- 1Laboratory of Infectious Diseases, Department of Disease Control, Faculty of Veterinary Medicine, Hokkaido University, Sapporo, Japan
- 2Department of Advanced Pharmaceutics, Faculty of Veterinary Medicine, Hokkaido University, Sapporo, Japan
- 3Vaxxinova Japan K.K., Tokyo, Japan
- 4Department of Pharmacology and Parasitology, University of Veterinary Science, Nay Pyi Taw, Myanmar
- 5Department of Livestock and Aquaculture Research, Ministry of Agriculture, Livestock and Irrigation, Nay Pyi Taw, Myanmar
- 6International Affairs Office, Faculty of Veterinary Medicine, Hokkaido University, Sapporo, Japan
Introduction: Poultry red mites (PRMs, Dermanyssus gallinae), blood-sucking ectoparasites, are a threat to the poultry industry because of reduced production caused by infestation. In addition, tropical fowl mites (TFMs, Ornithonyssus bursa) and northern fowl mites (NFMs, Ornithonyssus sylviarum) are hematophagous, distributed in various regions, genetically and morphologically close to PRMs, and cause similar problems to the poultry industry. Vaccine approaches have been studied for PRM control, and several molecules have been identified in PRMs as candidates for effective vaccine antigens. The development of an anti-PRM vaccine as a universal vaccine with broad efficacy against avian mites could improve the productivity of poultry farms worldwide. Molecules that are highly conserved among avian mites and have critical functions in the physiology and growth of mites could be ideal antigen candidates for the development of universal vaccines. Ferritin 2 (FER2), an iron-binding protein, is critical for the reproduction and survival of PRMs and has been reported as a useful vaccine antigen for the control of PRMs and a candidate for the universal vaccine antigen in some tick species.
Method and results: Herein, we identified and characterized FER2 in TFMs and NFM. Compared with the sequence of PRM, the ferroxidase centers of the heavy chain subunits were conserved in FER2 of TFMs and NFMs. Phylogenetic analysis revealed that FER2 belongs to clusters of secretory ferritins of mites and other arthropods. Recombinant FER2 (rFER2) proteins from PRMs, TFMs, and NFMs exhibited iron-binding abilities. Immunization with each rFER2 induced strong antibody responses in chickens, and each immune plasma cross-reacted with rFER2 from different mites. Moreover, mortality rates of PRMs fed with immune plasma against rFER2 from TFMs or NFMs, in addition to PRMs, were higher than those of control plasma.
Discussion: rFER2 from each avian mite exhibited anti-PRM effects. This data suggests that it has the potential to be used as an antigen candidate for a universal vaccine against avian mites. Further studies are needed to access the usefulness of FER2 as a universal vaccine for the control of avian mites.
Introduction
The poultry red mite (PRM, Dermanyssus gallinae), is a harmful ectoparasite for poultry and is prevalent worldwide (1). Blood feeding by PRMs leads to reduced animal welfare and serious economic losses on poultry farms. Tropical fowl mites (TFMs, Ornithonyssus bursa) and northern fowl mites (NFMs, Ornithonyssus sylviarum) are ectoparasites of untamed birds (2), and are widely spread as key pests of poultry because of their introduction to farms via wild birds (3). Once invaded, these mites can persist long-term in farm facilities and poultry (4). PRMs feed on the host blood for a short period, mainly at night, and leave the hosts after blood feeding, residing in cracks and crevices for the rest of the time (5). In contrast, TFMs and NFMs are parasitic on the hosts throughout their life cycle (6). PRMs are widespread worldwide, with >46% of poultry farms in China and Japan (7) and 90% of the layer industry in Europe being affected (8). TFMs are cosmopolitan in tropical and subtropical countries (7, 9). Although the issues caused by NFMs are not uniform across the world, they are included as key pests in the poultry industry in North America, Brazil, Australia, and Asia (7, 10–12).
The success of acaricide treatment is hindered by the selection of mites resistant to acaricides owing to prolonged or improper application on farms (13). Because of the diminished efficacy of commercially available acaricides, they may have short residual action on mites. Consequently, the subclinical stages of mites or their eggs could enable a cycle of mite repopulation on farms (8). Some natural products, such as essential oils and plant derivatives, have been studied for their non-chemical acaricidal effects (14, 15). However, they may contain some active ingredients and may be harmful to humans and animals (16). Currently, vaccine-based control strategies are considered promising. Several recombinant protein-based anti-PRM vaccines have been reported by our research group (17–21) and other groups (22–25). Immunization with anti-PRM vaccines induces antigen-specific immunoglobulin (Ig)-Y in chickens, leading to reduced PRM survival in in vivo or in vitro studies. However, vaccine efficacy has not been sufficient for practical use in farms (23, 26). Therefore, the search for more effective antigens against PRMs is required, and antigens with broad protective efficacies across avian mites are more suitable for reducing the economic losses on poultry farms in various areas than those with limited protective efficacies.
For research on anti-tick vaccine development, molecules that are in direct contact with the host during blood feeding and are required to create an environment for blood feeding (exposed antigens) and molecules that are not exposed to the host and have essential physiological functions for the mites (concealed antigens) are considered vaccine candidates (27). Similar to the strategy of anti-tick vaccines, the development of anti-mite vaccines should focus on molecules involved in the key physiological functions of avian mites. As blood meal is the nutrient source for blood-sucking ectoparasites, including avian mites, the molecules involved in blood digestion and acquisition of essential nutrients could be suitable candidates for vaccine antigens with a broad spectrum across hematophagous avian mites. Iron is an essential nutrient for blood-feeding ectoparasites; however, the excessive presence of iron could be toxic. Therefore, iron homeostasis must be precisely controlled in the blood-feeding ectoparasites. Ferritin (FER), an iron-binding protein, is involved in iron homeostasis in most organisms (28). Two types of FER, FER1 and FER2, have been identified in ticks (29, 30), and both are fundamentally involved in blood feeding, reproduction, iron transport, and antioxidant defense (31). FER1 plays a role in intracellular iron storage and serves as an antioxidant by sequestering excess intracellular iron, whereas FER2 is a secreted ferritin that plays a role in the transportation of iron to peripheral tissues (29). In PRMs, two FERs have been identified, and their detrimental effects on survival, reproduction, and blood digestion have been demonstrated by RNA interference (RNAi) assays. Furthermore, both ferritins showed acaricidal potentials as vaccine antigens; importantly, the survival rate of PRMs fed with the plasma of chickens immunized with rFER2 (rDg-FER-1 in the original study) was significantly reduced compared with those of the rFER1-immunized group (rDgFER-2 in the original study) (25). Therefore, the potential of FER2 as a vaccine antigen has induced our interest in the development of a universal vaccine with broad-spectrum efficacy across mite species.
Development of a universal vaccine is of significant importance in veterinary practice. The successful application of universal vaccines offers cost-effectiveness by reducing the number of vaccine antigens, because there is no requirement to prepare species-specific antigens. A vaccine using Bm86, which has the potential to cross-react with different species of ticks, has been highlighted as a benefit to the livestock industry (32, 33). Additionally, glutathione S-transferase (34, 35), FER2 (27, 36), and subolesin (37) have been reported as vaccine antigen candidates for cross-species universal vaccines. However, the number of tick species for which the vaccine showed efficacy is limited and its effectiveness at various developmental stages has not yet been established (38). As for the control of avian mites, the development of vaccines against PRMs has progressed, whereas there are no reports of vaccine development against other avian mites, such as TFMs and NFMs, to the best of our knowledge. Therefore, the development of a cross-protective vaccine could be a sustainable management strategy for avian hematophagous mites on poultry farms, and it may save the economic losses on poultry farms and improve the cost-effectiveness of commercial production. Thus, in the present study, we aimed to investigate the potential of FER2 as a common antigen for developing a universal vaccine for avian mites. We identified the FER2 genes from TFMs and NFMs, evaluated the iron-binding ability of each rFER2, and investigated the cross-reactivity of immune plasmas against each rFER2 with the rFER2 of different mites to assess the acaricidal potential of immunization with rFER2 for avian mites. Additionally, the acaricidal effects on PRMs by the immune plasmas from TFMs and NFMs were assessed.
Material and methods
Sample availability, RNA extraction, and complementary DNA synthesis
PRMs were collected into a TubeSpin Bioreactor 600 bottle (TPP Techno Plastic Products AG, Trasadingen, Switzerland) from egg-laying farms contaminated with PRMs in Japan and transferred to the laboratory at 4°C. PRMs were kept at 25°C for a week without blood feeding, designated as starved PRMs, and stored at 5°C for further use. TFMs and NFMs, collected in the Republic of the Union of Myanmar (Burma), which were morphologically and genetically characterized in a previous study (39), were used for analysis in this experiment. Total RNA was extracted using TRIzol reagent (Invitrogen, Carlsbad, CA, USA), according to the manufacturer's instructions. Complementary DNA (cDNA) was synthesized from 1 μg of isolated RNA using PrimeScript Reverse Transcriptase (Takara Bio Inc., Shiga, Japan) and 200 pmol of oligo (dT)18 primer (Hokkaido System Science, Hokkaido, Japan). The synthesized cDNAs was treated with DNase I (Invitrogen) to remove unwanted DNA.
Identification of ferritin 2 genes from TFMs and NFMs
To determine the open reading frames (ORFs) of FER2 from TFMs and NFMs, partial segments were amplified using primers designed based on the conserved region of FER2 from Dermanyssus gallinae (HZ459285) and Varroa destructor (XM022808086). Primers used in this study are listed in Supplementary Table 1. The amplified fragments were cloned into a pMD20 vector (Takara Bio Inc.). Nucleotide sequences were analyzed using the CEQ GeXP automated sequencer (Beckman Coulter Inc., Brea, CA, USA). The primers used for the 3′ and 5′ RACE polymerase chain reaction (PCR) amplifications were designed based on the partial sequences of FER2. We conducted 3′ and 5′ RACE PCR using the RACE system (Invitrogen) according to the manufacturer's instructions. The PCR products were separated by agarose gel electrophoresis, purified, and cloned into the pGEMT-Easy vector (Promega, Madison, WI, USA).
Genetic characterization of FER2
Homologies of FER2 genes of TFMs and NFMs with reported sequences (Supplementary Table 2) from the National Center for Biotechnology Information gene bank were compared using the Basic Local Alignment Search Tool program. We constructed a phylogenetic tree using the nucleotide sequences of the FER2 genes of arthropods, including other mites, ticks, and chickens, and their sequences, using MEGA X software (40). These sequences were aligned using the MUSCLE (codon) option. A maximum-likelihood phylogenetic tree was constructed using the same software with 1,000 bootstrap replicates and a discrete gamma distribution (+G) to improve the tree topology.
Expression and purification of recombinant ferritin 2 proteins
The coding regions of FER2 genes were amplified with Taq polymerase (Takara Bio Inc.) using specific primers containing the sites of NdeI and XhoI for introduction into the pET19b vector (Merck & Co., Inc., Rahway, NJ, USA) (Supplementary Table 1). The amplified fragments were cloned into the pET19b vector (Merck) and transformed into Escherichia coli strain Rosetta-gami B (DE3, pLysS) (Merck). We generated N-terminal His-tagged rFER2 proteins of PRMs, TFMs, and NFMs using the E. coli expression system, termed as rFER2 PRM, rFER2 TFM, and rFER2 NFM, respectively. For rFER2 PRM generation, the reference sequence of FER2 (HZ459284) from Japan was used. Recombinant protein expression and purification were performed according to the manufacturer's instructions. The cell pellets were fractionated with BugBuster solution (Merck), and the insoluble fractions were solubilized in the buffer containing 0.3% N-lauroylsarcosine, 50-mM N-cyclohexyl-3-aminopropanesulfonic acid (CAPS) (Merck) (pH 11.0). Recombinant proteins were purified from insoluble fractions using Ni Sepharose™ 6 Fast Flow resin (GE Healthcare, Chicago, IL, USA) according to the manufacturer's instructions. The recombinant proteins were eluted with 0.3% N-lauroylsarcosine, 50-mM CAPS (pH 11.0) containing 250-mM imidazole (Nacalai Tesque, Tokyo, Japan). The purified FER2 proteins were refolded by dialysis against a 10-mM Tris-HCL (pH 8.5) buffer containing 0.1-mM DL-dithiothreitol (Merck) at 4°C overnight. The purity of the recombinant proteins was analyzed using 13% sodium dodecyl sulfate-polyacrylamide gel electrophoresis (SDS-PAGE) and stained with Coomassie brilliant blue (FUJIFILM Wako Pure Chemical Corporation, Osaka, Japan). The concentration of recombinant proteins was determined using the Pierce™ BCA Protein Assay Kit (Thermo Fisher Scientific, Waltham, MA, USA) with bovine serum albumin (BSA) as the standard, according to the manufacturer's instructions.
Iron binding assay
A ferrozine-based iron-binding assay was performed to analyze the iron-binding ability of rFER2 proteins (31). Different concentrations of rFER2 were dissolved in 954 μL of double distilled water and mixed with 20 μL of 1-M HEPES (pH 7.0) and 1 μL of 40-mM Fe2(NH4)2(SO4)2. After incubation at 30°C for 30 min, 20 μL of 10-mM ferrozine (Sigma-Aldrich, St. Louis, MO, USA) was added and incubated for 30 min. Each mixture (300 μL) was transferred to 3 wells of a microplate. The absorbance was measured at 570 nm using a microplate reader (Corona Electric, Hitachinaka, Japan). For the analyses, we used 2.5, 5, and 10 μg/mL of each rFER2 protein, apoferritin from equine spleen (Sigma-Aldrich) as the positive control, and BSA (Merck) as the negative control. All values were indicated as means, and error bars indicate standard deviations.
Immunization of chickens with rFER2 proteins
Sixteen chickens were randomly allocated to four groups: rFER2 PRM, rFER2 TFM, and rFER2 NFM immunization groups and the control group. To generate immune plasma, four chickens per group were subcutaneously immunized with 20 μg of each rFER2 mixed with the Freund incomplete adjuvant (FUJIFILM Wako Pure Chemical Corporation) at 3 weeks of age. The chickens were boosted at 3 weeks after the first immunization with 20 μg of recombinant proteins with the same adjuvant. As the control, four chickens were immunized with phosphate-buffered saline (PBS) and mixed with the same adjuvant. Three weeks after the second immunization, heparinized blood was collected from each chicken, and immune plasma was isolated.
Western blotting
Western blotting was performed to ascertain the antibody responses to each rFER2 vaccination and analyze the cross-reactivity of each immune plasma. The rFER2 proteins were electrophoresed on 13% SDS-PAGE gel and transferred onto polyvinylidene difluoride membranes (Merck). The membranes were blocked with 3% skim milk at 4°C overnight. Membranes were incubated with isolated immune plasma (1:1,000) at 25°C for 1 h and washed 3 times with PBST. The membranes were incubated with an anti-chicken IgY peroxidase rabbit antibody (1:10,000) (Sigma-Aldrich) at 25°C for 1 h and washed 3 times with PBST. Finally, the signal was detected using the Immobilon Western Chemiluminescent HRP Substrate (Merck).
Enzyme-linked immunosorbent assay
The enzyme-linked immunosorbent assay (ELISA) was performed to determine the antibody titers of the immune plasma against each rFER2. Briefly, 100 ng/well of each rFER2 protein was coated onto an ELISA plate (Sumitomo Bakelite Co. Ltd., Tokyo, Japan) with a carbon-bicarbonate buffer (pH 9.8) at 4°C overnight. The plate was washed 3 times with PBST and blocked with PBST-containing 1% BSA at 37°C for 2 h. After blocking, diluted immune plasma was added to each well and incubated at 25°C for 30 min. The wells were then washed with PBST 5 times and incubated with anti-chicken IgY[IgG](H+L)-HRP (goat; Bethyl Laboratories, Inc., Montgomery, TX, USA) at 37°C for 1 h. The reaction was detected by adding TMB One Component HRP Microwell Substrate (Bethyl Laboratories, Inc.) to each well, followed by incubation at 37°C for 15 min. After adding 100 μL of 0.18 M H2SO4 to stop the reaction, the absorbance was measured at 450 nm in a microplate reader (Corona Electric).
In vitro feeding assay
Fresh heparinized blood was collected from healthy chickens maintained at the Field Science Center for Northern Biosphere, Hokkaido University and incubated at 40°C before use. Plasma samples from chickens unimmunized or immunized with each rFER2 were pooled separately for each group. Plasma from heparinized fresh blood was replaced with each pool of plasma. Starved PRMs of mixed developmental stages were collected into in vitro feeding devices (17), and blood feeding was performed for 4 h at 40°C in dark and humid conditions with shaking at 100 rpm. Only blood-fed PRMs were collected using Pasteur pipettes and maintained at 25°C in 60% humidity throughout the monitoring period. The number of dead PRMs in each group was counted daily for a week, and the acaricidal effects of immune plasma against rFER2 were evaluated based on the survival rate of PRMs (the number of dead PRMs / the number of blood-fed PRMs).
Statistical analysis
In the iron-binding assay, statistical comparisons were performed using the Kruskal–Wallis test and the Steel–Dwass comparison test; asterisks indicate significant differences (*P < 0.05 and **P < 0.01). To compare PRM mortality between the immunized and control groups after in vitro feeding, we generated Kaplan–Meier curves and performed a log-rank test with Bonferroni corrections on multiple comparisons. Additionally, the Fisher exact test was performed to compare the mortality of PRMs between the groups on each day. All statistical analyses were performed using EZR, an easy-to-use software based on R and R commander (41). Moreover, the odds ratios and 95% confidence intervals were calculated. P < 0.01 for log-rank test and P < 0.05 for Fisher exact test were considered statistically significant.
Results
Identification and genetic characterization of the FER2 genes in TFMs and NFMs
The nucleotide sequences of the ORFs of FER2 genes from TFMs (LC752110) and NFMs (LC752111) consisted of 588 bp with 195 amino acids and signal peptides at positions 1–17. The FER2 genes of TFMs and NFMs showed 99.0% homology with each other and 65.0% homology with those of PRMs (Supplementary Figure 1; Table 1). In addition, the ferroxidase centers, which are iron-binding sites for the oxidation of Fe(II) in heavy-chain (H) ferritin, were completely conserved with those of PRMs. To genetically characterize the FER2 genes of TFMs and NFMs, we constructed a phylogenetic tree using the sequences of FER genes from mites, including PRMs, ticks, and chickens (Figure 1). The FER2 genes of TFMs and NFMs were distinctly classified into cluster 1, consisting of secreted ferritin (FER2) genes, and were most closely related to the secreted ferritin genes of PRM, Varroa destructor, and Tropilaelaps mercedesae in subcluster 1-1 consisting of secreted ferritin genes of mites. The subcluster 1-2 included secreted ferritin genes in ticks. Cluster 2 included intracellular ferritin (FER1) genes from PRMs and other mites, ticks, and chicken. Thus, FER2 genes of mites, including TFMs and NFMs, clearly belong to the secretory type of ferritins.
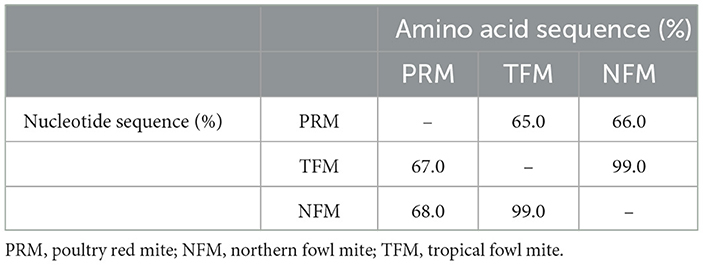
Table 1. Comparison of sequences of ferritin 2 from poultry red mites, northern fowl mites, and tropical fowl mites.
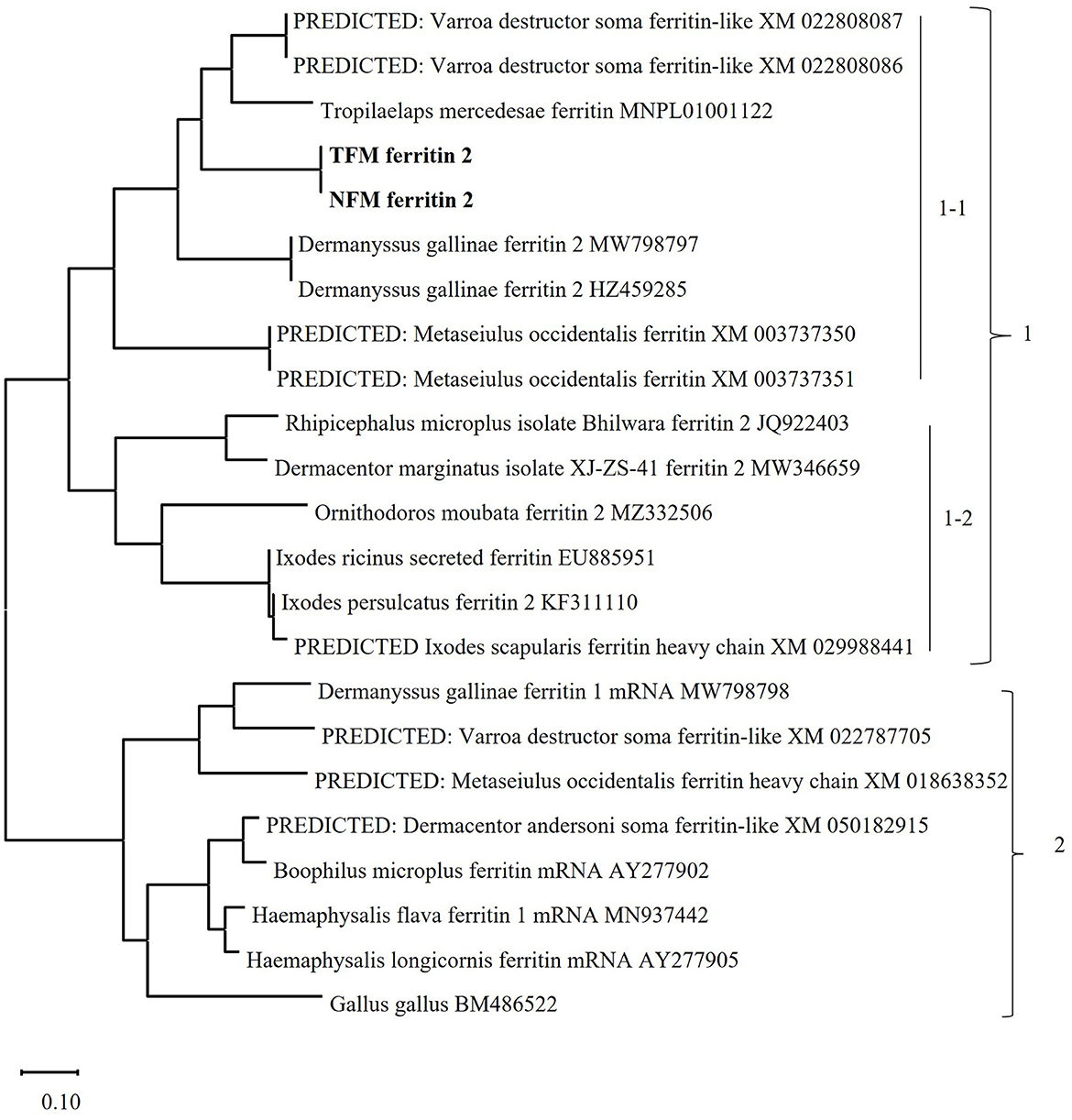
Figure 1. Phylogenetic analysis of the ferritin (FER) genes from poultry red mites (PRM), tropical fowl mite (TFM), northern fowl mite (NFM), arthropods, including other mites and ticks, and chickens. The phylogenetic tree was constructed using the maximum-likelihood method with MEGA X software. The numbers on the right indicate the clusters. Cluster 1 includes secretory types of FER2 genes, and is divided into 2 subclusters. Subcluster 1-1: The secretory types of FER2 genes of mites are classified into this subcluster, and the FER2 genes of PRMs, TFMs (bold), and NFMs (bold) belong to this cluster. Subcluster 1-2: This cluster consist of secretory types of FER2 genes from ticks. Cluster 2: The intracellular FER genes of mites, ticks, and chickens are classified into this cluster.
Iron binding ability of FER2 proteins of PRMs, TFMs, and NFMs
The whole regions of FER2 from PRMs, TFMs, and NFMs, excluding the signal peptides, were generated as recombinant proteins fused with His-tag, termed as rFER2 TFM, rFER2 NFM, and rFER2 PRM. The recombinant proteins were purified from the insoluble fractions by affinity chromatography, and their purities were confirmed by SDS-PAGE and Western blotting (Figures 2A, B). To assess whether each rFER2 has iron-binding ability, we conducted a ferrozine-based colorimetric assay. The absorbance of rFER2 proteins in PRMs, TFMs, and NFMs decreased in a dose-dependent manner, similar to that of apoferritin, the positive control (Figure 3). Thus, these results showed that rFER2 proteins of PRMs, TFMs, and NFMs have iron-binding abilities.
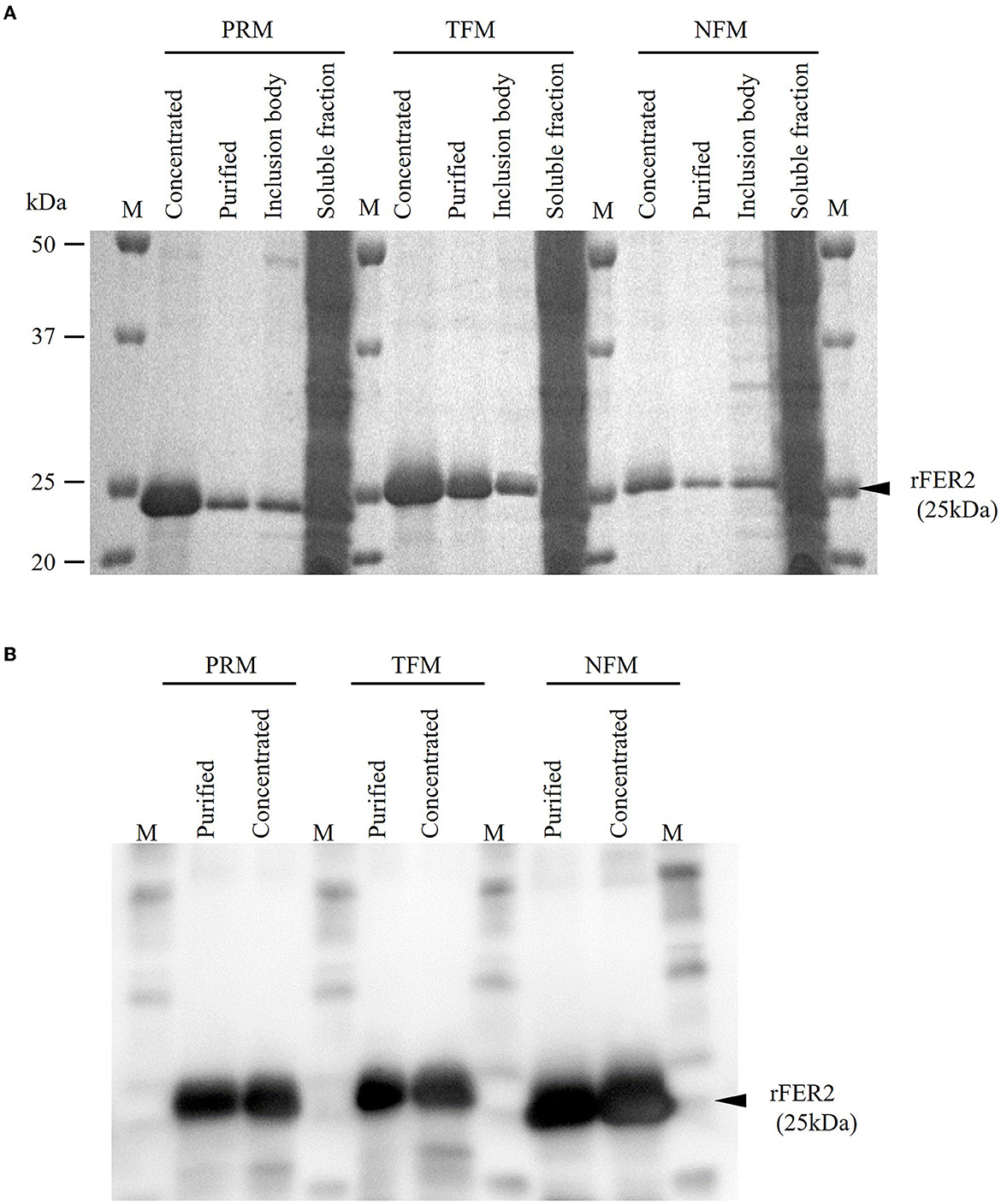
Figure 2. Expression and purification of recombinant ferritin 2 (rFER2) proteins. The ferritin 2 from poultry red mites (PRMs), tropical fowl mite (TFMs), and northern fowl mites (NFMs) were expressed and purified as recombinant proteins fused with histidine tag, and named as rFER2 PRM, rFER2 TFM, and rFER2 NFM, respectively. rFER2 from each mite was expressed in Escherichia coli and purified from the inclusion body fraction by affinity chromatography. The purity of rFER2 was confirmed by sodium dodecyl sulfate-polyacrylamide gel electrophoresis (A) and Western blotting (B). M, Marker (Precision Plus Protein™ All Blue Prestained Protein Standards; Bio-Rad, Hercules, CA, USA).
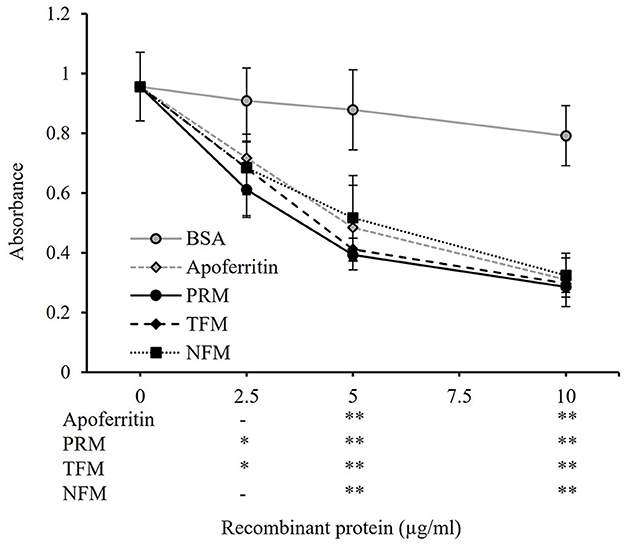
Figure 3. Iron-binding ability of recombinant ferritin 2 (rFER2) proteins. The iron-binding ability of rFER2 proteins from poultry red mite, tropical fowl mite, and northern fowl mite was assessed by a ferrozine-based colorimetric assay using different concentrations of each rFER2. Ferrozine was used as an indicator agent. Bovine serum albumin and horse apoferritin were used as the negative and positive controls, respectively. The x-axis indicates the amounts of rFER2 proteins used in this assay. Error bars indicate standard deviations. Statistical differences are shown as a comparison to bovine serum albumin (BSA). Asterisks indicate significant differences (*P < 0.05 and **P < 0.01).
Cross-reactivities of antibodies produced by the immunization with rFER2
To examine the potential of rFER2 as a universal vaccine antigen against avian mites, immune plasma was isolated from chickens immunized with each rFER2. As shown in Table 2, increased production of antibodies in the immunized groups was confirmed. Western blotting revealed the presence of chicken IgY specific to rFER2 in the immune plasma (Figure 4). Additionally, the immune plasma against each rFER2 reacted with all rFER2 proteins, including those from different mites. However, the intensity of the signals was slightly different when the reactivity of immune plasma against rFER2 PRM was compared between rFER2 PRM and those of TFMs and NFMs, and vice versa. These results suggest that rFER2s have potential as common vaccine antigens in avian mites.
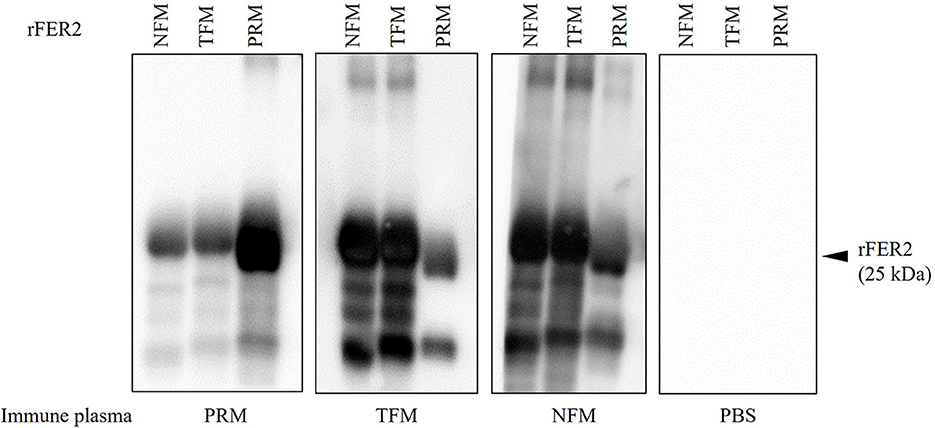
Figure 4. The production of specific antibodies in the plasma from chickens immunized with recombinant ferritin 2 (rFER2) proteins. Four chickens per group were immunized with rFER2, separately. The plasmas were isolated from each immunized chicken, and the production of antibodies specific to rFER2 in the plasma were detected by Western blotting. The cross-reactivities of immune plasmas with each rFER2 were tested by Western blotting. The arrowhead indicates the predicted molecular weight of rFER2 (~25 kDa).
Assessment of acaricidal activity of the plasmas from chickens immunized with each rFER2
To assess the acaricidal effects of each FER2, we performed in vitro feeding assays and monitored the mortality of PRMs fed immune plasmas against each FER2. In this study, we used PRMs for in vitro feeding assays because of the limited distribution of NFMs and the absence of TFMs in Japan. To examine the acaricidal effects, we compared mortality between the immunized and control groups using the Fisher exact and log-rank tests. In this study, we used pooled plasma of chickens from each immunized group and found that the antibody titer of each group was different. According to a previous report, the acaricidal effects could depend on the antibody titer (21). Therefore, we only compared the mortality between the control group and each immunized group. The in vitro feeding assays were performed twice. In experiment 1, the mortality rate of PRMs reached 40.55, 32.03, and 39.34% in immunized groups of rFER2 PRM, rFER2 TFM, and rFER2 NFM, respectively, at 7 days post-feeding; moreover, according to the Fisher exact test, we observed significant differences in the mortality of PRMs fed the immune plasma against rFER2 PRM at 2–7 days post-feeding, and 3–7 days post-feeding in the immunized group of rFER2 TFM and rFER2 NFM, compared with those of the control group (Table 3). Kaplan–Meier curves revealed that the survival rate of PRMs fed with the immune plasma against each rFER2 was significantly lower than that of the control group (Figure 5A). Similar results were recorded in experiment 2. The survival rate of PRMs fed with immune plasmas against each rFER2 was significantly decreased (Figure 5B), and significant differences in the mortality rates were observed within 3–7 days post-feeding in the immunized groups of rFER2 PRM and rFER2 NFM and within 4–7 days post-feeding in the immunized group of rFER2 TFM (Table 4). Thus, the immune plasma against each rFER2 exhibited acaricidal effects on PRMs. Therefore, rFER2 could be a candidate antigen for the development of a universal vaccine across avian mites.
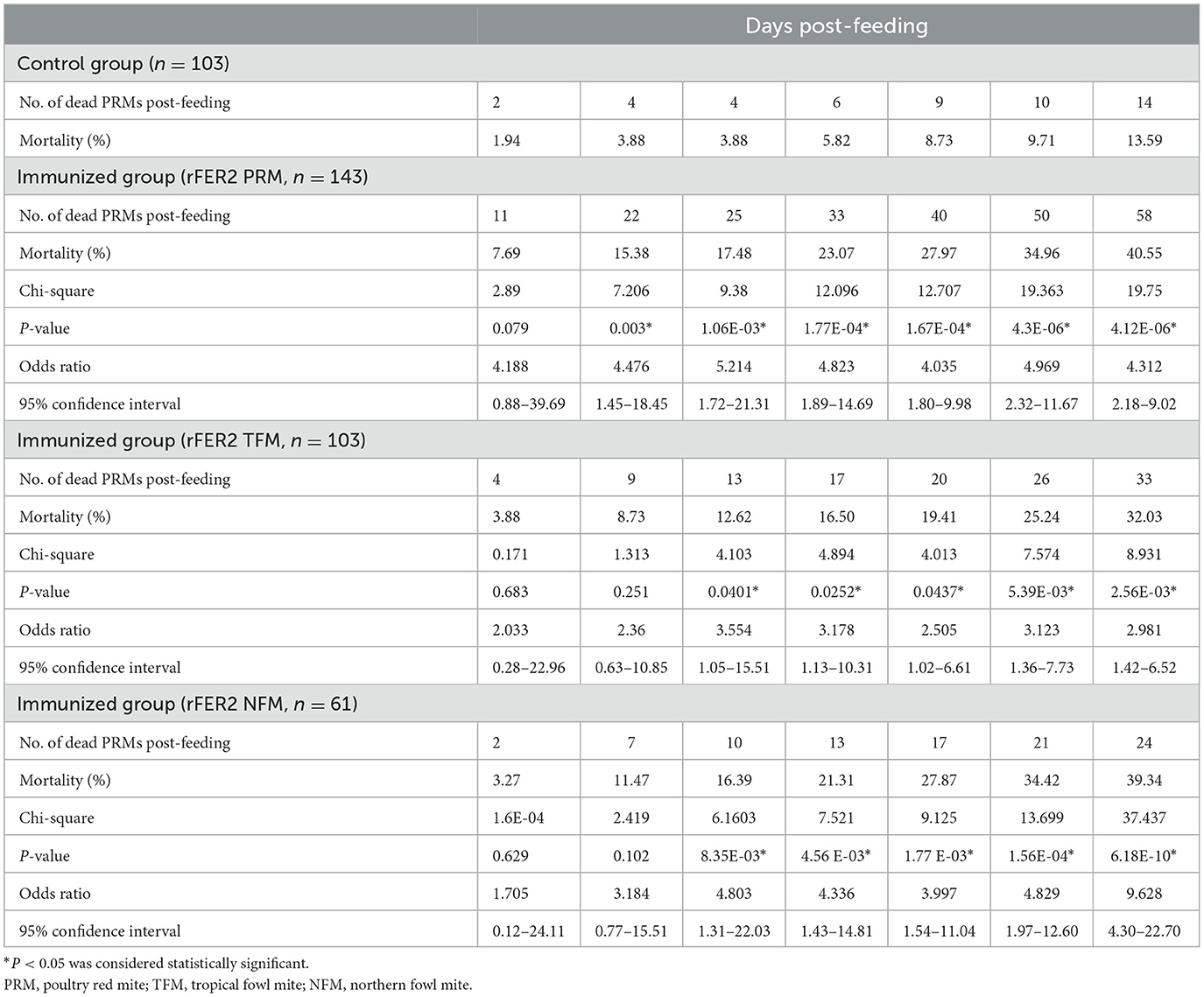
Table 3. Mortality of PRMs fed plasma from chickens immunized with recombinant ferritin 2 from different species of mites (experiment 1).
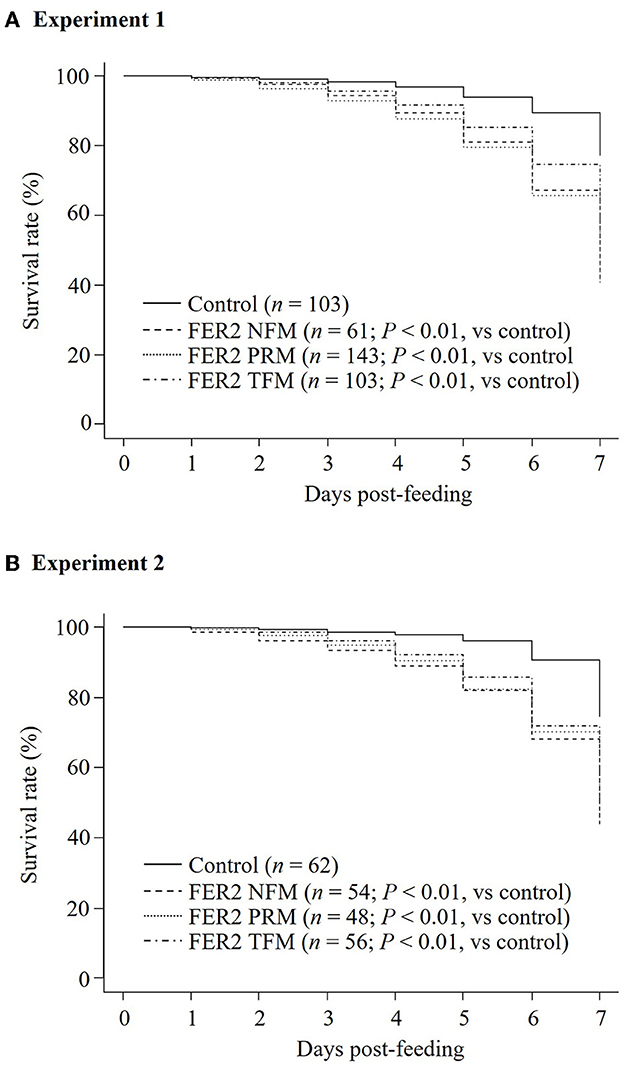
Figure 5. Assessment of the acaricidal potential of plasma obtained from chickens immunized with recombinant ferritin 2 (rFER2) proteins. Artificial blood feeding to poultry red mite (PRMs) was performed by the in vitro feeding assay. The survival rates of PRMs fed with immune plasmas or control plasma were monitored daily for a week. The in vitro feeding assays were performed 2 times. The total number of PRMs used in this study is as follows: (A) experiment 1: fed with immune plasma: n = 143 (rFER2 PRM), n = 103 [rFER2 tropical fowl mite (TFM)], and n = 61 [rFER2 northern fowl mite (NFM)] and fed with control plasma: n = 103; (B) experiment 2: fed with immune plasma: n = 48 (rFER2 PRM), n = 56 (rFER2 TFM), and n = 54 (rFER2 NFM) and fed with control plasma: n = 62. The number of dead PRMs were recorded and plotted on the graph to generate Kaplan–Meier survival curves. Statistical analysis was performed using a log-rank test with Bonferroni corrections on multiple comparisons. P < 0.01 was considered statistically significant between each immunized and control group.
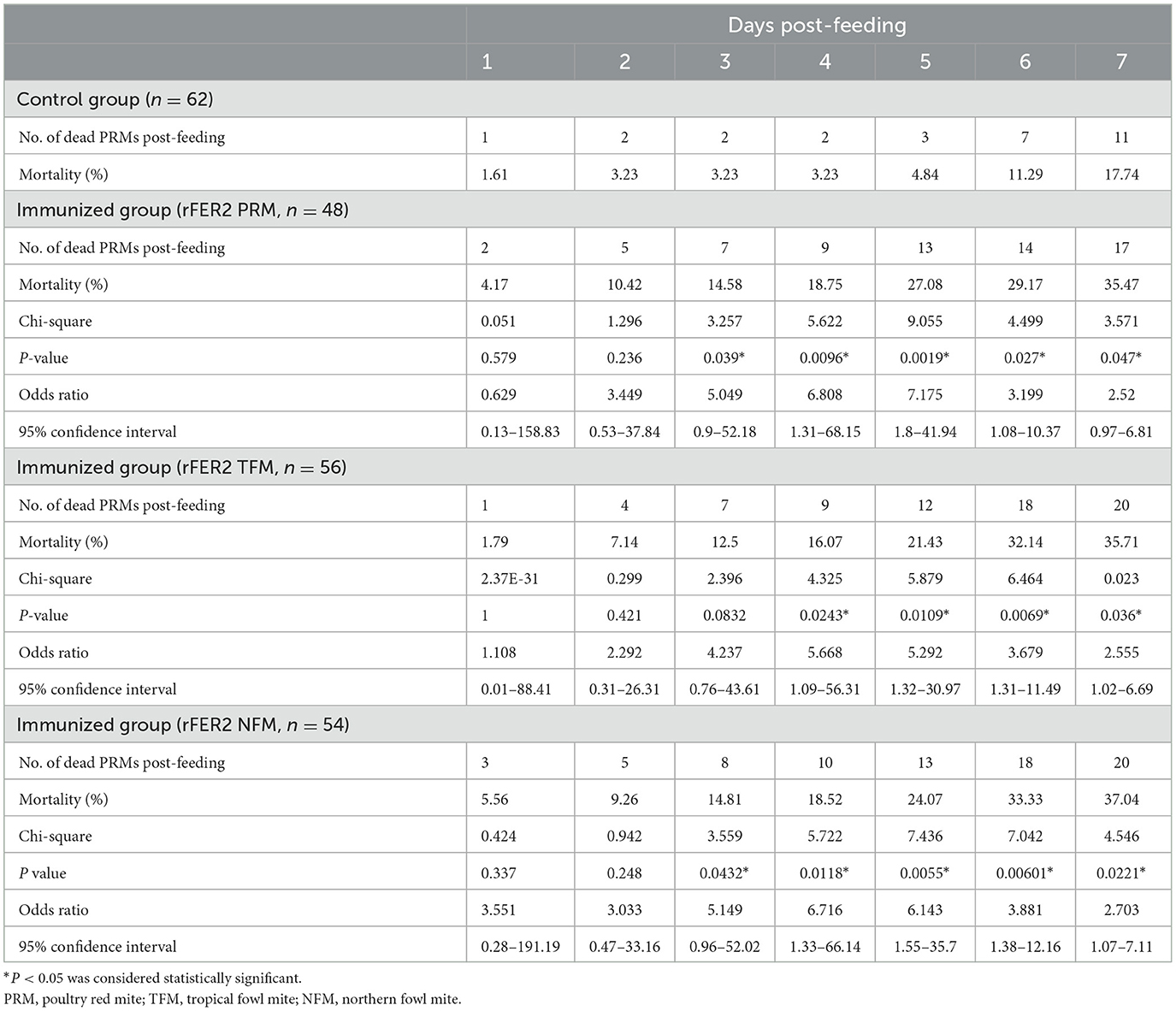
Table 4. Mortality of PRMs fed plasma from chickens immunized with recombinant ferritin 2 from different species of mites (experiment 2).
Discussion
Vaccine approaches have been focused on as a method for controlling PRMs to overcome the diminished effectiveness of acaricides and the selection of acaracide-resistant mites on poultry farms, in addition to their cost-effectiveness, low toxicity to the environment, and long-lasting action (42). However, other hematophagous mites, TFMs and NFMs, which are genetically similar to PRMs, cause problems similar to those of PRMs on poultry farms. Therefore, the development of a vaccine with broad protection efficacy against these avian mites could be a promising approach for their control in the poultry industry. The molecules involved in the critical physiological functions of avian mites are suitable vaccine antigens. FER2 is involved in iron transport and is critical for blood feeding and reproduction in ticks; moreover, FER2 is highly immunogenic and a useful candidate for anti-tick vaccines with broad protective efficacy across some tick species (27, 30, 43). FER2 has also been reported as a crucial molecule for the survival, reproduction, and blood digestion of PRMs. Moreover, acaricidal effects on PRMs by immunization have been demonstrated (25). Herein, we genetically identified and characterized FER2 genes from TFMs and NFMs. In addition, the recombinant FER2 proteins from PRMs, TFMs, and NFMs were shown to be iron-binding proteins. Moreover, immune plasmas against each rFER2 showed cross-reactivity with rFER2 of different mites and acaricidal effects on PRMs, even when we used immune plasmas against rFER2 of TFMs and NFMs. Collectively, FER2 could be used as a vaccine antigen with protective efficacy against avian mites.
Genetic analysis showed that the FER2 genes of PRMs, TFMs, and NFMs belonged to the cluster of secretory ferritins and were distinct from the cluster of intracellular ferritins. The secretory FER2 proteins of avian mites include signal peptides at the N-terminus. Similar to ferritins in vertebrates, ferritins of insects consist of H subunits containing ferroxidase centers (iron-binding sites) and light-chain (L) subunits containing amino acid residues with ferrihydrite nucleation centers (44). In this study, the ferroxidase centers of FER2 of avian mites were completely conserved, showing H-type subunits that are highly functional for catalytic activity in Fe(II) oxidation. We also observed the iron-binding abilities of rFER2-PRM, rFER2-TFM, and rFER2-NFM. Two types of ferritins have been identified in ticks (31, 45), and unlike FER1, FER2 has been recognized as a secretory protein in the tick hemolymph (29). Two types of ferritin have been identified in PRMs (25). The FER2 gene of PRMs is expressed in all developmental stages, and RNAi analysis revealed critical functions for survival and reproduction (25). Unfortunately, in the present study, we could not elucidate the expression patterns of the FER2 genes in the developmental stages of TFMs and NFMs and could not perform RNAi analysis on TFMs and NFMs because of the limited availability of TFM and NFM samples in Japan. However, we confirmed that the immune plasma against rFER2 of TFMs and NFMs cross-reacted with PRM rFER2 and exhibited anti-PRM effects. Collectively, these data suggest that the FER2 proteins identified in this study are secretory ferritins and have essential roles in the physiology of avian mites.
Host blood is an essential source of nutrients required for the growth and reproduction of hematophagous ectoparasites. During a single feed, a PRM can suck ~0.2 μL of the host blood (16) and is exposed to a large amount of iron. Excessive exposure to non-heme iron after hemoglobin digestion in midguts could be toxic to mites. Similar to the mechanisms in ticks (46), FERs are considered to play a role in iron homeostasis, although iron metabolism remains poorly understood in avian mites. According to a previous report, silencing of FER1 and FER2 affects feeding and oviposition in ticks, and FER2 depletion is linked to FER1 expression and altered iron homeostasis in ticks (31). Therefore, FER2 has been targeted as an effective vaccine candidate for ticks (27, 30, 43). Knockdown of FER1 and FER2 led to decreased blood digestion and oviposition, and increased mortality in PRMs; moreover, a significant increase in mortality of PRMs were recorded by immunization of chickens with rFER2 (rDg-FER1 in the original study) (25). In the present study, we observed the anti-PRM effects of rFER2 from TFMs and NFMs, and all immune plasmas cross-reacted with FER2 proteins of different mites. Therefore, these findings suggest the usefulness of FER2 as a vaccine antigen against TFMs and NFMs, and highlight FER2 as a candidate for the development of a universal vaccine against avian mites.
The development of a cross-protective vaccine for multi-tick species has been emphasized because of the non-uniform distribution of ticks worldwide. Cattle can get infected by various ticks due to their preferences, and the usefulness of common antigens with broad protective efficacy against different ticks has been reported (27, 30, 43, 47). Our research group has introduced a similar concept for avian mites, which poses a serious problem to the poultry industry. In addition to the development of anti-PRM vaccines, we are extending our work to determine the usefulness of anti-PRM vaccines for different avian mites. In this study, the immune plasma of chickens against each rFER2 cross-reacted with rFER2 proteins of different mites, and immune plasma against TFMs and NFMs showed acaricidal effects on PRMs by in vitro feeding. The development of a universal vaccine could be cost-effective for commercial production. The potential application of this kind of vaccine in poultry farms could prevent economic losses in production. However, there is a limitation in evaluating the acaricidal effects on TFMs and NFMs. To demonstrate the acaricidal effects of immune plasmas on TFMs and NFMs, in vitro assays using TFMs and NFMs for the assessment of vaccine antigens must be established, and challenge trials on chickens immunized with each mite are required to develop an effective universal vaccine for controlling avian hematophagous mites. Moreover, it is difficult to assess if immunization with rFER2 contributes to the improved economics based on the in vitro data; therefore, field trials are required to precisely evaluate the impact of vaccination with FER2. In addition, the search for other common antigens and the combined use of multiple antigens as a cocktail vaccine could further enhance the acaricidal effects on PRMs, TFMs, and NFMs.
Conclusions
In the present study, we characterized FER2 from PRMs, TFMs, and NFMs and investigated its acaricidal effects as a vaccine antigen to assess the potential application of a universal vaccine across avian mites. The amino acid residues crucial for the oxidization of Fe(II) were conserved among the three species, and all rFER2 proteins tested showed iron-binding ability. Most importantly, the immune plasmas against rFER2 of PRMs, TFMs, and NFMs cross-reacted with rFER2 from different mites and exhibited acaricidal effects on PRMs, even in assays using immune plasmas against rFER2 of TFMs and NFMs. Thus, FER2 may be a useful vaccine antigen for avian mites. Further studies are needed to assess the usefulness of FER2 as a universal vaccine against avian mites.
Data availability statement
The original contributions presented in the study are included in the article/Supplementary material, further inquiries can be directed to the corresponding author.
Ethics statement
All animal experiments were performed in accordance with the guidelines and regulations of the Faculty of Veterinary Medicine, Hokkaido University, which is fully accredited by the Association for Assessment and Accreditation of Laboratory Animal Care International, and approved by the Institutional Animal Care and Use Committee of Hokkaido University (approval number: 20–0051).
Author contributions
SW and SM conceived of and designed the study, analyzed the data, and drafted the manuscript. SW, SF, HS, JS, YM, LH, and SB conducted the experiments. SM, TO, NM, TS, EO, AT, SK, and KO provided intellectual input, laboratory materials, reagents, and analytical tools. All authors have reviewed and approved the final manuscript.
Funding
This research was supported in part by Grants-in-Aid for Scientific Research (B: 18H02332 and B: 20H03137) and a Grant-in-Aid for Challenging Research (Exploratory) (20K21357) from the Japan Society for the Promotion of Science.
Acknowledgments
We thank all poultry farmers, Dr. Wataru Hashimoto, Japan Layer K.K. (Gifu, Japan), and Dr. Akio Enya for their assistance in sample collection. We also thank Editage (www.editage.jp) for English language editing.
Conflict of interest
TS, EO, and AT were employed by Vaxxinova Japan K.K, Tokyo, Japan. SM, SK, and KO are authors of the patent-covering materials and techniques described in this manuscript (EU patent, EP15800403.6; Japanese patent, 2016-523581).
The remaining authors declare that the research was conducted in the absence of any commercial or financial relationships that could be construed as a potential conflict of interest.
Publisher's note
All claims expressed in this article are solely those of the authors and do not necessarily represent those of their affiliated organizations, or those of the publisher, the editors and the reviewers. Any product that may be evaluated in this article, or claim that may be made by its manufacturer, is not guaranteed or endorsed by the publisher.
Supplementary material
The Supplementary Material for this article can be found online at: https://www.frontiersin.org/articles/10.3389/fvets.2023.1182930/full#supplementary-material
References
1. Sparagano OAE, George DR, Harrington DWJ, Giangaspero A. Significance and control of the poultry red mite, Dermanyssus gallinae. Annu Rev Entomol. (2014) 59:447–66. doi: 10.1146/annurev-ento-011613-162101
2. Knee W, Proctor H. Host records for Ornithonyssus sylviarum (Mesostigmata: Macronyssidae) from birds of North America (Canada, United States and Mexico). J Med Entomol. (2007) 44:709–13. doi: 10.1093/jmedent/44.4.709
3. Wood HP. Tropical Fowl Mite in the United States: With Notes on Life History and Control. Washington, DC: US Dept of Agriculture (1920).
4. McCulloch JB, Owen JP, Hinkle NC, Mullens BA, Busch JB. Genetic structure of northern fowl mite (Mesostigmata: Macronyssidae) populations among layer chicken flocks and local house sparrows (Passeriformes: Passeridae). J Med Entomol. (2020) 57:122–30. doi: 10.1093/jme/tjz136
5. Chauve C. The poultry red mite Dermanyssus gallinae (De Geer, 1778): current situation and future prospects for control. Vet Parasitol. (1998) 79:239–45. doi: 10.1016/S0304-4017(98)00167-8
6. Chen BL, Mullens BA. Temperature and humidity effects on off-host survival of the northern fowl mite (Acari: Macronyssidae) and the chicken body louse (Pthiraptera: Menoponidae). J Econ Entomol. (2008) 101:637–46. doi: 10.1093/jee/101.2.637
7. Sparagano O, Ho J. Parasitic mite fauna in Asian poultry farming systems. Front Vet Sci. (2020) 7:400. doi: 10.3389/fvets.2020.00400
8. Sigognault Flochlay A, Thomas E, Sparagano O. Poultry red mite (Dermanyssus gallinae) infestation: a broad impact parasitological disease that still remains a significant challenge for the egg-laying industry in Europe. Parasit Vect. (2017) 10:357. doi: 10.1186/s13071-017-2292-4
9. Murthy GSS, Panda R. Prevalence of Dermanyssus and Ornithonyssus species of mites in poultry farms of Vikarabad area of Hyderabad. J Parasit Dis. (2016) 40:1372–5. doi: 10.1007/s12639-015-0693-x
10. Jansson DS, Otman F, Lundqvist L, Höglund J, Engström A, Chirico J. Northern fowl mite (Ornithonyssus sylviarum) in Sweden. Med Vet Entomol. (2014) 28:443–6. doi: 10.1111/mve.12053
11. Soares NM, Tucci EC, Guastalli, Yajima, H. Control of Orinthonyssus sylviarum (Canestrini and Fanzago, 1877) (Acari: Macronyssidae) infestation in commercial laying hens by using Azadirachta indica extract. Rev Bras Parasitol Vet. (2008) 17:175–8. doi: 10.1590/S1984-29612008000400001
12. Watson CR. Human infestation with bird mites in Wollongong. Commun Dis Intell Q Rep. (2003) 27:259–61.
13. Marangi M, Cafiero MA, Capelli G, Camarda A, Sparagano OAE, Giangaspero A. Evaluation of the poultry red mite, Dermanyssus gallinae (Acari: Dermanyssidae) susceptibility to some acaricides in field populations from Italy. Exp Appl Acarol. (2009) 48:11–8. doi: 10.1007/s10493-008-9224-0
14. Kim SI Yi JH, Tak J, Ahn YJ. Acaricidal activity of plant essential oils against Dermanyssus gallinae (Acari: Dermanyssidae). Vet Parasitol. (2004) 120:297–304. doi: 10.1016/j.vetpar.2003.12.016
15. Tomer H, Blum T, Arye I, Faigenboim A, Gottlieb Y, Ment D. Activity of native and commercial strains of Metarhizium spp. against the poultry red mite Dermanyssus gallinae under different environmental conditions. Vet Parasitol. (2018) 262:20–5. doi: 10.1016/j.vetpar.2018.09.010
16. Mul M, van Niekerk T, Chirico J, Maurer V, Kilpinen O, Sparagano O, et al. Control methods for Dermanyssus gallinae in systems for laying hens: Results of an international seminar. Worlds Poult Sci J. (2009) 65:589–600. doi: 10.1017/S0043933909000403
17. Ariizumi T, Murata S, Fujisawa S, Isezaki M, Sato T, Oishi E, et al. In vitro evaluation of a cysteine protease from poultry red mites, Demanyssus gallinae, as a vaccine antigen for chickens. Poult Sci. (2021) 101:101638. doi: 10.1016/j.psj.2021.101638
18. Fujisawa S, Murata S, Isezaki M, Ariizumi T, Sato T, Oishi E, et al. Characterization of a novel cysteine protease inhibitor from poultry red mites: potential vaccine for chickens. Vaccines. (2021) 9:1472. doi: 10.3390/vaccines9121472
19. Fujisawa S, Murata S, Takehara M, Aoyama J, Morita A, Isezaki M, et al. In vitro characterization of adipocyte plasma membrane-associated protein from poultry red mites, Dermanyssus gallinae, as a vaccine antigen for chickens. Vaccine. (2021) 39:6057–66. doi: 10.1016/j.vaccine.2021.08.104
20. Fujisawa S, Murata S, Isezaki M, Ariizumi T, Sato T, Oishi E, et al. Characterization of copper transporter 1 from Dermanyssus gallinae as a vaccine antigen. Parasitology. (2022) 149:105–15. doi: 10.1017/S0031182021001608
21. Murata S, Taniguchi A, Isezaki M, Fujisawa S, Sakai E, Taneno A, et al. Characterisation of a cysteine protease from poultry red mites and its potential use as a vaccine for chickens. Parasite. (2021) 28:9. doi: 10.1051/parasite/2021005
22. Bartley K, Huntley JF, Wright HW, Nath M, Nisbet AJ. Assessment of cathepsin D and L-like proteinases of poultry red mite, Dermanyssus gallinae (De Geer), as potential vaccine antigens. Parasitology. (2012) 139:755–65. doi: 10.1017/S0031182011002356
23. Bartley K, Wright HW, Huntley JF, Manson ED, Inglis NF, McLean K, et al. Identification and evaluation of vaccine candidate antigens from the poultry red mite (Dermanyssus gallinae). Int J Parasitol. (2015) 45:819–30. doi: 10.1016/j.ijpara.2015.07.004
24. Price DRG, Kuster T, Qines Q, Oliver EM, Bartley K, Nunn F, et al. Evaluation of vaccine delivery systems for inducing long-lived antibody responses to Dermanyssus gallinae antigen in laying hens. Avian Pathol. (2019) 48:60–74. doi: 10.1080/03079457.2019.1612514
25. Xu X, Wang C, Liu Q, Huang Y, Sun W, Pan B. Two ferritins from Dermanyssus gallinae: characterization and in vivo assessment as protective antigens. Pest Manag Sci. (2022) 78:561–71. doi: 10.1002/ps.6664
26. Harrington D, Canales M, de la Fuente J, Robinson K, Guy J, Sparagano O. Immunisation with recombinant proteins subolesin and Bm86 for the control of Dermanyssus gallinae in poultry. Vaccine. (2009) 27:4056–63. doi: 10.1016/j.vaccine.2009.04.014
27. Githaka NW, Konnai S, Isezaki M, Goto S, Xavier MA, Fujisawa S, et al. Identification and functional analysis of ferritin 2 from the Taiga tick Ixodes persulcatus Schulze. Ticks Tick Borne Dis. (2020) 11:101547. doi: 10.1016/j.ttbdis.2020.101547
28. Arosio P, Ingrassia R, Cavadini P. Ferritins: a family of molecules for iron storage, antioxidation and more. Biochim Biophys Acta. (2009) 1790:589–99. doi: 10.1016/j.bbagen.2008.09.004
29. Hajdusek O, Sojka D, Kopacek P, Buresova V, Franta Z, Sauman I, et al. Knockdown of proteins involved in iron metabolism limits tick reproduction and development. Proc Natl Acad Sci USA. (2009) 106:1033–8. doi: 10.1073/pnas.0807961106
30. Hajdusek O, Almazán C, Loosova G, Villar M, Canales M, Grubhoffer L, et al. Characterization of ferritin 2 for the control of tick infestations. Vaccine. (2010) 28:2993–8. doi: 10.1016/j.vaccine.2010.02.008
31. Galay RL, Aung KM, Umemiya-Shirafuji R, Maeda H, Matsuo T, Kawaguchi H, et al. Multiple ferritins are vital to successful blood feeding and reproduction of the hard tick Haemaphysalis longicornis. J Exp Biol. (2013) 216:1905–15. doi: 10.1242/jeb.081240
32. García-García JC, Gonzalez IL, González DM, Valdés M, Méndez L, Lamberti J, et al. Sequence variations in the Boophilus microplus Bm86 locus and implications for immunoprotection in cattle vaccinated with this antigen. Exp Appl Acarol. (1999) 23:883–95. doi: 10.1023/A:1006270615158
33. García-García JC, Montero C, Redondo M, Vargas M, Canales M, Boue O, et al. Control of ticks resistant to immunization with Bm86 in cattle vaccinated with the recombinant antigen Bm95 isolated from the cattle tick, Boophilus microplus. Vaccine. (2000) 18:2275–87. doi: 10.1016/S0264-410X(99)00548-4
34. Parizi LF, Utiumi KU, Imamura S, Onuma M, Ohashi K, Masuda A, et al. Cross immunity with Haemaphysalis longicornis glutathione S transferase reduces an experimental Rhipicephalus (Boophilus) microplus infestation. Exp Parasitol. (2011) 127:113–8. doi: 10.1016/j.exppara.2010.07.001
35. Sabadin GA, Parizi LF, Kiio I, Xavier MA, Matos RS, Camargo-Mathias MI, et al. Effect of recombinant glutathione S-transferase as vaccine antigen against Rhipicephalus appendiculatus and Rhipicephalus sanguineus infestation. Vaccine. (2017) 35:6649–56. doi: 10.1016/j.vaccine.2017.10.026
36. Xavier MA, Konnai S, Parizi LF, Githaka NW, Isezaki M, Goto S, et al. Cross-species reactivity of antibodies against Ixodes persulcatus ferritin 2 to Rhipicephalus microplus. Jpn J Vet Res. (2021) 69:57–65. doi: 10.14943/jjvr.69.1.57
37. Kasaija PD, Contreras M, Kabi F, Mugerwa S, de la Fuente J. Vaccination with recombinant subolesin antigens provides cross-tick species protection in Bos indicus and crossbred cattle in Uganda. Vaccines. (2020) 18:319. doi: 10.3390/vaccines8020319
38. Parizi LF, Githaka NW, Logullo C, Konnai S, Masuda A, Ohashi K, et al. The quest for a universal vaccine against ticks: cross-immunity insights. Vet J. (2012) 194:158–65. doi: 10.1016/j.tvjl.2012.05.023
39. Takehara H, Murata S, Katakura K, Fujisawa S, Hmoon MM, Win SY, et al. Haematophagous mites on poultry farms in the Republic of the Union of Myanmar. Heliyon. (2019) 5:e01544. doi: 10.1016/j.heliyon.2019.e01544
40. Kumar S, Stecher G, Li M, Knyaz C, Tamura K, MEGA X. Molecular evolutionary genetics analysis across computing platforms. Mol Biol Evol. (2018) 35:1547–9. doi: 10.1093/molbev/msy096
41. Kanda Y. Investigation of the freely available easy-to-use software ‘EZR' for medical statistics. Bone Marrow Transplant. (2013) 48:452–8. doi: 10.1038/bmt.2012.244
42. Wright HW, Bartley K, Huntley JF, Nisbet AJ. Characterisation of tropomyosin and paramyosin as vaccine candidate molecules for the poultry red mite, Dermanyssus gallinae. Parasite Vector. (2016) 12:544. doi: 10.1186/s13071-016-1831-8
43. Oleaga A, González-Pérez S, Peréz-Sánchez R. First molecular and functional characterisation of ferritin 2 proteins from Ornithodoros argasid ticks. Vet Parasitol. (2022) 304:109684. doi: 10.1016/j.vetpar.2022.109684
44. Pham DQD, Winzerling JJ. Insect ferritins: typical or atypical? Biochim Biophys Acta. (2010) 1800:824–33. doi: 10.1016/j.bbagen.2010.03.004
45. Kopácek P, Zdychová J, Yoshiga T, Weise C, Rudenko N, Law JH. Molecular cloning, expression and isolation of ferritins from two tick species-Ornithodoros moubata and Ixodes ricinus. Insect Biochem Mol Biol. (2003) 33:103–13. doi: 10.1016/S0965-1748(02)00181-9
46. Kopáček P, Perner J, Sojka D, Šíma R, Hajdušek O. Molecular targets to impair blood meal processing in ticks. In:Meng CQ, Sluder AE, , editors. Ectoparasites: Drug Discovery Against Moving Targets. John Wliley & Sons (2018). p. 139–65.
Keywords: poultry red mite, tropical fowl mite, northern fowl mite, ferritin 2, vaccine
Citation: Win SY, Murata S, Fujisawa S, Seo H, Sato J, Motai Y, Sato T, Oishi E, Taneno A, Htun LL, Bawm S, Okagawa T, Maekawa N, Konnai S and Ohashi K (2023) Potential of ferritin 2 as an antigen for the development of a universal vaccine for avian mites, poultry red mites, tropical fowl mites, and northern fowl mites. Front. Vet. Sci. 10:1182930. doi: 10.3389/fvets.2023.1182930
Received: 09 March 2023; Accepted: 30 March 2023;
Published: 17 April 2023.
Edited by:
Guillermo Tellez-Isaias, University of Arkansas, United StatesReviewed by:
Victor Manuel Petrone-García, National Autonomous University of Mexico, MexicoLuis-Miguel Gómez-Osorio, Solla S.A., Colombia
Copyright © 2023 Win, Murata, Fujisawa, Seo, Sato, Motai, Sato, Oishi, Taneno, Htun, Bawm, Okagawa, Maekawa, Konnai and Ohashi. This is an open-access article distributed under the terms of the Creative Commons Attribution License (CC BY). The use, distribution or reproduction in other forums is permitted, provided the original author(s) and the copyright owner(s) are credited and that the original publication in this journal is cited, in accordance with accepted academic practice. No use, distribution or reproduction is permitted which does not comply with these terms.
*Correspondence: Shiro Murata, bXVyYXRhQHZldG1lZC5ob2t1ZGFpLmFjLmpw