- 1Department of Pathobiology, Faculty of Veterinary Medicine, Shahid Bahonar University of Kerman, Kerman, Iran
- 2Nuclear Agriculture Research School, Nuclear Science and Technology Research Institute (NSTRI), Karaj, Iran
- 3Department of Biology and Control of Diseases Vector, School of Health, Shiraz University of Medical Sciences, Shiraz, Iran
Cold plasma therapy is a novel approach that has shown significant promise in treating bacterial infections in veterinary medicine. Cold plasma possesses the potential to eliminate various bacteria, including those that are resistant to antibiotics, which renders it a desirable substitute for traditional antibiotics. Furthermore, it can enhance the immune system and facilitate the process of wound healing. However, there are some challenges associated with the use of cold plasma in veterinary medicine, such as achieving consistent and uniform exposure to the affected area, determining optimal treatment conditions, and evaluating the long-term impact on animal health. This paper explores the potential of cold plasma therapy in veterinary medicine for managing bacterial diseases, including respiratory infections, skin infections, and wound infections such as Clostridium botulinum, Clostridium perfringens, Bacillus cereus, and Bacillus subtilis. It also shows the opportunities and challenges associated with its use. In conclusion, the paper highlights the promising potential of utilizing cold plasma in veterinary medicine. However, to gain a comprehensive understanding of its benefits and limitations, further research is required. Future studies should concentrate on refining treatment protocols and assessing the long-term effects of cold plasma therapy on bacterial infections and the overall health of animals.
1. Introduction
In the realm of veterinary medicine, bacterial infections stand as formidable adversaries, casting a long shadow of economic losses and endangering the health of livestock and companion animals alike. This review embarks on a journey to explore innovative strategies in the fight against these infections, with a particular focus on cold plasma (CP) technology. To illuminate the path forward, let us first cast a wider net, acknowledging the diverse landscapes in which CP’s potential can flourish.
Bacterial infections, triggered by a multitude of pathogenic species, afflict animals across the spectrum, from livestock to beloved pets. Among the bacterial protagonists are Campylobacter spp., Salmonella spp., Staphylococcus spp., Streptococcus spp., Pseudomonas aeruginosa, Escherichia coli, Bacillus spp., and Actinobacillus spp. (1). These microbes have etched their presence within the animal kingdom, causing ailments that resonate through various species.
For instance, Campylobacter spp., a group of gram-negative bacteria, orchestrate gastrointestinal turmoil in a wide array of domesticated animals, spanning cattle, chickens, turkeys, pigs, sheep, dogs, and cats (1). Similarly, the shadow of Salmonella spp. looms large, casting its influence over humans and a menagerie of animals, including poultry, pigs, cattle, horses, cats, and dogs (2) Staphylococcus species establish their dominion on skin and mucous membranes, ushering in mastitis among dairy cows, a condition that exacts significant tolls on the dairy industry. Notably, Staphylococcus aureus, E. coli, and various streptococcal species, such as Streptococcus uberis and Streptococcus agalactiae (3), are principal actors in this costly drama (3). Meanwhile, Staphylococcus pseudintermedius orchestrates its narrative, inflicting skin, ear, and tissue infections in cats and dogs (4–6). A concerning twist in this tale is its zoonotic (7, 8) nature, posing a threat to human health (7, 8). Moreover, the disconcerting revelation that approximately 97.8% of methicillin-resistant S. pseudintermedius (MRSP) strains defy multiple antibiotics raises alarms within the veterinary realm (9, 10).
The opportunistic P. aeruginosa, resident on human and mammalian skin and mucous linings, casts its influence on pets like dogs and cats. It paints a canvas of diverse afflictions, ranging from skin and systemic infections (11) to ulcers, papules (12), ear infections (13), eye inflammations (14), sinus (15) issues, dental abscesses (16), and gum diseases (17).
In the case of E. coli, typically a benign member of animal microbiota, certain strains wield the power to unleash severe illnesses. Among them, enterotoxigenic E. coli (ETEC) infection emerges as the prevalent face of colibacillosis, primarily affecting young animals, notably pigs and calves (18) Bacillus subtilis, residing within the Bacillus genus, is implicated in food poisoning and associated with bovine mastitis and ovine abortion (19). Lastly, Actinobacillus species, gram-negative bacteria, stand accused of inciting diverse animal diseases (20).
In the face of these bacterial adversaries, veterinary medicine has long relied on the administration of broad-spectrum antibiotics. These antimicrobial agents, ubiquitous in procedures such as umbilical cord cutting, tail docking, vaccination courses, and castration, seek to mitigate the risk of infection by confronting stress and potential pathogens (21) in animals. However, a shadow looms over this practice in the form of antimicrobial resistance (AMR).
AMR, a global crisis recognized by the World Health Organization, extends its reach not only to human health but also to animals, domestic and wild, and the environment (22). The misuse and overuse of antimicrobial drugs in animal feed facilitate the emergence and dissemination of resistant bacterial strains (23, 24). This alarming trend has birthed a new era, characterized by the ascent of multi-drug resistant (MDR) pathogens and the prevalence of biofilms in food sources (22).
The battlefront of AMR primarily revolves around gram-negative pathogens and their production of β-lactamases, undermining the efficacy of β-lactam antibiotics, essential for treating both human and animal infections (25). Furthermore, microbial communities, known as biofilms, have emerged as a key player in AMR, offering sanctuary to pathogens on various surfaces, living and non-living (26).
As the arsenal of effective antibiotics dwindles, the susceptibility of both animals and humans to infections experiences a resurgence. In the current landscape, the emergence of antibiotic-resistant pathogenic bacteria, such as Klebsiella pneumoniae, Acinetobacter baumannii, and P. aeruginosa, paints a concerning picture (27). This escalating dilemma underscores the need for urgent action. The cunning propensity of these pathogens to form biofilms further compounds the challenge, intensifying concerns about food safety and the proliferation of antibiotic resistance (28). This complex issue demands a comprehensive approach, calling upon the scientific community to seek innovative solutions. In this critical juncture of combating MDR pathogens and biofilms, cold plasma (CP) technology emerges as a beacon of hope (29, 30).
2. Understanding cold plasma: its composition, generation, and applications
2.1. What is the plasma and cold plasma?
Often referred to as the fourth state of matter as described by Fitzpatrick (31), plasma undergoes a series of transformations with escalating energy levels in a substance. Its progression spans from solid to liquid, then to gas, culminating in a distinct ionized gas form as observed by Tabares and Junkar (32). Furthermore, plasma can be categorized based on the circumstances of its production either as low-atmospheric or high-pressure and it can also be classified into thermal or non-thermal plasmas, as discussed by Murphy and Uhrlandt (33). Additionally, non-thermal plasma forms such as CP, cold atmospheric plasma (CAP), and atmospheric pressure plasma (APP) are all linked with low-temperature plasma generation, though they exhibit slight differences in terms of their properties and uses. CP denotes a partially ionized gas with ion and electron temperatures significantly lower than those observed in traditional high-temperature plasmas. This term is often associated with plasmas that function at or near room temperature or moderate temperatures. The designation “cold” does not necessarily imply that the plasma is ice-cold; instead, it conveys that the electrons and ions are not in thermodynamic equilibrium with the gas temperature.
CAP is a specific form of CP that operates at, or close to, room temperature and at atmospheric pressure. This suggests that the plasma is formed under ordinary conditions, simplifying its handling and making it suitable for a variety of uses. CAPs are often non-equilibrium plasmas that contain different reactive species like ions, radicals, and excited molecules, which makes them particularly valuable for purposes such as surface modification, sterilization, and biomedical applications. Also known as non-thermal plasma (NTP), CAP can be generated using a range of electrical discharges. These include corona discharge, micro-hollow cathode discharge, gliding arc discharge, one atmospheric uniform glow discharge, dielectric barrier discharge (DBD), atmospheric pressure plasma jet (APPJ), and plasma needle (34).
Matrix metalloproteases (MMPs) play a critical role in the breakdown of extracellular matrix components, underpinning essential physiological processes such as tissue remodeling, wound healing, inflammation, and immune responses. Given the substantial impact of MMP dysregulation on various animal diseases, including musculoskeletal disorders, skin conditions, and tissue injuries (35) exploring novel interventions becomes imperative. In this context, cold plasma therapy (CAP) emerges as a potential avenue with its unique capacity to generate reactive species and modulate cellular responses. A closer examination reveals CAP’s intriguing implications for veterinary applications, particularly it is potential to influence MMPs. CAP holds promise in diverse veterinary scenarios—accelerating wound healing through tissue remodeling, mitigating skin disorders via anti-inflammatory effects, impacting orthopedic conditions like osteoarthritis, aiding oral tissue regeneration for dental health, offering antimicrobial efficacy against infections, and even influencing aspects of tumor management and equine health, notably wound healing and lameness management (36). This alignment between CAP and MMPs presents a novel avenue for enhancing veterinary therapeutic approaches and warrants further exploration.
Atmospheric pressure plasma (APP) designates any plasma formed at or close to atmospheric pressure, regardless of it being “cold” or “thermal” (high-temperature). Systems utilizing APP operate under standard pressure, thereby removing the requirement for vacuum chambers, which subsequently enhances their utility for numerous industrial applications. In summary, the primary distinctions between various types of plasma are rooted in their operating temperature, pressure conditions, and the reactive species they contain.
2.2. Applications of cold plasma
The method employed to generate CP and the resulting composition of the plasma source plays a significant role in determining its applicability in different fields. In environmental, biological, and biomedical applications, the commonly utilized methods for CP generation are DBD and plasma jet. CP possesses a complex chemical composition, and the inactivation of microbial targets is believed to be facilitated by multiple reactive agents that act either independently or synergistically. Atmospheric air CP, in particular, contains a diverse array of reactive agents, including electrons, positive and negative ions, free radicals, stable conversion products like ozone, excited atoms and molecules, and UV photons (Figure 1) (37). Plasma sources commonly utilized produce a diverse range of reactive species, including electronically and vibrationally excited oxygen and nitrogen molecules, as well as active forms of oxygen molecules and atoms referred to as reactive oxygen species (ROS). These ROS consist of atomic oxygen, singlet oxygen, superoxide anion, and ozone (38). Reactive nitrogen species (RNS), such as atomic nitrogen, excited nitrogen, and nitric oxide, are also generated (39). In the presence of humidity, additional species such as H2O+, OH anion, OH radicals, or H2O2 can be produced. These reactive species, combined with UV radiation and charged particles, contribute to the antimicrobial properties exhibited by plasma. Among these reactive species, ozone, atomic oxygen, singlet oxygen, superoxide, peroxide, and hydroxyl radicals are believed to play a role in the inactivation of bacteria (40). The effectiveness of CP in reducing microbial growth is influenced by various factors, including environmental conditions such as temperature and relative humidity, food properties like moisture content, pH, product composition, surface properties, and surface area/volume ratio, as well as processing parameters including voltage, frequency, gas composition, flow rate, treatment time, electrode type, interelectrode gap, headspace, and exposure pattern time. Additionally, characteristics of the microorganisms themselves, such as type, strain, growth phase, and initial count, also play a role in the efficacy of CP treatment (41).
Low pH levels enhance the efficiency of CP by causing partial denaturation of protein structures and cell leakage. Moreover, the presence of water vapor in the gas phase leads to the formation of H2O2, a potent oxidizer that induces cell death by oxidizing the outer cell structure (42). CP treatment disrupts the structural integrity of microorganisms, ultimately resulting in their demise. The reactive species present in CP interact with different components of the microorganism, contributing to this effect.
Researchers confirm CP efficacy in purging surfaces and instruments from harmful organisms (43, 44). Consequently, CP stands out as a solution to decontaminate livestock environments, countering the health risks these pathogens pose to the animals (45, 46). CP short-term exposure has demonstrated efficacy in neutralizing micro-organisms within sealed spaces like airtight containers and fridges, which indicates its potential in preserving agricultural goods. This system can be applied to the storage to keep agricultural products fresh and exclusion of harmful materials from the products (47). Furthermore, CP has proven successful in neutralizing specific micromycete species and their spores, including Cladosporium sphaerospermum, Aspergillus oryzae, Alternaria species, and Byssochlamys mivea (48, 49). This fungicidal trait further underscores its value in sanitizing livestock habitats. Notably, CP can neutralize endotoxins, lipids, and prions found in micro-organisms (50–52). Additionally, CP enhances water quality on multiple fronts microbiologically, physically, and chemically by neutralizing harmful micro-organisms. Since water quality, directly and indirectly, impacts animal health and output, it is essential to monitor and maintain its quality. This management aspect falls under the infrastructure category in livestock farming (53).
3. Various factors affecting the inactivation efficacy of CP in bacteria
To effectively design and optimize CP devices for applications involving microbial inactivation, it is crucial to have a comprehensive understanding of how various factors influence the antimicrobial efficacy of CP. The key factors that significantly impact CP’s effectiveness include: (i) type of CP device: CP devices can be categorized into three main types: direct CP, indirect CP, and hybrid CP. (ii) Gas composition and flow rate: the composition of the gas used in CP, as well as the flow rate at which it is supplied, plays a critical role in determining its antimicrobial efficacy. (iii) Changes in source frequency, voltage, and power: alterations in the frequency, voltage, and power settings of the CP source can affect its ability to inactivate microorganisms. (iv) Changes in the safe distance for CP treatment: the distance between the CP source and the object being treated can impact the microbial inactivation efficiency. Finding the optimal safe distance is important for effective treatment. (v) CP treatment time: the duration of exposure to CP is an essential factor in determining its antimicrobial effectiveness. Longer treatment times may result in improved inactivation, but there is a need to balance treatment duration with practical considerations. (vi) Initial concentration of microorganisms: the initial concentration of microorganisms present on the treated object can influence the efficacy of CP treatment. Higher initial microbial loads may require longer or more intense CP exposure for effective inactivation. Direct CP involves one of the electrodes being part of the electrical circuit of the treated object, which can increase temperature. Examples of direct CP methods include corona discharge, DBD volume, and spark discharge. Understanding and optimizing these factors will contribute to the development of efficient CP devices for microbial inactivation applications.
Indirect CP involves the transfer of plasma generated between two electrodes to the treated object through diffusion and convection mechanisms. Various studies have noted differences in antimicrobial effectiveness between direct and indirect CP treatments. In the case of S. aureus, the direct CAP treatment exhibited a larger inactive zone compared to the indirect CP treatment (54). Moreover, when treating P. aeruginosa, both N2 CP and direct air CP treatments achieved a 6 log reduction in colony-forming units (CFU) within 60 s. However, in the case of indirect treatment, a 4 log CFU reduction was achieved for N2 CP, and a 5 log CFU reduction was achieved for air CP within the same treatment time (55). Gas plays a crucial role in CP discharge production. Typically, gases like Ar, He, air, N2, O2, or their mixtures are used for igniting plasma discharges in CP devices. Some studies have only focused on the impact of gas flow, revealing no significant effect (54, 56–58).
Limited research has addressed the impact of variations in gas flow rate on the effectiveness of CP in eliminating microbes. When the helium (He) gas flow rate was increased from 10 liters per minute (lpm) to 14 lpm, the survival count decreased from 5 × 108 colony-forming units per milliliter (CFU/mL) to 6 × 102 CFU/mL for E. coli and from 5 × 108 CFU/mL to 7 × 103 CFU/mL for S. aureus. This reduction could be attributed to an elevated concentration of active species within CP due to the increased flow rate. In a study conducted by Das et al. (59), raising the power of the cold atmospheric plasma jet (CAPJ) from 0.15 W to 1.6 W demonstrated a decreased likelihood of methicillin-resistant S. aureus (MRSA) biofilm formation. This outcome can be attributed to a higher flux of chemical species at greater frequencies and power, as suggested by Sharma et al. (60) and Wiegand et al. (61). In terms of the CP microjet, 13.56 MHz radiofrequency exhibited superior E. coli inactivation compared to the lower frequency of 50 kHz. However, similar antimicrobial effects as radiofrequency can be achieved at lower frequencies by increasing the voltage, as indicated by Kim et al. (56).
The distance between the nozzle of the CAPJ and the sample is a crucial factor that impacts the effectiveness of CP in combating microbes. Studies by Sharma et al. (62) and Shouzhe and Jinpyo (63), have shown that increasing the exposure distance leads to a decrease in the antimicrobial effect. For example, when the exposure distance was increased from 0.5 cm to 2 cm in the case of air CAPJ, Pedroni et al. (64) observed a reduction in the log colony-forming unit (CFU) reduction value from 2.5 to approximately 1.2. Therefore, the decline in CP’s antimicrobial activity with an extended exposure interval, as reported by Pedroni et al. (64) and Shouzhe and Jinpyo (63), may be attributed to a decrease in the concentration of active species during CP, as suggested by Lotfy et al. (65).
Additionally, the timing of exposure to CP also plays a significant role. When considering a fixed exposure interval in CAPJ, the duration of CP exposure required to achieve the same log reduction of B. subtilis is shorter in Ar + O2 CP compared to He + O2 CP. Guimin et al. (66) observed that in the case of argon CAPJ, the lethal log volume (KLV) reached 5.38 after 90 s of CP treatment for S. aureus and 5.36 after 60 s of CP treatment for E. coli.
The enhanced effectiveness of CP against microorganisms may be attributed to the interaction between active species within CP and microorganisms. This interaction intensifies with longer exposure times, resulting in a greater lethal impact. Another contributing factor is the chemical activity present in the growth environment, which ultimately influences the size of the inhibition zone. For instance, when the concentration of E. coli was raised from 102 CFU/mL to 105 CFU/mL, the CP treatment time increased from 30 s to 120 s. Similarly, elevating the concentration of S. aureus from 102 CFU/mL to 105 CFU/mL required a longer CP treatment time of 45 s to 90 s. In a study by Deng et al. (67), a 3 log CFU reduction was achieved for B. subtilis exposed to CP for 200 s and 360 s, respectively, when the initial concentrations were 106 CFU/mL and 109 CFU/mL. Hence, increasing the initial concentration of microorganisms necessitates a longer treatment time with CP, as indicated by Lee et al. (68).
4. Effect of cold plasma on bacterial structure
CP has been shown to have various effects on bacteria, ultimately leading to cell destruction (69). The primary impacts encompass damage to the bacterial cell membrane, intracellular protein damage, and direct DNA damage, as highlighted by Niedźwiedź et al. (70). The presence of loaded particles, such as ions and electrons, gives rise to an electrostatic field that permeates the bacterial cell wall, resulting in the breakage of chemical bonds, erosion, and the formation of lesions and openings in the membranes. Additionally, these particles facilitate the entry of plasma toxic compounds into the bacterial cell, intensifying the destructive process, as stated by Olatunde et al. (71). Furthermore, proteins and bacterial DNA are directly affected by oxidative stress, as emphasized by Winter et al. (72). Reactive oxygen radicals induce significant oxidative stress, leading to lipid-oxygenation, loss of cellular cytoplasm and proteins, and oxidative DNA damage (Figure 2). This ultimately culminates in cell death when the repair mechanism becomes overwhelmed. Lastly, Winter et al. (72) illustrated in Figure 1 that before inactivation, cells undergo morphological and chemical changes.
4.1. Response of gram-positive and gram-negative bacteria to cold-plasma treatment
The antimicrobial effectiveness of CP varies depending on the cell wall structure of different microorganisms (59, 73). Due to their thicker peptidoglycan layer, gram-positive bacteria are less susceptible to CP compared to gram-negative bacteria (74). CP treatment causes irreversible wall damage and oxidation of GN cells due to ROS and ultraviolet (UV) radiation attacking the cell envelope, leading to the release of intracellular compounds such as proteins, DNA, and lipids. On the other hand, CP causes severe intracellular damage to GP bacteria without cell leakage. Some studies have suggested that CP is more effective against GN than GP bacteria due to the relatively thinner peptidoglycan cell wall structure of GN bacteria, which is less than 10 nm, compared to 20–80 nm for GP bacteria (73, 75).
Indeed, the susceptibility of microorganisms to CP cannot be generalized solely based on their gram characteristics, as concluded by Kim and Min (76) and Min et al. (77). The research conducted by Mai-Prochnow et al. (78) revealed that bacterial resistance to CP may be influenced by their growth conditions. For instance, P. aeruginosa demonstrated greater resistance to CP when in co-culture compared to a single-species biofilm. Han et al. (79) also observed distinct reactions of different bacterial strains to CP. Specifically, E. coli was inactivated through cell leakage due to damage to the lipopolysaccharides in the outer cell membrane and the thin peptidoglycan layers of gram-negative bacteria. On the other hand, S. aureus, being a gram-positive bacterium with significantly thicker peptidoglycan layers compared to E. coli, experienced damage to intracellular components while maintaining an intact cell wall, as demonstrated by Han et al. (79). In a study conducted by Wan et al. (80), the efficacy of high voltage atmospheric CP (HVACP) in inactivating Salmonella and its impact on egg quality were evaluated. The experiment involved treating inoculated eggs with HVACP (85 kV) in both dry air and a modified atmosphere gas environment. Direct treatment of egg surfaces under the modified atmosphere gas for 15 min resulted in a reduction of 5.53 log CFU/egg, indicating significant inactivation of Salmonella.
Another investigation by Lührmann et al. (81) examined the effect of cold atmospheric pressure argon plasma on the antibiotic sensitivity of methicillin-resistant strains of S. aureus in vitro. The study observed a statistically significant decrease in the growth inhibition zone. For the S. aureus strain SZ 148, the decrease was 6.3 mm with cefuroxime and 14.5 mm with oxacillin. Additionally, for strain 05-01825, there was a decrease of 36.8 mm with oxacillin (81). This indicates that the antibiotic sensitivity of the methicillin-resistant S. aureus strains was affected by cold atmospheric pressure argon plasma.
Numerous studies have been conducted in various countries to investigate the effect of CP on both gram-positive and gram-negative bacteria, as shown in Table 1.
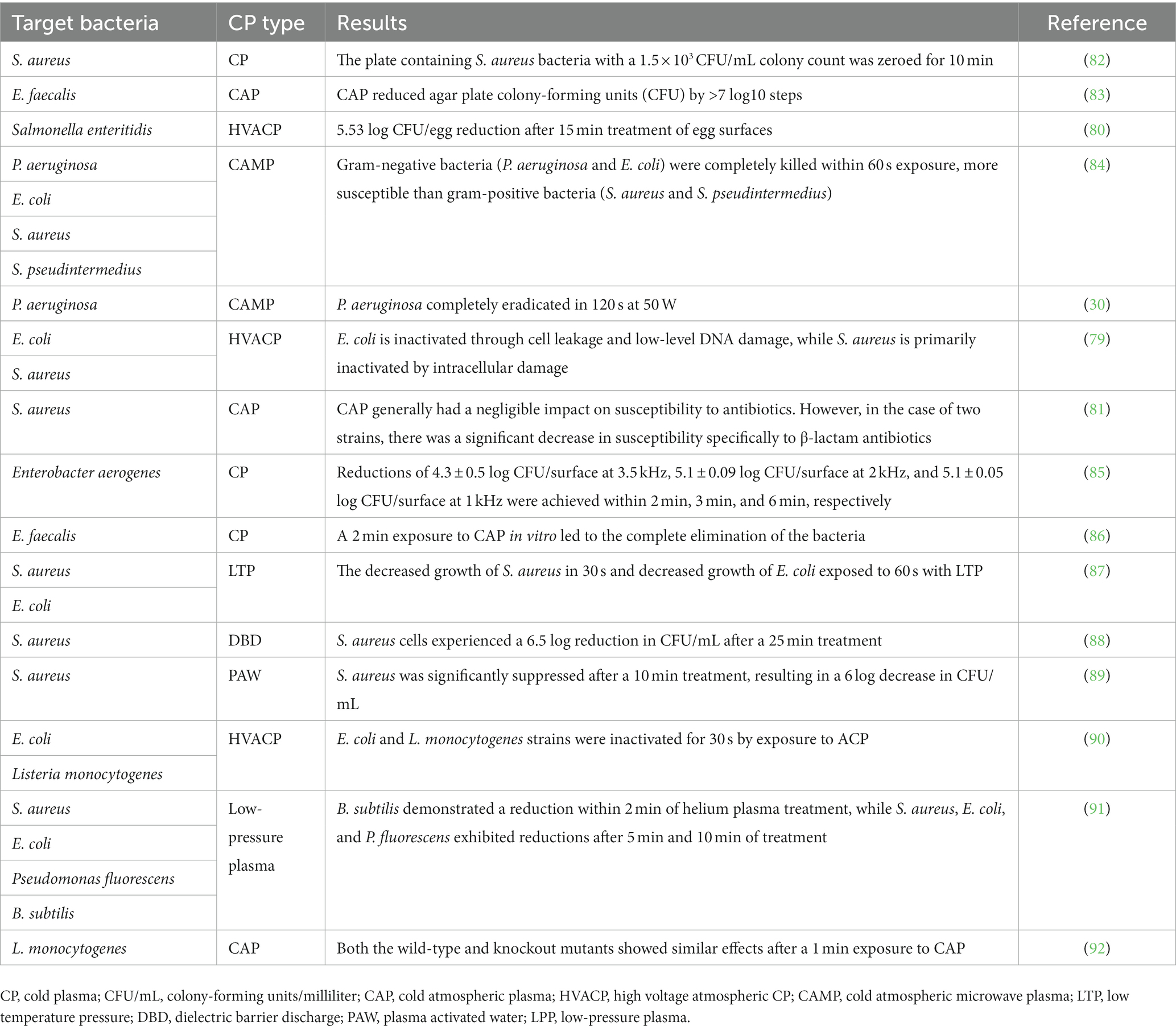
Table 1. Studies related to the effect of cold plasma on gram-positive and gram-negative pathogenic bacteria in animals.
5. Effect of cold plasma on bacterial biofilm and MDR
Biofilms are communities of bacteria that flourish in various environments by attaching to solid surfaces and creating an extracellular matrix comprised of polysaccharides, lipids, proteins, and nucleic acids (93). These biofilms demonstrate enhanced resilience against external challenges such as antibiotics, temperature variations, and pH fluctuations. Consequently, they pose a significant hurdle in the progression of bacterial diseases in animals. Eradicating biofilms, produced by attached bacteria, presents additional complications (94). Although most research has focused on chemical bactericidal agents to combat opportunistic infections, physical treatments like CP (specific treatment) offer an alternative approach when the efficacy of chemical agents is compromised due to the natural resistance of pathogens or biofilms (95). As microorganisms can develop resistance to certain drugs over time through genetic mutation or alterations in gene sequences, the inactivation of drug-resistant microorganisms becomes increasingly challenging compared to non-resistant ones (96). Nevertheless, numerous studies have provided evidence of the effectiveness of CP in deactivating drug-resistant microorganisms, as indicated in Table 2. Furthermore, CP has shown the ability to target biofilms formed by both gram-positive and gram-negative bacteria (74, 95, 105, 110, 111). These biofilms primarily consist of water along with extracellular polysaccharides, proteins, and DNA (112). The efficacy of CP in deactivating multidrug-resistant pathogens and biofilms varies depending on the microorganisms’ structure, composition, and resistance (98, 99). The eradication of MDR superbugs, such as the “ESKAPE” pathogens, poses a major clinical challenge in the 21st century (28). Among these pathogens is Enterococcus faecalis, a gram-positive coccus that forms biofilms, increasing its tolerance and reducing sensitivity to antimicrobial measures (113, 114). In vitro, studies have demonstrated that CP exhibits antibacterial effects against both planktonic cultures and biofilms of E. faecalis (115).
The application of CAP treatment resulted in a significant reduction of 7 log10 steps in colony forming units (CFU) on agar plates. When 24 h E. faecalis biofilms were treated with CAP, a 3 log10-step reduction in CFU was observed after 5 min of treatment. In comparison, the positive controls chlorhexidine (CHX) and UVC exhibited slightly greater effectiveness than CAP in the conducted biofilm experiments. Notably, CAP treatment did not cause damage to the cytoplasmic membrane of E. faecalis, suggesting that membrane disruption is not the primary mechanism of action against this bacterium. Moving on to P. aeruginosa, an opportunistic pathogen that causes purulent inflammation in dogs’ ears and skin, its biofilms are known to contribute to antibiotic resistance and therapeutic limitations. Therefore, the antibacterial and antibiofilm properties of cold atmospheric microwave plasma against P. aeruginosa were studied under laboratory conditions (30). The findings indicated that complete elimination of both the ATCC strain and clinical isolates of P. aeruginosa was achieved within 120 s at 50 W, with the clinical isolates requiring 60 s less than the ATCC strain for complete eradication at the same power level (83). In another study by Cao et al. (101), the effectiveness of NTP (non-thermal plasma) against E. faecalis and S. aureus suspensions on agar plates, as well as E. faecalis biofilm on nitrate membrane filters, was assessed. Following NTP treatment, inhibition zones were observed in agar cultures, and the size of these zones increased with longer treatment durations.
DBD can be effective against MRSA strains that carry the β-lactamase gene and mecA antibiotic resistance. Through DBD, penicillin-binding proteins and regulatory factors that may affect peptidoglycan (PG) are inactivated and result in decreased β-lactamase activity, which may be due to inactivation of the β-lactamase enzyme as well as its associated gene (97). In a study by Napp et al. (98), it was observed that the effect of DBD on MRSA and MSSA is different, which could be due to the stiffer cell wall of MRSA compared to MSSA, as for certain strains by Kawai et al. (116) showed an association between thickened cell wall (MRSA) and resistance to disinfectants in vitro has been documented. Additional research on the impact of CP on bacterial biofilms is presented in Table 2.
6. Effect of cold plasma on bacterial spores
Livestock diseases often involve bacteria such as C. botulinum, C. perfringens, B. cereus, and B. subtilis. These bacterial species have developed various mechanisms to survive harsh environments, including the formation of protective spores (117). CP treatment has emerged as a promising method for inactivating bacterial spores. The primary mechanisms through which CP inactivates spores are as follows: (i) DNA damage caused by emitted UV radiation (118), (ii) erosion of the spore surface, penetration into the cortex, and damage to the spore nucleus, resulting in changes to protein structure, internal lipids, and degradation of dipicolinic acid (119), and (iii) etching of the spore coat through the action of reactive nitrogen species (RNS) (120).
Spores typically possess multi-layered outer structures that serve as the first line of defense against chemicals and physical aggression, contributing to their resistance. The external structures are primarily responsible for the spores’ resilience. Some spore species, such as B. cereus, possess an additional outermost layer called the exosporium, composed of proteins, lipids, and carbohydrates. Studies have shown that these extrinsic structures are targeted by CP for spore inactivation (121–123). For instance, in a study utilizing a helium atmosphere, CP treatment resulted in the complete rupture of B. subtilis spores, with oxidation by active plasma species identified as the primary cause of spore death (124). CP-induced damage to the spore coat leads to the release of dipicolinic acid (DPA) and increased hydration of the spore nucleus, which is a key factor in spore inactivation (125).
Multiple research studies have provided evidence that CP-generated UV photons primarily target the DNA within spores, leading to their inactivation (109, 126). Damage to the intracellular DNA can result in the death of spores. In atmospheric pressure plasma-jet (ACP-PJ), an alternative approach to increasing reactive oxygen species (ROS) production is to raise the excitation voltage, which influences energy and electron density.
In a study by Pina-Perez et al. (120), atmospheric pressure plasma-surface barrier discharge (ACP-SBD) with air as the process gas was investigated for its impact on B. subtilis spores. They achieved approximately 4 log inactivation of B. subtilis spores using a low plasma power density of 5 mW/cm2 and an exposure time of 7 min. Furthermore, they demonstrated that the composition of the matrix material or food substance can influence the efficiency of B. subtilis spore inactivation. Numerous research studies have been conducted worldwide to explore the effects of CP on bacterial spores (Table 2).
7. The effect of cold plasma on skin
The skin serves as a vital immune organ, playing a crucial role in protecting the body against pathogens and physical or chemical threats from the environment (127). Any damage to the integrity of the skin can result in significant impairment or even mortality. CP therapy, an innovative therapeutic approach, has been proposed for the treatment of extensive and chronic wounds. The selective eradication of severely damaged cells and the concurrent promotion of cell proliferation and migration stand as pivotal facets of this method. At the forefront of this technique is its remarkable capacity to generate stress-induced reactive oxygen species (ROS), a mechanism that enables the targeted elimination of compromised cells. Importantly, this selective action is achieved while ensuring the preservation of normal cells from harm. This fundamental capability of CP to eliminate bacteria without inducing injury to host tissue underscores a vital theme in this discussion. While the intricate details of ROS may be reserved for subsequent paragraphs, the overarching concept of CP’s ability to maintain a delicate balance between therapeutic efficacy and tissue well-being deserves prominence from the outset (128–130). In veterinary medicine, effective wound management is crucial to prevent permanent disability and economic burden.
The main components of CAP include ions, electrons, metastable, photons, and electromagnetic fields. After a reaction with environmental air, CAP forms a hierarchical group of reactive oxygen and nitrogen species (RONS) that promote increased skin tissue microcirculation, increased monocyte stimulation, increased cell migration, and stimulation of the keratinocytes and fibroblasts primarily involved in wound healing (131).
The potential influence of cold atmospheric plasma extends to the production of nitric oxide. Current understanding points to the significant part played by NO in healing wounds and regenerating tissues by managing blood vessel contraction and clotting, along with the immune system and early-stage cell death or apoptosis (132). External introduction of NO-donors—molecules that hold, release, or create nitric oxide when they come into contact with tissue—to a wound site can enhance and accelerate the healing process (133, 134). CAP may further contribute to wound recovery via its disinfectant properties, the stimulation of skin cell growth and movement through the activation or suppression of integrin receptors on the cell surface, or its pro-angiogenic effects (135).
A study by Amini et al. (136) showed that CAP treatment can alter the durability of inflammatory cytokines and growth factors, including IL-1, IL-8, TGF-β, TNF-α, and INF-γ, thereby facilitating healing by initiating the proliferative phase at a quicker rate. Another research piece by Arndt et al. (137) revealed that when CAP treatment was analyzed in vitro, it resulted in the heightened expression of crucial genes involved in the wound healing process, such as IL-6, IL-8, MCP-1, TGF-ß1, TGF-ß2. In addition, it boosted the production of collagen type I and alpha-SMA, which play significant roles in tissue repair. According to research conducted by Zhang et al. (138), the application of CP has been found to accelerate wound healing by reducing the healing time, decreasing wound size, and promoting wound contraction and re-epithelialization. Additionally, the study showed a significant increase in the protein level of smooth muscle actin, indicating improved wound healing.
Before the integration of plasma technologies into medical applications, a comprehensive evaluation of their impact on living tissues is imperative. This assessment extends to encompass an exploration of potential toxic effects resulting from plasma exposure. The significance of this pursuit lies not only in its scientific underpinnings but also in its direct implications for clinical practice. Indeed, the determination of safe and efficacious implementation avenues, free from the specter of long-term adverse effects, forms a pivotal cornerstone in advocating for the broader utilization of CP in the realm of veterinary medicine. By harmonizing the scientific and clinical dimensions, this approach resonates with the quest to establish a solid foundation for the expansion of CP’s applications within this critical domain. To evaluate the safety of plasma treatment on both intact and wounded skin, a Yorkshire pig model, which has comparable skin characteristics to humans, was utilized (139). The findings of the study indicated that plasma treatment is safe for both types of skin, even at doses higher than those needed for effective bacterial inactivation in agar or liquid media. Further research has focused on investigating the molecular and physiological consequences of CP treatment on mouse skin (115). The cells in the dermis and epidermis respond to reactive oxygen species (ROS) through redox signaling, which occurs due to environmental stress or disruptions in tissue homeostasis (140). Plasma-derived ROS induces changes in the junctional network, improves tissue oxygenation, oxidizes stratum corneum lipids, and limits the penetration of substances like the model drug curcumin (115).
In a study conducted by Lee et al. (141), the impact of CAP on skin physiological parameters and tolerance in dogs was assessed. The CAP treatment was applied to various points on the dogs’ natural skin in the groin area for durations of 30 s, 1 min, 2 min, and 4 min. Hydration, trans epidermal water loss (TEWL), and surface temperature were measured before and after the CAP treatment, with measurements taken five times, three times, and three times, respectively. The results showed that, except for mild erythema observed in the treatment area after 4 min, the majority of dogs did not exhibit significant pain responses or side effects. In another research study by Li et al. (142), the possible mechanisms of a cold atmospheric plasma jet on a rabbit wound infected with methicillin-resistant S. aureus (MRSA) were investigated in vivo. The researchers found that the treatment could regulate cytokine secretion, limit the inflammatory response and excessive cell proliferation, accelerate re-epithelialization, and ultimately promote the wound healing process while inactivating bacteria. Furthermore, the potential of atmospheric pressure plasma jet therapy for wound healing was studied using a mouse model of ear wounds in research conducted by Schmidt et al. (143). The effectiveness of CP on skin regeneration was explored in this study.
The in vitro experiments investigating the effect of CP on keratinocyte and fibroblast migration, specifically in the context of scratch assays, showed that CP stimulation led to significant stimulation and rapid closure of the gaps. To enhance the astringent effect of CP jets on acute wounds in mice, researchers applied microliters of distilled water to the wounds before treatment. The combined treatment of water and plasma was found to be more effective than plasma treatment alone. The water, when combined with plasma, may chemically and physically modify the wound surface, leading to enhanced wound-healing effects. These findings suggest that the combination of CP and water can potentially have synergistic effects on wound healing processes.
The study by Nakajima et al. (144) demonstrated that the combination of plasma treatment and pouring water had a significant impact on enhancing the strength of myofibroblasts. This suggests a correlation between the reduction of wound size and an increase in the number of myofibroblasts. Furthermore, the combined treatment of plasma and water pouring was more effective in promoting wound contraction compared to plasma treatment alone.
In a study conducted on New Zealand white rabbits, Alhabshan et al. (145) analyzed the effects of CAP on wound healing after corneal epithelial and basement membrane ablation. The results indicated that CAP did not hinder the rate of wound closure, induce increased inflammation, or adversely affect corneal wound healing. These findings highlight the potential of CP as a beneficial treatment for wound healing, and further research on the impact of CP on wound healing in animals can be explored in Table 3.
8. Advantages and limitations of cold plasma for veterinary bacterial diseases
In veterinary medicine, there is growing interest in utilizing CP, also known as non-thermal plasma, as a promising treatment option for bacterial diseases. This innovative technology creates a plasma field that generates active species such as reactive oxygen and nitrogen species (RONS), known for their antimicrobial properties. The use of CP in animal healthcare brings various advantages, including its ability to combat bacterial infections effectively. However, there are also certain limitations and restrictions associated with its application. Despite these restrictions, CP holds great potential as an alternative treatment for bacterial diseases in veterinary medicine (59).
Advantages: safety: CP is a non-thermal process that poses no risk of heat generation, which makes it a safe option for treating animals. Unlike traditional thermal plasma, it does not harm tissues by causing thermal injury, therefore it can be used in sensitive areas such as the eye or ear. Efficacy: CP has exhibited antimicrobial properties against a broad spectrum of bacteria, including resistant strains. In veterinary medicine, it has been successfully employed to treat bacterial infections in animals, including skin and wound infections. Versatility: CP can be administered in various forms, including sprays and topical applications, rendering it a versatile treatment option for different kinds of bacterial infections. Environmental sustainability: CP does not produce hazardous waste; thus, it is an environmentally friendly alternative to traditional antibiotics. Limitations: cost: the equipment required for CP treatment can be expensive, making it a cost-prohibitive option for some veterinary practices. Lack of standardization: there is currently a lack of standardization in the field of CP treatment, making it difficult to compare the efficacy of different devices. Limited research: although CP has shown promise as a treatment option for bacterial infections in animals, further research is needed to fully understand its mechanism of action and efficacy. Regulatory approval: CP is currently not approved by regulatory agencies for the treatment of bacterial infections in animals, making it difficult for veterinary practices to use it in a clinical setting.
In conclusion, CP shows promise as a treatment option for bacterial diseases in veterinary medicine. However, more research is necessary to gain a comprehensive understanding of its mechanisms of action, effectiveness, and safety. Furthermore, the high cost of equipment and the absence of regulatory approval may restrict its widespread adoption in the field of veterinary medicine. Despite these challenges, continued exploration and development of CP technology could potentially overcome these limitations and contribute to improved treatment options for bacterial diseases in animals.
8.1. Future directions for cold plasma research in veterinary bacteriology
Although the use of CP has demonstrated promising outcomes in managing a range of bacterial diseases in veterinary medicine, there is still a lot to be explored in this domain. To further enhance the use of CP in veterinary bacteriology, various areas of research must be considered. One of the crucial areas for future exploration is the development of new plasma sources. At present, most CP devices are based on high-voltage discharge systems, which can be intricate and costly to operate. There is a requirement for the development of more compact and cost-efficient plasma sources that can be easily integrated into veterinary clinics. Furthermore, research is needed to evaluate the effectiveness of these new plasma sources in controlling various bacterial infections (147). Optimization: the optimization of treatment protocols is a crucial field of study that requires attention. At present, information is scarce regarding the ideal conditions for employing CP for managing bacterial infections in animals. It is necessary to conduct research to determine the most efficient plasma parameters, including treatment duration, power density, and exposure range, for various bacterial strains and hosts. This initiative will aid in enhancing the effectiveness and safety of CP treatments in veterinary medicine (148). Evaluation of the mechanisms of action: the precise means by which CP eradicates bacteria is yet to be fully comprehended. Additional research is necessary to assess the mechanisms of action of CP and its interactions with various bacterial species and hosts. This knowledge will be essential in the creation of novel plasma sources and treatment protocols that are tailored to manage specific bacterial infections (149). Although several studies have deemed CP safe and non-toxic, further research is required to evaluate its safety and toxicity in animals. This will enable us to determine any potential side effects of plasma treatments and verify their safety for use in veterinary medicine. To summarize, while CP exhibits potential as a tool for controlling bacterial infections in veterinary medicine, extensive research and development are still necessary. Key areas for future investigation in this field include the development of new plasma sources, optimization of treatment protocols, evaluation of mechanisms of action, and assessment of safety and toxicity (125).
9. Conclusion
In conclusion, the use of CP in veterinary medicine for treating bacterial diseases is a promising and emerging field of research. CP has demonstrated significant potential in managing various bacterial infections in veterinary medicine, including respiratory infections, skin infections, and wound infections (36). One of the most notable advantages of implementing CP is its capability to eradicate a wide spectrum of bacteria, which includes strains that are resistant to antibiotics. Moreover, it can boost the immune system and expedite the process of wound healing. Nevertheless, CP therapy in veterinary medicine has certain limitations. Ensuring consistent and uniform exposure of the affected area to the plasma can pose a challenge and may affect its efficacy. Additionally, more research needs to be conducted to establish optimal treatment conditions, such as exposure time and plasma density, for different types of bacterial infections. Although the use of CP in veterinary medicine holds great potential, further investigation is imperative to fully comprehend its advantages and drawbacks. Future studies should concentrate on optimizing treatment protocols and assessing the long-term consequences of CP therapy on both bacterial infections and animal health.
Author contributions
PM and NF wrote the first draft. AG revised the manuscript. All authors contributed to the article and approved the submitted version.
Funding
This work is based upon research funded by Iran National Science Foundation (INSF) under Project No. 4015898.
Conflict of interest
The authors declare that the research was conducted in the absence of any commercial or financial relationships that could be construed as a potential conflict of interest.
Publisher’s note
All claims expressed in this article are solely those of the authors and do not necessarily represent those of their affiliated organizations, or those of the publisher, the editors and the reviewers. Any product that may be evaluated in this article, or claim that may be made by its manufacturer, is not guaranteed or endorsed by the publisher.
References
1. Whitehouse, CA, Zhao, S, and Tate, H. Antimicrobial resistance in Campylobacter species: mechanisms and genomic epidemiology. Adv Appl Microbiol. (2018) 103:1–47. doi: 10.1016/bs.aambs.2018.01.001
2. McDermott, PF, Zhao, S, and Tate, H. Antimicrobial resistance in nontyphoidal Salmonella. Microbiol Spectr. (2018). 6:261–87. doi: 10.1128/microbiolspec.ARBA-0014-2017
3. Haag, AF, Ross Fitzgerald, J, and Penadés, JR. Staphylococcus aureus in animals. Microbiol Spectr. (2019) 7:3–7. doi: 10.1128/9781683670131.ch46
4. Abraham, JL, Morris, DO, Griffeth, GC, Shofer, FS, and Rankin, SC. Surveillance of healthy cats and cats with inflammatory skin disease for colonization of the skin by methicillin-resistant coagulase-positive staphylococci and Staphylococcus schleiferi ssp. schleiferi. Vet Dermatol. (2007) 18:252–9. doi: 10.1111/j.1365-3164.2007.00604.x
5. Griffeth, GC, Morris, DO, Abraham, JL, Shofer, FS, and Rankin, SC. Screening for skin carriage of methicillin-resistant coagulase-positive staphylococci and Staphylococcus schleiferi in dogs with healthy and inflamed skin. Vet Dermatol. (2008) 19:142–9. doi: 10.1111/j.1365-3164.2008.00663.x
6. Weese, JS, and van Duijkeren, E. Methicillin-resistant Staphylococcus aureus and Staphylococcus pseudintermedius in veterinary medicine. Vet Microbiol. (2010) 140:418–29. doi: 10.1016/j.vetmic.2009.01.039
7. van Hoovels, L, Vankeerberghen, A, Boel, A, van Vaerenbergh, K, and de Beenhouwer, H. First case of Staphylococcus pseudintermedius infection in a human. J Clin Microbiol. (2006) 44:4609–12. doi: 10.1128/JCM.01308-06
8. Robb, AR, Wright, ED, Foster, AME, Walker, R, and Malone, C. Skin infection caused by a novel strain of Staphylococcus pseudintermedius in a Siberian husky dog owner. JMM Case Rep. (2017) 4:e005087. doi: 10.1099/jmmcr.0.005087
9. Wegener, A, Broens, EM, Zomer, A, Spaninks, M, Wagenaar, JA, and Duim, B. Comparative genomics of phenotypic antimicrobial resistances in methicillin-resistant Staphylococcus pseudintermedius of canine origin. Vet Microbiol. (2018) 225:125–31. doi: 10.1016/j.vetmic.2018.09.013
10. Worthing, KA, Abraham, S, Coombs, GW, Pang, S, Saputra, S, Jordan, D, et al. Clonal diversity and geographic distribution of methicillin-resistant Staphylococcus pseudintermedius from Australian animals: discovery of novel sequence types. Vet Microbiol. (2018) 213:58–65. doi: 10.1016/j.vetmic.2017.11.018
11. Płókarz, D, Czopowicz, M, Bierowiec, K, and Rypuła, K. Virulence genes as markers for Pseudomonas aeruginosa biofilm formation in dogs and cats. Animals. (2022) 12:422. doi: 10.3390/ani12040422
12. Hillier, A, Alcorn, JR, Cole, LK, and Kowalski, JJ. Pyoderma caused by Pseudomonas aeruginosa infection in dogs: 20 cases. Vet Dermatol. (2006) 17:432–9. doi: 10.1111/j.1365-3164.2006.00550.x
13. Robinson, VH, Paterson, S, Bennett, C, and Steen, SI. Biofilm production of Pseudomonas spp. isolates from canine otitis in three different enrichment broths. Vet Dermatol. (2019) 30:218–e67. doi: 10.1111/vde.12738
14. Prado, MR, Rocha, MFG, Brito, ÉHS, Girão, MD, Monteiro, AJ, Teixeira, MFS, et al. Survey of bacterial microorganisms in the conjunctival sac of clinically Normal dogs and dogs with ulcerative keratitis in Fortaleza, Ceará, Brazil. Vet Ophthalmol. (2005) 8:33–7. doi: 10.1111/j.1463-5224.2005.04061.x
15. Sharma, D, Pakravan, N, Pritchard, JC, Hartmann, FA, and Young, KM. Mucoid Pseudomonas aeruginosa infection in a cat with severe chronic rhinosinusitis. Vet Clin Pathol. (2019) 48:300–4. doi: 10.1111/vcp.12749
16. Chawnan, N, Lampang, KN, Mektrirat, R, Awaiwanont, N, and Thongkorn, K. Cultivation of bacterial pathogens and antimicrobial resistance in canine periapical tooth abscesses. Vet Integr Sci. (2021) 19:513–24. doi: 10.12982/VIS.2021.040
17. Riggio, MP, Lennon, A, Taylor, DJ, and Bennett, D. Molecular identification of bacteria associated with canine periodontal disease. Vet Microbiol. (2011) 150:394–400. doi: 10.1016/j.vetmic.2011.03.001
18. Pormohammad, A, Nasiri, MJ, and Azimi, T. Prevalence of antibiotic resistance in Escherichia coli strains simultaneously isolated from humans, animals, food, and the environment: a systematic review and Meta-analysis. Infect Drug Resist. (2019) 12:1181–97. doi: 10.2147/IDR.S201324
19. Olmos, J, Acosta, M, Mendoza, G, and Pitones, V. Bacillus subtilis, an ideal probiotic bacterium to shrimp and fish aquaculture that increase feed digestibility, prevent microbial diseases, and avoid water pollution. Arch Microbiol. (2020) 202:427–35. doi: 10.1007/s00203-019-01757-2
20. Fenwick, BW, and Rycroft, AN. Pasteurellaceae: Actinobacillus. Veterinary Microbiology (United States:Wiley). (2022) 16: 118–28. doi: 10.1002/9781119650836.ch11
21. Sargison, N, Crilly, JP, and Hopker, A. Practical lambing and lamb care: a veterinary guide. United States: John Wiley & Sons (2018).
22. Prestinaci, F, Pezzotti, P, and Pantosti, A. Antimicrobial resistance: a global multifaceted phenomenon. Pathog Glob Health. (2015) 109:309–18. doi: 10.1179/2047773215Y.0000000030
23. Aarestrup, FM. Veterinary drug usage and antimicrobial resistance in bacteria of animal origin. Basic Clin Pharmacol Toxicol. (2005) 96:271–81. doi: 10.1111/j.1742-7843.2005.pto960401.x
24. Van, TT, Hao, ZY, Smooker, PM, and Coloe, PJ. Antibiotic use in food animals worldwide, with a focus on Africa: pluses and minuses. J Glob Antimicrob Resist. (2020) 20:170–7. doi: 10.1016/j.jgar.2019.07.031
25. Reygaert, WC. An overview of the antimicrobial resistance mechanisms of bacteria. AIMS Microbiol. (2018) 4:482–501. doi: 10.3934/microbiol.2018.3.482
26. Kırmusaoğlu, S. Staphylococcal biofilms: pathogenicity, mechanism and regulation of biofilm formation by quorum sensing system and antibiotic resistance mechanisms of biofilm embedded microorganisms. Microb. biofilms importance Appl. United Kingdom: IntechOpen, (2016) 189–209.
27. Theuretzbacher, U. Global antimicrobial resistance in gram-negative pathogens and clinical need. Curr Opin Microbiol. (2017) 39:106–12. doi: 10.1016/j.mib.2017.10.028
28. Pontes, JT, de, C, Borges, ABT, Roque-Borda, CA, and Pavan, FR. Antimicrobial peptides as an alternative for the eradication of bacterial biofilms of multi-drug resistant bacteria. Pharmaceutics. (2022) 14:642. doi: 10.3390/pharmaceutics14030642
29. Daeschlein, G, Napp, M, von Podewils, S, Lutze, S, Emmert, S, Lange, A, et al. In vitro susceptibility of multidrug resistant skin and wound pathogens against low temperature atmospheric pressure plasma jet (APPJ) and dielectric barrier discharge plasma (DBD). Plasma Process Polym. (2014) 11:175–83. doi: 10.1002/ppap.201300070
30. Kim, E-J, Hyun, J-E, Kang, Y-H, Baek, S-J, and Hwang, C-Y. In vitro antibacterial and antibiofilm effects of cold atmospheric microwave plasma against Pseudomonas aeruginosa causing canine skin and ear infections. Vet Dermatol. (2022) 33:29–e10. doi: 10.1111/vde.13030
32. Tabares, FL, and Junkar, I. Cold plasma systems and their application in surface treatments for medicine. Molecules. (2021) 26:1903. doi: 10.3390/molecules26071903
33. Murphy, AB, and Uhrlandt, D. Foundations of high-pressure thermal plasmas. Plasma Sources Sci Technol. (2018) 27:63001. doi: 10.1088/1361-6595/aabdce
34. Gururani, P, Bhatnagar, P, Bisht, B, Kumar, V, Joshi, NC, Tomar, MS, et al. Cold plasma technology: advanced and sustainable approach for wastewater treatment. Environ Sci Pollut Res. (2021) 28:65062–82. doi: 10.1007/s11356-021-16741-x
35. Berthiaume, F, and Hsia, HC. Regenerative approaches for chronic wounds. Annu Rev Biomed Eng. (2022) 24:61–83. doi: 10.1146/annurev-bioeng-010220-113008
36. Bekeschus, S, Kramer, A, and Schmidt, A. Gas plasma-augmented wound healing in animal models and veterinary medicine. Molecules. (2021) 26:5682. doi: 10.3390/molecules26185682
37. Mouele, ES, Massima, JO, Tijani, KO, Badmus, OP, Babajide, O, Fatoba, OO, et al. A critical review on ozone and co-species, generation and reaction mechanisms in plasma induced by dielectric barrier discharge technologies for wastewater remediation. J Environ Chem Eng. (2021) 9:105758. doi: 10.1016/j.jece.2021.105758
38. Mouele, M, Emile, S, Fatoba, OO, Babajide, O, Badmus, KO, and Petrik, LF. Review of the methods for determination of reactive oxygen species and suggestion for their application in advanced oxidation induced by dielectric barrier discharges. Environ Sci Pollut Res. (2018) 25:9265–82. doi: 10.1007/s11356-018-1392-9
39. Lamichhane, P, Acharya, TR, Kaushik, N, Nguyen, LN, Lim, JS, Hessel, V, et al. Non-thermal argon plasma jets of various lengths for selective reactive oxygen and nitrogen species production. J Environ Chem Eng. (2022) 10:107782. doi: 10.1016/j.jece.2022.107782
40. Sarangapani, C, Ziuzina, D, Behan, P, Boehm, D, Gilmore, BF, Cullen, PJ, et al. Degradation kinetics of cold plasma-treated antibiotics and their antimicrobial activity. Sci Rep. (2019) 9:1–15. doi: 10.1038/s41598-019-40352-9
41. Bangar, P, Sneh, SS, Nayi, P, and Phimolsiripol, Y. Cold plasma for microbial safety: principle, mechanism, and factors responsible. J Food Process Preserv. (2022) 46:e16850. doi: 10.1111/jfpp.16850
42. Chen, Z, Chen, G, Obenchain, R, Zhang, R, Bai, F, Fang, T, et al. Cold atmospheric plasma delivery for biomedical applications. Mater Today. (2022) 54:153–88. doi: 10.1016/j.mattod.2022.03.001
43. Burts, ML, Alexeff, I, Meek, ET, and McCullers, JA. Use of atmospheric non-thermal plasma as a disinfectant for objects contaminated with methicillin-resistant Staphylococcus aureus. Am J Infect Control. (2009) 37:729–33. doi: 10.1016/j.ajic.2009.03.010
44. Cahill, OJ, Claro, T, O’Connor, N, Cafolla, AA, Stevens, NT, Daniels, S, et al. Cold air plasma to decontaminate inanimate surfaces of the hospital environment. Appl Environ Microbiol. (2014) 80:2004–10. doi: 10.1128/AEM.03480-13
45. Hamza, E, Dorgham, SM, and Hamza, DA. Carbapenemase-producing Klebsiella pneumoniae in broiler poultry farming in Egypt. J Glob Antimicrob Resist. (2016) 7:8–10. doi: 10.1016/j.jgar.2016.06.004
46. Holland, RE. Some infectious causes of diarrhea in young farm animals. Clin Microbiol Rev. (1990) 3:345–75. doi: 10.1128/CMR.3.4.345
47. Heo, NS, Lee, M-K, Kim, GW, Lee, SJ, Park, JY, and Park, TJ. Microbial inactivation and pesticide removal by remote exposure of atmospheric air plasma in confined environments. J Biosci Bioeng. (2014) 117:81–5. doi: 10.1016/j.jbiosc.2013.06.007
48. Julák, JHS, Soušková, H, Scholtz, V, Kvasničková, E, Savická, D, and Kříha, V. Comparison of fungicidal properties of non-thermal plasma produced by corona discharge and dielectric barrier discharge. Folia Microbiol. (2018) 63:63–8. doi: 10.1007/s12223-017-0535-6
49. Soušková, H, Scholtz, V, Julák, J, Kommová, L, Savická, D, and Pazlarová, J. The survival of micromycetes and yeasts under the low-temperature plasma generated in electrical discharge. Folia Microbiol. (2011) 56:77–9. doi: 10.1007/s12223-011-0005-5
50. Fricke, K, Koban, I, Tresp, H, Jablonowski, L, Schröder, K, Kramer, A, et al. Atmospheric pressure plasma: a high-performance tool for the efficient removal of biofilms. PLoS One. (2012) 7:e42539. doi: 10.1371/journal.pone.0042539
51. Julák, J, Scholtz, V, and Vaňková, E. Medically important biofilms and non-thermal plasma. World J Microbiol Biotechnol. (2018) 34:1–15. doi: 10.1007/s11274-018-2560-2
52. Xu, L, Tu, Y, Yu, Y, Tan, M, Li, J, and Chen, H. Augmented survival of Neisseria gonorrhoeae within biofilms: exposure to atmospheric pressure non-thermal plasmas. Eur J Clin Microbiol Infect Dis. (2011) 30:25–31. doi: 10.1007/s10096-010-1047-3
53. Di Martino, G, Piccirillo, A, Giacomelli, M, Comin, D, Gallina, A, Capello, K, et al. Microbiological, chemical and physical quality of drinking water for commercial turkeys: a cross-sectional study. Poult Sci. (2018) 97:2880–6. doi: 10.3382/ps/pey130
54. Lu, XP, Ye, T, Cao, YG, Sun, ZY, Xiong, Q, Tang, ZY, et al. The roles of the various plasma agents in the inactivation of bacteria. J Appl Phys. (2008) 104:53309. doi: 10.1063/1.2977674
55. Xiao, D, Cheng, C, Lan, Y, Ni, GH, Shen, J, Meng, YD, et al. Effects of atmospheric-pressure Nonthermal nitrogen and air plasma on bacteria inactivation. IEEE Trans Plasma Sci. (2016) 44:2699–707. doi: 10.1109/TPS.2016.2598564
56. Kim, SJ, Chung, TH, Bae, SH, and Leem, SH. Bacterial inactivation using atmospheric pressure single pin electrode microplasma jet with a ground ring. Appl Phys Lett. (2009) 94:141502. doi: 10.1063/1.3114407
57. Kostov, KG, Rocha, V, Koga-Ito, CY, Matos, BM, Algatti, MA, Honda, RY, et al. Bacterial sterilization by a dielectric barrier discharge (DBD) in air. Surf Coat Technol. (2010) 204:2954–9. doi: 10.1016/j.surfcoat.2010.01.052
58. Xiong, Z, Lu, XP, Feng, A, Pan, Y, and Ostrikov, K. Highly effective fungal inactivation in He+ O2 atmospheric-pressure nonequilibrium plasmas. Phys Plasmas. (2010) 17:123502. doi: 10.1063/1.3526678
59. Das, S, Gajula, VP, Mohapatra, S, Singh, G, and Kar, S. Role of cold atmospheric plasma in microbial inactivation and the factors affecting its efficacy. Health Sci Rev. (2022) 4:100037. doi: 10.1016/j.hsr.2022.100037
60. Sharma, A, Pruden, A, Stan, O, and Collins, GJ. Bacterial inactivation using an RF-powered atmospheric pressure plasma. IEEE Trans Plasma Sci. (2006) 34:1290–6. doi: 10.1109/TPS.2006.878377
61. Wiegand, C, Fink, S, Hipler, UC, Beier, O, Horn, K, Pfuch, A, et al. Cold atmospheric pressure plasmas exhibit antimicrobial properties against critical bacteria and yeast species. J Wound Care. (2017) 26:462–8. doi: 10.12968/jowc.2017.26.8.462
62. Sharma, J, Sharma, M, and Ray, P. Detection of TEM & SHV genes in Escherichia coli & Klebsiella pneumoniae isolates in a tertiary care hospital from India. Indian J Med Res. (2010) 132:332–6.
63. Shouzhe, L, and Jinpyo, L. Comparison of sterilizing effect of nonequilibrium atmospheric-pressure He/O2 and Ar/O2 plasma jets. Plasma Sci Technol. (2008) 10:61–4. doi: 10.1088/1009-0630/10/1/13
64. Pedroni, M, Morandi, S, Silvetti, T, Cremona, A, Gittini, G, Nardone, A, et al. Bacteria inactivation by atmospheric pressure plasma jet treatment. J Vac Sci Technol B. (2018) 36:01A107. doi: 10.1116/1.4995546
65. Lotfy, K, Khalil, SM, and El-Raheem, HA. Inactivation by helium cold atmospheric pressure plasma for Escherichia coli and Staphylococcus aureus. J Theor Appl Phys. (2020) 14:37–45. doi: 10.1007/s40094-019-00362-4
66. Guimin, X, Guanjun, Z, Shi Xingmin, MA, Yue, WN, and Yuan, L. Bacteria inactivation using DBD plasma jet in atmospheric pressure argon. Plasma Sci Technol. (2009) 11:83–8. doi: 10.1088/1009-0630/11/1/17
67. Deng, XT, Shi, JJ, Shama, G, and Kong, MG. Effects of microbial loading and sporulation temperature on atmospheric plasma inactivation of Bacillus subtilis spores. Appl Phys Lett. (2005) 87:153901. doi: 10.1063/1.2103394
68. Lee, K-N, Paek, K-h, Won-Tae, J, and Lee, Y-H. Sterilization of bacteria, yeast, and bacterial endospores by atmospheric-pressure cold plasma using helium and oxygen. J Microbiol. (2006) 44:269–75.
69. Shaw, P, Kumar, N, Mumtaz, S, Lim, JS, Jang, JH, Kim, D, et al. Evaluation of non-thermal effect of microwave radiation and its mode of action in bacterial cell inactivation. Sci Rep. (2021) 11:1–12. doi: 10.1038/s41598-021-93274-w
70. Niedźwiedź, I, Waśko, A, Pawłat, J, and Polak-Berecka, M. The state of research on antimicrobial activity of cold plasma. Pol J Microbiol. (2019) 68:153–64. doi: 10.33073/pjm-2019-028
71. Olatunde, OO, Benjakul, S, and Vongkamjan, K. Dielectric barrier discharge cold atmospheric plasma: bacterial inactivation mechanism. J Food Saf. (2019) 39:e12705. doi: 10.1111/jfs.12705
72. Winter, S, Meyer-Lindenberg, A, Wolf, G, Reese, S, and Nolff, MC. In vitro evaluation of the decontamination effect of cold atmospheric argon plasma on selected bacteria frequently encountered in small animal bite injuries. J Microbiol Methods. (2020) 169:105728. doi: 10.1016/j.mimet.2019.105728
73. Huang, M, Zhuang, H, Zhao, J, Wang, J, Yan, W, and Zhang, J. Differences in cellular damage induced by dielectric barrier discharge plasma between Salmonella typhimurium and Staphylococcus aureus. Bioelectrochemistry. (2020) 132:107445. doi: 10.1016/j.bioelechem.2019.107445
74. Brun, P, Bernabè, G, Marchiori, C, Scarpa, M, Zuin, M, Cavazzana, R, et al. Antibacterial efficacy and mechanisms of action of low power atmospheric pressure cold plasma: membrane permeability, biofilm penetration and antimicrobial sensitization. J Appl Microbiol. (2018) 125:398–408. doi: 10.1111/jam.13780
75. Gan, Z, Feng, X, Hou, Y, Sun, A, and Wang, R. Cold plasma jet with dielectric barrier configuration: investigating its effect on the cell membrane of E. coli and S. cerevisiae and its impact on the quality of chokeberry juice. LWT. (2021) 136:110223. doi: 10.1016/j.lwt.2020.110223
76. Kim, JH, and Min, SC. Moisture vaporization-combined helium dielectric barrier discharge-cold plasma treatment for microbial decontamination of onion flakes. Food Control. (2018) 84:321–9. doi: 10.1016/j.foodcont.2017.08.018
77. Min, SC, Roh, SH, Niemira, BA, Sites, JE, Boyd, G, and Lacombe, A. Dielectric barrier discharge atmospheric cold plasma inhibits Escherichia coli O157:H7, Salmonella, Listeria monocytogenes, and Tulane virus in Romaine lettuce. Int J Food Microbiol. (2016) 237:114–20. doi: 10.1016/j.ijfoodmicro.2016.08.025
78. Mai-Prochnow, A, Clauson, M, Hong, J, and Murphy, AB. Gram positive and gram negative bacteria differ in their sensitivity to cold plasma. Sci Rep. (2016) 6:1–11. doi: 10.1038/srep38610
79. Han, L, Patil, S, Daniela Boehm, V, Milosavljević, PJC, and Bourke, P. Mechanisms of inactivation by high-voltage atmospheric cold plasma differ for Escherichia coli and Staphylococcus aureus. Appl Environ Microbiol. (2016) 82:450–8. doi: 10.1128/AEM.02660-15
80. Wan, Z, Chen, Y, Pankaj, SK, and Keener, KM. High voltage atmospheric cold plasma treatment of refrigerated chicken eggs for control of Salmonella enteritidis contamination on egg shell. LWT. (2017) 76:124–30. doi: 10.1016/j.lwt.2016.10.051
81. Lührmann, A, Matthes, R, and Kramer, A. Impact of cold atmospheric pressure argon plasma on antibiotic sensitivity of methicillin-resistant Staphylococcus aureus strains in vitro. GMS Hyg Infect Control. (2016) 11:17. doi: 10.3205/dgkh000277
82. Mazandarani, A, Goudarzi, S, Jafarabadi, M, and Nekoo, EA. Effects of cold plasma on Staphylococcus aureus. J Family Reprod Health. (2022) 16:212–6. doi: 10.18502/jfrh.v16i3.10583
83. Theinkom, F, Singer, L, Cieplik, F, Cantzler, S, Weilemann, H, Cantzler, M, et al. Antibacterial efficacy of cold atmospheric plasma against Enterococcus faecalis planktonic cultures and biofilms in vitro. PLoS One. (2019) 14:e0223925. doi: 10.1371/journal.pone.0223925
84. Jin, H-J, Hwang, C-Y, Kang, J-H, Baek, S-J, and Hyun, J-E. In vitro antimicrobial activity of cold atmospheric microwave plasma against bacteria causing canine skin and ear infections. Vet Dermatol. (2021) 32:462–e126. doi: 10.1111/vde.13012
85. Arserim, EH, Salvi, D, Fridman, G, Schaffner, DW, and Karwe, MV. Microbial inactivation by non-equilibrium short-pulsed atmospheric pressure dielectric barrier discharge (cold plasma): numerical and experimental studies. Food Eng Rev. (2021) 13:136–47. doi: 10.1007/s12393-020-09256-7
86. Dijksteel, GS, Ulrich, MMW, Vlig, M, Sobota, A, Middelkoop, E, and Boekema, BKHL. Safety and bactericidal efficacy of cold atmospheric plasma generated by a flexible surface dielectric barrier discharge device against Pseudomonas aeruginosa in vitro and in vivo. Ann Clin Microbiol Antimicrob. (2020) 19:37. doi: 10.1186/s12941-020-00381-z
87. Nicol, MKJ, Brubaker, TR, Honish, BJ, Simmons, AN, Kazemi, A, Geissel, MA, et al. Antibacterial effects of low-temperature plasma generated by atmospheric-pressure plasma jet are mediated by reactive oxygen species. Sci Rep. (2020) 10:3066. doi: 10.1038/s41598-020-59652-6
88. Laurita, R, Barbieri, D, Gherardi, M, Colombo, V, and Lukes, P. Chemical analysis of reactive species and antimicrobial activity of water treated by nanosecond pulsed DBD air plasma. Clin Plasma Med. (2015) 3:53–61. doi: 10.1016/j.cpme.2015.10.001
89. Zhang, Q., Feng, H., Tian, Y., Ma, R., Fang, J., Zhang, J., et al.. (2013). “A study of oxidative stress induced by non-thermal plasma activated water for bacterial damage.” IEEE International Conference on Plasma Science (ICOPS)
90. Lu, H, Patil, S, Keener, KM, Cullen, PJ, and Bourke, P. Bacterial inactivation by high-voltage atmospheric cold plasma: influence of process parameters and effects on cell leakage and DNA. J Appl Microbiol. (2014) 116:784–94. doi: 10.1111/jam.12426
91. Ulbin-Figlewicz, N, Jarmoluk, A, and Marycz, K. Antimicrobial activity of low-pressure plasma treatment against selected foodborne bacteria and meat microbiota. Ann Microbiol. (2015) 65:1537–46. doi: 10.1007/s13213-014-0992-y
92. Patange, A, O’Byrne, C, Daniela Boehm, PJ, Cullen, KK, and Bourke, P. The effect of atmospheric cold plasma on bacterial stress responses and virulence using Listeria monocytogenes knockout mutants. Front Microbiol. (2019) 10:2841. doi: 10.3389/fmicb.2019.02841
93. Dragoš, A, and Kovács, ÁT. The peculiar functions of the bacterial extracellular matrix. Trends Microbiol. (2017) 25:257–66. doi: 10.1016/j.tim.2016.12.010
94. Del Pozo, JL, and Patel, R. The challenge of treating biofilm-associated bacterial infections. Clin Pharmacol Ther. (2007) 82:204–9. doi: 10.1038/sj.clpt.6100247
95. Gilmore, BF, Flynn, PB, O’Brien, S, Hickok, N, Freeman, T, and Bourke, P. Cold plasmas for biofilm control: opportunities and challenges. Trends Biotechnol. (2018) 36:627–38. doi: 10.1016/j.tibtech.2018.03.007
96. Mancuso, G, Midiri, A, Gerace, E, and Biondo, C. Bacterial antibiotic resistance: the most critical pathogens. Pathogens. (2021) 10:1310. doi: 10.3390/pathogens12010116
97. Park, H, Ji, NK, Park, DH, Yusupov, M, Neyts, EC, Verlackt, CCW, et al. A comparative study for the inactivation of multidrug resistance bacteria using dielectric barrier discharge and nano-second pulsed plasma. Sci Rep. (2015) 5:13849. doi: 10.1038/srep13849
98. Napp, M, Daeschlein, G, von Podewils, S, Hinz, P, Emmert, S, Haase, H, et al. In vitro susceptibility of methicillin-resistant and methicillin-susceptible strains of Staphylococcus aureus to two different cold atmospheric plasma sources. Infection. (2016) 44:531–7. doi: 10.1007/s15010-016-0888-9
99. Ziuzina, D, Boehm, D, Patil, S, Cullen, PJ, and Bourke, P. Cold plasma inactivation of bacterial biofilms and reduction of quorum sensing regulated virulence factors. PLoS One. (2015) 10:e0138209. doi: 10.1371/journal.pone.0138209
100. Alkawareek, MY, Qais, T, Algwari, GL, Gorman, SP, Graham, WG, O’Connell, D, et al. Eradication of Pseudomonas aeruginosa biofilms by atmospheric pressure non-thermal plasma. PLoS One. (2012) 7:e44289. doi: 10.1371/journal.pone.0044289
101. Cao, Y, Yang, P, Lu, X, Xiong, Z, Ye, T, Xiong, Q, et al. Efficacy of atmospheric pressure plasma as an antibacterial agent against Enterococcus faecalis in vitro. Plasma Sci Technol. (2011) 13:93–8. doi: 10.1088/1009-0630/13/1/19
102. Puač, N, Miletić, M, Mojović, M, Popović-Bijelić, A, Vuković, D, Miličić, B, et al. Sterilization of bacteria suspensions and identification of radicals deposited during plasma treatment. Open Chem. (2015) 13:000010151520150041. doi: 10.1515/chem-2015-0041
103. Modic, M, McLeod, NP, Mark Sutton, J, and Walsh, JL. Cold atmospheric pressure plasma elimination of clinically important single- and mixed-species biofilms. Int J Antimicrob Agents. (2017) 49:375–8. doi: 10.1016/j.ijantimicag.2016.11.022
104. Cotter, JJ, Maguire, P, Soberon, F, Daniels, S, O’Gara, JP, and Casey, E. Disinfection of meticillin-resistant Staphylococcus aureus and Staphylococcus epidermidis biofilms using a remote non-thermal gas plasma. J Hosp Infect. (2011) 78:204–7. doi: 10.1016/j.jhin.2011.03.019
105. Flynn, PB, Graham, WG, and Gilmore, BF. Acinetobacter baumannii biofilm biomass mediates tolerance to cold plasma. Lett Appl Microbiol. (2019) 68:344–9. doi: 10.1111/lam.13122
106. Jiang, C, Schaudinn, C, Jaramillo, DE, Webster, P, and Costerton, J. In vitro antimicrobial effect of a cold plasma jet against Enterococcus faecalis biofilms. ISRN Dent. (2012) 2012:295736. doi: 10.5402/2012/295736
107. Hong, YF, Kang, JG, Lee, HY, Uhm, HS, Moon, E, and Park, YH. Sterilization effect of atmospheric plasma on Escherichia coli and Bacillus subtilis endospores. Lett Appl Microbiol. (2009) 48:33–7. doi: 10.1111/j.1472-765X.2008.02480.x
108. Morris, AD, McCombs, GB, Akan, T, Hynes, W, Laroussi, M, and Tolle, SL. Cold plasma technology: bactericidal effects on Geobacillus stearothermophilus and Bacillus cereus microorganisms. J Dent Hyg. (2009) 83:55–61.
109. Hertwig, C, Reineke, K, Rauh, C, and Schlüter, O. Factors involved in Bacillus spore’s resistance to cold atmospheric pressure plasma. Innov Food Sci Emerg Technol. (2017) 43:173–81. doi: 10.1016/j.ifset.2017.07.031
110. Niemira, BA, Boyd, G, and Sites, J. Cold plasma inactivation of Escherichia Coli O157: H7 biofilms. Front Sustain Food Syst. (2018) 2:47. doi: 10.3389/fsufs.2018.00047
111. Zhu, Y, Li, C, Cui, H, and Lin, L. Feasibility of cold plasma for the control of biofilms in food industry. Trends Food Sci Technol. (2020) 99:142–51. doi: 10.1016/j.tifs.2020.03.001
112. Flemming, H-C, van Hullebusch, ED, Neu, TR, Nielsen, PH, Seviour, T, Stoodley, P, et al. The biofilm matrix: multitasking in a shared space. Nat Rev Microbiol. (2023) 21:70–86. doi: 10.1038/s41579-022-00791-0
113. Benbelaïd, F, Khadir, A, Abdoune, MA, Bendahou, M, Muselli, A, and Costa, J. Antimicrobial activity of some essential oils against oral multidrug-resistant Enterococcus faecalis in both planktonic and biofilm state. Asian Pac J Trop Biomed. (2014) 4:463–72. doi: 10.12980/APJTB.4.2014C1203
114. Khalifa, L, Brosh, Y, Gelman, D, Coppenhagen-Glazer, S, Beyth, S, Poradosu-Cohen, R, et al. Targeting Enterococcus faecalis biofilms with phage therapy. Appl Environ Microbiol. (2015) 81:2696–705. doi: 10.1128/AEM.00096-15
115. Schmidt, A, Liebelt, G, Striesow, J, Freund, E, von Woedtke, T, Wende, K, et al. The molecular and physiological consequences of cold plasma treatment in murine skin and its barrier function. Free Radic Biol Med. (2020) 161:32–49. doi: 10.1016/j.freeradbiomed.2020.09.026
116. Kawai, M, Yamada, S, Ishidoshiro, A, Oyamada, Y, Ito, H, and Yamagishi, J-i. Cell-wall thickness: possible mechanism of acriflavine resistance in meticillin-resistant Staphylococcus aureus. J Med Microbiol. (2009) 58:331–6. doi: 10.1099/jmm.0.004184-0
118. Moisan, M, Barbeau, J, Moreau, S, Pelletier, J, Tabrizian, M, and Yahia, LH. Low-temperature sterilization using gas plasmas: a review of the experiments and an analysis of the inactivation mechanisms. Int J Pharm. (2001) 226:1–21. doi: 10.1016/S0378-5173(01)00752-9
119. Huang, Y, Ye, XP, Doona, CJ, Feeherry, FE, Radosevich, M, and Wang, S. An investigation of inactivation mechanisms of Bacillus amyloliquefaciens spores in non-thermal plasma of ambient air. J Sci Food Agric. (2019) 99:368–78. doi: 10.1002/jsfa.9198
120. Pina-Perez, MC, Martinet, D, Palacios-Gorba, C, Ellert, C, and Beyrer, M. Low-energy short-term cold atmospheric plasma: controlling the inactivation efficacy of bacterial spores in powders. Food Res Int. (2020) 130:108921. doi: 10.1016/j.foodres.2019.108921
121. Bokhorst-van de Veen, H, Xie, Houyu, Esveld, Erik, Abee, Tjakko, Mastwijk, Hennie, and Groot, Masja Nierop. (2015). “Inactivation of chemical and heat-resistant spores of Bacillus and Geobacillus by nitrogen cold atmospheric plasma evokes distinct changes in morphology and integrity of spores.” Food Microbiol 45:26–33, doi: 10.1016/j.fm.2014.03.018
122. Deng, X, Shi, J, and Kong, MG. Physical mechanisms of inactivation of Bacillus subtilis spores using cold atmospheric plasmas. IEEE Trans Plasma Sci. (2006) 34:1310–6. doi: 10.1109/TPS.2006.877739
123. Raguse, M, Fiebrandt, M, Denis, B, Stapelmann, K, Eichenberger, P, Driks, A, et al. Understanding of the importance of the spore coat structure and pigmentation in the Bacillus subtilis spore resistance to low-pressure plasma sterilization. J Phys D Appl Phys. (2016) 49:285401. doi: 10.1088/0022-3727/49/28/285401
124. Liao, X, Muhammad, AI, Chen, S, Yaqin, H, Ye, X, Liu, D, et al. Bacterial spore inactivation induced by cold plasma. Crit Rev Food Sci Nutr. (2019) 59:2562–72. doi: 10.1080/10408398.2018.1460797
125. Liao, X, Liu, D, Xiang, Q, Ahn, J, Chen, S, Ye, X, et al. Inactivation mechanisms of non-thermal plasma on microbes: a review. Food Control. (2017) 75:83–91. doi: 10.1016/j.foodcont.2016.12.021
126. Lv, R, Liu, D, and Zhou, J. Bacterial spore inactivation by non-thermal technologies: resistance and inactivation mechanisms. Curr Opin Food Sci. (2021) 42:31–6. doi: 10.1016/j.cofs.2020.12.014
127. Varga, JFA, Bui-Marinos, MP, and Katzenback, BA. Frog skin innate immune defences: sensing and surviving pathogens. Front Immunol. (2019) 9:3128. doi: 10.3389/fimmu.2018.03128
128. Lertpatipanpong, P, Sillapachaiyaporn, C, Garam, O, Kang, Y-H, Hwang, C-Y, and Baek, SJ. Effect of cold atmospheric microwave plasma (CAMP) on wound healing in canine keratinocytes. Front Cell Dev Biol. (2023) 11:1105692. doi: 10.3389/fcell.2023.1105692
129. Martines, E, Brun, P, Cavazzana, R, Cordaro, L, Zuin, M, Martinello, T, et al. Wound healing improvement in large animals using an indirect helium plasma treatment. Clin Plasma Med. (2020) 17–18:100095. doi: 10.1016/j.cpme.2020.100095
130. Nolff, MC, Winter, S, Reese, S, and Meyer-Lindenberg, A. Comparison of polyhexanide, cold atmospheric plasma and saline in the treatment of canine bite wounds. J Small Anim Pract. (2019) 60:348–55. doi: 10.1111/jsap.12971
131. Bolgeo, T, Maconi, A, Gardalini, M, Gatti, D, Di Matteo, R, Lapidari, M, et al. The role of cold atmospheric plasma in wound healing processes in critically ill patients. J Pers Med. (2023) 13:736. doi: 10.3390/jpm13050736
132. Fridman, G, Friedman, G, Gutsol, A, Shekhter, AB, Vasilets, VN, and Fridman, A. Applied plasma medicine. Plasma Process Polym. (2008) 5:503–33. doi: 10.1002/ppap.200700154
133. Reshetov, IV, Kabisov, RK, Shekhter, AB, Pekshev, AV, and Maneilova, MV. The use of a ‘plason’ air-plasma apparatus for coagulation and NO-therapy in plastic reconstructive surgery for oncologic patients. Ann Plast, Reconstr Aesthetic Surg. (2000) 4:24–38.
134. Shekhter, AB, Kabisov, RK, Pekshev, AV, Kozlov, NP, and Perov, YL. Experimental and clinical validation of plasmadynamic therapy of wounds with nitric oxide. Bull Exp Biol Med. (1998) 126:829–34. doi: 10.1007/BF02446923
135. Haertel, B, von Woedtke, T, Weltmann, K-D, and Lindequist, U. Non-thermal atmospheric-pressure plasma possible application in wound healing. Biomol Ther. (2014) 22:477–90. doi: 10.4062/biomolther.2014.105
136. Amini, MR, Sheikh Hosseini, M, Fatollah, S, Mirpour, S, Ghoranneviss, M, Larijani, B, et al. Beneficial effects of cold atmospheric plasma on inflammatory phase of diabetic foot ulcers; a randomized clinical trial. J Diabetes Metab Disord. (2020) 19:895–905. doi: 10.1007/s40200-020-00577-2
137. Arndt, S, Unger, P, Wacker, E, Shimizu, T, Heinlin, J, Li, Y-F, et al. Cold atmospheric plasma (CAP) changes gene expression of key molecules of the wound healing machinery and improves wound healing in vitro and in vivo. PLoS One. (2013) 8:e79325. doi: 10.1371/journal.pone.0079325
138. Zhang, J-P, Guo, L, Chen, Q-L, Zhang, K-Y, Wang, T, An, G-Z, et al. Effects and mechanisms of cold atmospheric plasma on skin wound healing of rats. Contrib Plasma Physics. (2019) 59:92–101. doi: 10.1002/ctpp.201800025
139. Dobrynin, D, Andrew, W, Kalghatgi, S, Park, S, Chernets, N, Wasko, K, et al. Live pig skin tissue and wound toxicity of cold plasma treatment. Plasma Med. (2011) 1:93–108. doi: 10.1615/PlasmaMed.v1.i1.80
140. Ndiaye, MA, Nihal, M, Wood, GS, and Ahmad, N. Skin, reactive oxygen species, and circadian clocks. Antioxid Redox Signal. (2014) 20:2982–96. doi: 10.1089/ars.2013.5645
141. Lee, N-E, Kang, Y-H, Song, S-Y, Baek, S-J, and Hwang, C-Y. Evaluation of cold atmospheric microwave plasma on skin physiological parameters and tolerability in dogs. Vet Dermatol. (2022) 33:363–70. doi: 10.1111/vde.13080
142. Li, G, Li, D, Li, J, Jia, Y-N, Zhu, C, Zhang, Y, et al. Promotion of the wound healing of in vivo rabbit wound infected with methicillin-resistant Staphylococcus aureus treated by a cold atmospheric plasma jet. IEEE Trans Plasma Sci. (2021) 49:2329–39. doi: 10.1109/TPS.2021.3092946
143. Schmidt, A, Bekeschus, S, Wende, K, Vollmar, B, and von Woedtke, T. A cold plasma jet accelerates wound healing in a murine model of full-thickness skin wounds. Exp Dermatol. (2017) 26:156–62. doi: 10.1111/exd.13156
144. Nakajima, Y, Mukai, K, Komatsu, E, Rahayu, HSE, Nur, M, Ishijima, T, et al. A simple technique to improve contractile effect of cold plasma jet on acute mouse wound by dropping water. Plasma Process Polym. (2015) 12:1128–38. doi: 10.1002/ppap.201400236
145. Alhabshan, R, Belyea, D, Stepp, M, Barratt, J, and Grewal, S. Effects of in-vivo application of cold atmospheric plasma on corneal wound healing in New Zealand white rabbits. Int J Ophthalmic Pathol. (2013) 2:2. doi: 10.4172/2324-8599.1000118
146. Ale-Ebrahim, M, Janani, E, and Mortazavi, P. The effect of cold argon plasma in atmospheric pressure on increasing blood coagulation speed and full-thickness cutaneous wound healing in rats. Vet Clin Pathol Q Sci J. (2018) 4:323–36.
147. Weltmann, K-D, Polak, M, Masur, K, von Woedtke, T, Winter, J, and Reuter, S. Plasma processes and plasma sources in medicine. Contrib Plasma Physics. (2012) 52:644–54. doi: 10.1002/ctpp.201210061
148. Metelmann, H-R, Seebauer, C, Miller, V, Fridman, A, Bauer, G, Graves, DB, et al. Clinical experience with cold plasma in the treatment of locally advanced head and neck cancer. Clin Plasma Med. (2018) 9:6–13. doi: 10.1016/j.cpme.2017.09.001
Keywords: cold plasma therapy, bacterial infections, veterinary medicine, antibiotic resistance, wound healing
Citation: Mohseni P, Ghorbani A and Fariborzi N (2023) Exploring the potential of cold plasma therapy in treating bacterial infections in veterinary medicine: opportunities and challenges. Front. Vet. Sci. 10:1240596. doi: 10.3389/fvets.2023.1240596
Edited by:
Linda A. Dahlgren, Virginia Maryland College of Veterinary Medicine, Virginia Tech, United StatesReviewed by:
Jessica Marie Gilbertie, Edward Via College of Osteopathic Medicine, United StatesNeelesh Sharma, Sher-e-Kashmir University of Agricultural Sciences and Technology of Jammu, India
Christoph Klinger, AniCura Tierklinik Stuttgart, Veterinary Specialist Hospital, Germany
Copyright © 2023 Mohseni, Ghorbani and Fariborzi. This is an open-access article distributed under the terms of the Creative Commons Attribution License (CC BY). The use, distribution or reproduction in other forums is permitted, provided the original author(s) and the copyright owner(s) are credited and that the original publication in this journal is cited, in accordance with accepted academic practice. No use, distribution or reproduction is permitted which does not comply with these terms.
*Correspondence: Abozar Ghorbani, YWJnaG9yYmFueUBhZW9pLm9yZy5pcg==