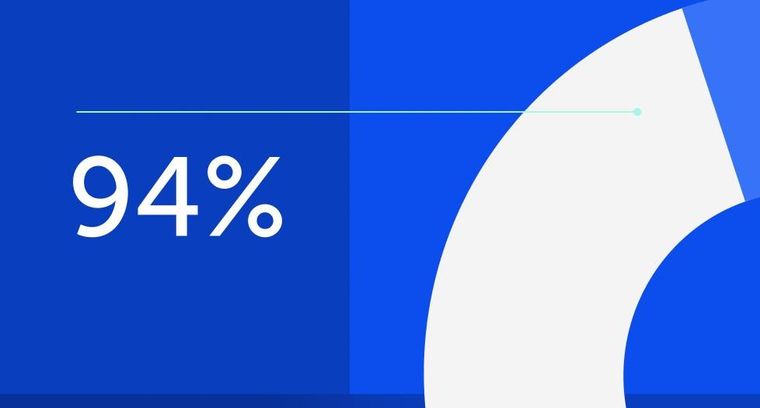
94% of researchers rate our articles as excellent or good
Learn more about the work of our research integrity team to safeguard the quality of each article we publish.
Find out more
ORIGINAL RESEARCH article
Front. Vet. Sci., 21 December 2023
Sec. Veterinary Clinical, Anatomical, and Comparative Pathology
Volume 10 - 2023 | https://doi.org/10.3389/fvets.2023.1258244
This article is part of the Research TopicAnimal Models for Basic and Applied Research in NeuroscienceView all 6 articles
Introduction: The relationship between epilepsy and cognitive dysfunction has been investigated in canines, and memory impairment was prevalent in dogs with epilepsy. Additionally, canines with epilepsy have greater amyloid-β (Aβ) accumulation and neuronal degeneration than healthy controls. The present study investigated plasma Aβ42 levels and performed proteomic profiling in dogs with refractory epilepsy and healthy dogs.
Methods: In total, eight dogs, including four healthy dogs and four dogs with epilepsy, were included in the study. Blood samples were collected to analyze Aβ42 levels and perform proteomic profiling. Changes in the plasma proteomic profiles of dogs were determined by nano liquid chromatography tandem mass spectrometry.
Results and discussion: The plasma Aβ42 level was significantly higher in dogs with epilepsy (99 pg/mL) than in healthy dogs (5.9 pg/mL). In total, 155 proteins were identified, and of these, the expression of 40 proteins was altered in epilepsy. Among these proteins, which are linked to neurodegenerative diseases, 10 (25%) were downregulated in dogs with epilepsy, whereas 12 (30%) were upregulated. The expression of the acute phase proteins haptoglobin and α2-macroglobulin significantly differed between the groups. Complement factor H and ceruloplasmin were only detected in epilepsy dogs, suggesting that neuroinflammation plays a role in epileptic seizures. Gelsolin, which is involved in cellular processes and cytoskeletal organization, was only detected in healthy dogs. Gene Ontology annotation revealed that epilepsy can potentially interfere with biological processes, including cellular processes, localization, and responses to stimuli. Seizures compromised key molecular functions, including catalytic activity, molecular function regulation, and binding. Defense/immunity proteins were most significantly modified during the development of epilepsy. In Kyoto Encyclopedia of Genes and Genomes pathway analysis, complement and coagulation cascades were the most relevant signaling pathways affected by seizures. The findings suggested that haptoglobin, ceruloplasmin, α2-macroglobulin, complement factor H, and gelsolin play roles in canine epilepsy and Aβ levels based on proteomic profiling. These proteins could represent diagnostic biomarkers that, after clinical validation, could be used in veterinary practice as well as proteins relevant to disease response pathways. To determine the precise mechanisms underlying these relationships and their implications in canine epilepsy, additional research is required.
Epileptic seizures are described by the International Veterinary Epilepsy Task Force (IVETF) as “a transient occurrence of signs due to abnormal excessive or synchronous neuronal activity in the brain” (1). The prevalence of canine epilepsy has been estimated to be 0.6–0.75% in the general dog population (2). Canine seizures significantly affect the health and well-being of dogs as well as their owners. Repeated or prolonged seizures can cause damage to the brain and nervous system, which can lead to cognitive and behavioral problems, as well as increased seizure frequency and severity (3, 4). Dogs that experience seizures can injury themselves or others during an episode because of the loss of consciousness, loss of motor control, or other factors (3, 4). Seizures can be emotionally stressful for both dogs and their owners, and they can lead to anxiety, depression, and decreased quality of life (5).
The pathophysiology of canine seizures is complex and incompletely understood. However, it is believed to involve a disruption in the balance of excitatory and inhibitory neurotransmitters in the brain, leading to hyperexcitability and synchronized firing of neurons. Genetic mutations, brain injury, or other factors affect ion channels and neurotransmitter receptors in the brain. These changes can increase the excitability of neurons and make them more likely to fire abnormally (4, 6). Inflammatory changes in the brain, such as the activation of microglia and the release of cytokines, can contribute to seizure development and propagation (7–9). Additionally, reactive oxygen species and other oxidizing agents can damage neurons and increase their excitability, potentially leading to seizures (10, 11).
The potential relationship between canine seizures and cognitive dysfunction syndrome (CDS), a condition characterized by cognitive decline similar to Alzheimer’s disease (AD) in humans, has received extensive focus. Dogs with CDS are more likely to experience seizures than healthy dogs. A dog experiencing a seizure exhibited typical symptoms commonly linked with canine dementia. Additionally, dogs with epilepsy displayed a higher prevalence of canine dementia symptoms compared with the control group (12, 13). Seizures and cognitive impairment are interconnected, and they can interact in a vicious cycle. Specifically, seizures can cause damage to the nervous system, including cell death and alterations in neurotransmitters, thereby affecting cognitive function. In addition, CDS and dementia can increase the incidence of seizures by changing the structure and function of the brain (14–18). Previous research revealed that dogs that experienced seizures had a higher risk of developing cognitive impairment than their counterparts. Furthermore, dogs with cognitive dysfunction were more prone to seizures than dogs without cognitive dysfunction (15). Similarly, people with epilepsy have a higher risk of developing dementia than their counterparts, and the risk of dementia increases with the duration of epilepsy (14, 16, 19). There is a complex relationship among seizures, AD, and amyloid-β (Aβ) (20–22). Recent studies suggested that seizures can contribute to the formation of Aβ plaques in the brain and increase Aβ levels in cerebrospinal fluid (CSF), both of which are hallmarks of AD (21, 23, 24). Prior studies illustrated that Aβ can enhance neuronal excitability and contribute to seizures through a variety of mechanisms, including synaptic dysfunction and neuroinflammation (17, 18). In addition, recent studies identified a potential link between Aβ and epilepsy-associated comorbidities, such as cognitive impairment and depression (18, 25). These studies suggested a bidirectional relationship between seizures and Aβ accumulation in the brain. Specifically, seizures can contribute to the formation of Aβ plaques, and Aβ accumulation can increase the risk of seizures.
Currently, the diagnosis of epilepsy is based on clinical signs, history, and the elimination of other potential causes of seizures (1, 3). Advanced imaging techniques, such as MRI and CT, can provide valuable information about the underlying causes of seizures. Proteomic analysis is a powerful tool that can be used in the diagnosis and therapeutic monitoring of canine epilepsy. In particular, proteomic analysis can be used to identify potential biomarkers of epilepsy in dogs. By comparing the protein profiles of dogs with and without epilepsy, researchers can identify proteins that are differentially expressed in dogs with epilepsy. These proteins can then be validated as potential biomarkers for use in diagnosis and treatment response assessment and as targets for new drugs (26, 27).
The objectives of this investigation were to determine plasma Aβ levels and identify proteins potentially involved in the pathogenesis of refractory canine epilepsy using proteomic analysis. We demonstrated for the first time that increased plasma Aβ42 levels were accompanied by alterations in plasma protein expression in dogs with refractory epilepsy. These findings could provide additional insights into the mechanisms underlying epilepsy. In addition, potential plasma biomarkers obtained via proteomic analysis may be utilized for treatment management.
Eight plasma samples were collected from dogs at the Small Animal Teaching Hospital, Faculty of Veterinary Medicine, Mahidol University (Nakhon Pathom, Thailand). The sample size of four animals in each group was determined using the EpiTools epidemiological calculator,1 with a significance level of 5% and a confidence level of 95%. The cohort included four dogs diagnosed with refractory idiopathic epilepsy and four healthy dogs. Idiopathic epilepsy was described as a condition in which dogs aged 6 months to 6 years old experienced their first seizure without any other underlying cause for the seizures without significant abnormalities on minimum data base blood tests (according to the IVETF diagnostic Tier I) (28). All idiopathic epilepsy dogs received appropriate dose of phenobarbital and potassium bromide but become refractory to all medication (29). The population characteristics of dogs in this study have been included in the Supplementary material. The sample collection protocol was approved by the Mahidol University Animal Care and Use Committee (AICUC: MUVS-2020-08-37). Blood samples were collected and centrifuged at 3000 rpm for 10 min. The first portion of the plasma sample was stored at −80°C for proteomic analysis, whereas the second portion was stored at −80°C to assess Aβ42 levels using enzyme-linked immunosorbent assay (ELISA).
The concentrations of plasma proteins were measured using Bradford’s assay. Protein samples from each group were pooled. For protein identification using nano liquid chromatography tandem mass spectrometry (nano-LC–MS/MS), 30 μg of pooled protein were loaded onto a 12% polyacrylamide gel. The protein bands on the gel were stained using Coomassie Brilliant Blue R-250 (Bio-Rad, Hercules, CA, USA) and then de-stained using a solution of 30% ethanol in 10% acetic acid. The gel was scanned using a GS-710 scanner (Bio-Rad, Hercules, CA, USA). For further analysis, the protein bands were divided into 11 segments per lane based and cut into pieces. The gel band excision technique was based on the ability to separate different protein sizes physically on the gel and did not cut in the middle of the protein bands. Each individual piece was then subjected to tryptic digestion.
Gel pieces were equilibrated using absolute acetonitrile and 50 mM NH4HCO3. Disulfide bonds were reduced using 4 mM dithiothreitol in 50 mM NH4HCO3 for 10 min at 60°C and alkylated in 250 mM iodoacetamide in 50 mM NH4HCO3 for 30 min at room temperature in the dark. The gel pieces were dehydrated twice in absolute acetonitrile for 15 min each and allowed to air-dry. Next, the gel pieces were subjected to trypsin digestion in 50 mM NH4HCO3 overnight at 37°C. The resulting tryptic peptides were extracted from the gel using absolute acetonitrile. Finally, the peptide mixtures were dried using a speed vacuum and stored at −80°C until analysis by nano-LC–MS/MS.
Extracted peptides were dissolved in 0.1% formic acid in LC/MS-grade water. Each sample was injected into the UltiMate 3,000 RSLCnano System (Thermo Fisher Scientific, Waltham, MA, USA). Peptide separation was performed using a C18 column at a flow rate of 300 nL/min. Mobile phase A consisted of 0.1% formic acid in water, whereas mobile phase B consisted of 80% acetonitrile in 0.1% formic acid. The eluent was then infused into a microTOF-Q mass spectrometer (Bruker Daltonics, Billerica, MA, USA). The mass spectra from the mass spectrometry (MS) and tandem MS covered the mass ranges of m/z 400–2000 and m/z 50–1,500, respectively.
A mascot generic file (.mgf) was generated using DataAnalysis 3.4 version software. Mascot Daemon version 2.3.2 (Matrix Science, London, UK) was used to identify the proteins. Identification and quantification of the proteins were performed against an NCBInr database (March 02, 2023) specific for dogs. Protein abundance was determined by peptide count analysis using the exponentially modified protein abundance index (emPAI) value (30). The emPAI is a label-free approach for protein quantification. It provides a relationship of direct proportionality between the numbers of observed and expected peptides (30). This technique could inform the relative quantification of each protein in the protein mixture which is more informative than crude protein concentration assay. Three biological replications were performed. Proteins with significantly different expression in the two groups were used to perform clustering analysis. A Venn diagram was used to illustrate differences in protein expression between the groups.
Proteins were classified by GO annotation based on three categories: biological processes, cellular components, and molecular function. This analysis helps to understand the biological functions and processes associated with the differentially expressed proteins.
The protein–protein interactions between differentially expressed proteins in a protein map were assessed using the Search Tool for the Retrieval of Interacting Genes (STRING) database.2 This database provides information about known and predicted interactions between proteins, thereby assisting in the exploration of protein networks and potential functional relationships. Next, the Kyoto Encyclopedia of Genes and Genomes (KEGG) database3 was used to classify differentially expressed proteins into hierarchical categories. Pathways with false discovery rates smaller than 0.05 were considered statistically significant. Protein function was identified using the UniProt database.4
To quantify Aβ42 levels in plasma, specific sandwich ELISA kits designed for human Aβ42 (Elabscience®, Wuhan, China) were used following the manufacturer’s instructions. Several evidences in canine studies have utilized human Aβ42 ELISA kit across species (31, 32) due to the identical amino acid sequence of Aβ42 between humans and dogs (33). Briefly, ELISA plates were coated with 100 μL of each plasma sample. Then, biotinylated antibody was added to the plates, followed by incubation for 1 h. After several wash steps, HRP conjugate working solution was added to each well. The wash step was repeated, and substrate solution was added to each well. The reaction was stopped by the addition of stop solution. The absorbance of the samples was measured at a wavelength of 450 nm.
The statistical significance of differences between the groups was determined using a paired non-parametric Student’s t-test. Statistical calculations were performed using GraphPad Prism version 5.0 and significance was indicated by p < 0.05.
The mean plasma Aβ42 level with refractory idiopathic epilepsy was significantly greater levels when compared to normal dogs (99 pg/mL vs. 5.9 pg/mL, p < 0.05, Figure 1). Similarly, it was observed that dogs with cognitive impairment exhibited increased levels of plasma Aβ42 (31, 32, 34).
Plasma from dogs in the healthy control and epilepsy groups were pooled and prepared to measure differential protein expression using a proteomic approach. The protein bands in one-dimensional gel electrophoresis of both groups were separated into 11 pieces prior to in-gel trypsin digestion (Figure 2). Of the 155 canine proteins examined, 53 (34.19%) were commonly expressed in dogs in both groups (Figure 3).
Figure 3. Venn diagram of detected proteins in the healthy control and epilepsy groups (Canis spp.).
The downregulated and upregulated proteins in dogs with epilepsy compared to those in healthy dogs were specifically involved in several biological processes. Differential protein expression was demonstrated via semi-quantification by selecting the altered proteins with at least two replicates (35, 36). Of the 155 examined proteins, 40 displayed at least 1.5-fold differences in expression between the healthy control and epilepsy groups according to the emPAI values.
Pathway enrichment analysis was performed to map the proteins onto GO databases via PANTHER using three primary categories: biological process, protein class, and molecular function. In the GO molecular function category, the differentially expressed proteins between the healthy control and epilepsy groups were divided into four groups: biological process, molecular function regulator, protein class, and cellular component (Figure 4). Among the differentially expressed proteins in the epilepsy group, six, six, and five were mapped within cellular processes, response to stimuli, and biological regulation, respectively, in the biological process category (Figure 4A). Five proteins were clustered within catalytic activity and five proteins were involved in binding, molecular function regulation, and molecular transducer activity in the molecular function category (Figure 4B). Eleven proteins participated in defense/immunity protein in the protein class category (Figure 4C), and eight proteins participated in cellular anatomical entity in the cellular component category (Figure 4D).
Figure 4. GO annotation of; (A) biological process, (B) molecular function, (C) protein class, and (D) cellular component, for differentially expressed proteins between the healthy control and epilepsy groups.
These results illustrated compared to the findings in healthy dogs, biological processes such as cellular activities, localization, response to stimuli, and critical molecular activities such as catalytic activity, molecular function regulation, and binding were disrupted in dogs with epilepsy. Defense/immunity proteins were the most dramatically altered proteins throughout the development of epilepsy.
Twelve proteins were upregulated in the epilepsy group, whereas 10 proteins were downregulated (Table 1). To predict the cellular functions of differentially expressed proteins, their localization was analyzed in this study. Using subcellular location analysis with data from the UniProt annotation database, the distribution of the proteins was as follows: secreted, 14 (63.64); endoplasmic reticulum, 1 (4.55%); cell membrane, 3 (13.64%); cytoplasm, 4 (18.18%); mitochondria, 1 (4.55%); cytoskeleton, 2 (9.09%); and extracellular space, 1 (4.55%, Table 1). The proteins of interest in dogs, identified from the MS/MS data reported here, exhibited a confidence level above 95%, as determined by the false discovery rate.
To explore the potential proteins involved in the pathogenesis of canine epilepsy and Aβ42 metabolism, we performed pathway analysis using STRING version 11.0. Total protein changes in the epilepsy group in comparison with the healthy control group were expanded to illustrate evidence of interactions, resulted in the identification of 17 proteins. We compared this protein set to those in the GO and KEGG databases. The proteins related with Aβ were haptoglobin (HP), α2-macroglobulin, ceruloplasmin, complement factor H (CFH), and gelsolin. The proteins potentially associated with the pathogenesis of epilepsy are involved in complement and coagulation cascades (Figure 5).
Figure 5. Protein differences between the healthy control and epilepsy group by STRING. Red = complement and coagulation cascades (KEGG pathway). Proteins in green circles are proteins of interest related to Aβ42 (APP, amyloid beta A4 protein; A2M, α2-macroglobulin; CP, ceruloplasmin; GSN, gelsolin; GC, vitamin D binding protein; LOC479668, haptoglobin).
A growing body of research has suggested an association between AD and epilepsy (18). Canine refractory epilepsy have a higher risk of developing CDS at a younger age than normal dogs (12). Multiple studies in animals and humans have revealed an association between Aβ and epilepsy (37–39). In our study, we first demonstrated significantly higher plasma Aβ levels in dogs with refractory epilepsy. Plasma Aβ42 levels in healthy dogs in this study were comparable to those recorded in a previous study (10.99 ± 5.45 pg/mL) (31), and plasma Aβ42 levels were elevated in age-matched dogs with epilepsy. The plasma proteomic pattern was assessed, and five major proteins potentially involved in the pathogenesis of epilepsy and elevated Aβ levels were identified: haptoglobin (HP), α2-macroglobulin, ceruloplasmin, complement factor H (CFH), and gelsolin.
The interplay between epilepsy and cognitive dysfunction has been studied in dogs. However, the evidence demonstrating that Aβ levels are higher in dogs with than in healthy controls is limited. A previous study found that dogs with severe early-onset epilepsy had higher Aβ accumulation and greater neuronal degeneration in the brain than healthy controls (40). Additionally, substantial evidence has revealed an association between Aβ and epilepsy in animals and humans. For example, the presence of Aβ plaques was associated with an increased frequency and duration of epileptic spiking in APP/PS1 mice (37). Aβ protein levels and their relationship with cognitive function have been investigated in patients with refractory epilepsy by analyzing cortical biopsies from the temporal lobes. The results revealed a strong compelling of Aβ deposits in the biopsied tissues, supporting the existence of Aβ deposits in patients with refractory epilepsy (39). In patients with refractory seizures, increased Aβ precursor protein was detected in temporal lobe or hippocampal sections (41). A study on rats illustrated that epilepsy can lead to increased Aβ expression (42, 43). Seizures contribute to neurodegenerative processes by triggering electrical currents and promoting the production and release of Aβ (37, 44). The presence of Aβ42 leads to an increase in neuronal excitability in AD, which subsequently initiates the development of progressive epilepsy (17). Our findings demonstrate that increased levels of Aβ42 in dogs with treatment-resistant seizures may be attributed to prolonged epileptiform discharges in the brain, leading to the development of cognitive impairment. Further research is needed to fully understand the mechanisms underlying this relationship and to identify potential therapeutic targets.
Plasma HP, which is primarily produced by hepatocytes in the liver, is a particularly important hemoglobin-binding protein that removes hemoglobin from the circulation (45). Various additional functions of HP have been discovered, including serving as an acute phase 2-acid response glycoprotein, an antioxidant of apolipoprotein E (APOE), an anti-inflammatory protein, and an Aβ clearance facilitator (46, 47). In the current study, HP was significantly upregulated in the epilepsy group compared to that in the healthy control group. A potential association of HP with neurological disorders was discovered. Significant upregulation of HP, interferon gamma, and interleukin-1β was associated with refractory epilepsy, as determined by proteomic analysis of plasma isolated from children with refractory epilepsy (48). Serum HP levels were significantly higher in patients with idiopathic seizures than in healthy controls (49). Similarly, proteomic analysis of CSF from dogs with recurrent epileptic seizures revealed a significant increase in HP levels, suggesting that HP participates in disruption of the blood–brain barrier, which is potentially linked to the inflammatory response triggered by seizures within the brain (26). Patients with AD have significantly higher serum HP levels than healthy controls. Additionally, a significant positive correlation between the serum HP level and the severity of cognitive impairment was observed in patients with AD (47, 50). This association is believed to occur through the modification of the effects of APOE, another genetic factor implicated in AD progression (46). Although research on the relationship among HP, epilepsy, and Aβ is currently limited, the present findings suggest possible links among these factors.
In the present study, ceruloplasmin was only detected in dogs with epilepsy. Ceruloplasmin is a circulating copper-binding protein that participates in copper homeostasis, oxidative stress, and neuroinflammation, and its expression is increased during the acute phase response (51, 52). Evidence suggests that plasma ceruloplasmin levels reflect its levels in the brain (53). There is some evidence that ceruloplasmin is involved in seizure activity, although the exact relationship is not fully understood (28, 54, 55). Plasma ceruloplasmin concentrations and oxidase activity were substantially higher in adults with epilepsy than in age- and gender-matched controls (28). Ceruloplasmin mRNA expression in the peripheral blood was significantly higher in patients with refractory epilepsy than in drug-responsive patients and healthy controls. The researchers additionally found a link between increased ceruloplasmin expression and different treatment strategies, potentially revealing a resistance mechanism for combination medications used to treat refractory epilepsy (28). The relationship between epilepsy and partial duplication of the ceruloplasmin gene in mice in epilepsy, which is coinherited with seizures, was studied, and duplication of the gene was associated with increased ceruloplasmin mRNA expression and ceruloplasmin oxidase activity (55). In addition, the role of ceruloplasmin in Aβ metabolism has been studies. There is evidence that High CSF ceruloplasmin levels in patients with AD and underlying Aβ pathogenesis was related to faster cognitive deterioration (51). These studies suggest the involvement of ceruloplasmin in seizure activity and Aβ metabolism through its roles in oxidative stress and copper homeostasis.
In the present study, CFH was only detected in the plasma of dogs with epilepsy. The protein regulates complement system activity and modulates inflammation in the brain and periphery. Despite being mainly synthesized and secreted by the liver, CFH has been found in different tissues, including the brain (56). Recent studies have suggested a possible link between mutations or dysregulation of CFH and epilepsy. Ten complement analytes were measured in patients with focal or generalized epilepsy, and plasma CFH levels were significantly higher in such patients than in controls (56). Although the exact mechanisms underlying the relationship between CFH and seizures are not fully understood, it is believed that dysregulation of the complement system contributes to neuroinflammation and neuronal damage, leading to an increased risk of seizures. In addition, recent studies have attempted to demonstrate the possible relationship between CFH and Aβ in AD (57, 58). Plasma CFH levels were reduced in patients with late-onset AD, and this reduction was associated with serum C-reactive protein levels. Unfortunately, it was recently suggested that plasma CFH is not an appropriate biomarker for AD (57).
α2-Macroglobulin is a broad-spectrum proteinase inhibitor and an acute phase protein of the innate immune system that is extensively found in the plasma of animals and distributed in various body fluids, including CSF (59–61). The plasma levels of α2-macroglobulin and cytokines are elevated in patients with neurodegenerative diseases including AD and Parkinson’s disease (61). It has been proposed that increased activity of α2-macroglobulin has a role in AD pathogenesis involving Aβ plaque accumulation (62). Plasma α2-Macroglobulin functions as a carrier protein and specifically binds to soluble Aβ and facilitates its degradation (63–65). This process may help in the clearance of Aβ from tissues, including the brain. The current study showed a reduced expression of α2-macroglobulin in seizure group, which may result in a diminished clearance of Aβ. This can explain for why elevated plasma levels of Aβ were identified in the seizure group.
In the present study, plasma gelsolin was only detected in healthy dogs. Gelsolin is a calcium and phosphatidylinositol 4,5-bisphosphate–regulated protein with actin-binding properties that is involved in various cellular processes, including cell signaling, inflammation, and cytoskeletal organization (66). There is evidence of the potential of gelsolin as a biomarker for epilepsy, with people with epilepsy having considerably lower CSF levels of gelsolin than healthy controls. Gelsolin protein levels were similarly reduced in the temporal lobe in patients with epilepsy (67). Seizure-induced damage to hippocampal pyramidal neurons was exacerbated in gelsolin-deficient adult mice, suggesting that gelsolin activates N-methyl-D-aspartate receptors and voltage-dependent calcium channels, leading to pathophysiological events (68). In addition, gelsolin m expression was lower in the hippocampus of mice with epilepsy and seizures than in mice without seizures (69). Gelsolin is also considered to be involved in AD and the regulation of Aβ levels according to the finding that diverse gelsolin alterations are connected with the progression of AD (70, 71). Gelsolin administration or overexpression induced a considerable reduction in the amyloid burden and Aβ level in AD transgenic mice (70, 72). Greater plasma and CSF gelsolin concentrations were detected in patients with AD than in controls, and a positive association was found between gelsolin and Aβ42 levels in CSF. The increase in plasma gelsolin levels is most likely a compensatory reaction in AD (71).
In conclusion, we first demonstrated that plasma Aβ42 levels were significantly higher in dogs with refractory epilepsy than in healthy dogs. The plasma proteomic pattern was identified, and five major proteins potentially involved in the pathogenesis of epilepsy and control of Aβ levels, including HP, α2-macroglobulin, ceruloplasmin, CFH, and gelsolin, were identified. HP and α2-macroglobulin are proteins involved in acute phase, immunological, and inflammatory responses. HP expression was substantially elevated in the epilepsy group, indicating relationships with early neuronal injury and enhanced Aβ clearance. Conversely, α2-macroglobulin was significantly upregulated in the healthy control group, and the role of α2-macroglobulin in seizure etiology remains unknown. CFH and ceruloplasmin were only detected in the plasma of dogs with epilepsy, suggesting potential roles in neuroinflammation and seizures. Contrarily, gelsolin, which is involved in cellular processes and cytoskeletal organization, was only found in the plasma of healthy dogs. However, the exact mechanisms underlying these relationships and their implications in canine epilepsy require further research (Figure 6). Although the sample size of animals in the study is sufficient to examine the difference in plasma levels of β-amyloid42 between healthy dogs and those with refractory epilepsy, the proteomics analysis is limited by the small number of dogs. The present study demonstrated that proteomic analysis has the potential to identify novel biomarkers, mechanisms, and therapeutic targets for seizure disorders. However, further research is needed to validate these biomarkers and overcome the challenges associated with proteomic analysis in neurological disorders.
The study was approved by the Committee on the Care and Use of Laboratory Animals in the Faculty of Veterinary Science, Mahidol University, Thailand (approval number: MUVS-2020-08-37). The owners were informed and signed in the consent form.
The datasets presented in this study can be found in online repositories. The names of the repository and accession number can be found here: https://www.ebi.ac.uk/pride/, PXD043962.
The animal studies were approved by Committee on the Care and Use of Laboratory Animals in the Faculty of Veterinary Science, Mahidol University, Thailand (approval number: MUVS-2020-08-37). The studies were conducted in accordance with the local legislation and institutional requirements. Written informed consent was obtained from the owners for the participation of their animals in this study.
SP: Conceptualization, Data curation, Investigation, Validation, Visualization, Writing – original draft, Writing – review & editing. BC: Conceptualization, Methodology, Resources, Supervision, Validation, Visualization, Writing – original draft, Writing – review & editing. OR: Data curation, Resources, Writing – review & editing. DC: Conceptualization, Funding acquisition, Investigation, Methodology, Supervision, Validation, Visualization, Writing – original draft, Writing – review & editing.
The author(s) declare financial support was received for the research, authorship, and/or publication of this article. The work was supported by Agricultural Research Development Agency, Thailand (Grant Number CRP6305031600).
The authors are deeply grateful with the entire dog’s owner at Prasu-arthorn hospital, Faculty of Veterinary Science, Mahidol University for their generous response. The authors would like to thank Miss Tipparat Thiangtrongjit for technical assistance with the nano-LC–MS/MS procedure.
The authors declare that the research was conducted in the absence of any commercial or financial relationships that could be construed as a potential conflict of interest.
All claims expressed in this article are solely those of the authors and do not necessarily represent those of their affiliated organizations, or those of the publisher, the editors and the reviewers. Any product that may be evaluated in this article, or claim that may be made by its manufacturer, is not guaranteed or endorsed by the publisher.
The Supplementary material for this article can be found online at: https://www.frontiersin.org/articles/10.3389/fvets.2023.1258244/full#supplementary-material
1. De Risio, L, Bhatti, S, Muñana, K, Penderis, J, Stein, V, Tipold, A, et al. International veterinary epilepsy task force consensus proposal: diagnostic approach to epilepsy in dogs. BMC Vet Res. (2015) 11:148. doi: 10.1186/s12917-015-0462-1
2. Heske, L, Nødtvedt, A, Jäderlund, KH, Berendt, M, and Egenvall, A. A cohort study of epilepsy among 665, 000 insured dogs: incidence, mortality and survival after diagnosis. Vet J. (2014) 202:471–6. doi: 10.1016/j.tvjl.2014.09.023
3. Moore, SA. A clinical and diagnostic approach to the patient with seizures. Top Companion Anim Med. (2013) 28:46–50. doi: 10.1053/j.tcam.2013.07.002
4. March, PA. Seizures: classification, etiologies, and pathophysiology. Clin Tech Small Anim Pract. (1998) 13:119–31. doi: 10.1016/S1096-2867(98)80033-9
5. Booth, S, Meller, S, Packer, RM, Farquhar, R, Maddison, JE, and Volk, HA. Owner compliance in canine epilepsy. Vet Rec. (2021) 188:e16. doi: 10.1002/vetr.16
6. Potschka, H, Fischer, A, Löscher, W, and Volk, HA. Pathophysiology of drug-resistant canine epilepsy. Vet J. (2023) 296-297:105990. doi: 10.1016/j.tvjl.2023.105990
7. Eyo, UB, Murugan, M, and Wu, LJ. Microglia-neuron communication in epilepsy. Glia. (2017) 65:5–18. doi: 10.1002/glia.23006
8. López-Meraz, ML, and Álvarez-Croda, DM. Microglia and status epilepticus in the immature brain. Epilepsia Open. (2023) 8:S73–s81. doi: 10.1002/epi4.12610
9. Sun, Y, Ma, J, Li, D, Li, P, Zhou, X, Li, Y, et al. Interleukin-10 inhibits interleukin-1β production and inflammasome activation of microglia in epileptic seizures. J Neuroinflammation. (2019) 16:66. doi: 10.1186/s12974-019-1452-1
10. Yuen, AWC, Keezer, MR, and Sander, JW. Epilepsy is a neurological and a systemic disorder. Epilepsy Behav. (2018) 78:57–61. doi: 10.1016/j.yebeh.2017.10.010
11. Pearson-Smith, JN, and Patel, M. Metabolic dysfunction and oxidative stress in epilepsy. Int J Mol Sci. (2017) 18:2365. doi: 10.3390/ijms18112365
12. Packer, RMA, McGreevy, PD, Salvin, HE, Valenzuela, MJ, Chaplin, CM, and Volk, HA. Cognitive dysfunction in naturally occurring canine idiopathic epilepsy. PLoS One. (2018) 13:e0192182. doi: 10.1371/journal.pone.0192182
13. Winter, J, Packer, RMA, and Volk, HA. Preliminary assessment of cognitive impairments in canine idiopathic epilepsy. Vet Rec. (2018) 182:633. doi: 10.1136/vr.104603
14. Stefanidou, M, Beiser, AS, Himali, JJ, Peng, TJ, Devinsky, O, Seshadri, S, et al. Bi-directional association between epilepsy and dementia: the Framingham heart study. Neurology. (2020) 95:e3241–7. doi: 10.1212/WNL.0000000000011077
15. Mac Quiddy, B, Moreno, JA, Kusick, B, and McGrath, S. Assessment of risk factors in dogs with presumptive advanced canine cognitive dysfunction. Front Vet Sci. (2022) 9:958488. doi: 10.3389/fvets.2022.958488
16. Sen, A, Capelli, V, and Husain, M. Cognition and dementia in older patients with epilepsy. Brain. (2018) 141:1592–608. doi: 10.1093/brain/awy022
17. Minkeviciene, R, Rheims, S, Dobszay, MB, Zilberter, M, Hartikainen, J, Fülöp, L, et al. Amyloid beta-induced neuronal hyperexcitability triggers progressive epilepsy. J Neurosci. (2009) 29:3453–62. doi: 10.1523/JNEUROSCI.5215-08.2009
18. Yang, F, Chen, L, Yu, Y, Xu, T, Chen, L, Yang, W, et al. Alzheimer’s disease and epilepsy: An increasingly recognized comorbidity. Front Aging Neurosci. (2022) 14:940515. doi: 10.3389/fnagi.2022.940515
19. Dun, C, Zhang, Y, Yin, J, Su, B, Peng, X, and Liu, L. Bi-directional associations of epilepsy with dementia and Alzheimer’s disease: a systematic review and meta-analysis of longitudinal studies. Age Ageing. (2022) 51:afac010. doi: 10.1093/ageing/afac010
20. Subota, A, Pham, T, Jetté, N, Sauro, K, Lorenzetti, D, and Holroyd-Leduc, J. The association between dementia and epilepsy: a systematic review and meta-analysis. Epilepsia. (2017) 58:962–72. doi: 10.1111/epi.13744
21. Costa, C, Romoli, M, Liguori, C, Farotti, L, Eusebi, P, Bedetti, C, et al. Alzheimer’s disease and late-onset epilepsy of unknown origin: two faces of beta amyloid pathology. Neurobiol Aging. (2019) 73:61–7. doi: 10.1016/j.neurobiolaging.2018.09.006
22. Romoli, M, Sen, A, Parnetti, L, Calabresi, P, and Costa, C. Amyloid-β: a potential link between epilepsy and cognitive decline. Nat Rev Neurol. (2021) 17:469–85. doi: 10.1038/s41582-021-00505-9
23. Costa, C, Parnetti, L, D’Amelio, M, Tozzi, A, Tantucci, M, Romigi, A, et al. Epilepsy, amyloid-β, and D1 dopamine receptors: a possible pathogenetic link? Neurobiol Aging. (2016) 48:161–71. doi: 10.1016/j.neurobiolaging.2016.08.025
24. Zhang, D, Chen, S, Xu, S, Wu, J, Zhuang, Y, Cao, W, et al. The clinical correlation between Alzheimer’s disease and epilepsy. Front Neurol. (2022) 13:922535. doi: 10.3389/fneur.2022.922535
25. Altuna, M, Olmedo-Saura, G, Carmona-Iragui, M, and Fortea, J. Mechanisms involved in Epileptogenesis in Alzheimer& rsquo; s disease and their therapeutic implications. Int J Mol Sci. (2022) 23:4307. doi: 10.3390/ijms23084307
26. Baka, R, Eckersall, D, Horvatic, A, Gelemanovic, A, Mrljak, V, McLaughlin, M, et al. Quantitative proteomics of cerebrospinal fluid using tandem mass tags in dogs with recurrent epileptic seizures. J Proteome. (2021) 231:103997. doi: 10.1016/j.jprot.2020.103997
27. Liu, X-Y, Yang, J-L, Chen, L-J, Zhang, Y, Yang, M-L, Wu, Y-Y, et al. Comparative proteomics and correlated signaling network of rat hippocampus in the pilocarpine model of temporal lobe epilepsy. Proteomics. (2008) 8:582–603. doi: 10.1002/pmic.200700514
28. Chen, H-Y, Pan, Y-X, Li, X-B, Yun, Y-F, Yang, G-X, Jiang, Y-M, et al. Expression of ceruloplasmin in the peripheral blood of patients with drug-resistant epilepsy. J Clin Pharmacol. (2023) 63:466–72. doi: 10.1002/jcph.2183
29. Muñana, KR. Management of refractory epilepsy. Top Companion Anim Med. (2013) 28:67–71. doi: 10.1053/j.tcam.2013.06.007
30. Ishihama, Y, Oda, Y, Tabata, T, Sato, T, Nagasu, T, Rappsilber, J, et al. Exponentially modified protein abundance index (em PAI) for estimation of absolute protein amount in proteomics by the number of sequenced peptides per protein * S. Mol Cell Proteomics. (2005) 4:1265–72. doi: 10.1074/mcp.M500061-MCP200
31. Panek, WK, Murdoch, DM, Gruen, ME, Mowat, FM, Marek, RD, and Olby, NJ. Plasma amyloid Beta concentrations in aged and cognitively impaired pet dogs. Mol Neurobiol. (2021) 58:483–9. doi: 10.1007/s12035-020-02140-9
32. Stylianaki, I, Polizopoulou, ZS, Theodoridis, A, Koutouzidou, G, Baka, R, and Papaioannou, NG. Amyloid-beta plasma and cerebrospinal fluid biomarkers in aged dogs with cognitive dysfunction syndrome. J Vet Intern Med. (2020) 34:1532–40. doi: 10.1111/jvim.15812
33. Youssef, SA, Capucchio, MT, Rofina, JE, Chambers, JK, Uchida, K, Nakayama, H, et al. Pathology of the aging brain in domestic and laboratory animals, and animal models of human neurodegenerative diseases. Vet Pathol. (2016) 53:327–48. doi: 10.1177/0300985815623997
34. González-Martínez, Á, Rosado, B, Pesini, P, Suárez, ML, Santamarina, G, García-Belenguer, S, et al. Plasma β-amyloid peptides in canine aging and cognitive dysfunction as a model of Alzheimer’s disease. Exp Gerontol. (2011) 46:590–6. doi: 10.1016/j.exger.2011.02.013
35. Reamtong, O, Srimuang, K, Saralamba, N, Sangvanich, P, Day, NPJ, White, NJ, et al. Protein profiling of mefloquine resistant plasmodium falciparum using mass spectrometry-based proteomics. Int J Mass Spectrom. (2015) 391:82–92. doi: 10.1016/j.ijms.2015.09.009
36. Pinto, PM, Klein, CS, Zaha, A, and Ferreira, HB. Comparative proteomic analysis of pathogenic and non-pathogenic strains from the swine pathogen Mycoplasma hyopneumoniae. Proteome Sci. (2009) 7:45. doi: 10.1186/1477-5956-7-45
37. Gureviciene, I, Ishchenko, I, Ziyatdinova, S, Jin, N, Lipponen, A, Gurevicius, K, et al. Characterization of epileptic spiking associated with brain amyloidosis in APP/PS1 mice. Front Neurol. (2019) 10:1151. doi: 10.3389/fneur.2019.01151
38. Joutsa, J, Rinne, JO, Hermann, B, Karrasch, M, Anttinen, A, Shinnar, S, et al. Association between childhood-onset epilepsy and amyloid burden 5 decades later. JAMA Neurol. (2017) 74:583–90. doi: 10.1001/jamaneurol.2016.6091
39. Aroor, A, Nguyen, P, Li, Y, Das, R, Lugo, JN, and Brewster, AL. Assessment of tau phosphorylation and β-amyloid pathology in human drug-resistant epilepsy. Epilepsia Open. (2023) 8:609–22. doi: 10.1002/epi4.12744
40. Hytönen, MK, Sarviaho, R, Jackson, CB, Syrjä, P, Jokinen, T, Matiasek, K, et al. In-frame deletion in canine PITRM1 is associated with a severe early-onset epilepsy, mitochondrial dysfunction and neurodegeneration. Hum Genet. (2021) 140:1593–609. doi: 10.1007/s00439-021-02279-y
41. Sima, X, Xu, J, Li, J, Zhong, W, and You, C. Expression of β-amyloid precursor protein in refractory epilepsy. Mol Med Rep. (2014) 9:1242–8. doi: 10.3892/mmr.2014.1977
42. Jang, SS, Royston, SE, Lee, G, Wang, S, and Chung, HJ. Seizure-induced regulations of amyloid-β, STEP61, and STEP61 substrates involved in hippocampal synaptic plasticity. Neural Plast. (2016) 2016:1–13. doi: 10.1155/2016/2123748
43. Dolev, I, Fogel, H, Milshtein, H, Berdichevsky, Y, Lipstein, N, Brose, N, et al. Spike bursts increase amyloid-β 40/42 ratio by inducing a presenilin-1 conformational change. Nat Neurosci. (2013) 16:587–95. doi: 10.1038/nn.3376
44. Gourmaud, S, Shou, H, Irwin, DJ, Sansalone, K, Jacobs, LM, Lucas, TH, et al. Alzheimer-like amyloid and tau alterations associated with cognitive deficit in temporal lobe epilepsy. Brain. (2020) 143:191–209. doi: 10.1093/brain/awz381
46. Bai, H, Naj, AC, Benchek, P, Dumitrescu, L, Hohman, T, Hamilton-Nelson, K, et al. A haptoglobin (HP) structural variant alters the effect of APOE alleles on Alzheimer’s disease. Alzheimers Dement. (2023) 19:4886–95. doi: 10.1002/alz.13050
47. Song, IU, Kim, YD, Chung, SW, and Cho, HJ. Association between serum haptoglobin and the pathogenesis of Alzheimer’s disease. Intern Med. (2015) 54:453–7. doi: 10.2169/internalmedicine.54.2876
48. Saengow, VE, Chiangjong, W, Khongkhatithum, C, Changtong, C, Chokchaichamnankit, D, Weeraphan, C, et al. Proteomic analysis reveals plasma haptoglobin, interferon-γ, and interleukin-1β as potential biomarkers of pediatric refractory epilepsy. Brain Dev. (2021) 43:431–9. doi: 10.1016/j.braindev.2020.11.001
49. Sadrzadeh, SM, Saffari, Y, and Bozorgmehr, J. Haptoglobin phenotypes in epilepsy. Clin Chem. (2004) 50:1095–7. doi: 10.1373/clinchem.2003.028001
50. Zhu, CJ, Jiang, GX, Chen, JM, Zhou, ZM, and Cheng, Q. Serum haptoglobin in Chinese patients with Alzheimer’s disease and mild cognitive impairment: a case-control study. Brain Res Bull. (2018) 137:301–5. doi: 10.1016/j.brainresbull.2018.01.005
51. Diouf, I, Bush, AI, and Ayton, S. Cerebrospinal fluid ceruloplasmin levels predict cognitive decline and brain atrophy in people with underlying β-amyloid pathology. Neurobiol Dis. (2020) 139:104810. doi: 10.1016/j.nbd.2020.104810
52. Brewer, GJ, Kanzer, SH, Zimmerman, EA, Celmins, DF, Heckman, SM, and Dick, R. Copper and ceruloplasmin abnormalities in Alzheimer’s disease. Am J Alzheimers Dis Other Dement. (2010) 25:490–7. doi: 10.1177/1533317510375083
53. Wang, B, and Wang, XP. Does Ceruloplasmin defend against neurodegenerative diseases? Curr Neuropharmacol. (2019) 17:539–49. doi: 10.2174/1570159X16666180508113025
54. Tutor-Crespo, MJ, Hermida, J, and Tutor, JC. Assessment of copper status in epileptic patients treated with anticonvulsant drugs by measuring the specific oxidase activity of ceruloplasmin. Epilepsy Res. (2003) 56:147–53. doi: 10.1016/j.eplepsyres.2003.08.008
55. Garey, CE, Schwarzman, AL, Rise, ML, and Seyfried, TN. Ceruloplasmin gene defect associated with epilepsy in EL mice. Nat Genet. (1994) 6:426–31. doi: 10.1038/ng0494-426
56. Kopczynska, M, Zelek, WM, Vespa, S, Touchard, S, Wardle, M, Loveless, S, et al. Complement system biomarkers in epilepsy. Seizure. (2018) 60:1–7. doi: 10.1016/j.seizure.2018.05.016
57. Williams, MA, Haughton, D, Stevenson, M, Craig, D, Passmore, AP, and Silvestri, G. Plasma complement factor H in Alzheimer’s disease. J Alzheimers Dis. (2015) 45:369–72. doi: 10.3233/JAD-142742
58. Lu, G, Liu, W, Huang, X, and Zhao, Y. Complement factor H levels are decreased and correlated with serum C-reactive protein in late-onset Alzheimer’s disease. Arq Neuropsiquiatr. (2020) 78:76–80. doi: 10.1590/0004-282x20190151
59. Garcia-Ferrer, I, Marrero, A, Gomis-Rüth, FX, and Goulas, T. α2-macroglobulins: structure and function In: JR Harris and J Marles-Wright, editors. Macromolecular protein complexes: structure and function. Cham: Springer International Publishing (2017). 149–83.
60. Garton, MJ, Keir, G, Lakshmi, MV, and Thompson, EJ. Age-related changes in cerebrospinal fluid protein concentrations. J Neurol Sci. (1991) 104:74–80. doi: 10.1016/0022-510X(91)90218-V
61. Varma, VR, Varma, S, An, Y, Hohman, TJ, Seddighi, S, Casanova, R, et al. Alpha-2 macroglobulin in Alzheimer’s disease: a marker of neuronal injury through the RCAN1 pathway. Mol Psychiatry. (2017) 22:13–23. doi: 10.1038/mp.2016.206
62. Wetterling, T, and Tegtmeyer, KF. Serum alpha 1-antitrypsin and alpha 2-macroglobulin in Alzheimer’s and Binswanger’s disease. Clin Investig. (1994) 72:196–9. doi: 10.1007/BF00189310
63. Lauer, D, Reichenbach, A, and Birkenmeier, G. α2-macroglobulin-mediated degradation of amyloid β1–42: a mechanism to enhance amyloid β catabolism. Exp Neurol. (2001) 167:385–92. doi: 10.1006/exnr.2000.7569
64. Cater, JH, Wilson, MR, and Wyatt, AR. Alpha-2-macroglobulin, a hypochlorite-regulated chaperone and immune system modulator. Oxidative Med Cell Longev. (2019) 2019:1–9. doi: 10.1155/2019/5410657
65. Kovacs, DM. α2-macroglobulin in late-onset Alzheimer’s disease. Exp Gerontol. (2000) 35:473–9. doi: 10.1016/S0531-5565(00)00113-3
66. Suprewicz, Ł, Tran, KA, Piktel, E, Fiedoruk, K, Janmey, PA, Galie, PA, et al. Recombinant human plasma gelsolin reverses increased permeability of the blood–brain barrier induced by the spike protein of the SARS-CoV-2 virus. J Neuroinflammation. (2022) 19:282. doi: 10.1186/s12974-022-02642-4
67. Peng, X, Zhang, X, Wang, L, Zhu, Q, Luo, J, Wang, W, et al. Gelsolin in cerebrospinal fluid as a potential biomarker of epilepsy. Neurochem Res. (2011) 36:2250–8. doi: 10.1007/s11064-011-0549-4
68. Furukawa, K, Fu, W, Li, Y, Witke, W, Kwiatkowski, DJ, and Mattson, MP. The actin-severing protein gelsolin modulates calcium channel and NMDA receptor activities and vulnerability to excitotoxicity in hippocampal neurons. J Neurosci. (1997) 17:8178–86. doi: 10.1523/JNEUROSCI.17-21-08178.1997
69. Lee, T-S, Li, AY, Rapuano, A, Mantis, J, Eid, T, Seyfried, TN, et al. Gene expression in the epileptic (EL) mouse hippocampus. Neurobiol Dis. (2021) 147:105152. doi: 10.1016/j.nbd.2020.105152
70. Ji, L, Zhao, X, and Hua, Z. Potential therapeutic implications of gelsolin in Alzheimer’s disease. J Alzheimers Dis. (2015) 44:13–25. doi: 10.3233/JAD-141548
71. Jiang, Y, Wan, M, Xiao, X, Lin, Z, Liu, X, Zhou, Y, et al. GSN gene frameshift mutations in Alzheimer’s disease. J Neurol Neurosurg Psychiatry. (2023) 94:436–47. doi: 10.1136/jnnp-2022-330465
Keywords: amyloid beta, epilepsy, proteomics, canine, signaling pathways
Citation: Phochantachinda S, Chantong B, Reamtong O and Chatchaisak D (2023) Protein profiling and assessment of amyloid beta levels in plasma in canine refractory epilepsy. Front. Vet. Sci. 10:1258244. doi: 10.3389/fvets.2023.1258244
Received: 13 July 2023; Accepted: 01 December 2023;
Published: 21 December 2023.
Edited by:
Elsayed Metwally, Suez Canal University, EgyptReviewed by:
Sam Long, Veterinary Referral Hospital, AustraliaCopyright © 2023 Phochantachinda, Chantong, Reamtong and Chatchaisak. This is an open-access article distributed under the terms of the Creative Commons Attribution License (CC BY). The use, distribution or reproduction in other forums is permitted, provided the original author(s) and the copyright owner(s) are credited and that the original publication in this journal is cited, in accordance with accepted academic practice. No use, distribution or reproduction is permitted which does not comply with these terms.
*Correspondence: Duangthip Chatchaisak, ZHVhbmd0aGlwLmNoYUBtYWhpZG9sLmVkdQ==
Disclaimer: All claims expressed in this article are solely those of the authors and do not necessarily represent those of their affiliated organizations, or those of the publisher, the editors and the reviewers. Any product that may be evaluated in this article or claim that may be made by its manufacturer is not guaranteed or endorsed by the publisher.
Research integrity at Frontiers
Learn more about the work of our research integrity team to safeguard the quality of each article we publish.