- 1Engineering Laboratory for Tarim Animal Diseases Diagnosis and Control, College of Animal Science and Technology, Tarim University, Alar, Xinjiang, China
- 2The State Key Laboratory of Agricultural Microbiology, College of Veterinary Medicine, Huazhong Agricultural University, Wuhan, China
- 3Department of Medicine and Infectious Diseases, Faculty of Veterinary Medicine, University of Sadat City, Sadat City, Egypt
- 4Key Laboratory of Ministry of Education for Conservation and Utilization of Special Biological Resources in the Western China, School of Life Sciences, Ningxia University, Yinchuan, China
- 5National Reference Laboratory of Veterinary Drug Residues (HZAU) and MAO Key Laboratory for Detection of Veterinary Drug Residues (HZAU), Wuhan, China
- 6Department of Clinical Pathology, Faculty of Veterinary Medicine, Benha University, Toukh, Egypt
- 7Key Laboratory of Veterinary Biological Engineering and Technology, Institute of Veterinary Medicine, Jiangsu Academy of Agricultural Sciences, Nanjing, China
Brucellosis is considered one of the most hazardous zoonotic diseases all over the world. It causes formidable economic losses in developed and developing countries. Despite the significant attempts to get rid of Brucella pathogens in many parts of the world, the disease continues to spread widely. Recently, many attempts proved to be effective for the prevention and control of highly contagious bovine brucellosis, which could be followed by others to achieve a prosperous future without rampant Brucella pathogens. In this study, the updated view for worldwide Brucella distribution, possible predisposing factors for emerging Brucella pathogens, immune response and different types of Brucella vaccines, genomics and proteomics approaches incorporated recently in the field of brucellosis, and future perspectives for prevention and control of bovine brucellosis have been discussed comprehensively. So, the current study will be used as a guide for researchers in planning their future work, which will pave the way for a new world without these highly contagious pathogens that have been infecting and threatening the health of humans and terrestrial animals.
1. Introduction
Brucellosis is a zoonotic infection caused by intracellular Brucella pathogens (1). Twelve species are now considered in the genus Brucella that infect different domestic and wildlife animal species (2). Among them, six Brucella species have been classified according to their pathogenicity and natural hosts as Brucella (B.) abortus (cattle), B. melitensis (sheep and goats), B. suis (pigs), B. canis (dogs), B. ovis (rams), and B. neotomae (Common voles and desert wood rat) (3, 4). B. melitensis and B. abortus are the most significant species in animals and humans (5). Later, two new Brucella spp., namely, B. pinnipedialis (walruses and seals) and B. ceti (dolphins, porpoises, and whales), have been reported to infect marine mammals (6). Brucellosis is a major health concern for animals because it causes significant economic losses in developing countries across the world in the form of abortion, stillbirth, delayed conception, retained placenta, reduced milk production, contagious epididymitis, and the cost of treatment and productivity loss (7) (Table 1). Abortion and infertility are the most common clinical symptoms of animal brucellosis, and they are not specific in nature. Abortion always occurs during the first pregnancy, but it is less commonly present after that due to persistent immunity (24). In addition, Xie et al. proved that there are three parameters, including animal species, vaccination dose, and immunization route, that were statistically linked to the occurrence of abortion as an adverse reaction to Brucella vaccination (25). Since it is easily transmissible by exposure to aborted fetuses, placentae, vaginal fluids, and milk, congenitally and/or venereally, the disease is very contagious in all circumstances (26).
Human infections mostly occur after contact with infected cattle, sheep, goats, and camels. In humans, brucellosis is characterized by a variety of non-specific symptoms such as malaise, lethargy, arthritis, and fever. These symptoms are mostly chronic lasting for years, and treatment needs to ensure compliance with a costly and prolonged therapy (27). It also can be manifested as a neurological disorder (28). People can get the infection by contact with infected animals during their usual work (occupational) as in the case of veterinarians, abattoir workers, and livestock keepers. Consumption of unpasteurized milk is also regarded as a common source of infection (29). Although pasteurizing milk kills Brucella and prevents infection in humans, it is not commonly performed in some communities with a widespread lack of public awareness about the danger of drinking raw milk (30). In addition, human-to-human transmission was reported via breastfeeding, hardly through sexual intercourse, blood transfusions, organ transplantation, and transplacentally (31). Brucella infections are more likely to be prevented and controlled when immunization is combined with adequate preventive measures (32).
As a result of increasing the severity of brucellosis, in this study, we described its global distribution and possible predisposing factors that facilitate the infection’s spreading. The immune response and how Brucella can evade the host immune system, as well as the recent trends and advances in the diagnosis, vaccination, treatment, prevention, and control of bovine brucellosis, have been discussed comprehensively. Subsequently, this review can be used as a guide for veterinarians and health workers to stop the further spread of this contagion all over the world.
2. Brucellosis worldwide
Brucellosis is one of the most common zoonotic diseases that increase poverty levels in poor nations (1). It has a negative influence on the world economy and public health. Annually, from 5 to 12.5 million human cases of brucellosis are reported, making it one of the most serious zoonoses on the globe (33). Human Brucella seroprevalence has been reported in many countries all over the world, especially among a variety of highly occupational groups as follows: China (15.5%) (34); India (1.83%) (35); Pakistan (17%) (36); Malaysia (5.4%) (37); Saudi Arabia (33.9%) (38); Greece (3%) (39); Egypt (31.3%) (40); South Sudan (33.3%) (41); Nigeria (17.6%) (42); Cameroon (5.6%) (43); Kenya (32.3%) (44); Uganda (17%) (45), and Tanzania (1.41%) (46) (Supplementary Table S1).
The annual incidence rate of human brucellosis is between 0.5/100,000 and 70/100,000, depending on the area of study (47), while the prevalence of brucellosis in animals ranges from 0.2 to 20%, depending on the location and species (48) (Supplementary Table S1). Azerbaijan is now ranked 13th in the world for the incidence of human brucellosis, with an estimated yearly incidence of over 50 cases per million (49). Human brucellosis has recently been linked to raw milk consumption and the RB51 vaccination status (50, 51). Therefore, the raw milk movement in the United States has been controlled, and numerous states have approved legislation that restricts the selling of raw milk and raw milk products to customers. Nowadays, less than 100 cases are recorded annually in the United States, with the majority coming in the South and Southwest from illegally imported soft cheeses (unpasteurized) from Mexico (52). In Tanzania, the upfront cost of pasteurization facilities is now unaffordable; hence, alternative management techniques are regarded as more cost-effective. In a Tanzanian investigation, 56% of the inspected milk samples (n = 59) tested positive for brucellosis (53).
Brucella is regarded as a spillover organism that begins to affect other species suddenly. From this point, cattle can be infected with both B. melitensis and B. abortus (49). In Azerbaijan, milk and blood samples were collected during early lactation in farms with a history of abortions and previous seropositive results for brucellosis. Out of 57 milk samples collected from seropositive cows, 22 samples revealed microbial growth on Farrell’s media with 5% CO2 incubation. On the other hand, 8 milk samples revealed growth in the absence of CO2. According to biotyping classification, these 22 and 8 samples were classified as B. abortus and B. melitensis, respectively. By using multiple-locus variable number tandem repeat analysis (MLVA) and matching the two types of Brucella, B. abortus strains were matched with B. abortus strains isolated in East Europe, Central Asia, and China. B. melitensis strains had a new genotype circulating among cattle, sheep, and goats in Azerbaijan, and these strains belong to the American clade that is hardly identified in the region (49). In Mongolia, seropositive camel herds were closely associated with cattle infection (54). In China, the total prevalence of bovine brucellosis was 1.9% according to a meta-analysis conducted over a 10-year period (2008–2018). Northern China has a higher prevalence than Southern China. More specifically, Jilin province has the highest prevalence of more than 30% (55). During previous Pakistani research, RBPT analysis of cow and buffalo samples revealed that 170 serum samples (6.3%) and 47 herds (18.6%) were seropositive for brucellosis (56). In a recent meta-analysis of animal diseases in North Eastern India, bovine brucellosis was shown to be prevalent in 17% of cattle (57). In addition, in India, Pathak et al. examined 481 samples from 296 animals, including milk, blood, vaginal swabs, vaginal discharges, placental tissue, and fetal tissues. Of these samples, 30.4 and 41.6% were positive for brucellosis using RBPT and iELISA, respectively, whereas 27.0% were seropositive by both tests (58). In domestic animals, only Canada, the United States, Japan, Australia, New Zealand, and Western and Central European countries are almost free of both B. abortus and B. melitensis (59).
On the other hand, the disease has a devastating impact on Africa, the Middle East, Latin America, Eastern Europe, and large parts of Asia (60). In Jordan, Musallam et al. estimated seroprevalence levels of 18.1% in cow herds (61). In Egypt, the prevalence frequency varies greatly across the country (62). B. melitensis, B. abortus, and B. suis are the three zoonotic Brucella species found in Egypt. B. melitensis biovar 3 is the most frequent Brucella isolate in Egypt right now in both livestock and people (63). Hosein et al. studied the outbreak in buffalo caused by B. melitensis biovar 3. The study revealed that being free of brucellosis required 6 months, which is considered a long time for transmitting infection to other areas, particularly in unhygienic conditions and husbandry systems that allow mixed populations of different ages and sexes and abortion with a lack of controlled animal movement (64). A novel Brucella strain closely related to B. melitensis biovar 3 was identified in Croatian cattle during testing within a brucellosis eradication program (65). Chaka et al. discovered that the herd-level prevalence of brucellosis in Ethiopian cattle was 32%, while an overall cattle-level prevalence of 9.7% was recorded dependent on serological tests (66). Moreover, Getachew et al. tested 278 serum samples for brucellosis in dairy animals in Ethiopia by RBPT, indirect ELISA (iELISA), and complement fixation test (CFT). The sensitivity was reported as 89.6, 96.8, and 94%, respectively, and the specificity was reported as 84.5, 96.3, and 88.5%, respectively. Comparing the three tests, iELISA was shown to have the highest sensitivity and specificity (67).
In Abuja, Nigeria, Aworh et al. tested 376 cattle for Brucella infection. Twenty-one animals were RBPT positive, and two animals gave positive competitive ELISA (cELISA) results (68). Using the milk ring test and iELISA, Kamwine et al. reported a prevalence of 26.5% of Brucella in 185 raw milk samples in Uganda (69). In Tanzania, milk samples were used for iELISA-based Brucella surveillance, and the study revealed a herd frequency of 44.4% (70). In Ghana, blood samples were collected from 315 cattle and 178 cattle farmers to measure Brucella seroprevalence, and the RBPT results demonstrated 22.9 and 10.1% in bovines and humans, respectively (71). Madut et al. demonstrated a high prevalence level of brucellosis in cattle and their owners in Bahr El-Ghazal, Sudan (41). In Ecuador, brucellosis seroprevalence and risk variables have been investigated in dairy and dairy-beef mixed cattle herds, and the results indicated that the true Brucella seropositivity was 17.0% (72) (Figure 1).
3. Risk and predisposing factors for bovine brucellosis
Common risk factors related to the difficulty of brucellosis control are social and political instabilities and inappropriate diagnosis, reporting, and application of control measures. Livestock husbandry systems and their interactions with other animals and wildlife, as well as using unpasteurized dairy products as one of the traditional cultural habits, played a major role in transmitting brucellosis (32, 73–75) (Figure 2).
The outbreaks of brucellosis in animals are increasingly important nowadays because of its asymptomatic features in humans. The follow-up outcomes of asymptomatic brucellosis without treatment were investigated in 3,610 studies from 1990 to 2021. Of which, 13 studies included that during a 0.5- to 18-month follow-up period, 40.3% of the cases remained asymptomatic. Moreover, investigations on brucellosis transmission vary between different geographies and populations (76). Moreover, many studies have detected substantial correlations among species, gender, age, and breed of animals with seropositivity (77–79). Lack of clean water, insufficient manure collection, neglected cleaning, bad management of aborted materials, the introduction of new animals from infected herds or herds with unclear status, and mixed herds are among the other risk factors that have been highlighted (80) (Table 2). Also, various studies have shown that brucellosis has a significant influence on Middle Eastern countries, where contaminated milk products have been responsible for several outbreaks of human brucellosis during the previous two decades (75, 91). The knowledge and awareness of farmers about brucellosis significantly reduce Brucella infection in animals (98). The large-scale organized dairy farms have higher incidence rates than the individual native animals, which could be related to the disease’s higher prevalence in exotic and cross-bred animals compared to native cattle (77). Disease transmission usually occurs through natural mating/artificial insemination and physical contact and exposure to affected animals (99). The microorganism is more vulnerable to infection in sexually mature pregnant cattle than in sexually immature cattle of either sex, and susceptibility increases as the pregnancy progresses (100). Most animals get infected and harbor infection for the rest of their lives.
The prevalence of disease and the difficulty of controlling infection in a population are closely related to herd size and animal density (101). Animal species also affect disease susceptibility, and so a survey carried out for cattle, camels, sheep, and goats in a pastoral area in Isiolo County, Kenya, using RBPT and iELISA showed that camels and cattle have a greater incidence of brucellosis than sheep and goats (102).
According to the chairman of the Egyptian Chamber of Food Industries, social misconceptions about the nutritional quality of pasteurized milk have led to a decrease in the consumption of commercial milk products. More and more people buy fresh, unprocessed raw milk from small-scale dairy producers to account for up to 80% of the total milk industry (up to 4 billion liters per year) (103). In Fayoum province, Upper Egypt, Abdel-Hamid and her group have investigated the risk factors and molecular genotyping of B. melitensis strains. From a total of 183 cattle cases, 78 (42.6%) were seropositive cattle cases. All seropositive cattle were female, and 85.9% (n = 67) of them were older than 2 years. One-third of the seropositive cattle (n = 28) were reared alone. Nine of the seropositive cattle (11.5%) and one of the seronegative cattle (0.9%) had abortions. According to the socio-demographic variables, uneducated individuals, workers of an animal-related occupation, or owners of household cattle had a greater risk of brucellosis. In addition, in a multivariable model, soft cheese consumption was 2.3 times more likely to be responsible for brucellosis cases than controls (p 0.03) (104).
In France, the country has been declared free since 2005. So, a study was performed to measure the impact of veterinarians and all local institutional stakeholders on the compulsory notification of bovine abortions by farmers. The results showed that the proportion of notifying farmers was influenced by the number of veterinarians per practice and the veterinary practice’s membership of a technical association (105). In South China, the major source of brucellosis for human infection was goats, so a cross-sectional study was performed to investigate the true prevalence and risk factors for goat brucellosis in Ningxiang County, South China. The herd prevalence in commercial goat farms was 4.5% with nine potential risk factors identified by logistic regression analysis (p < 0.2). Among these factors, introduction in the past 12 months, self-breeding, and safe disposal of sick or dead animals were the factors with the strongest association with disease presence (106). In Nepal, another cross-sectional study was performed to investigate brucellosis in sheep and goats. At the farm level, 31.6% of sheep farms and 3.3% of goat farms were seropositive to brucellosis. Age > 1.5 years (older sheep; p 0.02) and herd size >100 (larger herds; p 0.03) were demonstrated as significant risk factors for brucellosis in the sheep population. While in the goat population, none of them was identified as a significant factor. Because sheep were frequently moved for grazing and selling, a management scheme for the sheep population should be implemented with strict biosecurity (107).
4. Immunity
4.1. Innate immunity
The first line of defense against pathogen attacks is the innate immune system. Anatomical barriers (skin and internal epithelial layers), secretory molecules (chemokines and cytokines, complement system, and opsonins), cellular populations such as phagocytes [neutrophils, monocytes/macrophages, and dendritic cells (DCs)], and innate lymphocyte subsets [natural killer (NK) cells and T cells] are all parts of the innate immune system (108). Brucella antigenic components, such as lipopolysaccharide (LPS), Type IV secretion system (T4SS), BvrR/BvrS, outer membrane protein (Omp), proline racemase subunit A (PrpA), and Btp1, regulate the unique techniques that enable Brucella to elude the immune system. Intracellular survival, slowed phagocytosis, antibacterial action, and tumor necrosis factor-alpha (TNF-α) production are among these mechanisms (109).
Brucella are considered resilient pathogens that elude innate immunity and stimulate polymorphonuclear cells (PMNCs) to enhance their microbicidal activity, allowing leukocytes to live longer and resist phagocyte mechanisms (110). It was discovered that eliminating PMNCs before inducing adaptive immunity helped mice to get rid of bacteria, which in turn proved that neutrophils suppress the host immune response against Brucella (111). The interaction of pattern recognition receptors (PRRs) and pathogen-associated molecular patterns (PAMPs) allows host cells to recognize threatening compounds and encourages the body to figure out how to fight pathogens (112). Brucella LPS influences the complement system; thereby, the O-chain lacking a free hydroxyl is beneficial for connecting with C3, which suppresses the formation of C3a and C5a by associating with Brucella LPS’s unique O-chain and avoiding host immunity (113) (Figure 3). Brucella flagellin is also crucial for immune evasion and lacks the distinctive characteristic identified by toll-like receptor-5 (TLR5) (114). In addition, host peroxiredoxin 6 (Prdx6), a bifunctional protein with peroxidase and phospholipase activities, has a function in Brucella infection by increasing the intracellular survival of the B. suis S2 strain (115).
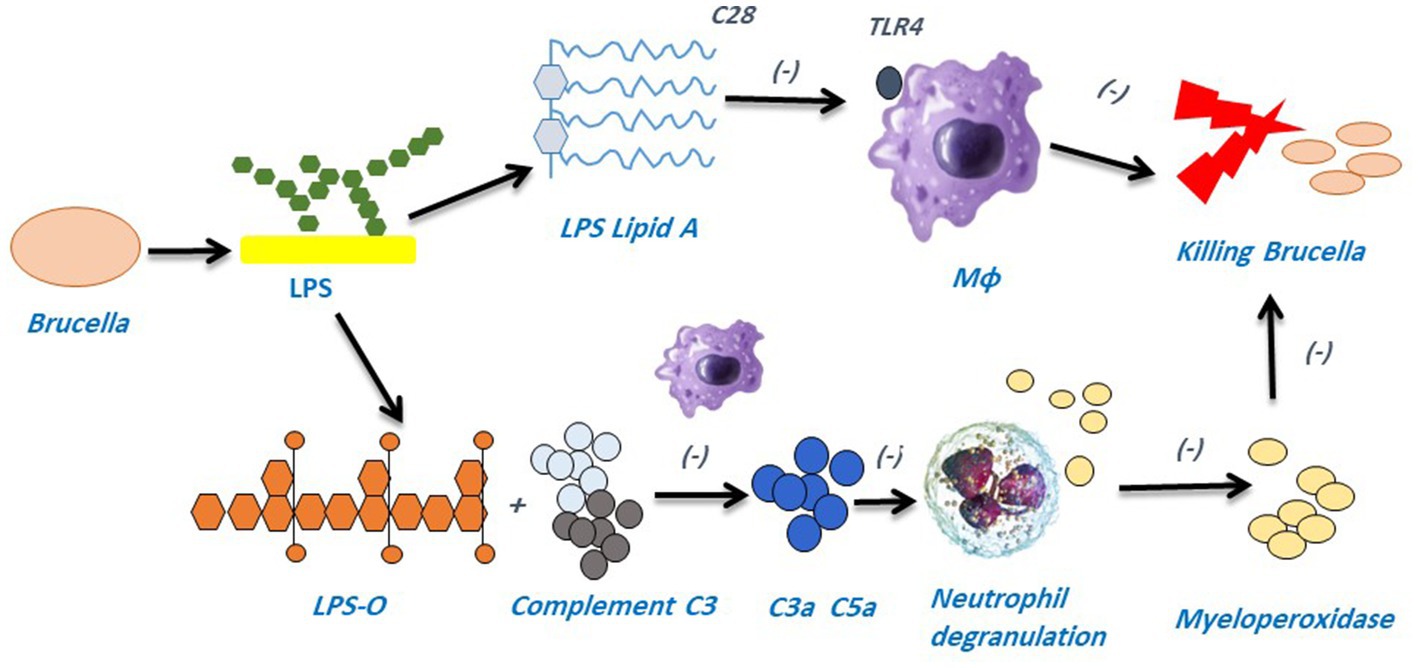
Figure 3. LPS is a pivotal component of Brucella’s pathogenicity and aids in the pathogen’s ability to evade the host immune system. TLR4 is not recognized by Brucella LPS’s acetyl side chain (C28), which prevents the host immune system from being monitored. The O-chain found in Brucella LPS can block complement C3 from producing C3a and C5a, which in turn can stop neutrophils from degranulation and releasing myeloperoxidase (MPO) and other lysosomal chemicals that could otherwise be collected by the host immune system.
4.2. Intracellular life of Brucella pathogens
Intracellular pathogens are characterized by many occult pathways that need further experimental work to overcome the growing threat of many zoonotic pathogens (116). In parallel, the growing concern regarding their resistance to different antimicrobials made further studying of their interaction with host cells increasingly important (117–119). Brucella has a multistage, complex intracellular replication process that affects the endocytic, secretory, and autophagic compartments through type IV secretion system (T4SS)-mediated delivery of bacterial effectors. These effectors enhance the conversion of the initial endosome-like Brucella-containing vacuole (eBCV) into a replication-permissive organelle derived from the host endoplasmic reticulum replicating BCV (rBCV), after that, to an autophagy-related vacuole (aBCV) that mediates bacterial egress (120).
T4SS regulates the inflammatory response and manipulates vesicle trafficking inside host cells, thereby playing crucial roles in the inhibition of the host immune response and intracellular survival during infection. In brief, after the entrance of Brucella into the host cell, it lives in acidified phagosomal compartments that are recognized as (eBCVs), and the pH can reach 4, which is essential for the survival of Brucella and the intracellular expression of the T4SS. The eBCVs avoid fusion with the terminally degraded lysosomes, thus ensuring not only the intracellular survival of bacteria but also triggering intracellular bacterial growth before rBCV is formed (121). The rBCVs maintain Brucella’s chronic intracellular persistence in the host. On the other hand, aBCVs are thought to be crucial for bacterial infection and cell-to-cell spread in the host and are created when the rBCVs engage with elements of the host cell’s autophagy process (121). Overall, Brucella can resist the phagocytic bactericidal effect; and it can also induce the host cells to create a microenvironment that is favorable for bacterial survival, reproduction, and replication. This ability allows Brucella to remain in the host cells for extended periods, which ultimately results in the development of chronic persistent infection (113) (Figure 4).
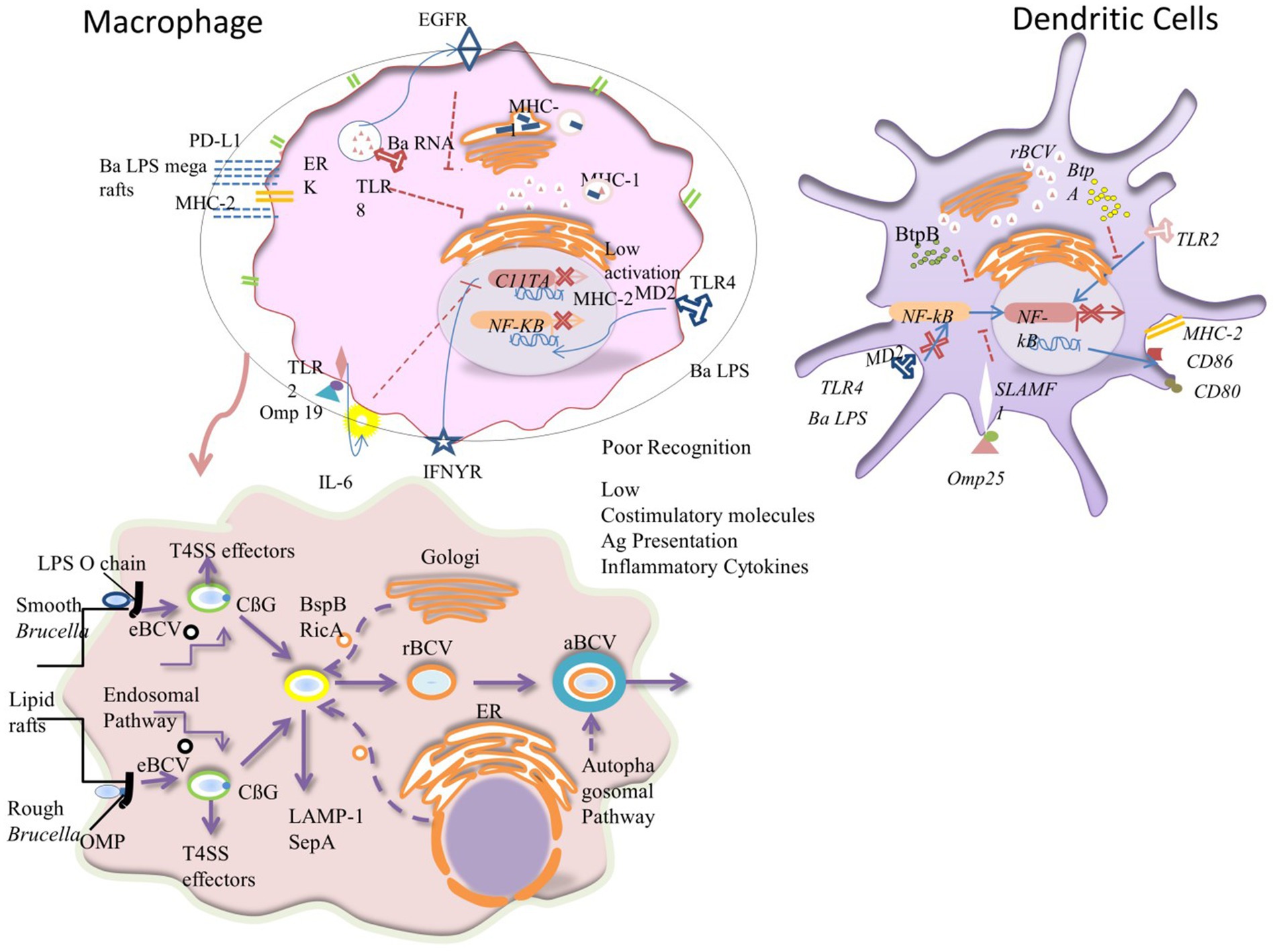
Figure 4. Mechanisms causing poor antigen presentation, intracellular trafficking, and penetration of Brucella inside macrophage: Brucella has developed a number of strategies to inhibit DCs’ and macrophages’ efficient antigen presentation, impairing the development of an adaptive immune system. (A) Brucella lipoprotein recognition by TLR2 results in IL-6-dependent suppression of the transcription factor CIITA, which reduces the transcription and expression of MHC-II that is triggered by IFN-γ. Additionally, B. abortus (Ba) LPS reaches the cell surface where it joins with MHC-II molecules to generate macrodomains that prevent peptides from being presented to CD4+ T cells. Since B. abortus RNA detection results in the retention of MHCI molecules within the Golgi apparatus via TLR8 and the EGFR pathway, this impairment also affects cytotoxic CD8+ T cells. Pro-inflammatory cytokine production and the expression of co-stimulatory molecules in DCs are decreased because of the Omp25-SLAMF1 interaction’s restriction of NF-kβ translocation to the nucleus. The maturation of DCs is regulated by the Brucella effectors BtpA and BtpB, which are translocated to the cytoplasm during infection and disrupt TLR2 and TLR4 signaling. Due to the Ba LPS’s unusual shape, particularly its core, it is poorly recognized by the TLR4-MD2 complex in both cell types, which prevents full activation, NF-kβ translocation, and inhibits DCs maturation and T-cell activation. Red represents nuclear phosphorylated active NF-β dimers. The T4SS effectors, the LPS O-chain, OMP22, OMP25d, and cyclic 1, 2-d-glucan (CβG) assist in the remodeling of the lipid-rich regions of the exterior vacuole that results in a fusion with lysosomes for bacterial replication. T4SS proteins in the cytosol of the host cell enable interaction with the endoplasmic reticulum that is converted into the replicative vacuole. The black and orange circles characterize membrane vesicle trafficking from the endolysosomal pathway, Golgi apparatus, and endoplasmic reticulum to the Brucella-containing vacuoles (BCVs). The alteration in the colors of the BCV membranes symbolizes their change in structure as they switch from eBCVs to rBCVs. The outer blue membrane of the aBCV shows the engulfment of the rBCV by the host cell autophagosomal pathway.
4.3. Acquired immunity
The other arm of host defense is acquired immunity. It consists of T lymphocytes, which are involved in cytokine production and cytotoxicity (cellular immunity), in addition to antibody-producing B lymphocytes (humoral immunity) (108). Primarily, the antigen-specific T cells secrete interferon-gamma (IFN-γ) as part of the Th1 immune response against Brucella. Then, the IFN-γ activates macrophage bactericidal machinery, enhances the production of antigen presenting cells (APCs), stimulates cytotoxic T-cells (CTL)-mediated cytotoxicity, and potentiates infected macrophage apoptosis (Figure 5). Finally, antibody-mediated opsonization (by IgG1, IgG2a, and IgG3) improves the phagocytic uptake of bacteria by lowering the initial level of Brucella infection (122). Studies showed that Brucella induces APCs to generate IL-2 and activates NK cells. These NK cells release TNF-α, IFN-γ, granulocyte-macrophage colony-stimulating factor (GM-CSF), and other cytokines that play a key role in Th1 and type 1 CD8+ T-cell (Tc1) responses (112). TNF-α can boost macrophage bactericidal ability. On the other side, IL-12 can activate the Th1 immune response and produce IFN- γ (123). IFN- γ secretion controls MHC-I and MHC-II expressions, which in turn, works for Brucella elimination through mediating Th1 immune response (111, 124). All in all, the decreased CD8+ T-lymphocyte activation, IL-12, and TNF-α lead to immunosuppression, and promote Brucella multiplication and infection persistence (125).
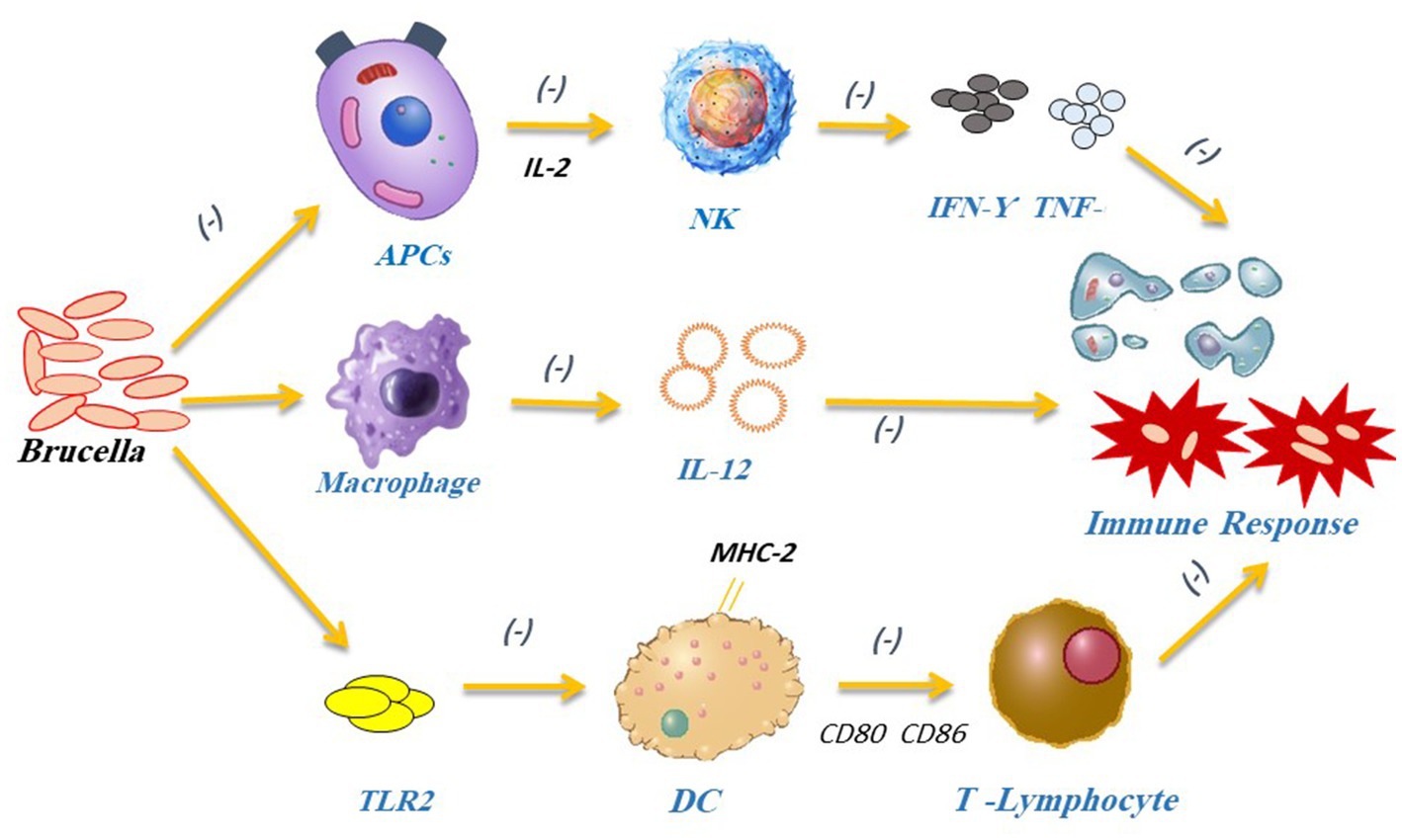
Figure 5. Brucella can stop APCs from secreting IL-2, which subsequently prevents the production of inflammatory substances such as IFN-γ and TNF-α by NK cells. Brucella can block IFN-γ-mediated phagocytosis to avoid being destroyed by the immune system. Brucella interferes with the Th1 immune response causing macrophages to lower IL-12 release and preventing DCs from activating T lymphocytes. It also impacts DCs maturation by blocking the TLR2 receptor pathway.
Recent studies clarified the roles of Brucella TIR protein 1 (Btp1), LPS, and PrpA as being significant immunomodulatory molecules with the capability to modify host immune mechanisms. Moreover, they can inhibit the secretion of IFN-γ and increase the secretion of IL-10 affecting Th1 immune response (126). Btp1 shows a sequence resembling the Toll/IL-1 receptors (TIRs) domain family. Different studies investigated the role of Btp1 in DCs maturation due to the significance of the TIR domain in TLR signaling. Btp1 inhibits both the production of proinflammatory cytokines and DCs maturation which leads to inhibition of TLR2 and TLR4 signaling. Brucella lumazine synthase (BLS) also induces negative effects by blocking the TLR4-myeloid differentiation protein-2 (MD2) complex and inducing CD8+ T-lymphocyte toxicity. This inhibition of CD8+ T-cell killing of Brucella target cells represents an adaptive immune evasion strategy (127). In addition, Btp1 binds to adapter TIRAP (TIR domain-containing adaptor protein) at the cell membrane and blocks NF-κβ activation (128). PrpA also plays a significant role in the early stages of Brucella infection by regulating IFN-γ, TNF-α, IL-10, and transforming growth factor-1 (TGF-1) pathways (129) (Figure 6).
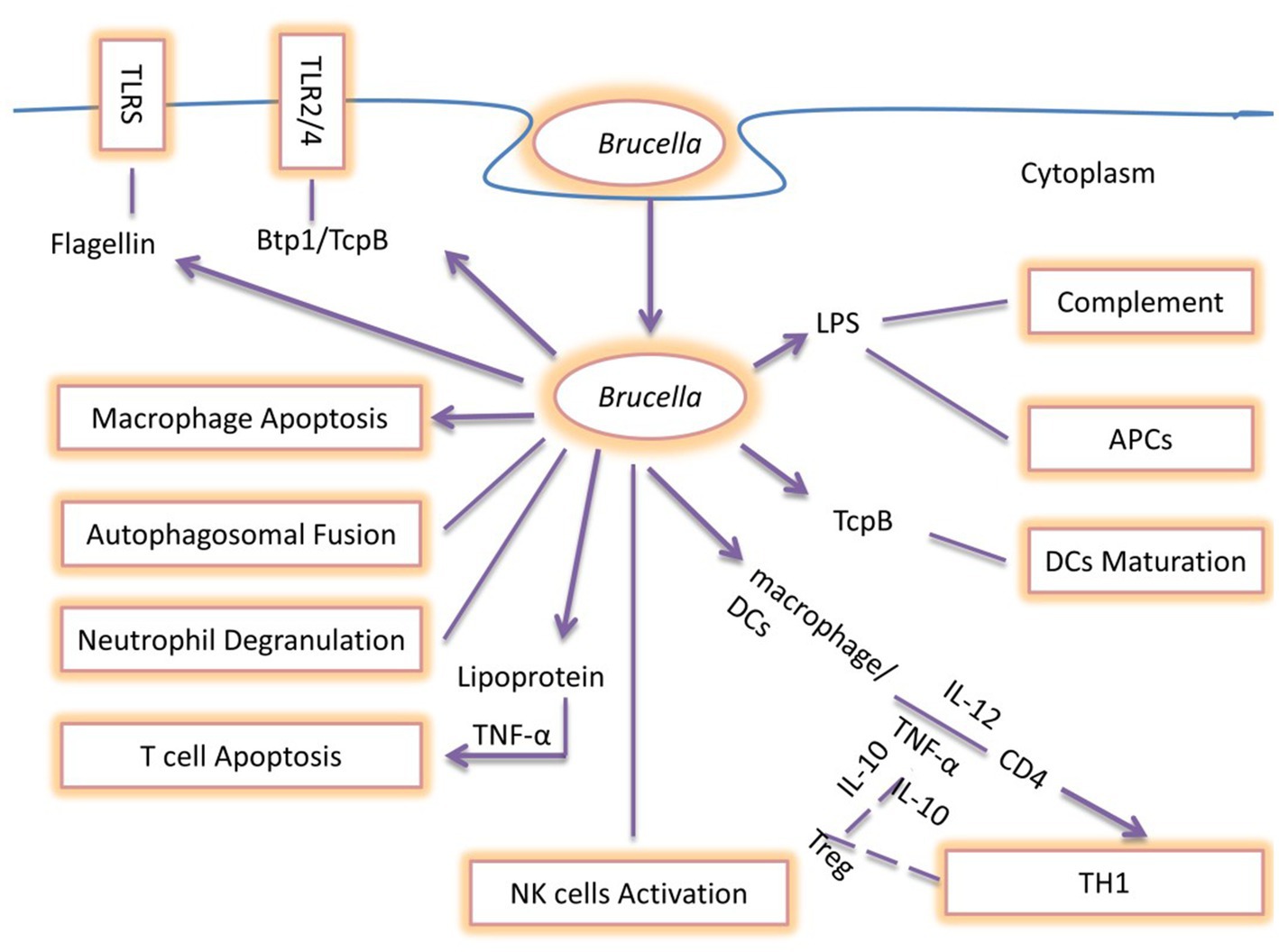
Figure 6. Summary of immune escaping Strategies of Brucella: During the early stages of infection, TNF-α and IL-12 are secreted by DCs and macrophage cells as a result of the detection of Brucella, which promotes intracellular survival and replication. Flagellin suppresses the TLR5 receptor, whereas Brucella produces the Btp1/TcpB protein, which blocks the TLR2/4 signaling pathway. The Brucella LPS O-antigen binds to C3, preventing the complement cascade from being activated. Brucella blocks neutrophil degranulation and NK cell activation, interfering with the host’s innate immune response. These bacteria produce Btp1/TcpB to prevent DCs maturation. Brucella LPS prevents antigen presentation and interferes with the host’s innate and adaptive defense, making chronic infection more likely. TNF-α secretion is necessary for Brucella lipoprotein to promote T-cell apoptosis, which in turn directly suppresses the T-cell response. Additionally, Brucella prevents macrophage apoptosis and autophagolysosomal fusion, which represents the primary evading mechanisms of Brucella pathogens. The end effect of interfering with these mechanisms is the development of human brucellosis and its clinical symptoms in natural hosts.
4.3.1. Vaccination
4.3.1.1. Requirement of brucellosis vaccines
Although Brucella vaccines have been employed as a control measure, they are not 100% successful in preventing the disease (130). Average exposure to Brucella protects only 65% of vaccinated animals (131). The requirements for an optimal Brucella vaccination have remained unchanged since they were first established many years ago. Such a vaccine should be safe and effective, does not enhance antibodies interfering with serodiagnosis, cannot be transmitted to humans or other animals (including no contamination of milk, edible organs, dairy products, or meat), be stable in vitro and in vivo, be easily cultivable for large-scale production, and be supplemented with markers to be differentiated from the field isolates (29).
4.3.1.2. Classic attenuated Brucella vaccines
Brucella S19, RB51, 45/20, and Rev1 strains are commonly used for designing vaccines to protect livestock against Brucella infections and subsequent abortions (132, 133) (Table 3). Natural attenuation of the erythritol catabolic genes in B. abortus S19 resulted in the loss of a 720-bp area compared to the primary strain which resulted in a less virulent strain (137). Vaccination with this attenuated strain stimulates a high level of immunity and protects animals against Brucella infection for a long period, which can extend to the entire productive life of the animal (138). On the other hand, the S19 vaccine has several drawbacks, including interfering with serodiagnostic brucellosis tests, inducing abortions in some vaccinated and pregnant animals, reducing milk production, and being pathogenic in humans (139). It is administered to female calves between the ages of 3 and 6 months in a single subcutaneous dosage of (2.5–12 × 1010) colony-forming units (CFUs) (140). Adult cattle can be injected with a lower dosage of organisms subcutaneously, but some animals will acquire persisting antibody titers and may abort and excrete the vaccine strain in their milk. When used for calves aged 3–5 months, the conjunctival technique is completely safe and minimizes the risk of serological interference. It can also be given to cattle of any age as one or two doses of 5 × 109 CFU via the conjunctival route, which protects without a prolonged antibody response and minimizes the risk of abortion and microbial shedding in milk when vaccinating adult cattle (141). Subsequently, Saidu et al. confirmed that the intraocular route is still the safest route for vaccinating adult cattle than the subcutaneous route (134).
B. abortus strain RB51, a rifampicin-resistant mutant, was isolated as a single rough colony from a virulent smooth strain of B. abortus 2,308. Serial subculturing of B. abortus 2,308 resulted in the deletion of the wbo A gene, which encodes a glycosyltransferase required for O-side chain production (29, 142). Unlike the strain 19 vaccine, the strain RB51 vaccine does not affect Brucella serodiagnosis results (143). Therefore, it has replaced the B. abortus strain 19 vaccine, which is well-known as the calf hood vaccine in many countries. The term calfhood vaccine means vaccination of heifers between 4 and 10 months of age with Brucella abortus strain 19, and the best age of vaccination is at 5 months (144). RB51 vaccine can infect humans, and one of its primary drawbacks is the resistance to rifampicin antibiotic, which is used for human brucellosis therapy (145). B. melitensis Rev1 vaccine could be used to protect sheep and goats against brucellosis (146). However, it has a negative effect because it might cause abortions in pregnant animals after vaccination (24). Aside from being virulent for humans, lactating female animals can shed the vaccinal strain through milk, which can infect other animals and interfere with serological diagnosis (147).
B. abortus 45/20 strain was created by passing the B. abortus 45/0 virulent strain 20 times in guinea pigs, although multiple studies have shown that it can revert to smooth virulent forms when used as a live vaccine. So far, the 45/20 vaccine is combined with an oil-based adjuvant, needing repeat vaccination (143). A systematic review and meta-analysis were performed to recalculate the vaccine efficacy of B. abortus S19 and RB51 vaccine strains suggesting that a dose of 109 CFU for S19 and 1010 CFU for RB51 are the most effective for the prevention of abortion and infection caused by B. abortus (136).
The DIVA vaccines (Differentiating Infected from Vaccinated Animals) are defined as the vaccines that distinguish infected and vaccinated animals. S19 and Rev1 vaccine problems can be solved using DIVA vaccines. There are two main strategies to produce these vaccines. First, the removal of the diagnostic antigens found in field strains from current vaccines. In other words, creating Brucella vaccine candidates free from O-chain and NH-polysaccharides or synthesis of immunogenic proteins using Brucella mutants that could be used as neutral antigenic markers. Second, the addition of the foreign (xenogenic) antigens to the classical live-attenuated Brucella vaccine strains, thereby enabling the development of diagnostic tests that can recognize vaccinated animals in infected environments (141). Meanwhile, DIVA vaccines that can replace S19 and Rev1 vaccines should protect at least as good as the primary vaccines (29). Many studies have suggested several vaccine candidates for DIVA vaccines such as VjbR and BtpA proteins contributed to the virulence of the Y3 and M5 Brucella strains. Zur protein, ABC transporters, and thiamine metabolism-associated proteins may play crucial roles in Brucella survival and pathogenesis (148). In parallel, Uslu and Erganis revealed that B. melitensis Rev.1 ΔOmp19 can act as a DIVA marker vaccine using an ELISA test for the detection of the Omp19 protein (149).
4.3.1.3. Genomics and proteomics approaches for improved Brucella vaccines
4.3.1.3.1. Brucella subunit vaccines
Several Brucella fragments, such as recombinant peptides, proteins, DNA, LPS, and outer membrane proteins (OMPs) are now being tested as subunit vaccines against B. abortus (147). They have been proved to be a promising field for study and development because of their pioneer advantages over traditional live-attenuated vaccines, including high safety with no residual virulence and the ability to be used in people and pregnant animals. However, although they provide appealing alternatives to traditional live-attenuated vaccines, they face significant challenges. The low protective efficacy and the requirement for adjuvant and booster doses are among them. Consequently, subunit vaccines can be improved by using potent T-cell antigens that produce a Th1 immune response as dominant immunity against brucellosis (150, 151) (Table 4).
OMP16, OMP19, liposomes protein L7/L12, OMP25, p39 (a potential periplasmic binding protein), and AsnC are important Brucella subunit proteins (152–154). In general, these antigens stimulate Th1 immunity and provide protection similar to that provided by the commercial S19 live vaccine (153, 158). Other Brucella subunit vaccines such as dihydrolipoamide succinyltransferase (rE2o) and cysteine synthase A (rCysK) are capable of inducing Th2 immunity with a relatively low protection level (155, 156). In addition, compared to the S19 vaccine, the SurA and DnaK cytosolic proteins elicit lower levels of protection in a mouse model (157). Additionally, B. abortus rAdk and rSecB have been suggested as promising subunit vaccine candidates. BP26 and BLS proteins are also among the top candidates for serological diagnosis of brucellosis (154, 163).
Vaccinating mice with a recombinant protein cocktail (rOMP19 + rp39) resulted in Th1-mediated isotype antibodies and cellular immunity, which protected animals against the B. abortus 544 strain (158). Afterward, the B. abortus chimeric subunit protein with OMP19 and p39 domains was used as a booster dose-induced Th1-type immune response (159). Intriguingly, using Brucella OMP25c recombinant protein combined with Freund’s adjuvant produced both Th1 and Th2 immune responses in mice, with protection levels similar to the S19 vaccine (160). More interestingly, using a mixture of various recombinant B. abortus proteins, including AspC, Dps, Ndk, and lnpB as subunit vaccines, induced high levels of IgG2a titer and exhibited equal protective efficacy to the RB51 vaccine strain (161). In comparison with other recombinant subunit proteins, 3E-IL2 inoculation could be a superior candidate for further study into the manufacture of Brucella recombinant vaccines (162).
4.3.1.3.2. DNA vaccines
Another type of subunit vaccine that induces strong humoral and cellular immune responses with repeated doses is the DNA-based Brucella vaccine (164). Using laboratory and clinical testing, they were proven to be safe and effective. To examine their immunogenicity, BALB/c mice were immunized with multivalent DNA vaccines. These vaccines significantly induced the humoral immune response (IgM, IgG, and IgG2a) and cell-mediated immunity (high IFN-γ and increased splenic lymphoproliferative response) (165). The genomic island 3 (GI-3) region of B. abortus encodes multiple open reading frames (ORFs) that express critical antigens for microbial intracellular survival and pathogenicity. Therefore, the Brucella DNA vaccine based on the GI-3 region could be a good candidate for protection against B. abortus infection (166). More specifically, DNA vaccines expressing BAB1-0263 or BAB1-0278 genes from GI-3 ORFs induced substantial levels of IFN-γ production, which in turn elicited strong humoral and cellular immunity. In addition, when mice were challenged with the B. abortus 2,308 strain, a DNA vaccine expressing BAB1-0278 provided good protection (167).
In comparison with B. abortus RB51 vaccination, DNA vaccine encoding Cu-Zn superoxide dismutase (SOD)-IL-2 fusion protein stimulated IgG2a and TNF-α in mice, resulting in excellent protection against B. abortus 2,308 strain (165). The combination of SOD with L7/L12 and BCSP31 generated a robust cytotoxic CD8+ T cell and specific IgG, resulting in a higher level of protection compared to B. abortus S19 (168). In another investigation, Brucella genes (SOD, BCSP31, and L7/L12) were joined with several genes from Mycobacterium bovis or Mycobacterium TB, resulting in a dual efficient DNA vaccine for both infections (Brucella and Mycobacteria) (169, 170). Another study showed that a divalent DNA vaccine expressing both B. abortus L7/L12 and OMP16 genes boosted robust cellular and humoral immunity in mice by inducing IFN-γ and IgG2a production. In addition, compared to the univalent OMP16 or L7/L12 DNA vaccine, this divalent DNA vaccine induced higher levels of protection, even though the protective efficacy of the divalent OMP16 and L7/L12 was lower than that of the traditional RB51 vaccine (171).
The bacterial ghost (BG) vaccine is a novel strategy with great promise. In China, the B. abortus A19 strain is widely used as a vaccine. However, persistent pathogenicity in animals and humans is a common disadvantage (172). In China, He et al. developed the Brucella abortus A19 bacterial ghost (A19BG) vaccine; it was inactivated using a two-step process that included biological lysis and hydrogen peroxide treatment, which, in turn, completely inactivated the viable bacterial cells found even at high concentration of 1010 CFU/mL. Furthermore, no adverse effects have been noticed in guinea pigs. The levels of antibodies, interleukin-4, interferon-γ, and CD4+ T cells in guinea pigs inoculated with the A19BG vaccine were comparable to those inoculated with the conventional A19 vaccine. In guinea pigs and cattle, vaccination with both A19BG and A19 vaccines provided equal protection against B. melitensis M28 (173).
4.3.1.3.3. Genetically engineered live-attenuated vaccines
The identification of genes linked to virulence or organism survival can help in creating safe and efficient novel vaccines. In comparison with traditional live-attenuated vaccines, currently developed live-attenuated vaccines can induce significant levels of protection (137). The purine biosynthesis pathway genes, T4SS, virB genes, lipid A fatty acid transporting gene, ferrochelatase hem H mutant, phosphoglycerate kinase encoding gene, and the LPS biosynthesis pathway gene are considered under vaccinal development depending on multiple deletions in B. abortus genome that lead to significant attenuation (174, 175). These mutations provide supreme protection compared to traditional live-attenuated vaccines. In mouse and human cell experiments, deletion of B. abortus 2,308 norD and high-affinity zinc uptake system (znuA) genes causes adequate attenuation. Furthermore, this live recombinant strain effectively enhances T cells and produces potent immunity against virulent challenges, compared to traditional RB51 vaccinated groups (176).
By deleting the phosphoglucomutase (pgm) gene of B. abortus 2,308, Ugalde et al. created a recombinant strain with no serodiagnostic interference and protective Th1 immune responses compared to the S19 strain (177). Additionally, GntR, a transcriptional regulator of many virulent antigens of B. abortus 2,308, has been deleted resulting in an attenuated mutant with high protection levels in mice against the parental B. abortus 2,308 challenge (178). In parallel, the deletion of NodV and NodW genes in B. abortus 2,308 resulted in an attenuated live vaccine with reduced survival in cell lines and mouse models, and it did not affect serological diagnosis (179). In 2010, Yang et al. successfully deleted znuA and purE genes of B. abortus resulting in a live-attenuated mutant that required two doses to generate an appropriate immune response in mice (180).
The deletion of the cgs gene in B. abortus S19 increased the attenuation of the S19 strain without altering its protective efficacy against B. abortus 2,308 (181). In addition, the deletion of the vjbR gene, which is responsible for intracellular Brucella persistence, resulted in a recombinant mutant with high levels of protection and reduced inflammation (182). B. abortus targeted mutants with deletions in the membrane fusogenic protein (Mfp) or OMP19 genes decrease Brucella persistence in animal studies. However, challenge tests have revealed that traditional attenuated vaccines such as S19 and RB51 strains provide similar levels of protection (183). The deletion of the wbkC gene of B. abortus (ΔwbkC), which translated to formyltransferase enzyme, showed a protection level similar to B. abortus rough strain RB51 avoiding the rifampicin resistance which is the main disadvantage of RB51. However, B. abortus ΔwbkC did not produce the same level of protectin when compared to B. abortus S19 and avoided interfering with the serological diagnosis which is also the main disadvantage of the S19 vaccine strain (184). The Glycosyltransferase Wad C gene is involved in the production of the core oligosaccharide region of B. abortus LPS and is essential for efficient innate immune recognition (185). The deletion of this gene produced a better immune response compared to those provided by the S19 strain (186).
Single and double gene deletions of the virulent B. abortus RB51 cydD and cydC genes have created significantly attenuated mutants (RB51ΔcydC, RB51ΔcydD, RB51ΔpurD, RB51ΔcydCΔcydD, and RB51ΔcydCΔpurD). Vaccination with a single dose of these mutants induced lower protective immunity in mice compared to the RB51 vaccine. However, a booster dose provided significant levels of protection in mice against the challenge of the virulent B. abortus strain 2,308 and B. canis strain 26 (145, 187). Additionally, in South Korea, the double deletion in a field isolate of B. abortus biovar 1 (BA15) produced similar protective effects without the need for a subsequent booster shot (188). In B. abortus biovar 1 strain IVKB9007, deletion of the ATP/GDP-binding protein motif A (p-loop) and the ATP-binding/permease protein resulted in attenuated mutants that were unable to replicate intracellularly in cell line models. These mutants induced significant levels of protection against the pathogenic B. abortus strain 544 (189). Another study compared the whole-genome sequences of B. abortus S19 and A19 vaccine strains. Both strains were proved to have a significant degree of genetic similarity. The differences in genomic structure revealed that S19 had a 697 bp deletion and loss of function on both eryC and eryD genes compared to the A19 strain (190). Moreover, the Wzm/Wzt system has an important role in exporting the O-polysaccharide (O-PS) as the main virulence factor of Brucella. Single and double deletion mutants of wzm/wzt have been designed using the attenuated strain B. melitensis Rev1. Results revealed that Rev1Δwzm mutant is an immunogenic and effective vaccine candidate against B. melitensis and B. ovis in mice even though it had low persistence. So, it has minimal serological interference in sheep making Rev1Δwzm a highly promising vaccine candidate. Rev1Δwzm is a highly promising vaccine candidate in sheep because it has minimal serological interference and is safe to be used in pregnant ewes (191) (Table 5).
4.3.1.3.4. Vector-based B. abortus vaccines
Several Brucella vaccines based on bacterial or viral vectors have recently been designed, and they provide an efficient method of delivering a range of heterologous or homologous antigens (192) (Table 6). The optimum method for stimulating the target host immune system against Brucella pathogens can be achieved through induced cell-mediated immunity because of the intracellular tropism of this microorganism. These live vaccines produce many copies of the Brucella antigens because they reproduce inside the host cells. Despite all these benefits being highly appealing, there is no current efficient vector-based Brucella vaccine that provides the best protection.
Many studies demonstrated that attenuated Salmonella strains can express various Brucella antigens, such as BLS, PrpA, Omp19, and Cu-Zn SOD, which were employed as vaccine vectors. These formulations with pure Brucella LPS were inoculated intraperitoneally in specific pathogen-free (SPF) mice and produced an efficient delivery with a significant (p ≤ 0.05) reduction in splenic wild-type B. abortus 544 colonization compared to the control mice. More importantly, this vaccination system can provide a platform for the development of cross-protecting vaccines able to prevent multi-species brucellosis (199).
As vector-based vaccines, influenza viruses expressing Brucella ribosomal proteins L7/L12 and OMP16 have been produced (200). Cross-protection against B. melitensis can be obtained using the B. abortus vaccine. First, pregnant heifers received an influenza viral vector B. abortus vaccine and have been protected from abortions. After challenging with B. melitensis16 M, a remarkable cross-protection (90–100%) was obtained for the heifers, their calves, and fetuses. On the other hand, when compared to the B. abortus S19 vaccine, the influenza viral vector-B. abortus vaccine provided equal protection (197). In terms of safety, this recombinant vector vaccine showed superior safety characters. Notably, this vaccine prime-booster immunization produced humoral and cellular immunity with long-term protection against B. abortus infection, particularly in pregnant heifers (201, 202). In addition, adding OMP19 and SOD proteins to the vaccine formulation, as well as using montanide gel as an adjuvant, resulted in excellent protection in sheep and goats when challenged with B. melitensis (203).
An adenovirus vector-based vaccine was developed for protection against brucellosis and expressed the p39 and BLS proteins of B. abortus. This vaccine successfully induced strong humoral and cellular immune responses in a mouse model (198).
4.3.1.3.5. Proteomics approaches
Proteomics, the key post-genomic technique for analyzing the effects of regulatory mechanisms on the protein composition of microorganisms, has the potential to deal with issues of One-Health concern. It is a useful tool for researching gene expression, microbial physiology, and host–pathogen interactions. Scientists have been using proteomics technology to decipher numerous mysterious areas of Brucella research since the start of the 21st century. Proteomics techniques, including sodium dodecyl sulfate-polyacrylamide gel electrophoresis (SDS-PAGE), two-dimensional gel electrophoresis (2DE), matrix-assisted laser desorption ionization–time of flight mass spectrometry (MALDI-TOF MS), and label-free analysis, are advanced approaches for accurate analysis of pathogenic protein spots (204).
A study was performed to identify the phenotypic and pathophysiological variations among Brucella species (B. melitensis strain 16 M and B. ovis REO198) and to correlate these variations with virulence elements. SDS-PAGE was used to separate protein extracts from the two Brucella species. The nano-scale liquid chromatographic tandem mass spectrometry (nLC–MS/MS) was used to investigate the areas that were qualitatively and quantitatively different. A total of 274 and 606 proteins were identified for B. melitensis and B. ovis, respectively. Bioinformatics study was performed to examine their structural and functional characteristics. Outer membrane immunogenic protein N8LTS7 and outer membrane immunogenic protein Q45321 were identified as promising candidates for improving Brucella diagnostic procedures and vaccinations for B. ovis and B. melitensis, respectively (205). Moreover, outer membrane vesicles (OMVs) have been suggested as a paradigm for the development of cellular vaccines because they contain immunogenic proteins. To characterize the pan-proteome of these vesicles, OMVs of B. suis, B. ovis, B. canis, and B. neotomae were isolated and analyzed using SDS-PAGE, transmission electron microscopy, and liquid chromatography-mass spectrometry (LC–MS). Western blot was also used to identify antigenic proteins. Several homologous immunogenic proteins, including Omp25, Omp16, Omp2a, Omp31, SodC, and BhuA, were detected during in silico investigations. Anti-Brucella sera were able to identify the proteins in the vesicles from several Brucella species (206).
A comparative study between the vaccinal strain (B. melitensis Rev.1) and the field strain (B. melitensis 16 M) enabled us to get the detailed Brucella protein repertoires using label-free shotgun proteomics investigation which would help in discriminating the vaccinated from infected animals (207). MALDI-TOF MS, a quick, affordable, and trustworthy way for routinely identifying Brucellae, is an advanced technique used to reduce the time needed to identify pathogens and may soon replace the current conventional and molecular procedures. Hamidi et al. performed the MALDI-TOF MS technique for fast and trusty differentiation of B. abortus and B. melitensis, depending on proteomic mass patterns. They reported significant protein mass signals, which were mapped to ribosomal proteins and structural proteins, such as integration host factor subunit alpha, GNAT family N-acetyltransferase, HU family DNA-binding protein, cold-shock proteins, and ATP synthase subunit C. They used these specific biomarker peaks that have been recognized for virulent and vaccine strains as differentiating tools (208). Furthermore, a unique MALDI-TOF MS reference library was created by Christoforidou et al., and the effectiveness of its performance on species-level identification was assessed using 75 Brucella spp. isolates. Analysis of mass peak profiles allowed for 100% accurate genus and species identification of Brucella. Despite the significant intrageneric similarity, the MALDI-TOF MS database was able to classify 47 out of 62 B. melitensis bv. 3 isolates at the biovar level (75.81%) (209).
Proteins and genes are differently expressed in B. abortus cultured under biofilm and planktonic conditions. 2DE and MALDI-TOF MS were used to separate and identify these proteins, respectively. 2DE revealed 20 protein spots that were differentially expressed between biofilms and planktonic cells, which matched 18 distinct proteins, including enolase and the elongation factor Tu. According to an RT-qPCR study, all 18 genes were downregulated in biofilms. Fourteen function and pathway-associated genes were reported using high-throughput sequencing and bioinformatics analysis. The 14 genes were upregulated in biofilm conditions and they may play important roles in bacterial defense, colonization, invasion, and virulence (210). A comparative in silico study comparing the proteomes of B. melitensis Rev.1 and 16 M was performed to identify biomarkers proteins, which distinguish the vaccine and the field strain. MALDI-TOF MS has identified two unique biomarkers most similar to ribosomal proteins (L24 and S12) (211). For accurate identification of Brucella species in animals and humans, Elbehiry et al. have recognized the proteomics of Brucella species with the help of the MALDI-Biotyper (MBT) system and then confirmed the results by microfluidic electrophoresis. This strategy was proved to be effective for the diagnosis of Brucella pathogens in animals and humans. Eleven positive B. melitensis isolates and 14 positive B. abortus isolates were reported (212).
Furthermore, Mahmud et al. studied the entire proteome of B. abortus strain 2,308 by using subtractive genomic analysis, and the results showed that only three membrane proteins (acriflavine resistance protein B, ABC transporter permease, and penicillin-binding protein 2) were observed to be potential novel vaccine candidates in cattle, while ABC transporter permease was predicted as a novel drug target for humans (213). B. abortus and B. melitensis outbreak strains from cows and sheep, respectively, were subjected to label-free quantitative proteomic analysis, which identified 402 differentially expressed proteins. Among these, 63 and 103 proteins were only found in the whole-cell extracts of B. melitensis and B. abortus field strains, respectively (214). Following numerous environmental pressures and adaptations, 1,221 differently expressed proteins in B. abortus were reported using label-free relative quantitative proteomics and mass spectrometry (215).
To investigate protein biomarkers for Brucella in human and livestock serum, a targeted multiple reactions monitoring-mass spectrometry (MRM-MS) method was created. In total, 30 synthetic peptides that match 10 immunodominant B. abortus proteins were created using bioinformatics analysis; and 117 serum samples from people and animals, classified as clinically confirmed (45), suspected (62), and control (10), were used to optimize the MRM-MS technique for the precise detection of these peptides. Transitions for four peptides were found in various clinically confirmed and suspected human and cattle serum samples using high-throughput MRM tests. Of them, peptide NAIYDVVTR with 100% specificity was repeatedly found in the clinically proved serum samples of both people and livestock, and it corresponds to the B. abortus protein (BruAb2_0537) (216).
With vaccine antigen prediction based on bioinformatics analysis of pathogen genomes, reverse vaccinology (RV) has been demonstrated to be a highly successful method (217). Therefore, based on the Brucella protein-coding genome, RV has been utilized in several research projects to evaluate prospective vaccine candidates (218). Zai et al. carried out a wide-scale study to screen putative antigens against 213 pathogenic strains of Brucella spp. with global geographic distribution using core proteome analysis and a compositive RV technique. Six biological characteristics (subcellular localization, antigen similarity, antigenicity, mature epitope density, pathogenicity, and adhesion probability) were used to rank candidate proteins. A total of 32 potential antigens were chosen for the RV analysis. Omp19 was used as a positive control, and T4SS protein VirB8 and type I secretion system (T1SS) protein HlyD were chosen for the evaluation of immunogenicity and preliminary protection in a mouse model (219). In addition, Vishnu et al. revealed eight proteins as potential vaccine candidates after thoroughly screening the exoproteome and secretome of B. melitensis 16 M. These proteins include the LPS-assembly protein LptD, heme transporter BhuA, hemagglutinin, flagellin FliC, 7-alpha-hydroxysteroid dehydrogenase, and immunoglobulin-binding protein EIBE. The functions of BhuA and hemagglutinin are crucial for the establishment of infection (220).
Moreover, Aslam et al. obtained the core genes set from 56 full genome sequences of B. melitensis. To find prospective proteins as drug targets and multi-epitope vaccine constructions from core genes, strict bioinformatics architecture of comparative genomics and RV techniques were used. Based on their function and specific metabolic pathways, lack of homology to human and human gut microbiome proteins, and potential for acting as drug targets, the 23 proteins were predicted as novel drug targets (221). Furthermore, apolipoprotein N-acyltransferase (Int) was found to be the probable target of the most abundant short RNA released during early infection with bone marrow-derived macrophages (BMDMs) in B. abortus. Int expression was shown to be reduced during BMDM infection, and immunoinformatic analysis of the protein sequence allowed the reasonable selection of a potential immunogenic epitope that was investigated as a vaccine candidate (222).
Moreover, a multi-epitope vaccine (MEV) was designed using the RV technique of Omp25, Omp10, Omp31, and BtpB for creating MEV. In addition, 9 linear B-cell epitopes, 2 conformational B-cell epitopes, 13 cytotoxic T-lymphocyte (CTL) epitopes, and 17 helper T-lymphocyte (HTL) epitopes were predicted and proposed for further research. To increase immunogenicity, the vaccine peptide’s N-terminal was supplemented with the proper adjuvants. A final MEV with 806 amino acids was created using linkers and adjuvants. The outcomes of the trials on animals showed that the MEV had a significant immunogenicity and could be used as a Brucella vaccine (223). Small extracellular vesicles known as exosomes are released by cells and serve as a means of intercellular communication. Yi et al. investigated the extracellular interferon-inducible transmembrane protein 3 (IFITM3) role in the immunological response to Brucella infection. For the first time, they demonstrated that B. melitensis strain M5 can induce macrophages to release a significant amount of exosomes. The most significant finding was that the exosomes from Brucella M5-infected cells effectively reduced Brucella’s intracellular survival. Additionally, animals developed Brucella antibodies after immunization with exosomes containing IFITM3, reducing spleen CFU and tissue damage. Overall, this provides novel ideas for the progress of candidate vaccines for Brucella (224).
4.4. Basic techniques for Brucella diagnosis
Classical serological tests, such as RBPT and tube agglutination, are simple, rapid, and inexpensive. On the other hand, they produce non-specific reactions with other Gram-negative bacteria, such as E. coli O157, Vibrio cholera, Francisella tularensis, and Yersinia enterocolitica, which have antigenic matches to Brucella. To avoid these reactions, ELISA tests, mainly iELISA, have been used as a confirmatory test for brucellosis detection. ELISA tests are characterized by the usage of different antigens, enzyme conjugates, and substrates (109). CFT is a very specific test that can detect IgM and IgG1 antibodies. However, antibodies of the IgG2 type impede complement fixation resulting in false negative results (67). A recent comparative serological assay study for brucellosis diagnosis has used 2,317 sera samples [sheep (n = 552), goats (n = 1,345), and cattle (n = 420)]. The results indicated that 189 (8.2%), 191 (8.2%), and 48 (2.1%) tested positive using RBPT, iELISA, and CFT, respectively (225). Nucleic acid tests combine the ability to identify the pathogen with the rapidity of molecular-based assays to provide great performance. In this concern, Brucella spp. has been detected using multiplexed PCR and loop-mediated isothermal amplification (LAMP). LAMP is a method of nucleic acid amplification that is significantly rapid with high sensitivity and specificity. In addition, it does not require expensive reagents or instruments, and so it aids in cost reduction (226).
5. Future perspectives for controlling bovine brucellosis
5.1. Nanotechnology and recent trends for the diagnosis of bovine brucellosis
The development of novel tools for Brucella diagnosis is urgently needed for eliminating rampant Brucella pathogens in many parts of the world. Meanwhile, the existing methods for brucellosis diagnosis are time-consuming and expensive as they require a weary experimental procedure and sophisticated experimental devices. To overcome these shortcomings, it is truly necessary to establish real-time, on-site, sensitive, and rapid detection methods. For bovine brucellosis, Yang and co-workers investigated a simple, visible, specific, and reliable label-based polymer nanoparticles lateral flow immunoassay (LFIA) biosensor that can reduce the need for advanced equipment and simplify the detection technique. B. abortus-LAMP associated with nanoparticle-based LFIA targeting the BruAb2_0168 gene was established and performed successfully, and the technique demonstrated excellent sensitivity and specificity for the detection of many Brucella strains, including reference strains, vaccine strains, and field isolates (227). For on-site diagnosis of human brucellosis, LFIA was designed for the first time as a signal probe depending on blue silica nanoparticles (SiNPs) for quick detection with good sensitivity and specificity (228). Li et al. proved that the Brucella Multiple Cross Displacement Amplification-Lateral Flow Biosensor (MCDA-LFB) is a fast, easy, reliable, and sensitive method for detecting all Brucella spp. strains, and it can be utilized as a possible Brucella strain screening tool in many laboratories (229).
Nanotechnology has transformed the field of infectious disease diagnosis and the development of pharmaceutics (230, 231). Their importance in biological applications stems from their reduced size and distinct physicochemical features, which enabled regulated drug release, targeted drug delivery, and in vivo immunomodulation. In terms of sensitivity, specificity, time, and cost, nanotechnology has been proved to be a more efficient diagnostic tool. Metal nanoparticles, polymeric nanoparticles, nanoemulsions, liposomes, and nanocrystals are among the nanomaterials employed in veterinary diagnostics and therapies (232). Hybridization assays based on metal nanoparticles are simple to set up and can be read visually via a color shift caused by plasmonic interactions between the probes, so they are interesting approaches for diagnostic purposes. In particular, gold has been preferred in the application of these hybridization assays for the molecular detection of Brucella pathogens. Usually, with the addition of simple reagents such as salts, gold causes a color shift from red to purple when aggregated (233). Pal et al. have demonstrated the visual detection of Brucella in bovine biological samples using DNA-activated gold nanoparticles. They created a thiol-modified probe that was specific to a certain gene that codes for Brucella outer membrane protein. More importantly, they proved that the gold nanoparticle-based probe can be used for direct and simple visual identification of Brucella antigen from a wide range of bovine materials, including sperm, milk, and urine. Additionally, this test could be used to choose bulls for semen collection, to examine frozen semen before artificial insemination, or to examine bulk milk before packaging to ensure customer safety by decreasing exposure to Brucella pathogens (234).
A rapid and affordable method for diagnosing human brucellosis has been created using a gold nanobiosensor based on the localized surface plasmon resonance (LSPR). LPS of B. melitensis and B. abortus were isolated and covalently attached to the surface of gold nanoparticles for this purpose. The cutoff value for detecting captured anti-Brucella antibodies was determined by measuring the redshift on the LSPR peak, which revealed a significant difference between the healthy and true positive patients. Moreover, for an accurate assessment of this method, 40 sera from true negative and positive patients were used for comparing its results with the culture (standard method), standard tube agglutination test, and anti-brucellosis IgM and IgG levels using ELISA. The LSPR-based technique was accurate with 85, 100, 100, and 86% in terms of sensitivity, specificity, positive predictive value, and negative predictive value, respectively (235). In cattle, a comparative study was performed to evaluate the use of gold nanoparticles (GNPs) and quantum dots (QDs) as labels in the immunochromatographic serodiagnosis of brucellosis. For QDs, the results were highly sensitive in detecting specific antibodies against B. abortus LPS. So, the use of QDs in immunochromatographic serodiagnosis proved to be a good technique to increase the accuracy of immunochromatographic assay (ICA) (236).
The sensitivity and specificity for detecting Brucella pathogens have recently been increased using biosensors. They can turn biological responses into electrical signals for analysis. A bioreceptor (antibodies, enzymes, nucleic acids, microbes, etc.) is combined with a physical transducer (optical, electrochemical, or mass-based) to generate a measurable signal (237). Many new signal transduction systems based on nanoparticles have been developed to improve pathogen detection strategies (237, 238). Sandwich immunoassay nanoparticle-based biosensors are regarded as a promising way to introduce selective and sensitive diagnostic tools (239). Silica and magnetic nanoparticles have distinct properties linked to their hydrophobic surfaces and the capacity to modify their surfaces with chemical groups, and so they were used for visual and spectrophotometric detection of Brucella (240–242). An immunosensor was designed based on blue-SiNPs and paramagnetic nanoparticles (PMNPs). The synthesized immunosensor was conjugated with a polyclonal antibody against B. abortus and applied to the bacterial culture. Then, a magnet was used to create a sandwich structure of PMNPs B. abortus-blue-SiNPs. Afterward, the absorbance of the blue color produced by the silica structure was quantified using a spectrophotometer, combined with the visible color change, to determine the presence of bacterial cells in the samples (243). Li et al. developed another immunosensor using the immune magnetic beads (IMBs) probe and the QDs staphylococcal protein A (SPA) probe. The IMB probe and serum were combined first to allow the Brucella antibodies to interact with Brucella outer membrane protein coated on the IMB probe surface. The fluorescence intensity of QDs was correlated with the quantity of anti-Brucella antibodies. For rapid and label-free detection of B. melitensis in milk and milk products, a new quartz crystal microbalance (QCM) aptasensor has been developed. On the QCM chip, the particular aptamer sequence linked with magnetic nanoparticles was immobilized to identify B. melitensis (244). A rapid, accurate, simple, and sensitive fluorescent immunochromatographic strip test (ICST) based on a quantum dot fluorescent microsphere (QDFM) is another application for the field screening of brucellosis using animal serum.
The impedance technique is another rapid and inexpensive alternative to label-free biosensor technologies. For quick detection of B. melitensis, Wu et al. developed a label-free impedance immunosensor based on gold nanoparticles with modified screen-printed carbon electrodes (GNP-SPCEs). The interaction of B. melitensis antigens on the surface of GNP-SPCEs with antibody molecules was studied using cyclic voltammetry (CV) and electrochemical impedance spectroscopy (EIS). In less than 1.5 h, this biosensor was able to detect 1 × 104 and 4 × 105 CFU/ml of B. melitensis in pure culture and milk samples, respectively (245). Zinc oxide nanoparticles (ZnO-NPs) are novel types of low-cost, low-toxicity nanomaterials. In this concern, a Brucella identification biosensor has been designed for detecting Brucella in milk samples (246).
5.2. Nanotechnology for treatment of bovine brucellosis
Many host immunological responses, such as lysosomal enzymes and oxidative burst (247), cannot interact with the intracellular Brucella, and medications are unable to effectively target Brucella-infected cells as they lose their antimicrobial effectiveness in the intracellular environment (248). In humans, routine treatment regimens for brucellosis by using two or more antibiotics are the most effective way to evade relapses occurring and prevent prolonged use of these drugs (249). Khan et al. revealed that mutations in the rpoB, gyrA, and gyrB genes have been linked to antimicrobial resistance in rifampicin and ciprofloxacin (250). Resistance to rifampin, ampicillin–sulbactam, trimethoprim–sulfamethoxazole, and ampicillin in B. melitensis was estimated at 36.36, 31.82, 27.27, and 22.70%, respectively. Resistance to rifampin, trimethoprim–sulfamethoxazole, ampicillin, and ampicillin–sulbactam was detected at 35.71, 32.14, 28.57, and 32.14% of B. abortus isolates, respectively (212).
Gene therapy, which is beneficial in the clinic, may provide an alternate path to a complete cure for brucellosis by assisting in the deletion or inactivation of genes involved in Brucella multiplication in host cells. To simulate the host microorganism interaction in vitro, ovine macrophages were infected with B. melitensis and then the infected cells were transduced with CRISPR/Cas9 lentiviral vectors targeting Brucella RNA polymerase subunit A (RpolA) or virulence-associated gene (virB10) at a multiplicity of infection (MOI) of 60. When infected cells were transduced with the RpolA vector, the number of internalized Brucella per cell was decreased significantly, whereas when macrophages were transduced with a conventional lentiviral vector expressing the green fluorescence protein, the number of internalized brucellae per cell remained unaffected. This highlights the bactericidal effect of the CRISPR/Cas9 system (251).
Niosomes are drug-delivery materials made from cholesterol and a non-ionic surfactant that self-assemble (252, 253). They improve medication bioavailability by delaying drug release and changing drug half-lives, resulting in good drug accumulation within the targeted site (254). Chitosan and genetically modified organisms (GMOs) are minimal in toxicity and highly compatible with biological systems (255). The synergistic effect of chitosan/GMO is beneficial for drug targeting and maintenance by increasing the mucoadhesion characteristics of niosomes (254, 256). Abo EL-Ela et al. showed that the combination of doxycycline (DOX)-loaded chitosan–sodium alginate nanoparticles (257, 258) with in situ pH-responsive curcumin-loaded niosome hydrogel for brucellosis treatment and splenic count reduction is an effective recipe. The successful production of DOX and curcumin as nanomaterials for the treatment of intracellular bacteria may improve human brucellosis therapy due to their prolonged release, stability, and high trapping efficiency. However, the injected antimicrobial drugs did not completely eliminate Brucella in the artificially infected Guinea pigs. The results showed a considerable drop in viable Brucella numbers in the spleen and blood (259).
Using RB51 phage lysates (RL) and S19 lysates (SL), researchers have described unique and successful immunotherapy for the treatment of bovine brucellosis in cows. The combination of these two phage lysates (RL and SL) was administered subcutaneously in a 2-mL dose, and blood samples were found to be Brucella-free even after 3 months of phage cocktail immunization. RL elicited a stronger cell-mediated immune response than SL, while SL elicited a higher amount of humoral immunological response. The study’s findings are encouraging, suggesting that bacteriophage lysates could be used to treat bovine brucellosis because the conventional treatment regimens are ineffective (260).
Another study has produced polyanhydride nanoparticles of copolymers of sebacic acid to encapsulate the antibiotics doxycycline and rifampicin, and the best antibacterial activity was reported at 72 h and lasted up to a week after the nanoparticles released rifampicin for a week. In comparison with soluble drug controls, treatment of B. melitensis-infected macrophages with rifampicin-containing nanoparticles rapidly removed viable intracellular bacteria after 48 h, and pretreatment with the nano-formulations prevented intracellular infection (261–263). Infected BALB/c mice were treated for 5 days with a nanoparticle cocktail combining doxycycline and rifampicin, which reduced bacterial burden in the liver. Infected mice with B. melitensis were given either a daily 0.5 mg doxycycline dose or a single 0.5 mg doxycycline-encapsulated nanoparticles delivered once a week to demonstrate the in vivo extended antibiotic release. After 3 weeks, bacterial numbers in the spleen and liver of animals treated with the weekly nano-formulation and other animals that received daily soluble medication were statistically equivalent, indicating a 7-fold dosage sparing. These findings showed that using nanotherapeutics to treat persistent bacterial infections increased antimicrobial efficacy and improved in vivo activity through a combination of intracellular transport, dosage sparing, and prolonged release (264).
5.3. Methods for eradication of bovine brucellosis
The major goal during the settlement of Brucella control programs is decreasing the spread of microorganisms. Hosein et al. carried out a study measuring the time needed to reach Brucella-free herd status. They found that 6 months are required to reach free status, which is considered a long time, allowing infection to spread to other areas, especially under unsanitary conditions, a husbandry system that favors mixed populations of different ages, sex, aborted, and pregnant animals, and a lack of controlled animal movement. As a result, efficient animal brucellosis control needs surveillance to identify diseased animal herds, reservoir eradication, and vaccination of young heifers (64). In Dohuk Governorate, Iraq, the usage of the Rev. 1 vaccine for all female and male animals older than 3 months reduces the prevalence of brucellosis from 36 to 6.6% (23). The results revealed that successful animal vaccination, gradual culling of seropositive cattle, and small ruminants through slaughter, environmental hygiene, and human personal protection all have a role in limiting disease spread in livestock and human populations (265, 266), in addition to the eradication of carrier animals in the herd, such as dogs, cats, and mice, to eliminate infection sources (267).
Veterinary organizations should also use ongoing education and training initiatives to raise farmers’ knowledge and understanding of prevention methods and transmission routes as an important principle (91, 268). It is also worth noting that brucellosis risks are higher in dairy farms that use artificial insemination or natural breeding with non-certified brucellosis bulls, so it is important to use semen from certified free farms (97).
In the point of test and slaughter in most developed countries, culling of the suspected or reactor animals based on serology is practiced in addition to immunization. In most developing countries, this technique is not implemented due to financial constraints and the lack of healthcare infrastructure. A production strategy that avoids direct or indirect contact with diseased neighboring farms and/or contaminated feed or pasture is also essential as a part of the control plan (33). When the rate of infection is reduced to an acceptable level, a test and slaughter strategy could be implemented. So, if the disease prevalence is quite low (1 to 4%), then eradication of the disease in a short-to-medium timeframe could be achieved using a test and slaughter eradication program. At the second level, if the prevalence is low to moderate (5–10%) then eradication of the disease in a medium-long timescale could be achieved by simultaneous vaccination of young replacements as well as testing and slaughter of seropositive adult animals. At the third level, if there is a high rate of occurrence (10%), then the mass vaccination program is the only way to control the disease, regardless of the level of professional organization or financial resources available (29). Moreover, Pasteurization renders B. abortus inactive, and its survival outside the host is primarily dependent on environmental factors. Brucella can live for 2–3 months in wet soil, up to 8 months in an aborted fetus in the shade, 1–2 months in dry soil, 3–4 months in fecal matter, and up to 8 months in liquid sewage tanks. Bacterial viability is prolonged at cold temperatures, and organisms can survive in the frozen cadaver for many years (269). Raw or undercooked animal items (even bone marrow) and unpasteurized dairy products should not be ingested (270).
6. Conclusion
Brucellosis is regarded as one of the most hazardous pathogens and seriously affects humans and terrestrial animals. It is still a serious threat to animals and humans despite all the measures taken by the WHO and veterinary authorities with a significant economic effect all over the world. In the current study, we provided an updated insight into the worldwide Brucella distribution, possible predisposing factors incorporated in emerging Brucella pathogens, immune status and different types of Brucella vaccines (subunit vaccines, vector vaccines, and genetic engineering vaccines), genomics and proteomics approaches, and future perspectives for prevention and control of bovine brucellosis, which undoubtedly will be used as a platform for controlling Brucella pathogens in many endemic countries; this will help scientists, veterinarians, and animals’ owners to prevent introducing Brucella pathogens to the free areas. More specifically, many risk and predisposing factors have been summarized, including the control of fetal fluids, placenta, dogs and cats, and animal movements as the introduction of new animals to herds without certification. Moreover, pasteurization of dairy products should be done and watched as it is an important source of transmitting the disease to humans. The veterinary authorities should educate farmers to be aware of the dangers of the disease, how it can be transmitted between animals and humans, and how they can protect themselves. An important section of this review has discussed the novel techniques based on nanotechnology and genetic engineering of the antigenic structures of the pathogen to make an easy, simple, and proper diagnosis. Finally, we conclude that following many comprehensive studies in the field of genomics and proteomics of Brucella pathogens, vaccination proved to be effective for defeating these pathogens in the endemic areas as well as preventing disease introduction to the other free areas of the world.
Author contributions
ASD: Conceptualization, Data curation, Writing – original draft, Writing – review & editing. AlE: Conceptualization, Data curation, Investigation, Writing – original draft. MN: Investigation, Supervision, Review & editing. AS: Investigation, Visualization & editing. AG: Investigation, Supervision, Review & editing. GZ: Conceptualization, Writing – review & editing. SAA: Conceptualization, Investigation, Writing – review & editing. AZ: Visualization, Writing – review & editing. MZ: Investigation, Review & editing. AhE: Conceptualization, Review & editing. WM: Visualization, Review & editing. WL: Funding acquisition, Supervision, Writing – review & editing.
Funding
This study was supported by the President’s Fund of Tarim University (TDZKSS202144), National Natural Science Foundation Projects (#31772745), the Key Research and Development Program of the Ningxia Hui Autonomous Region (# 2021BEF02028), the China Agriculture Research System (Beef/yaks) of MOF and MARA (#CARS-37), and the Research and Innovation Program for Postgraduates of Tarim University (TDGRI202128).
Conflict of interest
The authors declare that the research was conducted in the absence of any commercial or financial relationships that could be construed as a potential conflict of interest.
Publisher’s note
All claims expressed in this article are solely those of the authors and do not necessarily represent those of their affiliated organizations, or those of the publisher, the editors and the reviewers. Any product that may be evaluated in this article, or claim that may be made by its manufacturer, is not guaranteed or endorsed by the publisher.
Supplementary material
The Supplementary material for this article can be found online at: https://www.frontiersin.org/articles/10.3389/fvets.2023.1255239/full#supplementary-material
References
1. Akoko, J, Pelle, R, Kivali, V, Schelling, E, Shirima, G, Machuka, EM, et al. Serological and molecular evidence of Brucella species in the rapidly growing pig sector in Kenya. BMC Vet Res. (2020) 16:1–7. doi: 10.1186/s12917-020-02346-y
2. Whatmore, AM, Koylass, MS, Muchowski, J, Edwards-Smallbone, J, Gopaul, KK, and Perrett, LL. Extended multilocus sequence analysis to describe the global population structure of the genus Brucella: phylogeography and relationship to biovars. Front Microbiol. (2016). doi: 10.3389/fmicb.2016.02049
3. Whatmore, AM. Current understanding of the genetic diversity of Brucella, an expanding genus of zoonotic pathogens. Infect Genet Evol. (2009) 9:1168–84. doi: 10.1016/j.meegid.2009.07.001
4. Blasco, JM, and Molina-Flores, B. Control and eradication of Brucella melitensis infection in sheep and goats. Vet Clin North Am - Food Anim Pract. (2011). doi: 10.1016/j.cvfa.2010.10.003
5. Wareth, G, Melzer, F, Elschner, MC, Neubauer, H, and Roesler, U. Detection of Brucella melitensis in bovine milk and milk products from apparently healthy animals in Egypt by real-time PCR. J Infect Dev Ctries. (2014) 8:1339–43. doi: 10.3855/jidc.4847
6. Cvetnić, Ž, Ðuras, M, Gomerčić, T, Reil, I, Zdelar-Tuk, M, Duvnjak, S, et al. The prevalence of brucellosis in marine mammals with a special review to Croatia. Vet Stanica. (2016) 47:229–38.
7. Dadar, M, Shahali, Y, and Whatmore, AM. Human brucellosis caused by raw dairy products: a review on the occurrence, major risk factors and prevention. Int J Food Microbiol. (2019) 292:39–47. doi: 10.1016/j.ijfoodmicro.2018.12.009
8. Poester, FP, Gonçalves, VSP, and Lage, AP. Brucellosis in Brazil. Vet Microbiol. (2002) 90:55–62. doi: 10.1016/S0378-1135(02)00245-6
9. Santos, RL, Martins, TM, Borges, ÁM, and Paixão, TA. Economic losses due to bovine brucellosis in Brazil. Pesqui Vet Bras. (2013) 33:759–64. doi: 10.1590/S0100-736X2013000600012
10. Samartino, LE. Brucellosis in Argentina. Vet Microbiol. (2002) 90:71–80. doi: 10.1016/S0378-1135(02)00247-X
11. Angara, TE, Ismail, AAA, Ibrahim, AM, and Osman, SZ. Assessment of the economic losses due to bovine brucellosis in Khartoum state, Sudan. Int J Tech Res Appl. (2016) 4:2320–8163.
12. Lindahl-Rajala, E, Hoffman, T, Fretin, D, Godfroid, J, Sattorov, N, Boqvist, S, et al. Detection and characterization of Brucella spp. in bovine milk in small-scale urban and Peri-urban farming in Tajikistan. PLoS Negl Trop Dis. (2017) 11:e0005367. doi: 10.1371/journal.pntd.0005367
13. Bamaiyi, PH, Abd-Razak, NS, and Zainal, MA. Seroprevalence and economic impact of eradicating zoonotic brucellosis in Malaysia: a case study of Melaka state of Malaysia. Vet World. (2012) 5:398–404. doi: 10.5455/vetworld.2012.398-404
14. Bamaiyi, PH, Khairani- Bejo, S, and Mohamed, ZA. The economic impact attributable to brucellosis among goat farms in Peninsula Malaysia and cost benefit analysis. Res Opin An Vet Sci. (2015) 5:52–57.
15. Robinson, C, Walter, C, Santiago, G, and Raúl, G. Economic affectation by Brucella Abortus in dairy farms, high Andean case report in Ecuador. J Dairy Vet Sci. (2019) 12:1–2. doi: 10.19080/JDVS.2019.12.555850
16. Charypkhan, D, Sultanov, AA, Ivanov, NP, Baramova, SA, Taitubayev, MK, and Torgerson, PR. Economic and health burden of brucellosis in Kazakhstan. Zoonoses Public Health. (2019) 66:487–94. doi: 10.1111/zph.12582
17. Zeng, JY, Robertson, ID, Ji, QM, La, DY, and Bruce, M. Evaluation of the economic impact of brucellosis in domestic yaks of Tibet. Transbound Emerg Dis. (2019) 66:476–87. doi: 10.1111/tbed.13049
18. Singh, BB, Dhand, NK, and Gill, JPS. Economic losses occurring due to brucellosis in Indian livestock populations. Prev Vet Med. (2015) 119:211–5. doi: 10.1016/j.prevetmed.2015.03.013
19. Singh, BB, Kostoulas, P, Gill, JPS, and Dhand, NK. Cost-benefit analysis of intervention policies for prevention and control of brucellosis in India. PLoS Negl Trop Dis. (2018) 12:e0006488. doi: 10.1371/journal.pntd.0006488
20. Panchasara, HH, Patel, JS, and Patel, PR. Economic implications of brucellosis in bovine. Indian J Vet Sci Biotechnol. (2012) 8:19–21.
21. Bardhan, D, Kumar, S, Verma, MR, and Bangar, YC. Economic losses due to brucellosis in India. Indian J Comp Microbiol Immunol Infect Dis. (2020) 41:19. doi: 10.5958/0974-0147.2020.00002.1
22. Lokamar, PN, Kutwah, MA, Atieli, H, Gumo, S, and Ouma, C. Socio-economic impacts of brucellosis on livestock production and reproduction performance in Koibatek and Marigat regions, Baringo County, Kenya. BMC Vet Res. (2020) 16:61. doi: 10.1186/s12917-020-02283-w
23. Al Hamada, A, Bruce, M, Barnes, A, Habib, I, and Robertson, D. Cost–benefit analysis of a mass vaccination strategy to control brucellosis in sheep and goats in northern Iraq. Vaccine. (2021) 9:878. doi: 10.3390/vaccines9080878
24. Godfroid, J, Nielsen, K, and Saegerman, C. Diagnosis of brucellosis in livestock and wildlife. Croat Med J. (2010) 51:296–305. doi: 10.3325/cmj.2010.51.296
25. Xie, J, Wang, J, Li, Z, Wang, W, Pang, Y, and He, Y. Ontology-based meta-analysis of animal and human adverse events associated with licensed brucellosis vaccines. Front Pharmacol. (2018). doi: 10.3389/fphar.2018.00503
26. Olsen, SC, and Palmer, MV. Advancement of knowledge of Brucella over the past 50 years. Vet Pathol. (2014) 51:1076–89. doi: 10.1177/0300985814540545
27. Dias, SP, Sequeira, J, and Almeida, M. Spastic paraparesis and sensorineural hearing loss: keep brucellosis in mind. J Neurol Sci. (2018) 385:144–5. doi: 10.1016/j.jns.2017.12.036
28. Sierra, E, Fernández, A, Felipe-Jiménez, I, Zucca, D, Di Francesco, G, Díaz-Delgado, J, et al. Neurobrucellosis in a common bottlenose dolphin (Tursiops truncatus) stranded in the Canary Islands. BMC Vet Res. (2019) 15:353. doi: 10.1186/s12917-019-2089-0
29. Blasco, JM, Moreno, E, and Moriyón, I. Brucellosis. Vet Vacc. (2021):295–316. doi: 10.1002/9781119506287.ch22
30. Welburn, SC, Beange, I, Ducrotoy, MJ, and Okello, AL. The neglected zoonoses--the case for integrated control and advocacy. Clin Microbiol Infect. (2015) 21:433–43. doi: 10.1016/j.cmi.2015.04.011
31. Tuon, FF, Gondolfo, RB, and Cerchiari, N. Human-to-human transmission of Brucella - a systematic review. Tropical Med Int Health. (2017) 22:539–46. doi: 10.1111/tmi.12856
32. Deka, RP, Magnusson, U, Grace, D, and Lindahl, J. Bovine brucellosis: prevalence, risk factors, economic cost and control options with particular reference to India- a review. Infect Ecol Epidemiol. (2018) 8:1556548. doi: 10.1080/20008686.2018.1556548
33. Hull, NC, and Schumaker, BA. Comparisons of brucellosis between human and veterinary medicine. Infect Ecol Epidemiol. (2018). doi: 10.1080/20008686.2018.1500846
34. Hu, J, Zhang, X, Yang, H, et al. Brucellosis screening and follow-up of seropositive asymptomatic subjects among household members of shepherds in China. Eur J Clin Microbiol Infect Dis. (2021) 40:1325–8. doi: 10.1007/s10096-020-04115-z
35. Ghugey, S, Setia, M, and Deshmukh, J. Human brucellosis: seroprevalence and associated exposure factors among the rural population in Nagpur, Maharashtra, India. J Fam Med Prim Care. (2021) 10:1028. doi: 10.4103/jfmpc.jfmpc_1153_20
36. Yousaf, R, Khan, I, Shehzad, W, Hussain, R, Ali, S, Neubauer, H, et al. Seroprevalence and molecular detection of brucellosis in hospitalized patients in Lahore hospitals, Pakistan. Infect Dis Rep. (2021) 13:166–72. doi: 10.3390/idr13010018
37. Bamaiyi, PH, Hassan, L, Khairani-Bejo, S, Zainalabidin, M, Adzhar, A, Mokhtar, N, et al. Seroprevalence of brucellosis among farmers and veterinary technical staff in peninsular Malaysia. Sains Malaysiana. (2017) 46:933–43. doi: 10.17576/jsm-2017-4606-13
38. Al-Hakami, A, Alqahtani, A, Moosa, R, et al. Seroprevalence of brucellosis among exposed agro-pastoral communities in southern Saudi Arabia. Asian Pac J Trop Med. (2019) 12:545. doi: 10.4103/1995-7645.272484
39. Andriopoulos, P, Floros, D, Gioti, N, Mariolis, A, Rojas Gil, AP, and Tsironi, M. Brucella seroprevalence in a high-risk population in Greece: a cross-sectional study. Interdiscip Perspect Infect Dis. (2018). doi: 10.1155/2018/8751921
40. El-Moselhy, EA, Zayet, H, El-Khateeb, AS, Mohammed, AS, and El-Tiby, DM. Human brucellosis: seroprevalence, risk factors, and barriers of protection among slaughterhouses’ Workers in El-Menia. Arch Clin Pathol. (2018) 1:1000102.
41. Madut, NA, Muwonge, A, Nasinyama, GW, Muma, JB, Godfroid, J, Jubara, AS, et al. The sero-prevalence of brucellosis in cattle and their herders in Bahr el Ghazal region, South Sudan. PLoS Negl Trop Dis. (2018) 12:e0006456. doi: 10.1371/JOURNAL.PNTD.0006456
42. Akinyemi, KO, Fakorede, CO, Amisu, KO, and Wareth, G. Human and animal brucellosis in Nigeria: a systemic review and meta-analysis in the last twenty-one years (2001-2021). Vet Sci. (2022). doi: 10.3390/vetsci9080384
43. Awah-Ndukum, J, Mouiche, MMM, Kouonmo-Ngnoyum, L, Bayang, HN, Manchang, TK, Poueme, RSN, et al. Seroprevalence and risk factors of brucellosis among slaughtered indigenous cattle, abattoir personnel and pregnant women in Ngaoundéré, Cameroon. BMC Infect Dis. (2018) 18:611. doi: 10.1186/S12879-018-3522-X
44. Maiyo, G, and Obey, JK. Distribution and prevalence of human brucellosis among patients reporting at chemundu dispensary, Nandi County, KENYA. Barat Interdiscip Res J. (2016):73–82.
45. Tumwine, G, Matovu, E, Kabasa, JD, Owiny, DO, and Majalija, S. Human brucellosis: sero-prevalence and associated risk factors in agro-pastoral communities of Kiboga District, Central Uganda. BMC Public Health. (2015) 15:900. doi: 10.1186/S12889-015-2242-Z
46. Sagamiko, FD, Muma, JB, Karimuribo, ED, Mwanza, AA, Mfune, RL, Sindato, C, et al. Seroprevalence of human Brucellosis and associated risk factors among high risk occupations in Mbeya region of Tanzania. bioRxiv. (2019):688705.
47. Elmonir, W, Hegazy, Y, AbdelHamid, N, and Elbauomy, E. Brucellosis at the human-animal interface in Kafrelsheikh governorate, Egypt. Alexandria J Vet Sci. (2016) 50:1. doi: 10.5455/ajvs.229337
48. Abd El-Wahab, EW, Hegazy, Y, El-Tras, WF, Mikeal, A, Kapaby, AF, Abdelfatah, M, et al. Knowledge, attitudes and practices (KAPs) and risk factors of brucellosis at the human-animal interface in the Nile Delta, Egypt. bioRxiv. (2019). doi: 10.1101/607655
49. Aliyev, J, Alakbarova, M, Garayusifova, A, Omarov, A, Aliyeva, S, Fretin, D, et al. Identification and molecular characterization of Brucella abortus and Brucella melitensis isolated from milk in cattle in Azerbaijan. BMC Vet Res. (2022). doi: 10.1186/s12917-022-03155-1
50. Cossaboom, CM, Kharod, GA, Salzer, JS, et al. Notes from the field: Brucella abortus vaccine strain RB51 infection and exposures associated with raw milk consumption-Wise County, Texas, 2017. MMWR Morb Mortal Wkly Rep. (2018) 67:286. doi: 10.15585/mmwr.mm6709a4
51. Benítez-Serrano, JC, Palomares-Resendiz, G, Díaz-Aparicio, E, Hernández-Castro, R, Martínez-Pérez, L, Suárez-Güemes, F, et al. Survival of Brucella abortus RB51 and S19 vaccine strains in fresh and ripened cheeses. Foodborne Pathog Dis. (2022) 19:535–42. doi: 10.1089/fpd.2022.0001
52. Gould, LH, Mungai, E, and Barton Behravesh, C. Outbreaks attributed to cheese: differences between outbreaks caused by unpasteurized and pasteurized dairy products, United States, 1998-2011. Foodborne Pathog Dis. (2014) 11:545–51. doi: 10.1089/fpd.2013.1650
53. Swai, ES, and Schoonman, L. Microbial quality and associated health risks of raw milk marketed in the Tanga region of Tanzania. Asian Pac J Trop Biomed. (2011) 1:217–22. doi: 10.1016/S2221-1691(11)60030-0
54. Bayasgalan, C, Chultemdorj, T, Roth, F, Zinsstag, J, Hattendorf, J, Badmaa, B, et al. Risk factors of brucellosis seropositivity in Bactrian camels of Mongolia. BMC Vet Res. (2018) 14:1–11. doi: 10.1186/s12917-018-1664-0
55. Ran, X, Cheng, J, Wang, M, Chen, X, Wang, H, Ge, Y, et al. Brucellosis seroprevalence in dairy cattle in China during 2008–2018: a systematic review and meta-analysis. Acta Trop. (2019). doi: 10.1016/j.actatropica.2018.10.002
56. Ali, S, Akhter, S, Neubauer, H, Melzer, F, Khan, I, Abatih, EN, et al. Seroprevalence and risk factors associated with bovine brucellosis in the Potohar Plateau, Pakistan. BMC Res Notes. (2017) 10:73. doi: 10.1186/S13104-017-2394-2
57. Barman, NN, Patil, SS, Kurli, R, Deka, P, Bora, DP, Deka, G, et al. Meta-analysis of the prevalence of livestock diseases in North eastern region of India. Vet World. (2020) 13:80–91. doi: 10.14202/vetworld.2020.80-91
58. Pathak, AD, Dubal, ZB, Karunakaran, M, et al. Apparent seroprevalence, isolation and identification of risk factors for brucellosis among dairy cattle in Goa, India. Comp Immunol Microbiol Infect Dis. (2016) 47:1–6. doi: 10.1016/j.cimid.2016.05.004
59. Fact Sheet - Brucellosis - Canadian Food Inspection Agency (n.d.). https://inspection.canada.ca/animal-health/terrestrial-animals/diseases/reportable/brucellosis/fact-sheet/eng/1305673222206/1305673334337 [Accessed February 13, 2022].
60. Pappas, G, Papadimitriou, P, Akritidis, N, Christou, L, and Tsianos, EV. The new global map of human brucellosis. Lancet Infect Dis. (2006). doi: 10.1016/S1473-3099(06)70382-6
61. Musallam, II, Abo-Shehada, M, Omar, M, and Guitian, J. Cross-sectional study of brucellosis in Jordan: prevalence, risk factors and spatial distribution in small ruminants and cattle. Prev Vet Med. (2015) 118:387–96. doi: 10.1016/j.prevetmed.2014.12.020
62. Wareth, G, El-Diasty, M, Melzer, F, et al. MLVA-16 genotyping of Brucella abortus and Brucella melitensis isolates from different animal species in Egypt: geographical relatedness and the Mediterranean lineage. PathoGenetics. (2020, 2020) 9:498.
63. Sayour, AE, Elbauomy, E, Abdel-Hamid, NH, Mahrous, A, Carychao, D, Cooley, MB, et al. MLVA fingerprinting of Brucella melitensis circulating among livestock and cases of sporadic human illness in Egypt. Transbound Emerg Dis. (2020) 67:2435–45. doi: 10.1111/tbed.13581
64. Hosein, HI, Zaki, HM, Safwat, NM, Menshawy, AMS, Rouby, S, Mahrous, A, et al. Evaluation of the general Organization of Veterinary Services control program of animal brucellosis in Egypt: an outbreak investigation of brucellosis in buffalo. Vet World. (2018) 11:748–57. doi: 10.14202/vetworld.2018.748-757
65. Spicic, S, Zdelar-Tuk, M, Ponsart, C, Hendriksen, RS, Reil, I, Girault, G, et al. New Brucella variant isolated from Croatian cattle. BMC Vet Res. (2021) 17:1–5. doi: 10.1186/s12917-021-02833-w
66. Chaka, H, Aboset, G, Garoma, A, Gumi, B, and Thys, E. Cross-sectional survey of brucellosis and associated risk factors in the livestock-wildlife interface area of Nechisar National Park, Ethiopia. Trop Anim Health Prod. (2018) 50:1041–9. doi: 10.1007/s11250-018-1528-4
67. Getachew, T, Getachew, G, Sintayehu, G, Getenet, M, and Fasil, A. Bayesian estimation of sensitivity and specificity of rose Bengal, complement fixation, and indirect ELISA tests for the diagnosis of bovine brucellosis in Ethiopia. Vet Med Int. (2016). doi: 10.1155/2016/8032753
68. Aworh, MK, Okolocha, EC, Awosanya, EJ, and Fasina, FO. Sero-prevalence and intrinsic factors associated with Brucella infection in food animals slaughtered at abattoirs in Abuja, Nigeria. BMC Res Notes. (2017) 10:499. doi: 10.1186/S13104-017-2827-Y
69. Kamwine, M, Orikiriza, P, Taseera, K, Iramiot, JS, Ojuka, P, Ikiriza, S, et al. Prevalence of antibodies to Brucella species in commercial raw bovine milk in southwestern Uganda. BMC Res Notes. (2017) 10:215. doi: 10.1186/s13104-017-2537-5
70. Asakura, S, Makingi, G, Kazwala, R, and Makita, K. Herd-level risk factors associated with Brucella sero-positivity in cattle, and perception and behaviours on the disease control among agro-pastoralists in Tanzania. Acta Trop. (2018) 187:99–107. doi: 10.1016/j.actatropica.2018.07.010
71. Tasiame, W, Emikpe, BO, Folitse, RD, Fofie, CO, Burimuah, V, Johnson, S, et al. The prevalence of brucellosis in cattle and their handlers in north Tongu district of VOLTA region, Ghana. Afr J Infect Dis. (2016) 10:111–7. doi: 10.21010/ajid.v10i2.6
72. Carbonero, A, Guzmán, LT, García-Bocanegra, I, Borge, C, Adaszek, L, Arenas, A, et al. Seroprevalence and risk factors associated with Brucella seropositivity in dairy and mixed cattle herds from Ecuador. Trop Anim Health Prod. (2018) 50:197–203. doi: 10.1007/s11250-017-1421-6
73. Lindahl, JF, Vrentas, CE, Deka, RP, Hazarika, RA, Rahman, H, Bambal, RG, et al. Brucellosis in India: results of a collaborative workshop to define one health priorities. Trop Anim Health Prod. (2020) 52:387–96. doi: 10.1007/s11250-019-02029-3
74. Bagheri Nejad, R, Krecek, RC, Khalaf, OH, Hailat, N, and Arenas-Gamboa, AM. Brucellosis in the Middle East: current situation and a pathway forward. PLoS Negl Trop Dis. (2020) 14:e0008071. doi: 10.1371/journal.pntd.0008071
75. Seimenis, A, Araj, GF, Moriyón, I, and Tabbaa, D. Brucellosis prevention and control in the Mediterranean & Middle East regions _ a guidance towards approaching the targets. J Med Liban. (2019). doi: 10.12816/0053749
76. Li, F, Du, L, Zhen, H, et al. Follow-up outcomes of asymptomatic brucellosis: a systematic review and meta-analysis. Emerg Microbes Infect. (2023). doi: 10.1080/22221751.2023.2185464
77. Elderbrook, M, Schumaker, B, Cornish, T, Peck, D, and Sondgeroth, K. Seroprevalence and risk factors of Brucella ovis in domestic sheep in Wyoming, USA. BMC Vet Res. (2019) 15:1–12. doi: 10.1186/s12917-019-1995-5
78. Zeng, J, Duoji, C, Yuan, Z, Yuzhen, S, Fan, W, Tian, L, et al. Seroprevalence and risk factors for bovine brucellosis in domestic yaks (Bos grunniens) in Tibet, China. Trop Anim Health Prod. (2017) 49:1339–44. doi: 10.1007/s11250-017-1331-7
79. Caetano, MC, Afonso, F, Ribeiro, R, Fonseca, AP, Abernethy, DA, and Boinas, F. Control of bovine brucellosis from persistently infected holdings using RB51 vaccination with test-and-slaughter: a comparative case report from a high incidence area in Portugal. Transbound Emerg Dis. (2016) 63:e39–47. doi: 10.1111/tbed.12228
80. Soomro, AH. A study on prevalence and risk factors of brucellosis in cattle and buffaloes in District Hyderabad, Pakistan. J Anim Heal Prod. (2014) 2:33–7. doi: 10.14737/journal.jahp/2014/2.3.33.37
81. Singh, A, and Reddy, N. Seroprevalence and risk factors associated with bovine brucellosis in western Uttar Pradesh, India. Indian J Anim Sci. (2016) 86:131–5.
82. Lindahl, E, Sattorov, N, Boqvist, S, Sattori, I, and Magnusson, U. Seropositivity and risk factors for Brucella in dairy cows in urban and peri-urban small-scale farming in Tajikistan. Trop Anim Health Prod. (2014) 46:563. doi: 10.1007/s11250-013-0534-9
83. Ul-Islam, MR, Gupta, MP, Gursimran, F, Sidhu, PK, Shafi, TA, Bhat, SA, et al. Sero-epidemiology of brucellosis in organized cattle and buffaloes in Punjab (India). Adv Anim Vet Sci. (2013) 1:5–8.
84. Shome, R. Bovine brucellosis in organized farms of India - an assessment of diagnostic assays and risk factors. Adv Anim Vet Sci. (2014) 2:557–64. doi: 10.14737/journal.aavs/2014/2.10.557.564
85. Ndazigaruye, G, Mushonga, B, Kandiwa, E, Samkange, A, and Segwagwe, BE. Prevalence and risk factors for brucellosis seropositivity in cattle in Nyagatare District, Eastern Province, Rwanda. J S Afr Vet Assoc. (2018) 89:1019–9128. doi: 10.4102/jsava.v89i0.1625
86. Khan, MZ, and Zahoor, M. An overview of brucellosis in cattle and humans, and its serological and molecular diagnosis in control strategies. Trop Med Infect Dis. (2018) 3:65. doi: 10.3390/tropicalmed3020065
87. Mubanga, M, Mfune, RL, Kothowa, J, Mohamud, AS, Chanda, C, Mcgiven, J, et al. Brucella Seroprevalence and associated risk factors in occupationally exposed humans in selected districts of Southern Province, Zambia. Front Public Health. (2021) 9:1601. doi: 10.3389/fpubh.2021.745244
88. López-Santiago, R, Sánchez-Argáez, AB, De Alba-Núñez, LG, Baltierra-Uribe, SL, and Moreno-Lafont, MC. Immune response to mucosal Brucella infection. Front Immunol. (2019) 10:1759. doi: 10.3389/fimmu.2019.01759
89. Avila-Calderón, ED, Flores-Romo, L, Sharon, W, Donis-Maturano, L, Becerril-García, MA, Arreola, MGA, et al. Dendritic cells and Brucella spp. interaction: the sentinel host and the stealthy pathogen. Folia Microbiol. (2019, 2019) 651:1–16.
90. Celli, J. The intracellular lifecycle of Brucella spp. Microbiol Spectr. (2019) 7:1–11. doi: 10.1128/microbiolspec.BAI-0006-2019
91. Dadar, M, Fakhri, Y, Shahali, Y, and Mousavi Khaneghah, A. Contamination of milk and dairy products by Brucella species: a global systematic review and meta-analysis. Food Res Int. (2020). doi: 10.1016/j.foodres.2019.108775
92. de Alencar Mota, ALA, Ferreira, F, Ferreira Neto, JS, Dias, RA, Amaku, M, Hildebrand Grisi-Filho, JH, et al. Large-scale study of herd-level risk factors for bovine brucellosis in Brazil. Acta Trop. (2016) 164:226–32. doi: 10.1016/j.actatropica.2016.09.016
93. Makita, K, Fèvre, EM, Waiswa, C, Eisler, MC, Thrusfield, M, and Welburn, SC. Herd prevalence of bovine brucellosis and analysis of risk factors in cattle in urban and peri-urban areas of the Kampala economic zone, Uganda. BMC Vet Res. (2011) 7:60. doi: 10.1186/1746-6148-7-60
94. Coelho, AM, Coelho, AC, Roboredo, M, and Rodrigues, J. A case–control study of risk factors for brucellosis seropositivity in Portuguese small ruminants herds. Prev Vet Med. (2007) 82:291–301. doi: 10.1016/j.prevetmed.2007.06.001
95. Patel, MD, Patel, PR, Prajapati, MG, Kanani, AN, Tyagi, KK, and Fulsoundar, AB. Prevalence and risk factor’s analysis of bovine brucellosis in peri-urban areas under intensive system of production in Gujarat, India. Vet World. (2014) 7:509–16. doi: 10.14202/vetworld.2014.509-516
96. Alhaji, NB, Wungak, YS, and Bertu, WJ. Serological survey of bovine brucellosis in Fulani nomadic cattle breeds (Bos indicus) of North-Central Nigeria: potential risk factors and zoonotic implications. Acta Trop. (2016) 153:28–35. doi: 10.1016/j.actatropica.2015.10.003
97. Cárdenas, L, Awada, L, Tizzani, P, Cáceres, P, and Casal, J. Characterization and evolution of countries affected by bovine brucellosis (1996–2014). Transbound Emerg Dis. (2019) 66:1280–90. doi: 10.1111/tbed.13144
98. Kant, N, Kulshreshtha, P, Singh, R, Mal, A, Dwivedi, A, Ahuja, R, et al. A study to identify the practices of the buffalo keepers which inadvertently lead to the spread of brucellosis in Delhi. BMC Vet Res. (2018) 14:329–8. doi: 10.1186/s12917-018-1670-2
99. Cárdenas, L, Peña, M, Melo, O, and Casal, J. Risk factors for new bovine brucellosis infections in Colombian herds. BMC Vet Res. (2019) 15:1–8. doi: 10.1186/s12917-019-1825-9
100. Pandian, SJ, Ray, PK, Chandran, PC, and Kumar, M. Seroprevalence of Brucella abortus and Leptospira hardjo in cattle. Vet World. (2015) 8:217. doi: 10.14202/vetworld.2015.217-220
101. Aznar, MN, Linares, FJ, Cosentino, B, Sago, A, La Sala, L, León, E, et al. Prevalence and spatial distribution of bovine brucellosis in San Luis and La Pampa, Argentina. BMC Vet Res. (2015) 11:1–7. doi: 10.1186/s12917-015-0535-1
102. Njeru, J, Nthiwa, D, Akoko, J, Oyas, H, and Bett, B. Incidence of Brucella infection in various livestock species raised under the pastoral production system in Isiolo County, Kenya. BMC Vet Res. (2021) 17:1–12. doi: 10.1186/s12917-021-03036-z
104. Abdel-Hamid, NH, Ghobashy, HM, Beleta, EI, Elbauomy, EM, Ismail, RI, Nagati, SF, et al. Risk factors and molecular genotyping of Brucella melitensis strains recovered from humans and their owned cattle in upper Egypt. One Health. (2021). doi: 10.1016/j.onehlt.2021.100281
105. Bronner, A, Morignat, E, and Calavas, D. Respective influence of veterinarians and local institutional stakeholders on the event-driven surveillance system for bovine Brucellosis in France. BMC Vet Res. (2015) 11:1–6. doi: 10.1186/s12917-015-0499-1
106. Li, Y, Tan, D, Xue, S, Shen, C, Ning, H, Cai, C, et al. Prevalence, distribution and risk factors for brucellosis infection in goat farms in Ningxiang, China. BMC Vet Res. (2021) 17:1–9. doi: 10.1186/s12917-021-02743-x
107. Gompo, TR, Shah, R, Tiwari, I, and Gurung, YB. Sero-epidemiology and associated risk factors of brucellosis among sheep and goat population in the south western Nepal: a comparative study. BMC Vet Res. (2021) 17:1–10. doi: 10.1186/s12917-021-02835-8
108. Skendros, P, and Boura, P. Immunity to brucellosis. OIE Rev Sci Tech. (2013) 32:137–47. doi: 10.20506/rst.32.1.2190
109. Elrashedy, A, Gaafar, M, Mousa, W, Nayel, M, Salama, A, Zaghawa, A, et al. Immune response and recent advances in diagnosis and control of brucellosis. Ger J Vet Res. (2022) 2:10–24. doi: 10.51585/gjvr.2022.1.0033
110. Moreno, E, and Barquero-Calvo, E. The role of neutrophils in brucellosis. Microbiol Mol Biol Rev. (2020). doi: 10.1128/MMBR.00048-20
111. Mora-Cartín, R, Gutiérrez-Jiménez, C, Alfaro-Alarcón, A, Chaves-Olarte, E, Chacón-Díaz, C, Barquero-Calvo, E, et al. Neutrophils dampen adaptive immunity in brucellosis. Infect Immun. (2019). doi: 10.1128/IAI.00118-19
112. Li, JY, Liu, Y, Gao, XX, Gao, X, and Cai, H. TLR2 and TLR4 signaling pathways are required for recombinant Brucella abortus BCSP31-induced cytokine production, functional upregulation of mouse macrophages, and the Th1 immune response in vivo and in vitro. Cell Mol Immunol. (2014) 11:477–94. doi: 10.1038/cmi.2014.28
113. Jiao, H, Zhou, Z, Li, B, Xiao, Y, Li, M, Zeng, H, et al. The mechanism of facultative intracellular parasitism of Brucella. Int J Mol Sci. (2021). doi: 10.3390/ijms22073673
114. Andersen-Nissen, E, Smith, KD, Strobe, KL, Rassoulian Barrett, SL, Cookson, BT, Logan, SM, et al. Evasion of toll-like receptor 5 by flagellated bacteria. Proc Natl Acad Sci U S A. (2005). doi: 10.1073/pnas.0502040102
115. Wang, LL, Chen, XF, Hu, P, Lu, SY, Fu, BQ, Li, YS, et al. Host Prdx6 contributing to the intracellular survival of Brucella suis S2 strain. BMC Vet Res. (2019) 15:1–12. doi: 10.1186/s12917-019-2049-8
116. Dawood, A, Algharib, SA, Zhao, G, Zhu, T, Qi, M, Delai, K, et al. Mycoplasmas as host pantropic and specific pathogens: clinical implications, gene transfer, virulence factors, and future perspectives. Front Cell Infect Microbiol. (2022) 0:513.
117. Zhu, T, Liu, H, Su, L, et al. MicroRNA-18b-5p downregulation favors Mycobacterium tuberculosis clearance in macrophages via HIF-1α by promoting an inflammatory response. ACS Infect Dis. (2021) 7:800–10. doi: 10.1021/acsinfecdis.0c00650
118. Zhu, T, Liu, H, Su, L, Dawood, A, Hu, C, Chen, X, et al. Identification of unique key miRNAs, TFs, and mRNAs in virulent MTB infection macrophages by network analysis. Int J Mol Sci. (2022). doi: 10.3390/ijms24010187
119. Zhao, G, Lu, D, Wang, S, et al. Novel mycoplasma nucleomodulin MbovP475 decreased cell viability by regulating expression of CRYAB and MCF2L2. Virulence. (2022) 13:1590–613. doi: 10.1080/21505594.2022.2117762
120. Guo, X, Zeng, H, Li, M, et al. The mechanism of chronic intracellular infection with Brucella spp. Front Cell Infect Microbiol. (2023). doi: 10.3389/fcimb.2023.1129172
121. Ke, Y, Wang, Y, Li, W, and Chen, Z. Type IV secretion system of Brucella spp. and its effectors. Front Cell Infect Microbiol. (2015). doi: 10.3389/fcimb.2015.00072
122. Martirosyan, A, Moreno, E, and Gorvel, JP. An evolutionary strategy for a stealthy intracellular Brucella pathogen. Immunol Rev. (2011) 240:211–34. doi: 10.1111/j.1600-065X.2010.00982.x
123. Kianmehr, Z, Soleimanjahi, H, Ardestani, SK, Fotouhi, F, and Abdoli, A. Influence of Brucella abortus lipopolysaccharide as an adjuvant on the immunogenicity of HPV-16 L1VLP vaccine in mice. Med Microbiol Immunol. (2015). doi: 10.1007/s00430-014-0356-z
124. Velásquez, LN, Milillo, MA, Delpino, MV, Trotta, A, Mercogliano, MF, Pozner, RG, et al. Inhibition of MHC-I by Brucella abortus is an early event during infection and involves EGFR pathway. Immunol Cell Biol. (2017). doi: 10.1038/icb.2016.111
125. Guimarães, ES, Martins, JM, Gomes, MTR, Cerqueira, DM, and Oliveira, SC. Lack of interleukin-6 affects ifn-γ and tnf-α production and early in vivo control of Brucella abortus infection. Pathogens. (2020) 9:1–14. doi: 10.3390/pathogens9121040
126. Salcedo, SP, Marchesini, MI, Degos, C, et al. BtpB, a novel Brucella TIR-containing effector protein with immune modulatory functions. Front Cell Infect Microbiol. (2013). doi: 10.3389/fcimb.2013.00028
127. Ahmed, W, Zheng, K, and Liu, Z-F. Establishment of chronic infection: Brucella’s stealth strategy. Front Cell Infect Microbiol. (2016). doi: 10.3389/fcimb.2016.00030
128. Rajpoot, S, Wary, KK, Ibbott, R, Liu, D, Saqib, U, Thurston, TLM, et al. TIRAP in the mechanism of inflammation. Front Immunol. (2021). doi: 10.3389/fimmu.2021.697588
129. Spera, JM, Ugalde, JE, Mucci, J, Comerci, DJ, and Ugalde, RA. A B lymphocyte mitogen is a Brucella abortus virulence factor required for persistent infection. Proc Natl Acad Sci. (2006) 103:16514–9. doi: 10.1073/pnas.0603362103
130. Olsen, SC, and Stoffregen, WS. Essential role of vaccines in brucellosis control and eradication programs for livestock. Expert Rev Vaccines. (2014) 4:915–28. doi: 10.1586/1476058446915
131. Aznar, MN, Arregui, M, Humblet, MF, Samartino, LE, and Saegerman, C. Methodology for the assessment of brucellosis management practices and its vaccination campaign: example in two argentine districts. BMC Vet Res. (2017) 13:1–11. doi: 10.1186/s12917-017-1201-6
132. Masjedian Jezi, F, Razavi, S, Mirnejad, R, and Zamani, K. Immunogenic and protective antigens of Brucella as vaccine candidates. Comp Immunol Microbiol Infect Dis. (2019) 65:29–36. doi: 10.1016/j.cimid.2019.03.015
133. Legesse, A, Mekuriaw, A, Gelaye, E, Abayneh, T, Getachew, B, Weldemedhin, W, et al. Comparative evaluation of RBPT, I-ELISA, and CFT for the diagnosis of brucellosis and PCR detection of Brucella species from Ethiopian sheep, goats, and cattle sera. BMC Microbiol. (2023) 23:216. doi: 10.1186/s12866-023-02962-2
134. Saidu, AS, Singh, M, Kumar, A, Mahajan, NK, Mittal, D, Chhabra, R, et al. Studies on intra-ocular vaccination of adult cattle with reduced dose Brucella abortus strain-19 vaccine. Heliyon. (2022) 8:e08937. doi: 10.1016/j.heliyon.2022.e08937
135. Mathur, S, Banai, M, and Cohen, D. Natural Brucella melitensis infection and rev. 1 vaccination induce specific Brucella O-polysaccharide antibodies involved in complement mediated Brucella cell killing. Vaccine. (2022) 10:317. doi: 10.3390/vaccines10020317
136. de Oliveira, MM, Pereira, CR, de Oliveira, IRC, Godfroid, J, Lage, AP, and Dorneles, EMS. Efficacy of Brucella abortus S19 and RB51 vaccine strains: a systematic review and meta-analysis. Transbound Emerg Dis. (2022) 69:3457–67. doi: 10.1111/tbed.14705
137. Gheibi, A, Khanahmad, H, Kashfi, K, Sarmadi, M, and Khorramizadeh, MR. Development of new generation of vaccines for Brucella abortus. Heliyon. (2018) 4:e01079. doi: 10.1016/j.heliyon.2018.e01079
138. Schurig, GG, Sriranganathan, N, and Corbel, MJ. Brucellosis vaccines: past, present and future. Vet Microbiol. (2002) 90:479–96. doi: 10.1016/S0378-1135(02)00255-9
139. Ivanov, AV, Salmakov, KM, Olsen, SC, and Plumb, GE. A live vaccine from Brucella abortus strain 82 for control of cattle brucellosis in the Russian Federation. Anim Health Res Rev. (2011) 12:113–21. doi: 10.1017/S1466252311000028
140. Alves, AJS, Rocha, F, Amaku, M, Ferreira, F, Telles, EO, Grisi Filho, JHH, et al. Economic analysis of vaccination to control bovine brucellosis in the states of Sao Paulo and Mato Grosso, Brazil. Prev Vet Med. (2015) 118:351–8. doi: 10.1016/j.prevetmed.2014.12.010
141. Chacón-Díaz, C, Zabalza-Baranguá, A, Román, BS, et al. Brucella abortus S19 GFP-tagged vaccine allows the serological identification of vaccinated cattle. PLoS One. (2021) 16:e0260288. doi: 10.1371/journal.pone.0260288
142. Suárez-Esquivel, M, Ruiz-Villalobos, N, Castillo-Zeledón, A, Jiménez-Rojas, C, Roop Ii, RM, Comerci, DJ, et al. Brucella abortus strain 2308 Wisconsin genome: importance of the definition of reference strains. Front Microbiol. (2016) 7:1557. doi: 10.3389/fmicb.2016.01557
143. Moriyón, I, Grilló, MJ, Monreal, D, González, D, Marín, C, López-Goñi, I, et al. Rough vaccines in animal brucellosis: structural and genetic basis and present status. Vet Res. (2004) 35:1–38. doi: 10.1051/vetres:2003037
144. Edmonds, MD, Cloeckaert, A, Booth, NJ, Fulton, WT, Hagius, SD, Walker, JV, et al. Attenuation of a Brucella abortus mutant lacking a major 25 kDa outer membrane protein in cattle. Am J Vet Res. (2001) 62:1461–6. doi: 10.2460/ajvr.2001.62.1461
145. Truong, QL, Cho, Y, Park, S, Park, BK, and Hahn, TW. Brucella abortus mutants lacking ATP-binding cassette transporter proteins are highly attenuated in virulence and confer protective immunity against virulent B. abortus challenge in BALB/c mice. Microb Pathog. (2016) 95:175–85. doi: 10.1016/j.micpath.2016.04.009
146. Dorneles, EMS, Teixeira-Carvalho, A, Araújo, MSS, Lima, GK, Martins-Filho, OA, Sriranganathan, N, et al. T lymphocytes subsets and cytokine pattern induced by vaccination against bovine brucellosis employing S19 calfhood vaccination and adult RB51 revaccination. Vaccine. (2014) 32:6034–8. doi: 10.1016/j.vaccine.2014.08.060
147. Lalsiamthara, J, and Lee, JH. Development and trial of vaccines against Brucella. J Vet Sci. (2017) 18:281–90. doi: 10.4142/jvs.2017.18.S1.281
148. Zhang, H, Wang, Y, Wang, Y, Deng, X, Ji, T, Ma, Z, et al. Using a relative quantitative proteomic method to identify differentially abundant proteins in Brucella melitensis Biovar 3 and Brucella melitensis M5-90. Front Immunol. (2022) 13:1. doi: 10.3389/fimmu.2022.929040
149. Uslu, A, and Erganis, O. Development of Brucella melitensis rev.1 ΔOmp19 mutants with DIVA feature and comparison of their efficacy against three commercial vaccines in a mouse model. Mol Immunol. (2021) 133:44–52. doi: 10.1016/j.molimm.2021.02.006
150. Lalsiamthara, J, Won, G, and Lee, JH. Effect of immunization routes and protective efficacy of Brucella antigens delivered via Salmonella vector vaccine. J Vet Sci. (2018) 19:416–25. doi: 10.4142/jvs.2018.19.3.416
151. Kim, WK, Moon, JY, Kim, S, and Hur, J. Comparison between immunization routes of live attenuated Salmonella Typhimurium strains expressing BCSP31, Omp3b, and SOD of Brucella abortus in murine model. Front Microbiol. (2016). doi: 10.3389/fmicb.2016.00550
152. Alizadeh, H, Dezfulian, M, Rahnema, M, Fallah, J, and Esmaeili, D. Protection of BALB/c mice against pathogenic Brucella abortus and Brucella melitensis by vaccination with recombinant Omp16. Iran J Basic Med Sci. (2019) 22:1302–7. doi: 10.22038/ijbms.2019.36369.8665
153. Gupta, S, Singh, D, Gupta, M, and Bhatnagar, R. A combined subunit vaccine comprising BP26, Omp25 and L7/L12 against brucellosis. Pathog Dis. (2019) 77:ftaa002. doi: 10.1093/femspd/ftaa002
154. Huy, TXN, Bernardo Reyes, AW, Vu, SH, Arayan, LT, Hop, HT, Min, WG, et al. Immunogenicity and protective response induced by recombinant Brucella abortus proteins Adk, SecB and combination of these two recombinant proteins against a virulent strain B. abortus 544 infection in BALB/c mice. Microb Pathog. (2020). doi: 10.1016/j.micpath.2020.104137
155. Jain, S, Afley, P, and Kumar, S. Immunological responses to recombinant cysteine synthase a of Brucella abortus in BALB/c mice. World J Microbiol Biotechnol. (2013) 29:907–13. doi: 10.1007/s11274-012-1247-3
156. Verma, SK, Jain, S, and Kumar, S. Immunogenicity and protective potential of a bacterially expressed recombinant dihydrolipoamide succinyltransferase (rE2o) of Brucella abortus in BALB/c mice. World J Microbiol Biotechnol. (2012) 28:2487–95. doi: 10.1007/s11274-012-1056-8
157. Delpino, MV, Estein, SM, Fossati, CA, Baldi, PC, and Cassataro, J. Vaccination with Brucella recombinant DnaK and SurA proteins induces protection against Brucella abortus infection in BALB/c mice. Vaccine. (2007) 25:6721–9. doi: 10.1016/j.vaccine.2007.07.002
158. Tadepalli, G, Singh, AK, Balakrishna, K, Murali, HS, and Batra, HV. Immunogenicity and protective efficacy of Brucella abortus recombinant protein cocktail (rOmp19+rP39) against B. abortus 544 and B. melitensis 16M infection in murine model. Mol Immunol. (2016) 71:34–41. doi: 10.1016/j.molimm.2016.01.001
159. Tadepalli, G, Konduru, B, Murali, HS, and Batra, HV. Intraperitoneal administration of a novel chimeric immunogen (rOP) elicits IFN-γ and IL-12p70 protective immune response in BALB/c mice against virulent Brucella. Immunol Lett. (2017) 192:79–87. doi: 10.1016/j.imlet.2017.10.013
160. Paul, S, Peddayelachagiri, BV, Nagaraj, S, Kingston, JJ, and Batra, HV. Recombinant outer membrane protein 25c from Brucella abortus induces Th1 and Th2 mediated protection against Brucella abortus infection in mouse model. Mol Immunol. (2018) 99:9–18. doi: 10.1016/j.molimm.2018.04.002
161. Hop, HT, Arayan, LT, Huy, TXN, Reyes, AWB, Min, WG, Lee, HJ, et al. Immunization of BALB/c mice with a combination of four recombinant Brucella abortus proteins, AspC, Dps, InpB and Ndk, confers a marked protection against a virulent strain of Brucella abortus. Vaccine. (2018) 36:3027–33. doi: 10.1016/j.vaccine.2018.04.019
162. Nazifi, N, Tahmoorespur, M, Sekhavati, MH, Haghparast, A, and Behroozikhah, AM. In vivo immunogenicity assessment and vaccine efficacy evaluation of a chimeric tandem repeat of epitopic region of OMP31 antigen fused to interleukin 2 (IL-2) against Brucella melitensis in BALB/c mice. BMC Vet Res. (2019) 15:402. doi: 10.1186/s12917-019-2074-7
163. Tian, M, Song, M, Yin, Y, et al. Characterization of the main immunogenic proteins in Brucella infection for their application in diagnosis of brucellosis. Comp Immunol Microbiol Infect Dis. (2020). doi: 10.1016/j.cimid.2020.101462
164. Clapp, B, Walters, N, Thornburg, T, Hoyt, T, Yang, X, and Pascual, DW. DNA vaccination of bison to brucellar antigens elicits elevated antibody and IFN-γ responses. J Wildl Dis. (2011) 47:501–10. doi: 10.7589/0090-3558-47.3.501
165. Gómez, L, Llanos, J, Escalona, E, Sáez, D, Álvarez, F, Molina, R, et al. Multivalent fusion DNA vaccine against Brucella abortus. Biomed Res Int. (2017). doi: 10.1155/2017/6535479
166. Gómez, L, Alvarez, F, Betancur, D, and Oñate, A. Brucellosis vaccines based on the open reading frames from genomic island 3 of Brucella abortus. Vaccine. (2018) 36:2928–36. doi: 10.1016/j.vaccine.2018.04.014
167. Sislema-Egas, F, Céspedes, S, Fernández, P, Retamal-Díaz, A, Sáez, D, and Oñate, A. Evaluation of protective effect of DNA vaccines encoding the BAB1_0263 and BAB1_0278 open reading frames of Brucella abortus in BALB/c mice. Vaccine. (2012) 30:7286–91. doi: 10.1016/j.vaccine.2012.09.039
168. Isore, D, Goswami, T, Chaudhury, P, Mukhopadhayay, S, and Ganguly, S. Recombinant protein and DNA vaccine construct of Brucella abortus L7/L12 gene elicits immune response. Asian Pacific J Health Sci. (2014) 1:425–37. doi: 10.21276/apjhs.2014.1.4.20
169. Hu, XD, Yu, DH, Chen, ST, Li, SX, and Cai, H. A combined DNA vaccine provides protective immunity against mycobacterium bovis and brucella abortus in cattle. DNA Cell Biol. (2009). doi: 10.1089/dna.2008.0790
170. Yu, DH, Li, M, Hu, XD, and Cai, H. A combined DNA vaccine enhances protective immunity against Mycobacterium tuberculosis and Brucella abortus in the presence of an IL-12 expression vector. Vaccine. (2007) 25:6744–54. doi: 10.1016/j.vaccine.2007.06.061
171. Luo, D, Ni, B, Li, P, Shi, W, Zhang, S, Han, Y, et al. Protective immunity elicited by a divalent DNA vaccine encoding both the L7/L12 and Omp16 genes of Brucella abortus in BALB/c mice. Infect Immun. (2006) 74:2734–41. doi: 10.1128/IAI.74.5.2734-2741.2006
172. He, C-Y, Zhang, Y-Z, Liu, M-Z, Zhao, H-L, Ren, L-S, Liu, B-S, et al. Combined immunization with inactivated vaccine reduces the dose of live B. abortus A19 vaccine. BMC Vet Res. (2022, 2022) 18:1–8.
173. He, C, Yang, J, Zhao, H, Liu, M, Wu, D, Liu, B, et al. Vaccination with a Brucella ghost developed through a double inactivation strategy provides protection in Guinea pigs and cattle. Microb Pathog. (2022). doi: 10.1016/j.micpath.2021.105363
174. Manafe, RP, Bhembe-Magadaza, NL, and Green, E. Antibiogram screening and detection of virulence-associated genes in Brucella species acquired from cattle in South Africa’s eastern Cape Province. Int J Environ Res Public Health. (2022) 19:2813.
175. Deng, X, He, J, Wang, Y, Yang, Q, Yi, J, Zhang, H, et al. Deletion of the type IV secretion system promoter VirB in Brucella abortus A19 strain attenuated the virulence of the bacteria and promotes autophagy. Can J Microbiol. (2021) 68:165–76. doi: 10.1139/cjm-2021-0053
176. Yang, X, Clapp, B, Thornburg, T, Hoffman, C, and Pascual, DW. Vaccination with a ΔnorD ΔznuA Brucella abortus mutant confers potent protection against virulent challenge. Vaccine. (2016) 34:5290–7. doi: 10.1016/j.vaccine.2016.09.004
177. Ugalde, JE, Comerci, DJ, Leguizamón, MS, and Ugalde, RA. Evaluation of Brucella abortus phosphoglucomutase (pgm) mutant as a new live rough-phenotype vaccine. Infect Immun. (2003) 71:6264–9. doi: 10.1128/IAI.71.11.6264-6269.2003
178. Li, ZQ, Zhang, JL, Xi, L, Yang, GL, Wang, SL, Zhang, XG, et al. Deletion of the transcriptional regulator GntR down regulated the expression of genes related to virulence and conferred protection against wild-type Brucella challenge in BALB/c mice. Mol Immunol. (2017) 92:99–105. doi: 10.1016/j.molimm.2017.10.011
179. Li, Z, Wang, S, Zhang, J, et al. Brucella abortus 2308ΔNodVΔNodW double-mutant is highly attenuated and confers protection against wild-type challenge in BALB/c mice. Microb Pathog. (2017) 106:30–9. doi: 10.1016/j.micpath.2017.01.043
180. Yang, X, Thornburg, T, Walters, N, and Pascual, DW. DeltaznuADeltapurE Brucella abortus 2308 mutant as a live vaccine candidate. Vaccine. (2010) 28:1069–74. doi: 10.1016/j.vaccine.2009.10.113
181. Briones, G, Iñón de Iannino, N, Roset, M, Vigliocco, A, Silva Paulo, P, and Ugalde, RA. Brucella abortus cyclic beta-1,2-glucan mutants have reduced virulence in mice and are defective in intracellular replication in HeLa cells. Infect Immun. (2001) 69:4528–35. doi: 10.1128/IAI.69.7.4528-4535.2001
182. Arenas-Gamboa, AM, Ficht, TA, Kahl-McDonagh, MM, Gomez, G, and Rice-Ficht, AC. The Brucella abortus S19 DeltavjbR live vaccine candidate is safer than S19 and confers protection against wild-type challenge in BALB/c mice when delivered in a sustained-release vehicle. Infect Immun. (2009) 77:877–84. doi: 10.1128/IAI.01017-08
183. de Souza Filho, JA, Martins, VP, Campos, PC, Alves-Silva, J, Santos, NV, de Oliveira, FS, et al. Mutant Brucella abortus membrane fusogenic protein induces protection against challenge infection in mice. Infect Immun. (2015) 83:1458–64. doi: 10.1128/IAI.02790-14
184. Lacerda, TLS, Cardoso, PG, Augusto de Almeida, L, Camargo, ILBC, Afonso, DAF, Trant, CC, et al. Inactivation of formyltransferase (wbkC) gene generates a Brucella abortus rough strain that is attenuated in macrophages and in mice. Vaccine. (2010) 28:5627–34. doi: 10.1016/j.vaccine.2010.06.023
185. Reyes, AWB, Simborio, HLT, Hop, HT, Arayan, LT, Huy, TXN, Min, W, et al. The two highly immunogenic antigens of Brucella: lipopolysaccharide (LPS) and outer membrane proteins (OMPs). J Prev Vet Med. (2015) 39:198–206. doi: 10.13041/jpvm.2015.39.4.198
186. Conde-álvarez, R, Arce-Gorvel, V, Gil-Ramírez, Y, Iriarte, M, Grilló, MJ, Gorvel, JP, et al. Lipopolysaccharide as a target for brucellosis vaccine design. Microb Pathog. (2013) 58:29–34. doi: 10.1016/j.micpath.2012.11.011
187. Truong, QL, Cho, Y, Kim, K, Park, BK, and Hahn, TW. Booster vaccination with safe, modified, live-attenuated mutants of Brucella abortus strain RB51 vaccine confers protective immunity against virulent strains of B. abortus and Brucella canis in BALB/c mice. Microbiology. (2015) 161:2137–48. doi: 10.1099/mic.0.000170
188. Truong, QL, Cho, Y, Park, S, Kim, K, and Hahn, TW. Brucella abortus ΔcydCΔcydD and ΔcydCΔpurD double-mutants are highly attenuated and confer long-term protective immunity against virulent Brucella abortus. Vaccine. (2016) 34:237–44. doi: 10.1016/j.vaccine.2015.11.030
189. Truong, QL, Cho, Y, Barate, AK, Kim, S, and Hahn, TW. Characterization and protective property of Brucella abortus cydC and looP mutants. Clin Vaccine Immunol. (2014) 21:1573–80. doi: 10.1128/CVI.00164-14
190. Wang, S, Wang, W, Sun, K, Bateer, H, and Zhao, X. Comparative genomic analysis between newly sequenced Brucella abortus vaccine strain A19 and another Brucella abortus vaccine S19. Genomics. (2020) 112:1444–53. doi: 10.1016/j.ygeno.2019.08.015
191. Mena-Bueno, S, Poveda-Urkixo, I, Irazoki, O, Palacios, L, Cava, F, Zabalza-Baranguá, A, et al. Brucella melitensis Wzm/Wzt system: changes in the bacterial envelope Lead to improved Rev1Δwzm vaccine properties. Front Microbiol. (2022). doi: 10.3389/fmicb.2022.908495
192. Al-Mariri, A, Mahmoud, NH, and Hammoud, R. Efficacy evaluation of live Escherichia coli expression Brucella P39 protein combined with CpG oligodeoxynucleotides vaccine against Brucella melitensis 16M, in BALB/c mice. Biologicals. (2012) 40:140–5. doi: 10.1016/j.biologicals.2012.01.002
193. Kim, WK, Moon, JY, Cho, JS, and Hur, J. Protective efficacy by various doses of a new brucellosis vaccine candidate based on Salmonella strains expressing Brucella abortus BSCP31, Omp3b and superoxide dismutase against brucellosis in murine model. Pathog Dis. (2017) 75:94. doi: 10.1093/femspd/ftx094
194. Senevirathne, A, Hewawaduge, C, and Lee, JH. Live vaccine consisting of attenuated Salmonella secreting and delivering Brucella ribosomal protein L7/L12 induces humoral and cellular immune responses and protects mice against virulent Brucella abortus 544 challenge. Vet Res. (2020) 51:1–10. doi: 10.1186/s13567-020-0735-y
195. Zhao, Z, Li, M, Luo, D, Xing, L, Wu, S, Duan, Y, et al. Protection of mice from Brucella infection by immunization with attenuated Salmonella enterica serovar typhimurium expressing a L7/L12 and BLS fusion antigen of Brucella. Vaccine. (2009) 27:5214–9. doi: 10.1016/j.vaccine.2009.06.075
196. Leya, M, Kim, WK, Cho, JS, et al. Vaccination of goats with a combination Salmonella vector expressing four Brucella antigens (BLS, PrpA, Omp19, and SOD) confers protection against Brucella abortus infection. J Vet Sci. (2018) 19:643–52. doi: 10.4142/jvs.2018.19.5.643
197. Tabynov, K, Ryskeldinova, S, and Sansyzbay, A. An influenza viral vector Brucella abortus vaccine induces good cross-protection against Brucella melitensis infection in pregnant heifers. Vaccine. (2015) 33:3619–23. doi: 10.1016/j.vaccine.2015.06.045
198. Lin, GZ, Yang, JT, Wei, SC, Chen, SE, Huo, SD, and Ma, ZR. Immunogenicity of adenovirus and DNA vaccines co-expressing P39 and lumazine synthase proteins of Brucella abortus in BALB/c mice. Trop Anim Health Prod. (2018) 50:957–63. doi: 10.1007/s11250-018-1517-7
199. Lalsiamthara, J, and Lee, JH. Brucella lipopolysaccharide reinforced Salmonella delivering Brucella immunogens protects mice against virulent challenge. Vet Microbiol. (2017) 205:84–91. doi: 10.1016/j.vetmic.2017.05.012
200. Tabynov, K, Sansyzbay, A, Kydyrbayev, Z, Yespembetov, B, Ryskeldinova, S, Zinina, N, et al. Influenza viral vectors expressing the Brucella OMP16 or L7/L12 proteins as vaccines against B. abortus infection. Virol J. (2014) 11:11-69. doi: 10.1186/1743-422X-11-69
201. Tabynov, K, Yespembetov, B, Ryskeldinova, S, Zinina, N, Kydyrbayev, Z, Kozhamkulov, Y, et al. Prime-booster vaccination of cattle with an influenza viral vector Brucella abortus vaccine induces a long-term protective immune response against Brucella abortus infection. Vaccine. (2016) 34:438–44. doi: 10.1016/j.vaccine.2015.12.028
202. Tabynov, K, Kydyrbayev, Z, Ryskeldinova, S, Yespembetov, B, Zinina, N, Assanzhanova, N, et al. Novel influenza virus vectors expressing Brucella L7/L12 or Omp16 proteins in cattle induced a strong T-cell immune response, as well as high protectiveness against B. abortus infection. Vaccine. (2014) 32:2034–41. doi: 10.1016/j.vaccine.2014.02.058
203. Mailybayeva, A, Yespembetov, B, Ryskeldinova, S, Zinina, N, Sansyzbay, A, Renukaradhya, GJ, et al. Improved influenza viral vector based Brucella abortus vaccine induces robust B and T-cell responses and protection against Brucella melitensis infection in pregnant sheep and goats. PLoS One. (2017). doi: 10.1371/journal.pone.0186484
204. Wareth, G, Pletz, MW, Neubauer, H, and Murugaiyan, J. Proteomics of brucella: technologies and their applications for basic research and medical microbiology. Microorganisms. (2020). doi: 10.3390/microorganisms8050766
205. Paci, V, Krasteva, I, Orsini, M, Di Febo, T, Luciani, M, Perletta, F, et al. Proteomic analysis of Brucella melitensis and Brucella ovis for identification of virulence factor using bioinformatics approachs. Mol Cell Probes. (2020). doi: 10.1016/j.mcp.2020.101581
206. Socorro Ruiz-Palma M,, Avila-Calderón, ED, Aguilera-Arreola, MG, López-Merino, A, Ruiz, EA, Morales-García, MR, et al. Comparative proteomic analysis of outer membrane vesicles from Brucella suis, Brucella ovis, Brucella canis and Brucella neotomae. Arch Microbiol. (2021) 203:1611–26. doi: 10.1007/s00203-020-02170-w
207. Tilocca, B, Soggiu, A, Greco, V, Sacchini, F, Garofolo, G, Paci, V, et al. Comparative proteomics of Brucella melitensis is a useful toolbox for developing prophylactic interventions in a one-health context. One Health. (2021) 13:100253. doi: 10.1016/j.onehlt.2021.100253
208. Hamidi, H, Bagheri Nejad, R, Es-Haghi, A, and Ghassempour, A. A combination of MALDI-TOF MS proteomics and species-unique biomarkers’ discovery for rapid screening of brucellosis. J Am Soc Mass Spectrom. (2022). doi: 10.1021/jasms.2c00110
209. Christoforidou, S, Kyritsi, M, Boukouvala, E, Ekateriniadou, L, Zdragas, A, Samouris, G, et al. Identification of Brucella spp. isolates and discrimination from the vaccine strain rev.1 by MALDI-TOF mass spectrometry. Mol Cell Probes. (2020) 51:101533. doi: 10.1016/j.mcp.2020.101533
210. Tang, T, Chen, G, Guo, A, Xu, Y, Zhao, L, Wang, M, et al. Comparative proteomic and genomic analyses of Brucella abortus biofilm and planktonic cells. Mol Med Rep. (2020) 21:731. doi: 10.3892/mmr.2019.10888
211. Kornspan, D, Brendebach, H, Hofreuter, D, Mathur, S, Blum, SE, Fleker, M, et al. Protein biomarker identification for the discrimination of Brucella melitensis field isolates from the Brucella melitensis Rev.1 vaccine strain by MALDI-TOF MS. Front Microbiol. (2021) 12:3132. doi: 10.3389/fmicb.2021.712601
212. Elbehiry, A, Aldubaib, M, Al, RO, Marzouk, E, Abaalkhail, M, Moussa, I, et al. Proteomics-based screening and antibiotic resistance assessment of clinical and sub-clinical Brucella species: an evolution of brucellosis infection control. PLoS One. (2022). doi: 10.1371/journal.pone.0262551
213. Mahmud, A, Khan, MT, and Iqbal, A. Identification of novel drug targets for humans and potential vaccine targets for cattle by subtractive genomic analysis of Brucella abortus strain 2308. Microb Pathog. (2019). doi: 10.1016/j.micpath.2019.103731
214. Wareth, G, Eravci, M, Weise, C, Roesler, U, Melzer, F, Sprague, LD, et al. Comprehensive identification of immunodominant proteins of Brucella abortus and Brucella melitensis using antibodies in the sera from naturally infected hosts. Int J Mol Sci. (2016) 17:659. doi: 10.3390/ijms17050659
215. Zai, X, Yang, Q, Yin, Y, Li, R, Qian, M, Zhao, T, et al. Relative quantitative proteomic analysis of Brucella abortus reveals metabolic adaptation to multiple environmental stresses. Front Microbiol. (2017) 8:2347. doi: 10.3389/fmicb.2017.02347
216. Husain, AA, Pinto, SM, Subbannayya, Y, Kapoor, S, Khulkhule, P, Bhartiya, N, et al. Development of multiple reaction monitoring (MRM) assays to identify Brucella abortus proteins in the serum of humans and livestock. PROTEOMICS – Clin Appl. (2022) :2200009.
217. Moxon, R, Reche, PA, and Rappuoli, R. Editorial: Reverse Vaccinology. Front Immunol. (2019). doi: 10.3389/fimmu.2019.02776
218. Hisham, Y, and Ashhab, Y. Identification of cross-protective potential antigens against pathogenic Brucella spp. through combining Pan-genome analysis with reverse vaccinology. J Immunol Res. (2018). doi: 10.1155/2018/1474517
219. Zai, X, Yin, Y, Guo, F, Yang, Q, Li, R, Li, Y, et al. Screening of potential vaccine candidates against pathogenic Brucella spp. using compositive reverse vaccinology. Vet Res. (2021). doi: 10.1186/s13567-021-00939-5
220. Vishnu, US, Sankarasubramanian, J, Gunasekaran, P, and Rajendhran, J. Novel vaccine candidates against Brucella melitensis identified through reverse vaccinology approach. OMICS. (2015) 19:722–9. doi: 10.1089/omi.2015.0105
221. Aslam, M, Shehroz, M, Hizbullah, SM, Khan, MA, Afridi, SG, and Khan, A. Potential druggable proteins and chimeric vaccine construct prioritization against Brucella melitensis from species core genome data. Genomics. (2020) 112:1734–45. doi: 10.1016/j.ygeno.2019.10.009
222. Oliveira, KC, Brancaglion, GA, Santos, NCM, Araújo, LP, Novaes, E, Santos, RL, et al. Epitope-based vaccine of a Brucella abortus putative small RNA target induces protection and less tissue damage in mice. Front Immunol. (2021) 12:778475. doi: 10.3389/fimmu.2021.778475
223. Li, M, Zhu, Y, Niu, C, Xie, X, Haimiti, G, Guo, W, et al. Zhang F (2022) design of a multi-epitope vaccine candidate against Brucella melitensis. Sci Rep. (2022) 12:1–18. doi: 10.1038/s41598-022-14427-z
224. Yi, J, Wang, Y, Zhang, H, Deng, X, Xi, J, Li, H, et al. Interferon-inducible transmembrane protein 3-containing exosome as a new carrier for the cell-to-cell transmission of anti-Brucella activity. Front Vet Sci. (2021). doi: 10.3389/fvets.2021.642968
225. Ali Hussei, H, Hassan Moh, R, Mohammed, AA, Mahmoud Ab, E, Salah Moha, R, Abd El-Nas, A, et al. Diagnosis of brucellosis in recently aborted ewes using serological tests and polymerase chain reaction. J Appl Sci. (2019). doi: 10.3923/jas.2019.77.81
226. Moeini-Zanjani, A, Pournajaf, A, Ferdosi-Shahandashti, E, Gholami, M, Masjedian, F, Khafri, S, et al. Comparison of loop-mediated isothermal amplification and conventional PCR tests for diagnosis of common Brucella species. BMC Res Notes. (2020). doi: 10.1186/s13104-020-05377-8
227. Yang, X, Wang, Y, Liu, Y, Huang, J, Tan, Q, Ying, X, et al. A label-based polymer nanoparticles biosensor combined with loop-mediated isothermal amplification for rapid, sensitive, and highly specific identification of Brucella abortus. Front Bioeng Biotechnol. (2021) 9:1111. doi: 10.3389/fbioe.2021.758564
228. Ge, L, Wang, D, Lian, F, Zhao, J, Wang, Y, Zhao, Y, et al. Lateral flow immunoassay for visible detection of human brucellosis based on blue silica nanoparticles. Front Vet Sci. (2021) 8:1396. doi: 10.3389/fvets.2021.771341
229. Li, S, Liu, Y, Wang, Y, Wang, M, Liu, C, and Wang, Y. Rapid detection of Brucella spp. and elimination of carryover using multiple cross displacement amplification coupled with nanoparticles-based lateral flow biosensor. Front Cell Infect Microbiol. (2019) 9:78. doi: 10.3389/FCIMB.2019.00078
230. Maan, MK, Sattar, A, Mi, K, Bakr Shabbir, MA, Xie, S, Xin, L, et al. Integration of PK/PD for dose optimization of aditoprim against Trueperella pyogenes causing endometritis in bovines. Microb Pathog. (2020) 142:104097. doi: 10.1016/j.micpath.2020.104097
231. Zhou, K, Huo, M, Ma, W, Mi, K, Xu, X, Algharib, SA, et al. Application of a physiologically based pharmacokinetic model to develop a veterinary amorphous enrofloxacin solid dispersion. Pharmaceutics. (2021). doi: 10.3390/pharmaceutics13050602
232. Zhou, K, Mi, K, Ma, W, Xu, X, Huo, M, Algharib, SA, et al. Application of physiologically based pharmacokinetic models to promote the development of veterinary drugs with high efficacy and safety. J Vet Pharmacol Ther. (2021) 44:663–78. doi: 10.1111/jvp.12976
233. Prakash, C, Kumar, B, Singh, RP, et al. Development and evaluation of a gold nanoparticle based lateral flow assay (LFA) strip test for detection of Brucella spp. J Microbiol Methods. (2021). doi: 10.1016/j.mimet.2021.106185
234. Pal, D, Boby, N, Kumar, S, Kaur, G, Ali, SA, Reboud, J, et al. Visual detection of Brucella in bovine biological samples using DNA-activated gold nanoparticles. PLoS One. (2017). doi: 10.1371/journal.pone.0180919
235. Vakili, S, Samare-Najaf, M, Dehghanian, A, Tajbakhsh, A, Askari, H, Tabrizi, R, et al. Gold nanobiosensor based on the localized surface plasmon resonance is able to diagnose human brucellosis, introducing a rapid and affordable method. Nanoscale Res Lett. (2021) 16:1–11. doi: 10.1186/s11671-021-03600-4
236. El-ghazali, HM, Mohammed, S, Ammar, S, Mohamed, SKA, and Ibrahim, SME. Ultrasonographic radiographic and computed tomography scan. Int J Vet Sci. (2022) 11:28–36. doi: 10.47278/journal.ijvs/2021.072
237. Ahangari, A, Mahmoodi, P, and Mohammadzadeh, A. Biosensors functionalized with nanoparticles for rapid detection of Brucella. Microchem J. (2022) 181:107697. doi: 10.1016/j.microc.2022.107697
238. Narmani, A, Kamali, M, Kooshki, H, Amini, B, Amini, A, and Hasani, L (2018). Highly sensitive and accurate detection of Vibrio cholera O1 OmpW gene by fluorescence DNA biosensor based on gold and magnetic nanoparticles.
239. Sun, Q, Zhao, G, and Dou, W. An optical and rapid sandwich immunoassay method for detection of Salmonella pullorum and Salmonella gallinarum based on immune blue silica nanoparticles and magnetic nanoparticles. Sens Act B Chem C. (2016) 226:69–75. doi: 10.1016/j.snb.2015.11.117
240. Abdel Rahman, AN, Shakweer, MS, Algharib, SA, Abdelaty, AI, Kamel, S, Ismail, TA, et al. Silica nanoparticles acute toxicity alters ethology, neuro-stress indices, and physiological status of African catfish (Clarias gariepinus). Aquac Rep. (2022) 23:101034. doi: 10.1016/j.aqrep.2022.101034
241. Taheri, H, Amini, B, Kamali, M, Asadi, M, and Naderlou, E. Functionalization of anti-Brucella antibody based on SNP and MNP nanoparticles for visual and spectrophotometric detection of Brucella. Spectrochim Acta A Mol Biomol Spectrosc. (2020). doi: 10.1016/j.saa.2019.117891
242. Elbehiry, A, Aldubaib, M, Al, RO, Marzouk, E, Moussa, I, El-Husseiny, M, et al. Brucella species-induced brucellosis: antimicrobial effects, potential resistance and toxicity of silver and gold nanosized particles. PLoS One. (2022) 17:e0269963. doi: 10.1371/journal.pone.0269963
243. Shams, A, Zarif, BR, Salouti, M, Shapouri, R, and Mirzaii, S. Designing an immunosensor for detection of Brucella abortus based on coloured silica nanoparticles. Artif Cells Nanomed Biotechnol. (2019) 47:2562–8. doi: 10.1080/2169140120191626403
244. Bayramoglu, G, Ozalp, VC, Oztekin, M, and Arica, MY. Rapid and label-free detection of Brucella melitensis in milk and milk products using an aptasensor. Talanta. (2019) 200:263–71. doi: 10.1016/j.talanta.2019.03.048
245. Wu, H, Zuo, Y, Cui, C, Yang, W, Ma, H, and Wang, X. Rapid quantitative detection of Brucella melitensis by a label-free impedance immunosensor based on a gold nanoparticle-modified screen-printed carbon electrode. Sensors (Switzerland). (2013) 13:8551–63. doi: 10.3390/s130708551
246. Wahab, R, Khan, ST, Ahmad, J, Musarrat, J, and Al-Khedhairy, AA. Functionalization of anti-Brucella antibody on ZnO-NPs and their deposition on aluminum sheet towards developing a sensor for the detection of Brucella. Vacuum. (2017) 146:592–8. doi: 10.1016/j.vacuum.2017.01.019
247. Kumar, A, Gupta, VK, Rahal, A, Mandil, R, Verma, AK, and Yadav, SK. Nanoparticle based Brucella melitensis vaccine induced oxidative stress acts in synergism to immune response. Indian J Anim Res. (2019) 53:648–54.
248. Shaker, DS, Shaker, MA, and Hanafy, MS. Cellular uptake, cytotoxicity and in-vivo evaluation of Tamoxifen citrate loaded niosomes. Int J Pharm. (2015) 493:285–94. doi: 10.1016/j.ijpharm.2015.07.041
249. Yousefi-Nooraie, R, Mortaz-Hejri, S, Mehrani, M, and Sadeghipour, P. Antibiotics for treating human brucellosis. Cochrane Database Syst Rev. (2012) 10:CD007179. doi: 10.1002/14651858.CD007179.pub2
250. Khan, AU, Melzer, F, Sayour, AE, et al. Whole-genome sequencing for tracing the genetic diversity of Brucella abortus and Brucella melitensis isolated from livestock in Egypt. Pathogens. (2021). doi: 10.3390/pathogens10060759
251. Karponi, G, Kritas, SK, Papadopoulou, G, Akrioti, EK, Papanikolaou, E, and Petridou, E. Development of a CRISPR/Cas9 system against ruminant animal brucellosis. BMC Vet Res. (2019) 15:1–10. doi: 10.1186/s12917-019-2179-z
252. Khan, S, Akhtar, MU, Khan, S, Javed, F, and Khan, AA. Nanoniosome-encapsulated levoflaxicin as an antibacterial agent against Brucella. J Basic Microbiol. (2020) 60:281–90. doi: 10.1002/jobm.201900454
253. Das, K, and Ram, A. Niosome as a novel drug delivery system a review. Int J Appl Pharm. (2014) 6:1–7.
254. Salem, HF, Kharshoum, RM, El-Ela, FIA, Gamal, AF, and Abdellatif, KRA. Evaluation and optimization of pH-responsive niosomes as a carrier for efficient treatment of breast cancer. Drug Deliv Transl Res. (2018) 8:633–44. doi: 10.1007/s13346-018-0499-3
255. Kassem, MA, Megahed, MA, Abu Elyazid, SK, Abd-Allah, FI, Abdelghany, TM, Al-Abd, AM, et al. Enhancing the therapeutic efficacy of tamoxifen citrate loaded span-based nano-vesicles on human breast adenocarcinoma cells. AAPS PharmSciTech. (2018) 19:1529–43. doi: 10.1208/s12249-018-0962-y
256. Salem, HF, Kharshoum, RM, Gamal, FA, Abo El-Ela, FI, and Abdellatif, KRA. Treatment of breast cancer with engineered novel pH-sensitive triaryl-(Z)-olefin niosomes containing hydrogel: an in vitro and in vivo study. J Liposome Res. (2020) 30:126–35. doi: 10.1080/08982104.2019.1601213
257. Leng, N, Ju, M, Jiang, Y, Guan, D, Liu, J, Chen, W, et al. The therapeutic effect of florfenicol-loaded carboxymethyl chitosan-gelatin shell nanogels against Escherichia coli infection in mice. J Mol Struct. (2022) 1269:133847. doi: 10.1016/j.molstruc.2022.133847
258. Luo, W, Ju, M, Liu, J, Algharib, SA, Dawood, AS, and Xie, S. Intelligent-responsive Enrofloxacin-loaded chitosan oligosaccharide-sodium alginate composite core-shell Nanogels for on-demand release in the intestine. Anim Open Access J MDPI. (2022). doi: 10.3390/ani12192701
259. Abo el-Ela, FI, Hussein, KH, el-Banna, HA, Gamal, A, Rouby, S, Menshawy, AMS, et al. Treatment of brucellosis in Guinea pigs via a combination of engineered novel pH-responsive curcumin Niosome hydrogel and doxycycline-loaded chitosan–sodium alginate nanoparticles: an in vitro and in vivo study. AAPS PharmSciTech. (2020) 21:1–11. doi: 10.1208/s12249-020-01833-7
260. Saxena, HM, and Raj, S. A novel immunotherapy of brucellosis in cows monitored non invasively through a specific biomarker. PLoS Negl Trop Dis. (2018). doi: 10.1371/journal.pntd.0006393
261. Algharib, SA, Dawood, A, and Xie, S. Nanoparticles for treatment of bovine Staphylococcus aureus mastitis. Drug Deliv. (2020) 27:292. doi: 10.1080/10717544.2020.1724209
262. Algharib, SA, Dawood, A, Zhou, K, Chen, D, Li, C, Meng, K, et al. Preparation of chitosan nanoparticles by ionotropic gelation technique: effects of formulation parameters and in vitro characterization. J Mol Struct. (2022) 1252:132129. doi: 10.1016/j.molstruc.2021.132129
263. Algharib, SA, Dawood, A, Zhou, K, Chen, D, Li, C, Meng, K, et al. Designing, structural determination and biological effects of rifaximin loaded chitosan- carboxymethyl chitosan nanogel. Carbohydr Polym. (2020). doi: 10.1016/j.carbpol.2020.116782
264. Lueth, P, Haughney, SL, Binnebose, AM, Mullis, AS, Peroutka-Bigus, N, Narasimhan, B, et al. Nanotherapeutic provides dose sparing and improved antimicrobial activity against Brucella melitensis infections. J Control Release. (2019) 294:288–97. doi: 10.1016/j.jconrel.2018.12.024
265. Mangtani, P, Berry, I, Beauvais, W, Holt, HR, Kulashri, A, Bharti, S, et al. The prevalence and risk factors for human Brucella species infection in a cross-sectional survey of a rural population in Punjab, India. Trans R Soc Trop Med Hyg. (2020) 114:255–63. doi: 10.1093/trstmh/trz133
266. Nyerere, N, Luboobi, LS, Mpeshe, SC, and Shirima, GM. Optimal control strategies for the infectiology of brucellosis. Int J Math Math Sci. (2020). doi: 10.1155/2020/1214391
267. Wareth, G, Melzer, F, Böttcher, D, El-Diasty, M, El-Beskawy, M, Rasheed, N, et al. Molecular typing of isolates obtained from aborted foetuses in Brucella-free Holstein dairy cattle herd after immunisation with Brucella abortus RB51 vaccine in Egypt. Acta Trop. (2016) 164:267–71. doi: 10.1016/j.actatropica.2016.09.019
268. Troupin, C, Ellis, I, Doukouré, B, Camara, A, Keita, M, Kagbadouno, M, et al. Seroprevalence of brucellosis, Q fever and Rift Valley fever in domestic ruminants in Guinea in 2017–2019. BMC Vet Res. (2022) 18:1–11. doi: 10.1186/s12917-022-03159-x
269. Maudlin, I, Eisler, MC, and Welburn, SC. Neglected and endemic zoonoses. Philos Trans R Soc B Biol Sci. (2009) 364:2777–87. doi: 10.1098/rstb.2009.0067
Keywords: emerging Brucella pathogens, contagious disease, prevention and control, Brucella vaccines, genomics and proteomics approaches, future perspectives
Citation: Dawood AS, Elrashedy A, Nayel M, Salama A, Guo A, Zhao G, Algharib SA, Zaghawa A, Zubair M, Elsify A, Mousa W and Luo W (2023) Brucellae as resilient intracellular pathogens: epidemiology, host–pathogen interaction, recent genomics and proteomics approaches, and future perspectives. Front. Vet. Sci. 10:1255239. doi: 10.3389/fvets.2023.1255239
Edited by:
Yasser Mahmmod, Higher Colleges of Technology, United Arab EmiratesReviewed by:
Himani Dhanze, Indian Veterinary Research Institute (IVRI), IndiaAman Ullah Khan, University of Veterinary and Animal Sciences, Pakistan
Copyright © 2023 Dawood, Elrashedy, Nayel, Salama, Guo, Zhao, Algharib, Zaghawa, Zubair, Elsify, Mousa and Luo. This is an open-access article distributed under the terms of the Creative Commons Attribution License (CC BY). The use, distribution or reproduction in other forums is permitted, provided the original author(s) and the copyright owner(s) are credited and that the original publication in this journal is cited, in accordance with accepted academic practice. No use, distribution or reproduction is permitted which does not comply with these terms.
*Correspondence: Wanhe Luo, bHVvd2FuaGUwNzI4QDE2My5jb20=