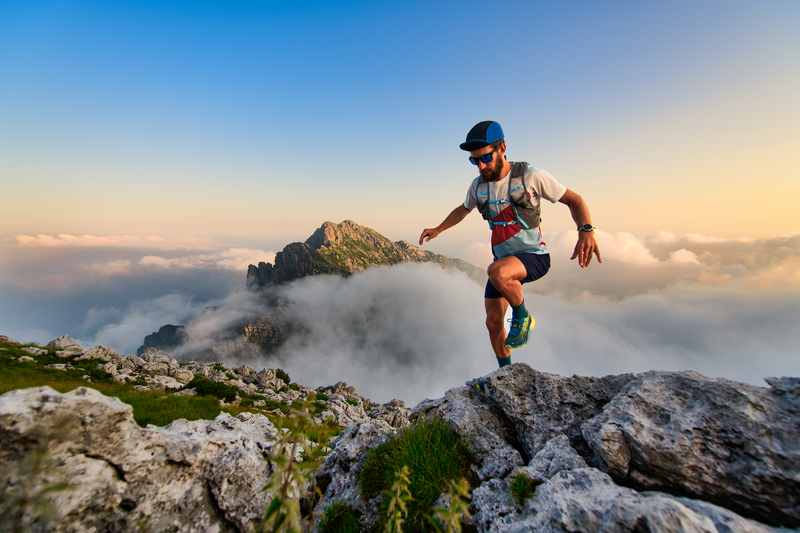
95% of researchers rate our articles as excellent or good
Learn more about the work of our research integrity team to safeguard the quality of each article we publish.
Find out more
REVIEW article
Front. Vet. Sci. , 17 April 2023
Sec. Animal Behavior and Welfare
Volume 10 - 2023 | https://doi.org/10.3389/fvets.2023.1167016
The stress response, which involves joint activity of the nervous and endocrine systems, is one of the basic adaptive mechanisms that ensures the survival of the individual. The activation of the sympathetic nervous system, the sympathetic-adrenal-medullary axis, and the hypothalamic–pituitary–adrenal axis enables organisms to respond to endogenous and exogenous challenges. Repeated short-term stress leads to long-term stress, which disrupts physiological homeostasis. Unlike domestic animals, wild animals are not protected from environmental and weather influences or treated for diseases. In addition, climate change, habitat fragmentation and loss, and urban stressors (such as light, noise and chemical pollution; xenobiotics; traffic; and buildings) affect individual wildlife and populations. In this review, we have attempted to depict the magnitude of the stress response in wildlife and related domestic animals as well as in captive and free-ranging animals. The intensity of the stress response can be estimated by determining the concentration of glucocorticoids in body fluids, tissues, and excreta. A comparison of results from different studies suggests that domestic animals have lower fecal and hair glucocorticoid concentrations than related wild animals. Additionally, fecal and hair glucocorticoid concentrations in captive animals are higher than in free-ranging animals of the same species. As there are limited data on this topic, we cannot draw definitive conclusions about glucocorticoid concentration and stress response. Further studies are needed to clarify these issues.
The concept of the fight-or-flight response and the notion of homeostasis were introduced by Harvard physiologist Walter Bradford Cannon in 1929 (1). Since then, the pathophysiological effects of stress have been extensively studied. Selye (2) described stress as a nonspecific response of the organism to a harmful factor. Selye (2) also introduced the general adaptation syndrome and divided the overall response to stress into three phases: alarm, resistance, and exhaustion. When individuals are exposed to a stressor, the alarm reaction is triggered, followed by the stage of resistance to maintain homeostasis; finally, in the stage of exhaustion, individuals succumb to the stressor. Today, stress is defined as the effect of all abiotic and biotic factors that can negatively affect the performance of an individual and alter the psychological, physiological and/or physical health of living organisms (3–5).
The current stress framework is broader than the classic approach. All organisms, including unicellular organisms and plants, have systems to protect against harmful factors; the purpose of these systems is to maintain homeostasis (6). Protective systems are activated when an endogenous or exogenous stimulus disrupts what Claude Bernard called the “milieu interieur” of an organism. At the cellular level, some highly potent metabolites called reactive oxygen species (ROS) are formed. ROS play a role in cell signaling but can also react with various organic cellular molecules to cause oxidative stress, which can lead to lipid and protein peroxidation and severe damage to bioactive molecules (7–9). Cells have an antioxidant capacity to protect against the effects of ROS. In fact, the mechanisms protecting against oxidative stress are not directly related to the stress response in higher organisms. However, oxidative stress can also be considered a stressor since it disrupts cell homeostasis. Thus, the stress effect is generally considered any disruption of homeostasis that may occur at the cellular or tissue level as well as a complex response of all organ systems.
As described by Lu et al. (10), the stress system consists of a stressful stimulus, stressor, stress, stress response, and stress effect. Therefore, the framework of stress includes exogenous factors and endogenous responses that eventually lead to the stress effect. The stressor → stress → stress response cascade ends in the stress effect. A stressor is a stimulus that threatens homeostasis and triggers a response to restore homeostasis (11, 12). The stress response, which emerges as a result of the joint activity of the nervous and endocrine systems, is one of the basic adaptive mechanisms to ensure the survival of the individual. From an evolutionary perspective, the stress response has evolved as a protective mechanism necessary for the survival of the species, thus ensuring its evolutionary conservation. The most frequently mentioned response to stressful situations in the animal world is the fight-or-flight response, to which the freeze response can also be added (13). Thus, in stressful situations, both predators and prey respond with intense physical activity triggered by stimulation of the sympathetic-adrenal-medullary (SAM) axis and the hypothalamic–pituitary–adrenal (HPA) axis. In addition to the predator-prey relationship, stress can also be triggered by various other exogenous and endogenous stimuli, such as weather conditions, food deprivation, diseases, ectoparasite infestations, reproductive activity, parturition, and social relationships. Considering the abovementioned stressful stimuli, it is obvious that the stress response can be divided into non-threatening (“good”) stress or eustress and harmful (“bad”) stress, called distress. Therefore, the general belief that all forms of stress have a negative impact on homeostasis is not correct (12). Living organisms respond to internal or external stressors to which they are constantly exposed. The body, on the other hand, analyzes and responds to the events that cause stress. Since eustress does not affect animal health, it is studied (less frequently) at the physiological level, while distress is studied in depth as a pathophysiological process that causes severe problems in intensive livestock production and in humans.
Wild animals are frequently or constantly exposed to stressful stimuli, as they are all either predator or prey. Unlike domestic animals, wild animals are exposed to environmental stress, food deprivation, parasite infestation and numerous untreated diseases. In addition, human pressure, wildlife management interventions, habitat fragmentation and loss, climate change, poaching, and invasive species are also stressors to wildlife populations (4, 14, 15). Therefore, stress in wildlife can be considered different from than in related domestic species. Furthermore, stress affects the growth, development, and survival of organisms and ultimately influences wildlife population dynamics (5).
In this review, we attempted to describe the stress response and compensatory mechanisms for adaptation to short-term stress and the effects of long-term stress. In addition, we tried to present the magnitude of the stress response in wildlife and related domestic species and free-ranging and captive animals.
Stressors evoke a series of reactions in organisms. Sensory organs or cells detect information about the stressful stimulus, which is transmitted via sensory nerves to cortical centers in the brain. The information is further transmitted through the limbic system and hypothalamus, thus stimulating the sympathetic nervous system and activating the HPA axis (16).
The first response following the perception of a stressor is activation of the sympathetic nervous system, which leads to two different but related pathways, i.e., sympathetic neurotransmission and activation of the SAM axis. The first pathway, which is faster, is neurotransmission in efferent peripheral sympathetic adrenergic nerves, leading to the release of noradrenaline at the neuroeffector junction. There, as a neurotransmitter, noradrenaline binds to the postsynaptic membrane α and β adrenergic receptors (with a higher affinity for α. α receptors (subtypes α1, α2, and α3) are found in cells of almost all organ systems. In vascular smooth muscle cells, they control baseline vascular tone and modulate systemic vascular resistance and venous capacitance. In the gastrointestinal tract, activated receptors provide vasoconstriction and slow digestive processes (17, 18). In addition to the effects mentioned above, activation of α1-receptors influences cognition, which is also an important factor in the stress response (19). Furthermore, neurotransmission in cholinergic preganglionic sympathetic nerves innervating the adrenal medulla and derived from splanchnic nerves activates the SAM axis (20). In the neuroeffector junction of medullary chromaffin cells, acetylcholine binds as a neurotransmitter to cholinergic receptors on the postsynaptic membrane and provides adrenalin secretion (21). Endogenous ligands for β adrenergic receptors (subtypes β1, β2, and β3) are catecholamines with adrenaline as the ligand with the highest affinity. Therefore, the receptors are activated after the release of adrenaline from the adrenal medulla. Adrenaline is released into the systemic circulation as a hormone and reaches target cells through the blood. Hence, the effects of the SAM axis occur a few moments later than the direct effects of the sympathetic nervous system. Increased heart rate, increased blood pressure, bronchodilatation, intense blood flow to the lungs and skeletal muscles, glycolysis, and gluconeogenesis during the fight-or-flight response are the result of the activated sympathetic nervous system and are maintained by the SAM axis.
In the next step of the stress response, the HPA axis is activated. The HPA axis is highly important to homeostasis as it responds to environmental variables that cause stress. It is a complex system of neuroendocrine pathways and regulatory circuits that are activated under stressful conditions (22). Glucocorticoids are steroid hormones that are released according to circadian rhythms as well as during stress. They influence cellular functions and are involved in both anabolic and catabolic reactions (23). In mammals, with the exception of rodents where corticosterone is present in high concentration, cortisol is the major glucocorticoid hormone affecting many different systemic activities and is secreted by the adrenal glands (24, 25). Activation of the HPA axis begins with the secretion of corticotropin-releasing hormone (CRH) from the paraventricular nucleus of the hypothalamus. CRH triggers pituitary stimulation and subsequent release of adrenocorticotropic hormone (ACTH) into circulation. ACTH binds to a G-protein-coupled receptor, the melanocortin-2 receptor. This binding leads to activation of adenyl cyclase, production of cAMP, and activation of protein kinase A (PKA). PKA alters the activity of certain transcription factors by phosphorylation. ACTH activates HMG-CoA reductase, increases LDL-C ester uptake, and activates hormone-sensitive lipase and acyl-coenzyme A (CoA) to increase the cholesterol pool for steroidogenesis. The primary site of ACTH action is the adrenal cortex, which produces glucocorticoids, mineralocorticoids, and androgens. When the adrenal cortex is stimulated by ACTH, the conversion of cholesterol to steroids occurs in a series of enzymatic steps, and cortisol is synthesized from pregnenolone through a series of isomerization and hydroxylation steps catalyzed by 11β-hydroxylase (26, 27). Cortisol is released into the blood and binds to corticosteroid-binding globulin (23, 28). The increase in cortisol levels in the body is sensed by the hypothalamus and pituitary receptors and regulated by negative feedback.
Cortisol exerts effects in organisms upon binding to intracellular glucocorticoid receptors (GRs). In cell signaling, the glucocorticoid-GR complex activates glucocorticoid-responsive elements in promoter or enhancer regions in a DNA sequence-specific manner to cause transcriptional induction of target genes or binds to negative glucocorticoid-responsive elements (29). The glucocorticoid-GR complex plays an important role in gene expression of kinase enzymes involved in the catabolism of carbohydrates, proteins, and lipids, but cortisol also has anabolic effects. Glycogenolysis during hepatic glucose production and gluconeogenesis (the anti-insulin effects of cortisol) are the result of the transcription of enzymes involved in these metabolic pathways. In addition, inhibition of the transcription of proinflammatory cytokines and an altered acquired immune response are the result of cortisol activity (13).
Thus, the activity of both the sympathetic nervous system and the HPA axis influence the responses of organisms that lead to adaptation to stress.
The fight-or-flight response (also known as the acute stress response) is a physiological response that occurs due to a perceived harmful event, attack, or life-threatening danger (stressor) (1). The fight-or-flight response is based on the theory that the sympathetic nervous system of organisms responds to stressors and prepares for fight or flight. In doing so, it elicits a series of responses through the actions of noradrenaline and adrenaline, two transmitters that act through α- and β-adrenergic receptors. These responses can occur in a variety of organ systems. The stress response includes increases in heart rate, increases in blood pressure and respiratory rate, and decreases in gastrointestinal tract activity (13, 21). These integrated physiological changes are a part of the fight-or-flight response observed following acute stressors.
Short-term glucocorticoid effects in the face of stressful situations (temporary climatic events, predator threats, dominance interactions) are referred to as acute stress and are adaptive, but when the effects of stressors extend over a longer period of time (resource limitation, famine, drought), chronic stress ensues, with more severe consequences (3, 4, 30).
When the stressful stimulus lasts for a long time, the HPA axis remains active. In such a situation, negative effects of glucocorticoids are detected (16). For example, long-term or chronic stress can lead to a negative feedback loop in the HPA axis, resulting in a downregulated response to any further acute stressor (31). Longitudinal studies and regular examinations are necessary to detect chronic stress. Such approaches, evaluating the population and stressors over the long term, are more common in the fields of wildlife management and planning (30, 32).
Animal species respond differently to different types of glucocorticoids. For example, the responses of certain animals to cortisol (the primary glucocorticoid in fish and most mammals) (3, 33) and corticosterone (the primary glucocorticoid in rodents, birds, reptiles, and amphibians) (34) can vary depending on age, sex, developmental stage, and body condition. In this review, we focused mainly on mammalian species to provide data on cortisol as a stress biomarker. The magnitude and patterns of elevated cortisol levels vary not only among species and sexes, but also among individuals. The differences in responses may be caused by genetic differences (35).
Determination of blood cortisol (or corticosterone) concentrations can be used to assess stress responses in animals. The blood cortisol concentration indicates the current glucocorticoid status. As shown in the study by Caroprese et al. (36), cortisol concentration increases rapidly after a stressful stimulus and can be fourfold higher than baseline in a couple of minutes after the stressful stimulus. Because blood cortisol concentrations vary daily and concentrations vary among individuals, it is difficult to determine the stress response based on a single blood cortisol measurement. In addition, blood collection and manipulations of the animal cause stress and may mask the true glucocorticoid status. Therefore, the determination of blood glucocorticoid levels as a stress biomarker is not recommended as an appropriate tool for assessing the stress response. Non-invasive methods of sampling are preferable because they prevent both the negative impact on animal welfare and the stress caused by capturing/restraint of the animals, which can be misleading when evaluating samples (37, 38).
Glucocorticoids are mostly metabolized and inactivated in the liver and excreted from the body through bile to the feces. They may also be found in urine, milk, saliva, and hair. In these materials, they are present in free and metabolized forms (39, 40). Due to the different dynamics of cortisol excretion in different materials, choosing the right material for analysis is important to gain insight into the cortisol status. Salivary cortisol levels indicate the current glucocorticoid status and are subject to circadian fluctuations similar to blood cortisol levels. Concentrations in saliva are approximately 10-fold lower than that in plasma (41). As samples can be collected non-invasively, salivary cortisol concentrations can be used to assess short-term stress (41). Evaluation of fecal glucocorticoid concentrations is used to assess stress in many animal species (42–45). Fecal glucocorticoid concentrations reflect the glucocorticoid status of the animal over one to 2 days or more, depending on the gut transit time, prior to sampling. The method is useful for assessing short- and long-term stress. More reliable results are obtained if the samples are taken several times at intervals of several days. Fecal samples offer several non-negligible advantages: they can be collected easily and without irritating the animal (46). Thus, this method provides the opportunity to take samples frequently, even over long periods of time.
Moreover, during hair growth, the hair shaft is loaded with cortisol. As hair growth is a long-lasting process, the determination of the hair glucocorticoid concentration can be used to evaluate long-term stress as well as to assess animal welfare (47–49). As suggested by Sheriff et al. (50), sometimes more than one material can be analyzed to obtain a correct result. Although the detection of glucocorticoids in feces or hair provides indirect insight into glucocorticoid status, the results should be considered a retrospective aspect of the stress response.
To ensure the reliability of results, methods for detecting glucocorticoids in feces and hair must be validated for the species of interest because the choice of substrate and hormone metabolite is important for accurate detection of biological changes in stress hormone levels.
Climate change, habitat fragmentation and loss affect wildlife populations just as much as they affect the environment (51). For this reason, these three factors dominate the One Health Paradigm (4, 52). One Health's approach is to tackle health problems at the human-animal-environment interface, including zoonotic diseases; to develop new concepts about pathogens, comparative immunology, and epidemiological dynamics; and to understand the role played by environmental factors in each of system (53, 54). This approach illustrates the intertwined nature of diseases among humans, livestock, and wildlife. Moreover, these relationship are affected by some factors, such as host, pathogen, environmental, political, and socioeconomic factors (53). As the human population is increasing worldwide, animal populations are also growing significantly. Therefore, human–wild animal conflict and interaction are ineluctably on the rise. It is very important to prioritize the One Health perspective in studies of wild animals. In addition, the increase in the human population is significantly changing the environmental conditions on Earth, which may expose some species to urban stressors such as light pollution, noise pollution, chemical pollution, traffic, buildings, and fragmented habitats, as well as other factors that may be beneficial, such as anthropogenic food supplies and warmer microclimates, especially during the colder months (55–57). These environmental stressors, which we call urban stressors, can be foreseeable or unforeseeable and greatly impact individual fitness and evolutionary adaptation. Especially because urban landscapes are managed primarily for humans, these factors can pose significant challenges to wildlife species. The stressful environments may cause morphological, physiological and parasitological differences between urban and non-urban populations (58, 59).
The HPA axis is the primary mechanism that boosts the adaptive response to environmental stressors in vertebrates (Table 1) (71). The physiological coping mechanism used by vertebrates to ensure survival under unfavorable environmental conditions is the acute stress response, which is characterized by the release of glucocorticoid hormones. The short-term secretion of the aforementioned steroid hormones can be beneficial; however, secretion of the same hormones over long periods has side effects on reproductive activity, the immune system and brain functions. Therefore, many species that inhabit urban ecosystems or urban areas cannot survive unless they their stress response adapts to the conditions of the city (56). However, while some studies have supported this idea and showed altered animal behavior and increased glucocorticoid concentrations, other studies have shown that, in contrast, stress responses decrease with human activity. Thus, studies have reported that although some wildlife species are negatively affected by anthropogenic disturbances (72, 73) and their populations decrease, some species exhibit no effects (74), and some exhibit a decreased physiological stress response (73) and may even benefit from urban conditions (56, 75). These differences among studies may be related to the complex responses of the neuroendocrine system to chronic stress, which may limit the physiological capacity to respond to the stressors, as well as to differences in species, populations, individuals (in terms of physiology and sex), and urban environments (50, 76, 77). Differences between populations are referred to as “synurbization”, i.e. adaptation to urban ecosystems (58, 59). However, the role of potential regulatory factors has not been formally and thoroughly studied.
Beyond the direct effects on wildlife, urbanization may have more devastating effects on wild populations in the future. As a result of urbanization, epidemiological processes may increase the morbidity of infectious diseases, which may impose substantial barriers to the conservation of wildlife populations and human health.
The designs of the studies included in this review are very different. The methods of sampling, glucocorticoid extraction from samples, and glucocorticoid detection vary from study to study. In most studies, cortisol or corticosterone concentrations were measured before and after stress exposure or ACTH challenge. The age and reproductive status of the animals also varied among studies, and several studies did not include both sexes. In addition, studies were conducted under different climatic conditions, and the timing of sampling varied among studies, which may influence the interpretation of the results, since daily and seasonal fluctuations of glucocorticoids have been observed (78–80). The studies also varied in terms of analytes since cortisol or glucocorticoid metabolites were determined in fecal samples. Additionally, in some studies, hair was obtained from dead animals. Therefore, the studies cannot be directly compared. When data are available, we report the range of cortisol, corticosterone or glucocorticoid metabolites concentrations; otherwise, mean values are reported.
Comparing the results of studies examining glucocorticoids in the feces or hair of domestic and wild species, glucocorticoid concentrations are higher in wild animals than in related domestic species. Tables 1, 2 display data from studies showing concentrations of glucocorticoids in the feces or hair of various domestic and wild animal species. Although a conclusion cannot be drawn due to differences in analytical approaches, the data are indicative of higher glucocorticoid levels in wild animals compared to domestic ones.
Wild boars and domestic pigs belong to the same species (Sus scrofa); thus, their metabolic status can be easily compared. The data from free-ranging wild boars (Table 1) were obtained from different tiger reserves in India (60). As is evident in Table 1, their fecal levels were higher than those obtained from domestic pigs. In addition, hair glucocorticoid concentrations (shown in Table 2) were also higher in wild boars. These samples were obtained from wild boars in Japan captured 10 years after the nuclear accident near the power plant in Fukushima, Japan (81). As was reported in the study, glucocorticoid levels in the hair were not affected by the increased radiation, but the glucocorticoid levels were higher than in domestic pigs (82).
Wild bison and domestic cattle belong to different but related species (Bison bison and Bos taurus, respectively). We compared fecal glucocorticoid levels in these species because data on wild Bos taurus are not available. The range of glucocorticoid concentrations in bison bulls correlates with their hierarchy rank; bulls with high social status must pay the physiological price of high glucocorticoid levels (62). Furthermore, Tallo-Parra et al. (64) measured fecal concentrations in Holstein cows (Bos taurus), while Hernandez-Cruz et al. (63) measured fecal glucocorticoid concentrations in zebu bulls (Bos indicus) of different ages from beef farms. Because of differences in species, design, and sex (males have higher cortisol levels), the studies cannot be directly compared. However, the higher fecal glucocorticoid concentrations in the wild bison population (Table 1) may support the hypothesis of a more active HPA axis in wild animals. Additionally, comparison of hair (or qiviut) glucocorticoid concentrations in two related species, domestic cattle and wild muskoxen (Ovibos moschatus), also revealed higher levels in the wild population (64, 83, 84).
In addition, we compared the glucocorticoid levels in some carnivores. The gray wolf and the dog are phylogenetically related, as both belong to the same genus (Canis), but the species are different (Canis lupus and Canis canis). In free-ranging wolves, fluctuations in fecal glucocorticoid levels have been observed. Several exogenous and endogenous factors, such as age, sex, reproductive activity, and season, influence the intensity of glucocorticoid secretion. Fecal glucocorticoid concentrations also greatly increased in the presence of humans (65). In dogs from animal resource centers, fecal glucocorticoid levels appear to be similar, but unlike wolves, high elevations in response to humans were not observed (66). Similarly, comparable hair glucocorticoid concentrations were found in many animals of both species, but only wolves were found to have very high levels. Moreover, compared to the findings of Bryan et al. (66), Accorsi et al. (67) reported lower physiological glucocorticoid levels in the feces and hair of dogs.
In the European wildcat (Felis silvestris), no significant differences in fecal glucocorticoid levels were found between populations from different habitats (68). However, fecal glucocorticoid levels were higher than those in domestic cats (67). As described by Franchini et al. (86), hair glucocorticoid concentrations are higher in European wildcats than in feral cats. Interestingly, Contreras et al. (87) reported that hair glucocorticoid levels depend on the body region where the sample was obtained. The lowest levels of glucocorticoids were found in hair samples from the neck. Hair can be contaminated with saliva and salivary cortisol; thus, significant differences were found in hair samples from different body regions. This explains the wide range of hair glucocorticoids concentrations in Table 2.
Even laboratory rats, which are somewhat domesticated, have lower fecal glucocorticoid levels than their wild counterparts (69, 70).
While we cannot draw a firm conclusion due to the differences in research design and study objectives, the data show higher cortisol levels in wild species. As mentioned earlier, wild individuals are either predator or prey and are exposed to various environmental and social stressors. Although the data in Tables 1, 2 originated from studies with different approaches, we hypothesize that wild animals are exposed to more stressful situations, which is reflected in higher glucocorticoid levels.
Data about stress and glucocorticoid concentrations in free-ranging animal species that also live in captivity are limited. Comparison is possible only in the species we mention in Tables 3–5.
As shown in the study by Benhaiem et al. (88), the range of fecal glucocorticoid levels in juvenile spotted hyenas (Crocuta crocuta) is broader, and levels are higher than in free-ranging females (89). However, a comparison of the mean values of the two studies revealed a different situation. In captive juveniles of different ages, fecal glucocorticoids were found at concentrations of 18.9 and 51 ng/g, whereas the concentrations in nonlactating and lactating free-ranging females were 40 and 112 ng/g, respectively. Young, pregnant, and lactating animals are known to have high glucocorticoid levels, so lower levels would be expected in other animal categories. Unfortunately, data are not available for other categories. Because of this variation, the studies cannot be directly compared; although mean glucocorticoid levels were higher in free-ranging animals, this cannot be considered a conclusion. Two Felinae species, the Canada lynx (Lynx canadensis) and cheetah (Acinonyx jubatus), showed similar fecal glucocorticoid patterns. In both species, captive animals had higher fecal corticosteroid levels than free-ranging animals (90, 91). In captive cheetahs, morphological evaluation of the adrenal glands showed evidence of increased activity suggestive of chronic stress (91). There are many factors that influence fecal corticosteroid concentration, thus we cannot definitively conclude that animals in captivity are more stressed than those in the wild. To confirm that the higher glucocorticoid levels in captive animals are a result of a stress response, further studies should be conducted to look at the activity of the SAM axis in addition to glucocorticoid concentration and HPA axis activity. It is important to note that while the lifespan of some captive species is longer due to preventive health care, adequate nutrition, and controlled environments, alterations in behavior may be indicative of a questionable welfare state (113).
Studies of glucocorticoid status in free-ranging and captive fallow deer (Dama dama) used the same method of fecal glucocorticoid determination and were also similar in design (79, 92). In both studies, glucocorticoid status was followed over a one-year period. As shown in Table 3, captive deer had a wider range of glucocorticoid levels. However, median levels were also several times higher in captive deer (79, 92).
Similarly, the Asian elephant has also been found to have higher fecal glucocorticoid concentrations in captivity (93, 94).
In addition, two primate species, lemur and Yucatan spider monkey, have been found to have higher fecal glucocorticoid concentrations in captive animals (95–97), although the studies by Pollastri et al. (95) and Pride (96) reporting glucocorticoid concentrations in lemurs are not directly comparable.
In contrast, in the Australian endangered marsupial, Gilbert's potoroo, higher glucocorticoid level was observed in free-ranging animals (98). Therefore, even though many studies show that captive populations of wild animals generally have higher glucocorticoid concentrations than their free-ranging counterparts, it is not definitive information unless comparable data are obtained. For instance, although not directly relevant to our research topic, according to a study by Shimamoto et al. (114), there are no significant differences in fecal glucocorticoid concentrations in Eurasian red squirrels' (Sciurus vulgaris) populations in rural and urban areas.
Considering the results of the studies shown in Table 3, we concluded that observed captive animals had higher fecal glucocorticoid levels than their wild counterparts.
Fecal glucocorticoid concentration, an indicator of cortisol status, reflects total glucocorticoid secretion, which can be used to assess the intensity of stressful events over a period of 1 to 2 days, whereas hair glucocorticoid concentration reflects long-term cortisol status. As shown in Table 4, similar to fecal glucocorticoid concentrations, hair glucocorticoid concentrations are also higher in captive than in free-ranging animals. Although the literature contains much information on hair glucocorticoid concentrations in animals, there are few studies directly comparing captive and free-ranging populations, which makes it challenging to draw a conclusion.
In contrast, blood cortisol concentrations reflect the current cortisol status. As shown in Table 5, free-ranging animals had higher cortisol levels than captive animals. These data appear to display the opposite pattern of fecal glucocorticoid levels; however, a definitive conclusion cannot be reached since the data are scarce. These results also suggest that captive animals are used to the presence of humans; thus, during blood collection, they do not respond with an intense stress response. In contrast, blood sampling of wild animals is a drastic procedure that requires manipulation of the animal and can elicit a strong stress response (37, 38). Perhaps this explains why few data are available. However, considering the higher fecal glucocorticoid levels of captive animals, we assume that the sum of all stress responses during the day that lead to activation of the HPA axis and excretion of cortisol (glucocorticoid metabolites) is higher in captive animals than in free-ranging individuals. We are aware that the comparison of some studies is not entirely reliable because the sensitivity of the methods used differs between studies, for example, in the study by Seal and Mech (107) and Thoresen et al. (108), which we consider a shortcoming of this review. Unfortunately, we were also unable to compare fecal glucocorticoids with hair or plasma glucocorticoid concentrations in the same species because of the lack of data. Therefore, comparison of the stress response between free-ranging and captive animals is a topic for further research.
An interesting question that currently lacks a no precise answer is why the natural lifespan of domestic animals is longer than that of related wildlife species and whether this is related to the stress response. If we consider glucocorticoid levels in wild animals to be species specific, domestication influenced the stress response. We believe that the HPA axis may also respond with the same intensity in domestic animals as in related wild animals, but the benefits of domestication and animal care provided by humans protect domestic animals from intense stressors. The question is what parameter(s) of domestic animal welfare facilitate longer lifespans. It has been reported that free-ranging animals with faster pace of life are subject to several environmentally-driven mortality and therefore live longer in captivity (zoos). In contrast, animals with slower pace of life live longer in natural environments (113). Thus, this review raises the question of whether frequent or chronic stress (among other external and internal factors) contributes to shorter lifespans in free-ranging species with faster pace of life.
In general, long-term stress or frequent exposure to stressors leads to activation of the SAM and HPA axes. In the long term, this can affect cardiovascular, digestive, immune as well as other systems (13, 17–19). Frequent stress in wildlife may be one of the reasons for their shorter lifespan compared to that of their domestic relatives. However, this hypothesis has not yet been confirmed and needs further investigation.
On the other hand, captive animals have higher glucocorticoid levels and longer lifespans than free-ranging animals. This information suggests benefits of human care, although the wellbeing of captive animals remains questionable.
In conclusion, a review of the available literature on glucocorticoid concentration shows higher levels in wild compared to related domestic animals. In addition, captive animals have higher glucocorticoid levels than their wild counterparts. While these are interesting trends, further studies are needed to investigate whether higher cortisol levels in wildlife compared to domestic animals and in captive compared to free-ranging animals are due to a stronger or more prolonged stress response.
MCK and TS: conceptualization, investigation, editing, and writing. NČK: editing and final validation. All authors contributed to the article and approved the submitted version.
This work was supported by Slovenian Research Agency grant no. P4-0053.
The authors declare that the research was conducted in the absence of any commercial or financial relationships that could be construed as a potential conflict of interest.
All claims expressed in this article are solely those of the authors and do not necessarily represent those of their affiliated organizations, or those of the publisher, the editors and the reviewers. Any product that may be evaluated in this article, or claim that may be made by its manufacturer, is not guaranteed or endorsed by the publisher.
1. Cannon WB. Bodily Changes in Pain, Hunger, Fear, and Rage: An Account of Recent Researches Into the Function of Emotional Excitement. New York, NY, USA: Appleton and Company. (1929).
3. Romero LM. Physiological stress in ecology: lessons from biomedical research. Trends Ecol Evol. (2004) 19:249–55. doi: 10.1016/j.tree.2004.03.008
4. Hing S, Narayan EJ, Thompson RA, Godfrey SS. The relationship between physiological stress and wildlife disease: consequences for health and conservation. Wildlife Res. (2016) 43:51–60. doi: 10.1071/WR15183
5. Wahl M, Jormalainen V, Eriksson BK, Coyer JA, Molis M, Schubert H, et al. Stress ecology in fucus: abiotic, biotic and genetic interactions. Advanc Marine Biol. (2011) 59:37–105. doi: 10.1016/B978-0-12-385536-7.00002-9
6. Demidchik V. Mechanisms of oxidative stress in plants: from classical chemistry to cell biology. Environ Exp Bot. (2015) 109:212–28. doi: 10.1016/j.envexpbot.2014.06.021
7. Hancock JT, Desikan R, Neill SJ. Role of reactive oxygen species in cell signaling pathways. Biochem Soc Trans. (2001) 29:345–50. doi: 10.1042/bst0290345
8. Ayala A, Muñoz MF, Argüelles S. Lipid peroxidation: production, metabolism, and signaling mechanisms of malondialdehyde and 4-hydroxy-2-nonenal. Oxid Med Cell Long. (2014) 31:360438. doi: 10.1155/2014/360438
9. Davies MJ. Protein oxidation and peroxidation. Biochem J. (2016) 473:805–25. doi: 10.1042/BJ20151227
10. Lu S, Wei F, Li G. The evolution of the concept of stress and the framework of the stress system. Cell Stress. (2021) 5:76–85. doi: 10.15698/cst2021.06.250
11. Chrousos GP. Stress and disorders of the stress system. Nat Rev Endocrinol. (2009) 5:374–81. doi: 10.1038/nrendo.2009.106
12. Koolhaas JM, Bartolomucci A, Buwalda B, de Boer SF, Flügge G, Korte SM, et al. Stress revisited: a critical evaluation of the stress concept. Neurosci Biobehav Rev. (2011) 35:1291–301. doi: 10.1016/j.neubiorev.2011.02.003
13. Chen Y, Arsenault R, Napper S, Griebel P. Models and methods to investigate acute stress responses in cattle. Animals. (2015) 5:1268–95. doi: 10.3390/ani5040411
14. Di Marco M, Boitani L, Mallon D, Hoffmann M, Iacucci A, Meijaard E, et al. A retrospective evaluation of the global decline of carnivores and ungulates. Conserv Biol. (2014) 28:1109–18. doi: 10.1111/cobi.12249
15. Yom-Tov Y, Balaban A, Hadad E, Weil G, Roll U. The plight of the endangered mountain gazelle Gazella gazella. Oryx. (2021) 55:71–8. doi: 10.1017/S003060531900108X
16. Mariotti A. The effects of chronic stress on health: new insights into the molecular mechanisms of brain–body communication. Future Sci OA. (2015) 1:FSO23. doi: 10.4155/fso.15.21
17. Ruffolo RR. Distribution and function of peripheral α-adrenoceptors in the cardiovascular system. Pharmacol Biochem Behav. (1985) 22:827–33. doi: 10.1016/0091-3057(85)90535-0
18. Scott-Solomon E, Boehm E, Kuruvilla R. The sympathetic nervous system in development in disease. Nat Rew Neurosci. (2021) 22:685–702. doi: 10.1038/s41583-021-00523-y
19. Perez DM. α1-adrenergic receptors in neurotransmission, synaptic plasticity, and cognition. Front Pharmacol. (2020) 11:581098. doi: 10.3389/fphar.2020.581098
20. Parker TL, Kesse WK, Mohamed AA, Afework M. The innervation of the mammalian adrenal gland. J Anat. (1993) 183:265–76.
21. Nassar-Gentina V, Catalán L, Luxoro M. Nicotinic and muscarinic components in acetylcholine stimulation of porcine adrenal medullary cells. Mol Cell Biochem. (1997) 169:107–13. doi: 10.1023/A:1006867423715
22. Sheng JA, Bales NJ, Myers SA, Bautista AI, Roueinfar M, Hale TM, et al. The hypothalamic-pituitary-adrenal axis: development, programming actions of hormones, and maternal-fetal interactions. Front Behav Neurosci. (2021) 14:601939. doi: 10.3389/fnbeh.2020.601939
23. Chourpiliadis C, Aeddula NR. Physiology, Glucocorticoids. Tampa, FL: StatPearls Publishing. (2022).
24. Oswald LM, Zandi P, Nestadt G, Potash JB, Kalaydjian AE. Wand GS. Relationship between cortisol responses to stress and personality. Neuropsychopharmacol. (2006) 31:1583–91. doi: 10.1038/sj.npp.1301012
25. Zänkert S, Bellingrath S, Wüst S, Kudielka BM, HPA. axis responses to psychological challenge linking stress and disease: what do we know on sources of intra-and interindividual variability? Psychoneuroendocrinology. (2019) 105:86–97. doi: 10.1016/j.psyneuen.2018.10.027
26. Sewer MB, Waterman MR, ACTH. modulation of transcription factors responsible for steroid hydroxylase gene expression in the adrenal cortex. Microsc Res Tech. (2003) 61:300–7. doi: 10.1002/jemt.10339
27. Payne AH, Hales DB. Overview of steroidogenic enzymes in the pathway from cholesterol to active steroid hormones. Endocr Rev. (2004) 25:947–70. doi: 10.1210/er.2003-0030
28. Henley D, Lightman S, Carrell R. Cortisol and CBG - Getting cortisol to the right place at the right time. Pharmacol Therapeut. (2016) 166:128–35. doi: 10.1016/j.pharmthera.2016.06.020
29. Biddie SC, Hager GL. Glucocorticoid receptor dynamics and gene regulation. Stress. (2009) 12:193–205. doi: 10.1080/10253890802506409
30. Fourie NH, Brown JL, Jolly CJ, Phillips-Conroy JE, Rogers J, Bernstein RM. Sources of variation in hair cortisol in wild and captive non-human primates. Zoology. (2016) 119:119–25. doi: 10.1016/j.zool.2016.01.001
31. Rich EL, Romero M. Exposure to chronic stress downregulates corticosterone responses to acute stressors. Am J Physiol Regul Integr Comp Physiol. (2005) 288:1628–36. doi: 10.1152/ajpregu.00484.2004
32. Pearson BL, Reeder DM, Judge PG. Crowding increases salivary cortisol but not self-directed behavior in captive baboons. Am J Primatol. (2015) 77:462–7. doi: 10.1002/ajp.22363
33. Lin CH, Tsai IL, Su CH, Tseng DY, Hwang PP. Reverse effect of mammalian hypocalcemic cortisol in fish: cortisol stimulates Ca2+ uptake via glucocorticoid receptor-mediated vitamin D3 metabolism. PLoS ONE. (2011) 6:e23689. doi: 10.1371/journal.pone.0023689
34. Injaian AS, Francis CD, Ouyang JQ, Dominoni DM, Donald J, Fuxjager MJ, et al. Baseline and stress-induced corticosterone levels across birds and reptiles do not reflect urbanization levels. Conserv Physiol. (2020) 8:coz110. doi: 10.1093/conphys/coz110
35. Cockrem JF. Individual variation in glucocorticoid stress responses in animals. Gen Comp Endocrinol. (2013) 181:45–58. doi: 10.1016/j.ygcen.2012.11.025
36. Caroprese M, Albenzio M, Marzano A, Schena L, Annicchiarico G, Sevi A. Relationship between cortisol response to stress and behavior, immune profile, and production performance of dairy ewes. J Dairy Sci. (2010) 3:2395–403. doi: 10.3168/jds.2009-2604
37. Costantini D, Wachter B, Melzheimer J, Czirják GA. Socioecological and environmental predictors of physiological stress markers in a threatened feline species. Conserv Physiol. (2017) 5:cox069. doi: 10.1093/conphys/cox069
38. Melvin ZE, Dhirani H, Mitchell C, Davenport TR, Blount JD, Georgiev AV. Methodological confounds of measuring urinary oxidative stress in wild animals. Ecol Evol. (2022) 12:e9115. doi: 10.1002/ece3.9115
39. Persichilli S, Gervasoni J, Iavarone F, Zuppi C, A. simple liquid chromatography-tandem mass spectrometry method for urinary free cortisol analysis: suitable for routine purpose. Clin Chem Lab Med. (2010) 48:1433. doi: 10.1515/CCLM.2010.282
40. Eleftheriou A, Palme R, Boonstra R. Assessment of the stress response in North American deermice: laboratory and field validation of two enzyme immunoassays for fecal corticosterone metabolites. Animals. (2020) 10:1120. doi: 10.3390/ani10071120
41. Plut J, Snoj T, Golinar Oven I, Štukelj M. The combination of serum and oral fluid cortisol levels and welfare quality protocol ® for assessment of pig welfare on intensive farms. Agriculture. (2023) 13:351. doi: 10.3390/agriculture13020351
42. Palme R, Möstl E. Measurement of cortisol metabolites in faeces of sheep as a parameter of cortisol concentration in blood. Proceed Suppl Physiol Ethyol Wild Zoo Anim. (1997) 21:192–7.
43. Van der Weyde LK, Martin GB, Paris MCJ. Monitoring stress in captive and free-ranging African wild dogs (Lycaon pictus) using faecal glucocorticoid metabolites. Gen Comp Endocrinol. (2016) 226:50–5. doi: 10.1016/j.ygcen.2015.12.022
44. Ebinghaus A, Knierim U, Simantke C, Palme R, Ivemeyer S. Fecal cortisol metabolites in dairy cows: a cross-Sectional exploration of associations with animal, stockperson, and farm characteristics. Animals. (2020) 10:1787. doi: 10.3390/ani10101787
45. Donini V, Iacona E, Pedrotti L. Macho-Maschler S, Palme R, Corlatti L. Temporal stability of fecal cortisol metabolites in mountain-dwelling ungulates. Sci Nature. (2022) 109:20. doi: 10.1007/s00114-022-01792-y
46. Palme R, Rettenbacher S, Touma C, El-Bahr SM, Moestl E. Stress hormones in mammals and birds: comparative aspects regarding metabolism, excretion, and noninvasive measurement in fecal samples. Ann N Y Acad Sci. (2005) 1040:162–71. doi: 10.1196/annals.1327.021
47. Caslini C, Comin A, Peric T, Prandi A, Pedrotti L, Mattiello S. Use of hair cortisol analysis for comparing population status in wild red deer (Cervus elaphus) living in areas with different characteristics. Eur J Wildl Res. (2016) 62:713–23. doi: 10.1007/s10344-016-1049-2
48. Nedić S, Kirovski D, Vujanac I, Prodanović R, Jovanović L, Kobal S, et al. Preventing ectoparasite infestations reduces glucocorticoid concentrations in the hair of cows – short communication. Acta Vet Hun. (2018) 66:390–3. doi: 10.1556/004.2018.035
49. Vesel U, Pavič T, JeŽek J, Snoj T, Starič J. Welfare assessment in dairy cows using hair cortisol as a part of monitoring protocols. J Dairy Res. (2020) 87:72–8. doi: 10.1017/S0022029920000588
50. Sheriff MJ, Dantzer B, Delehanty B, Palme R, Boonstra R. Measuring stress in wildlife: techniques for quantifying glucocorticoids. Oecologia. (2011) 166:869–87. doi: 10.1007/s00442-011-1943-y
51. Karaer MC, Yorulmaz T. Tavşanoglu, Ç. Iklim degişikliginin yarasalar üzerine olasi etkileri. JENAS. (2022) 4:174–98. doi: 10.53472/jenas.1149503
52. Daszak P, Cunningham AA, Hyatt AD. Emerging infectious diseases of wildlife: threats to biodiversity and human health. Science. (2000) 287:443–9. doi: 10.1126/science.287.5452.443
53. Miller M, Olea-Popelka F. One Health in the shrinking world: experiences with tuberculosis at the human–livestock–wildlife interface. Comp Immunol Microbiol Infect Dis. (2013) 36:263–8. doi: 10.1016/j.cimid.2012.07.005
54. Godfroid J. Brucellosis in livestock and wildlife: zoonotic diseases without pandemic potential in need of innovative one health approaches. Arch Pub Health. (2017) 75:1–6. doi: 10.1186/s13690-017-0207-7
55. Partecke J, Schwabl I, Gwinner E. Stress and the city: urbanization and its effects on the stress physiology in European blackbirds. Ecology. (2006) 87:1945–52. doi: 10.1890/0012-9658(2006)87[1945:SATCUA]2.0.CO;2
56. Beaugeard E, Brischoux F, Henry PY, Parenteau C, Trouvé C, Angelier F. Does urbanization cause stress in wild birds during development? Insights from feather corticosterone levels in juvenile house sparrows (Passer domesticus). Ecol Evol. (2019) 9:640–52. doi: 10.1002/ece3.4788
57. Huang P, St. Mary CM, Kimball RT. Habitat urbanization and stress response are primary predictors of personality variation in northern cardinals (Cardinalis cardinalis). J Urban Ecol. (2020) 6:juaa015. doi: 10.1093/jue/juaa015
58. Surov AV, Poplavskaya NS, Bogomolov PL, Kropotkina MV, Tovpinetz NN, Katzman EA, et al. Synurbization of the common hamster (Cricetus cricetus L., 1758). Russ J Biol Invasions. (2016) 7:69–76. doi: 10.1134/S2075111716010094
59. Francis RA. Chadwick MA. What makes a species synurbic? Appl Geogr. (2012) 32:514–21. doi: 10.1016/j.apgeog.2011.06.013
60. Allwin B, Jayathangaraj MG, Palanivelrajan M, Raman M. Evaluation of endogenous faecal cortisol as a non invasive assessment of stress in free ranging wild pigs (Sus scrofa). Ind J Vet Anim Sci Res. (2015) 44:89–92.
61. Mohan NH, Nath A, Thomas R, Kumar S, Banik S, Das AK, et al. Relationship between plasma, saliva, urinary and faecal cortisol levels in pigs. Ind J Anim Sci. (2020) 90:768–72. doi: 10.56093/ijans.v90i5.104628
62. Mooring MS, Patton ML, Lance VA, Hall BM, Schaad EW, Fetter GA, et al. Glucocorticoids of bison bulls in relation to social status. Hormon Behav. (2006) 49:369–75. doi: 10.1016/j.yhbeh.2005.08.008
63. Hernández-Cruz BC, Carrasco-García AA, Ahuja-Aguirre C, López-deBuen L, Rojas-Maya S, Montiel-Palacios F. Faecal cortisol concentrations as indicator of stress during intensive fattening of beef cattle in a humid tropical environment. Trop Anim Health Prod. (2015) 48:411–5. doi: 10.1007/s11250-015-0966-5
64. Tallo-Parra O, Manteca X, Sabes-Alsina M, CarbajalA, Lopez-Bejar M. Hair cortisol detection in dairy cattle by using EIA: protocol validation and correlation with faecal cortisol metabolites. Animal. (2015) 6:1059–1064. doi: 10.1017/S1751731115000294
65. Escobar-Ibarra I, Mayagoitia-Novales L, Alcántara-Barrera A, Cerda-Molina AL, Mondragón-Ceballos R, Ramírez-Necoechea R, et al. Long-term quantification of faecal glucocorticoid metabolite concentrations reveals that Mexican grey wolves may habituate tocaptivity. Eur Zoolog J. (2017) 84:311–20. doi: 10.1080/24750263.2017.1332111
66. Bryan HM, Adams AG, Invik RM, Wynne-Edwards KE, Smits JEG. Hair as a meaningful measure of baseline cortisol levels over time in dogs. J Am Assoc Lab Anim Sci. (2013) 52:189–96.
67. Accorsi PA, Carloni E, Valsecchi P, Viggiani R, Gamberoni M, Tamanini C, et al. Cortisol determination in hair and faeces from domestic cats and dogs. Gen Comp Endocrinol. (2008) 155:398–402. doi: 10.1016/j.ygcen.2007.07.002
68. Pineiro A, Barja I, Otero GP, Silvan G, Illera JC. No effects of habitat, prey, abundance and competitor carnivore abundance on fecal cortisol metabolite levels in wildcats (Felis silvestris). Ann Zool Fennici. (2015) 52:90–102. doi: 10.5735/086.052.0208
69. Fardell LL, Bedoya-Pérez MA, Dickman CR, Crowther MS, Pavey CR, Narayan EJ. Are physiological and behavioural responses to stressors displayed concordantly by wild urban rodents? Sci Nat. (2021) 108:5. doi: 10.1007/s00114-020-01716-8
70. Pihl L, Hau J. Faecal corticosterone and immunoglobulin A in young adult rats. Lab Anim. (2003) 37 166–71. doi: 10.1258/00236770360563822
71. Ramos-Güivas B, Jawor JM, Wright TF. Seasonal variation in fecal glucocorticoid levels and their relationship to reproductive success in captive populations of an endangered parrot. Diversity. (2021) 13:617. doi: 10.3390/d13120617
72. Müllner A, Linsenmair KE, Wikelski M. Exposure to ecotourism reduces survival and affects stress response in hoatzin chicks (Opisthocomus hoazin). Biol Conserv. (2004) 118:549–58. doi: 10.1016/j.biocon.2003.10.003
73. Walker BG, Boersma PD, Wingfield JC. Physiological and behavioral differences in Magellanic penguin chicks in undisturbed and tourist-visited locations of a colony. Conserv Biol. (2005) 19:1571–7. doi: 10.1111/j.1523-1739.2005.00104.x
74. Ibáñez-Álamo JD, Jimeno B, Gil D, Thomson RL, Aguirre JI, Díez-Fernández A, et al. Physiological stress does not increase with urbanization in European blackbirds: evidence from hormonal, immunological and cellular indicators. Sci Total Environ. (2020) 721:137332. doi: 10.1016/j.scitotenv.2020.137332
75. Kark S, Iwaniuk A, Schalimtzek A, Banker E. Living in the city: can anyone become an ‘urban exploiter'? J Biogeogr. (2007) 34:638–51. doi: 10.1111/j.1365-2699.2006.01638.x
76. Dantzer B, McAdam AG, Palme R, Fletcher QE, Boutin S, Humphries MM et al. Fecal cortisol metabolite levels in free-ranging North American red squirrels: assay validation and the effects of reproductive condition. Gen Comp Endocrinol. (2010) 167:279–86. doi: 10.1016/j.ygcen.2010.03.024
77. Sheriff M J, Wheeler H, Donker SA, Krebs CJ, Palme R, Hik DS, et al. Mountain-top and valley-bottom experiences: the stress axis as an integrator of environmental variability in arctic ground squirrel populations. J Zool. (2012) 287:65–75. doi: 10.1111/j.1469-7998.2011.00888.x
78. Ingram JR, Crockford JN, Matthews LR. (1999). Ultradian, circadian and seasonal rhythms in cortisol secretion and adrenal responsiveness to ACTH and yarding in unrestrained red deer (Cervus elaphus) stags. J Endocrinol. (1999) 162:289–300. doi: 10.1677/joe.0.1620289
79. Konjević D, Janicki Z, Slavica A, Severin K, Krapinec K, BoŽić F. Palme R. Non-invasive monitoring of adrenocortical activity in free-ranging fallow deer (Dama dama L). Eur J Wildl Res. (2011) 57:77–81. doi: 10.1007/s10344-010-0401-1
80. Snoj T, Jenko Z, Cebulj-Kadunc N. Fluctuations of serum cortisol, insulin and non-esterified fatty acid concentrations in growing ewes over the year. Irish Vet J. (2014) 67:22. doi: 10.1186/2046-0481-67-22
81. Cunningham K, Hinton TG, Luxton JJ, Bordman A, Okuda K, Taylor LE, et al. Evaluation of DNA damage and stress in wildlife chronically exposed to low-dose, low-dose rate radiation from the Fukushima Dai-ichi Nuclear Power Plant accident. Environ Int. (2021) 155:106675. doi: 10.1016/j.envint.2021.106675
82. Wiechers DH, Brunner S, Herbrandt S, Kemper N, Fels M. Analysis of hair cortisol as an indicator of chronic stress in pigs in two different farrowing systems. Front Vet Sci. (2021) 8:605078. doi: 10.3389/fvets.2021.605078
83. Di Francesco J, Kwong GPS, Deardon R, Checkley SL, Mastromonaco GF, Mavrot F, et al. Qiviut cortisol is associated with metrics of health and other intrinsic and extrinsic factors in wild muskoxen (Ovibos moschatus). Conserv Physiol. (2022) 10:coab103. doi: 10.1093/conphys/coab103
84. Burnett TA, Madureira AML, Silper BF, Tahmasbi A, Nadalin A, Veira DM, et al. Relationship of concentrations of cortisol in hair with health, biomarkers in blood, and reproductive status in dairy cows. J Dairy Sci. (2015) 98:4414–26. doi: 10.3168/jds.2014-8871
85. Pereira P, Fandos Esteruelas N, Nakamura M. Rio-Maior H, Krofel M, Di Blasio A, et al. Hair cortisol concentration reflects the life cycle and management of grey wolves across four European populations. Scient Rep. (2022) 12:5697. doi: 10.1038/s41598-022-09711-x
86. Franchini M, Prandi A, Filacorda S, Nilanthi Pezzin E, Fanin Y, et al. Cortisol in hair: a comparison between wild and feral cats in the north-eastern Alps. Eur J Wildl Res. (2019) 65:90. doi: 10.1007/s10344-019-1330-2
87. Contreras ET, Vanderstichel R, Hovenga C, Lappin MR. Evaluation of hair and nail cortisol concentrations and associations with behavioral, physical, and environmental indicators of chronic stress in cats. J Int Vet Med. (2021) 35:2662–72. doi: 10.1111/jvim.16283
88. Benhaiem S, Dehnhard M, Bonanni R, Hofer H, Goymann W, Eulenberger K, et al. Validation of an enzyme immunoassay for the measurement of faecal glucocorticoid metabolites in spotted hyenas (Crocuta crocuta). Gen Comp Endocrinol. (2012) 178:265–71. doi: 10.1016/j.ygcen.2012.05.006
89. Goymann W, East ML, Wachter B, Höner OP, Möstl E. Van't Hof TJ, et al. Social, state-dependent and environmental modulation of faecal corticosteroid levels in free-ranging female spotted hyenas. Proc R Soc Lond B. (2001) 268:2453–9. doi: 10.1098/rspb.2001.1828
90. Fanson KV, Wielebnowski NC, Shenk TM, Lucas JR. Comparative patterns of adrenal activity in captive and wild Canada lynx (Lynx canadensis). J Comp Physiol B. (2012) 182:157–65. doi: 10.1007/s00360-011-0597-8
91. Terio KA, Marker L, Munson L. Evidence for chronic stress in captive but not free-ranging cheetahs (Acinonyx jubatus) based on adrenal morphology and function. J Wildl Dis. (2004) 40:259–66. doi: 10.7589/0090-3558-40.2.259
92. Konjević D, Janicki Z, Slavica A, Severin K, Krapinec K, ŽeljeŽić D. et al. Monitoring cortisol metabolites in the faeces of captive fallow deer (Dama dama L). Veterinarski Arhiv. (2016) 86:363–71.
93. Fanson KV, Lynch M, Vogelnest L, Miller G, Keeley T. Response to long-distance relocation in Asian elephants (Elephas maximus): monitoring adrenocortical activity via serum, urine, and feces. Eur J Wildl Res. (2013) 59:655–64. doi: 10.1007/s10344-013-0718-7
94. Ghosal R, Ganswindt A, Seshagiri PB, Sukumar R. Endocrine correlates of musth in free-ranging Asian elephants (Elephas maximus) determined by non-invasive faecal steroid hormone metabolite measurements. PLoS ONE. (2013) 8:e84787. doi: 10.1371/journal.pone.0084787
95. Pollastri I, Normando S, Florio D, Ferrante L, Bandoli F, Macchi E, et al. The Animal-Visitor Interaction Protocol (AVIP) for the assessment of Lemur catta walk-in enclosure in zoos. PLoS ONE. (2022) 17:e0271409. doi: 10.1371/journal.pone.0271409
96. Pride RE. Optimal group size and seasonal stress in ring-tailed lemurs (Lemur catta). Behav Ecol. (2005) 16:550–60. doi: 10.1093/beheco/ari025
97. Rangel-Negrín A, Alfaro JL, Valdez RA, Romano MC, Serio-Silva JC. Stress in Yucatan spider monkeys: effects of environmental conditions on fecal cortisol levels in wild and captive populations. Anim Conserv. (2009) 12:496–502. doi: 10.1111/j.1469-1795.2009.00280.x
98. Stead-Richardson E, Bradshaw D, Friend T, Fletcher T. Monitoring reproduction in the critically endangered marsupial, Gilbert's potoroo (Potorous gilbertii): preliminary analysis of faecal oestradiol-17β, cortisol and progestagens. Gen Comp Endocrinol. (2010) 165:155–62. doi: 10.1016/j.ygcen.2009.06.009
99. Yamanashi Y, Teramoto M, Morimura N, Hirata S, Inoue-Murayama M, Idani GI. Effects of relocation and individual and environmental factors on the long-term stress levels in captive chimpanzees (Pan troglodytes): monitoring hair cortisol and behaviors. PLoS ONE. (2016) 11:e0160029. doi: 10.1371/journal.pone.0160029
100. Carlitz EH, Miller R, Kirschbaum C, Gao W, Hänni DC, van Schaik CP. Measuring hair cortisol concentrations to assess the effect of anthropogenic impacts on wild chimpanzees (Pan troglodytes). PLoS ONE. (2016) 11:e0151870. doi: 10.1371/journal.pone.0151870
101. Hein A, Baumgartner K, von Fersen L, Bechshoft T, Woelfing B, Kirschbaum C, et al. Analysis of hair steroid hormones in polar bears (Ursus maritimus) via liquid chromatography–tandem mass spectrometry: Comparison with two immunoassays and application for longitudinal monitoring in zoos. Gen Comp Endocrinol. (2021) 310:113837. doi: 10.1016/j.ygcen.2021.113837
102. Macbeth BJ, Cattet MRL, Obbard ME, Middel K, Janz DM. Evaluation of hair cortisol concentration as a biomarker of long-term stress in free-ranging polar bears. Wildl Soc Bull. (2012) 36:747–58. doi: 10.1002/wsb.219
103. Ashley NT, Barboza PS, Macbeth BJ, Janz DM, Cattet MRL, Booth RK. Glucocorticosteroid concentrations in feces and hair of captive caribou and reindeer following adrenocorticotropic hormone challenge. Gen Comp Endocrinol. (2011) 172:382–91. doi: 10.1016/j.ygcen.2011.03.029
104. Bondo KJ, Macbeth B, Schwantje H, Orsel K, Culling D, Culling B, et al. Health survey of boreal caribou (Rangifer tarandus caribou) in northeastern British Columbia, Canada. J Wildl Dis. (2019) 55:544–62. doi: 10.7589/2018-01-018
105. Phillips KA, Tukan AN, Rigodanzo AD, Reusch RT, Brasky KM, Meyer JS. Quantification of hair cortisol concentration in common marmosets (Callithrix jacchus) and tufted capuchins (Cebus apella). Am J Primatol. (2018) 80:e22879. doi: 10.1002/ajp.22879
106. Garber PA, McKenney A, Bartling-John E, Bicca-Marques JC. De la Fuente MF, Abreu F, et al. Life in a harsh environment: the effects of age, sex, reproductive condition, and season on hair cortisol concentration in a wild non-human primate. Peer J. (2020) 8:e9365. doi: 10.7717/peerj.9365
107. Seal US, Mech LD. Blood indicators of seasonal metabolic patterns in captive adult gray wolves. J Wildl Manag. (1983) 47:704–15. doi: 10.2307/3808606
108. Thoresen SI, Arnemo JM, Liberg O. Hematology and serum clinical chemistry reference intervals for free-ranging Scandinavian gray wolves (Canis lupus). Vet Clin Pathol. (2009) 38:224–9. doi: 10.1111/j.1939-165X.2008.00105.x
109. Steinman KJ, Robeck TR, O'Brien JK. Characterization of estrogens, testosterone, and cortisol in normal bottlenose dolphin (Tursiops truncatus) pregnancy. Gen Comp Endocrinol. (2016) 226:102–12. doi: 10.1016/j.ygcen.2015.12.019
110. Hart LB, Wells RS, Kellar N, Balmer BC, Hohn AA. Lamb SV, et al. Adrenal hormones in common bottlenose dolphins (Tursiops truncatus): Influential factors and reference intervals. PloS ONE. (2015) 10:e0127432. doi: 10.1371/journal.pone.0127432
111. Beer H. Comparison of comprehensive health score in North American Housed Giraffe and Free-Ranging Giraffe from South Africa. Nebraska: University of Nebraska, Lincoln Theses and Dissertations in Animal Science. (2020).
112. Quispe R, Villavicencio CP, Addis E, Wingfield JC, Vasquez RA. Seasonal variations of basal cortisol and high stress response to captivity in Octodon degus, a mammalian model species. Gen Comp Endocrinol. (2014) 197:65–72. doi: 10.1016/j.ygcen.2013.12.007
113. Tidière M, Gaillard JM, Berger V, Müller DWH, Bingaman Lackey L, Gimenez O, et al. Comparative analyses of longevity and senescence reveal variable survival benefits of living in zoos across mammals. Sci Rep. (2016) 6:36361. doi: 10.1038/srep36361
Keywords: stress response, wildlife, captive animals, domestic animals, glucocorticoids
Citation: Karaer MC, Čebulj-Kadunc N and Snoj T (2023) Stress in wildlife: comparison of the stress response among domestic, captive, and free-ranging animals. Front. Vet. Sci. 10:1167016. doi: 10.3389/fvets.2023.1167016
Received: 15 February 2023; Accepted: 30 March 2023;
Published: 17 April 2023.
Edited by:
Claudia Giannetto, University of Messina, ItalyReviewed by:
Raúl Delmar Cerutti, National University of Littoral, ArgentinaCopyright © 2023 Karaer, Čebulj-Kadunc and Snoj. This is an open-access article distributed under the terms of the Creative Commons Attribution License (CC BY). The use, distribution or reproduction in other forums is permitted, provided the original author(s) and the copyright owner(s) are credited and that the original publication in this journal is cited, in accordance with accepted academic practice. No use, distribution or reproduction is permitted which does not comply with these terms.
*Correspondence: Tomaž Snoj, dG9tYXouc25vakB2Zi51bmktbGouc2k=
Disclaimer: All claims expressed in this article are solely those of the authors and do not necessarily represent those of their affiliated organizations, or those of the publisher, the editors and the reviewers. Any product that may be evaluated in this article or claim that may be made by its manufacturer is not guaranteed or endorsed by the publisher.
Research integrity at Frontiers
Learn more about the work of our research integrity team to safeguard the quality of each article we publish.