Y, et al. Three-dimensional printed tissue engineered bone for canine mandibular defects. Genes Dis. (2020) 7:138–49. doi: 10.1016/j.gendis.2019.04.003
165. Donnaloja F, Jacchetti E, Soncini M, Raimondi MT. Natural and synthetic polymers for bone scaffolds optimization. Polymers. (2020) 12:905. doi: 10.3390/polym12040905
166. Puertas-Bartolomé M, Mora-Boza A, García-Fernández L. Emerging biofabrication techniques: a review on natural polymers for biomedical applications. Polymers. (2021) 13:1209. doi: 10.3390/polym13081209
167. Ragunathan S, Govindasamy G, Raghul D, Karuppaswamy M, VijayachandraTogo R. Hydroxyapatite reinforced natural polymer scaffold for bone tissue regeneration. Mater Today Proc. (2020) 23:111–8. doi: 10.1016/j.matpr.2019.07.712
168. Filippi M, Born G, Chaaban M, Scherberich A. Natural polymeric scaffolds in bone regeneration. Front Bioeng Biotechnol. (2020) 8:474. doi: 10.3389/fbioe.2020.00474
169. Reddy M, Ponnamma D, Choudhary R, Sadasivuni KK. A comparative review of natural and synthetic biopolymer composite scaffolds. Polymers. (2021) 13:1105. doi: 10.3390/polym13071105
170. Gao J, Dennis JE, Solchaga LA, Awadallah AS, Goldberg VM, Caplan AI. Tissue-engineered fabrication of an osteochondral composite graft using rat bone marrow-derived mesenchymal stem cells. Tissue Eng. (2001) 7:363–71. doi: 10.1089/10763270152436427
171. Wang M. Developing bioactive composite materials for tissue replacement. Biomaterials. (2003) 24:2133–51. doi: 10.1016/S0142-9612(03)00037-1
172. Abbas Z, Dapporto M, Tampieri A, Sprio S. Toughening of bioceramic composites for bone regeneration. J Composit Sci. (2021) 5:259. doi: 10.3390/jcs5100259
173. Kim RY, Fasi AC, Feinberg SE. Soft tissue engineering in craniomaxillofacial surgery. Ann Maxillofac Surg. (2014) 4:4. doi: 10.4103/2231-0746.133064
174. Pashuck ET, Stevens MM. Designing regenerative biomaterial therapies for the clinic. Sci Transl Med. (2012) 4:160sr4. doi: 10.1126/scitranslmed.3002717
175. Ayala-Ham A, López-Gutierrez J, Bermúdez M, Aguilar-Medina M, Sarmiento-Sánchez JI, López-Camarillo C, et al. Hydrogel-based scaffolds in oral tissue engineering. Front Mater. (2021) 2021:294. doi: 10.3389/fmats.2021.708945
176. Kriegebaum U, Mildenberger M, Mueller-Richter UD, Klammert U, Kuebler AC, Reuther T. Tissue engineering of human oral mucosa on different scaffolds: in vitro experiments as a basis for clinical applications. Oral Surg Oral Med Oral Pathol Oral Radiol. (2012) 114:S190–S8. doi: 10.1016/j.oooo.2011.10.019
177. Guan Y, You H, Cai J, Zhang Q, Yan S, You R. Physically crosslinked silk fibroin/hyaluronic acid scaffolds. Carbohydr Polym. (2020) 239:116232. doi: 10.1016/j.carbpol.2020.116232
178. Sangkert S, Kamolmatyakul S, Meesane J. Mimicked scaffolds based on coated silk woven fabric with gelatin and chitosan for soft tissue defect in oral maxillofacial area. Int J Artif Organs. (2020) 43:189–202. doi: 10.1177/0391398819877191
179. Joraku A, Sullivan CA, Yoo JJ, Atala A. Tissue engineering of functional salivary gland tissue. Laryngoscope. (2005) 115:244–8. doi: 10.1097/01.mlg.0000154726.77915.cc
180. Lee MH, Arcidiacono JA, Bilek AM, Wille JJ, Hamill CA, Wonnacott KM, et al. Considerations for tissue-engineered and regenerative medicine product development prior to clinical trials in the United States. Tissue Eng B Rev. (2010) 16:41–54. doi: 10.1089/ten.teb.2009.0449
181. Izadifar Z, Chen X, Kulyk W. Strategic design and fabrication of engineered scaffolds for articular cartilage repair. J Funct Biomater. (2012) 3:799–838. doi: 10.3390/jfb3040799
182. Ahtiainen K, Mauno J, Ellä V, Hagström J, Lindqvist C, Miettinen S, et al. Autologous adipose stem cells and polylactide discs in the replacement of the rabbit temporomandibular joint disc. J Royal Soc Interface. (2013) 10:20130287. doi: 10.1098/rsif.2013.0287
183. Bailey MM, Wang L, Bode CJ, Mitchell KE, Detamore MS. A comparison of human umbilical cord matrix stem cells and temporomandibular joint condylar chondrocytes for tissue engineering temporomandibular joint condylar cartilage. Tissue Eng. (2007) 13:2003–10. doi: 10.1089/ten.2006.0150
184. Bousnaki M, Bakopoulou A, Papadogianni D, Barkoula N-M, Alpantaki K, Kritis A, et al. Fibro/chondrogenic differentiation of dental stem cells into chitosan/alginate scaffolds towards temporomandibular joint disc regeneration. J Mater Sci. (2018) 29:1–17. doi: 10.1007/s10856-018-6109-6
185. Wu Y, Gong Z, Li J, Meng Q, Fang W, Long X. The pilot study of fibrin with temporomandibular joint derived synovial stem cells in repairing TMJ disc perforation. BioMed Res Int. (2014) 2014:454021. doi: 10.1155/2014/454021
186. Schaefer D, Martin I, Shastri P, Padera R, Langer R, Freed L, et al. In vitro generation of osteochondral composites. Biomaterials. (2000) 21:2599–606. doi: 10.1016/S0142-9612(00)00127-7
187. Mohammadzadeh F, Ajdary M, Mohammadzadeh A, Safdarian L, Amidi F, Sobhani A. Leukemia inhibitory factor's effect on the growth and survival of sheep's follicles of ovarian tissue during vitrification. Cell Tissue Bank. (2022) 22:4. doi: 10.1007/s10561-022-10018-4
188. Cicciù M. Growth Factor Applied to Oral and Regenerative Surgery. Basel: Multidisciplinary Digital Publishing Institute (2020). p. 7752.
189. Ramly EP, Alfonso AR, Kantar RS, Wang MM, Siso JRD, Ibrahim A, et al. Safety and efficacy of recombinant human bone morphogenetic protein-2 (rhBMP-2) in craniofacial surgery. Plast Reconstr Surg Glob Open. (2019) 7:2347. doi: 10.1097/GOX.0000000000002347
190. Sheikh Z, Javaid MA, Hamdan N, Hashmi R. Bone regeneration using bone morphogenetic proteins and various biomaterial carriers. Materials. (2015) 8:1778–816. doi: 10.3390/ma8041778
191. Tahriri M, Rasoulianboroujeni M, Bader R, Vashaee D, Tayebi L. Growth factors for oral and maxillofacial regeneration applications. Biomater Oral Dental Tissue Eng. (2017) 13:205–19. doi: 10.1016/B978-0-08-100961-1.00013-X
192. Xu J, Liu J, Gan Y, Dai K, Zhao J, Huang M, et al. High-dose TGF-β1 impairs mesenchymal stem cell-mediated bone regeneration via Bmp2 inhibition. J Bone Mineral Res. (2020) 35:167–80. doi: 10.1002/jbmr.3871
193. McNeilly T, McNally C, Finch M, Beringer T. Recombinant PTH: a study of the outcome of teriparatide therapy for 138 patients with osteoporosis. Ulster Med J. (2013) 82:89.
194. Bashutski JD, Eber RM, Kinney JS, Benavides E, Maitra S, Braun TM, et al. Teriparatide and osseous regeneration in the oral cavity. N Engl J Med. (2010) 363:2396–405. doi: 10.1056/NEJMoa1005361
195. Qian C, Xin T, Xiao W, Zhu H, Zhang Q, Liu L, et al. Vascularized silk electrospun fiber for promoting oral mucosa regeneration. NPG Asia Mater. (2020) 12:1–16. doi: 10.1038/s41427-020-0221-z
196. Kinoshita A, Oda S, Takahashi K, Yokota S, Ishikawa I. Periodontal regeneration by application of recombinant human bone morphogenetic protein-2 to horizontal circumferential defects created by experimental periodontitis in beagle dogs. J Periodontol. (1997) 68:103–9. doi: 10.1902/jop.1997.68.2.103
197. Khorsand A, Eslaminejad MB, Arabsolghar M, Paknejad M, Ghaedi B, Rokn AR, et al. Autologous dental pulp stem cells in regeneration of defect created in canine periodontal tissue. J Oral Implantol. (2013) 39:433–43. doi: 10.1563/AAID-JOI-D-12-00027
198. Kok IJD, Drapeau SJ, Young R, Cooper LF. Evaluation of mesenchymal stem cells following implantation in alveolar sockets: a canine safety study. Int J Oral Maxillof Implants. (2005) 20:511–8.
199. Behnia A, Haghighat A, Talebi A, Nourbakhsh N, Heidari F. Transplantation of stem cells from human exfoliated deciduous teeth for bone regeneration in the dog mandibular defect. World J Stem Cells. (2014) 6:505. doi: 10.4252/wjsc.v6.i4.505
200. Brown BN, Chung WL, Almarza AJ, Pavlick MD, Reppas SN, Ochs MW, et al. Inductive, scaffold-based, regenerative medicine approach to reconstruction of the temporomandibular joint disk. J Oral Maxillof Surg. (2012) 70:2656–68. doi: 10.1016/j.joms.2011.12.030
201. Wang S, Zhang Z, Xia L, Zhao J, Sun X, Zhang X, et al. Systematic evaluation of a tissue-engineered bone for maxillary sinus augmentation in large animal canine model. Bone. (2010) 46:91–100. doi: 10.1016/j.bone.2009.09.008
202. Iohara K, Utsunomiya S, Kohara S, Nakashima M. Allogeneic transplantation of mobilized dental pulp stem cells with the mismatched dog leukocyte antigen type is safe and efficacious for total pulp regeneration. Stem Cell Res Ther. (2018) 9:1–16. doi: 10.1186/s13287-018-0855-8
203. Kamm JL, Parlane NA, Riley CB, Gee EK, Dittmer KE, McIlwraith CW. Blood type and breed-associated differences in cell marker expression on equine bone marrow-derived mesenchymal stem cells including major histocompatibility complex class II antigen expression. PLoS ONE. (2019) 14:e0225161. doi: 10.1371/journal.pone.0225161
204. Zhou M, Chen X, Qiu Y, Chen H, Liu Y, Hou Y, et al. Study of tissue engineered vascularised oral mucosa-like structures based on ACVM-025% HLC-I scaffold in vitro and in vivo. Artif Cells Nanomed Biotechnol. (2020) 48:1167–77. doi: 10.1080/21691401.2020.1817055
205. Pradhan-Bhatt S, Harrington DA, Duncan RL, Farach-Carson MC, Jia X, Witt RL, et al. Novel in vivo model for evaluating functional restoration of a tissue-engineered salivary gland. Laryngoscope. (2014) 124:456–61. doi: 10.1002/lary.24297
206. Mautner K, Gottschalk M, Boden SD, Akard A, Bae WC, Black L, et al. Cell-based versus corticosteroid injections for knee pain in osteoarthritis: a randomized phase 3 trial. Nat Med. (2023) 2023:1–7. doi: 10.1038/s41591-023-02776-9
207. de Jong R, Houtgraaf JH, Samiei S, Boersma E, Duckers HJ. Intracoronary stem cell infusion after acute myocardial infarction: a meta-analysis and update on clinical trials. Circulation. (2014) 7:156–67. doi: 10.1161/CIRCINTERVENTIONS.113.001009
209. Kim SE, Shim KM, Jang K, Shim J-H, Kang SS. Three-dimensional printing-based reconstruction of a maxillary bone defect in a dog following tumor removal. In Vivo. (2018) 32:63–70. doi: 10.21873/invivo.11205
210. Spector DI, Keating JH, Boudrieau RJ. Immediate mandibular reconstruction of a 5 cm defect using rhBMP-2 after partial mandibulectomy in a dog. Vet Surg. (2007) 36:752–9. doi: 10.1111/j.1532-950X.2007.00332.x
211. Arzi B, Cissell DD, Pollard RE, Verstraete FJ. Regenerative approach to bilateral rostral mandibular reconstruction in a case series of dogs. Front Vet Sci. (2015) 2:4. doi: 10.3389/fvets.2015.00004
212. Arzi B, Verstraete FJ, Huey DJ, Cissell DD, Athanasiou KA. Regenerating mandibular bone using rhBMP-2: part 1-immediate reconstruction of segmental mandibulectomies. Vet Surg. (2015) 44:403–9. doi: 10.1111/j.1532-950X.2014.12123.x
213. Verstraete FJ, Arzi B, Huey DJ, Cissell DD, Athanasiou KA. Regenerating mandibular bone using rhBMP-2: part 2-treatment of chronic, defect non-union fractures. Vet Surg. (2015) 44:410–6. doi: 10.1111/j.1532-950X.2014.12122.x
214. Tsugawa AJ, Arzi B, Vapniarsky N, Verstraete FJ. A retrospective study on mandibular reconstruction following excision of canine acanthomatous ameloblastoma. Front Vet Sci. (2022) 9:900031. doi: 10.3389/fvets.2022.900031
215. Arzi B, Clark KC, Sundaram A, Spriet M, Verstraete FJ, Walker NJ, et al. Therapeutic efficacy of fresh, allogeneic mesenchymal stem cells for severe refractory feline chronic gingivostomatitis. Stem Cells Transl Med. (2017) 6:1710–22. doi: 10.1002/sctm.17-0035
216. Arzi B, Mills-Ko E, Verstraete FJ, Kol A, Walker NJ, Badgley MR, et al. Therapeutic efficacy of fresh, autologous mesenchymal stem cells for severe refractory gingivostomatitis in cats. Stem Cells Transl Med. (2016) 5:75–86. doi: 10.5966/sctm.2015-0127
217. He P, Zhang Q, Motiwala FI, Shanti RM, Chang BM, Le AD. Potential application of dental stem cells in regenerative reconstruction of oral and maxillofacial tissues: a narrative review. Front Oral Maxillof Med. (2022) 4:10. doi: 10.21037/fomm-21-10
218. Qin Y, Guan J, Zhang C. Mesenchymal stem cells: mechanisms and role in bone regeneration. Postgrad Med J. (2014) 90:643–7. doi: 10.1136/postgradmedj-2013-132387
219. Ivanovska A, Wang M, Arshaghi TE, Shaw G, Alves J, Byrne A, et al. Manufacturing mesenchymal stromal cells for the treatment of osteoarthritis in canine patients: challenges and recommendations. Front Vet Sci. (2022) 9:897150. doi: 10.3389/fvets.2022.897150
Keywords: oral and maxillofacial defects, oral and maxillofacial reconstruction, tissue engineering, maxillofacial regeneration, veterinary
Citation: Purbantoro SD, Taephatthanasagon T, Purwaningrum M, Hirankanokchot T, Peralta S, Fiani N, Sawangmake C and Rattanapuchpong S (2024) Trends of regenerative tissue engineering for oral and maxillofacial reconstruction in veterinary medicine. Front. Vet. Sci. 11:1325559. doi: 10.3389/fvets.2024.1325559
Received: 21 October 2023; Accepted: 05 February 2024;
Published: 21 February 2024.
Edited by:
Boaz Arzi, University of California, Davis, United StatesReviewed by:
João Requicha, University of Trás-os-Montes and Alto Douro, PortugalCopyright © 2024 Purbantoro, Taephatthanasagon, Purwaningrum, Hirankanokchot, Peralta, Fiani, Sawangmake and Rattanapuchpong. This is an open-access article distributed under the terms of the Creative Commons Attribution License (CC BY). The use, distribution or reproduction in other forums is permitted, provided the original author(s) and the copyright owner(s) are credited and that the original publication in this journal is cited, in accordance with accepted academic practice. No use, distribution or reproduction is permitted which does not comply with these terms.
*Correspondence: Sirirat Rattanapuchpong, c2lyaXJhdC5yYUBjaHVsYS5hYy50aA==; Chenphop Sawangmake, Y2hlbnBob3Auc0BjaHVsYS5hYy50aA==
Disclaimer: All claims expressed in this article are solely those of the authors and do not necessarily represent those of their affiliated organizations, or those of the publisher, the editors and the reviewers. Any product that may be evaluated in this article or claim that may be made by its manufacturer is not guaranteed or endorsed by the publisher.
Research integrity at Frontiers
Learn more about the work of our research integrity team to safeguard the quality of each article we publish.