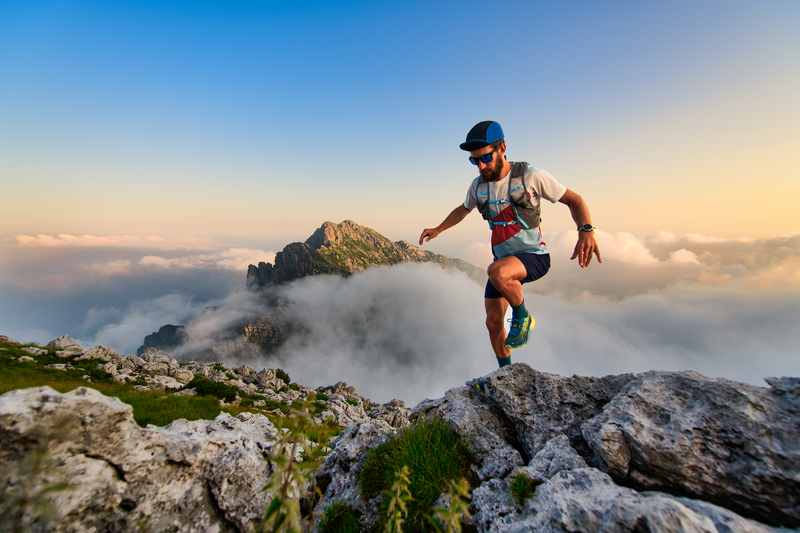
94% of researchers rate our articles as excellent or good
Learn more about the work of our research integrity team to safeguard the quality of each article we publish.
Find out more
REVIEW article
Front. Vet. Sci. , 19 June 2023
Sec. Veterinary Pharmacology and Toxicology
Volume 10 - 2023 | https://doi.org/10.3389/fvets.2023.1160350
This article is part of the Research Topic Green Veterinary Pharmacology and Toxicology: A “One Health” Approach Milestone View all 9 articles
Mastitis is considered the costliest disease on dairy farms and also adversely affects animal welfare. As treatment (and to a lesser extent prevention) of mastitis rely heavily on antibiotics, there are increasing concerns in veterinary and human medicine regarding development of antimicrobial resistance. Furthermore, with genes conferring resistance being capable of transfer to heterologous strains, reducing resistance in strains of animal origin should have positive impacts on humans. This article briefly reviews potential roles of non-steroidal anti-inflammatory drugs (NSAIDs), herbal medicines, antimicrobial peptides (AMPs), bacteriophages and their lytic enzymes, vaccination and other emerging therapies for prevention and treatment of mastitis in dairy cows. Although many of these approaches currently lack proven therapeutic efficacy, at least some may gradually replace antibiotics, especially as drug-resistant bacteria are proliferating globally.
Milk and its derivatives are rich in nutrients and a common food for people of all ages (1). In addition to its nutrient content, milk of BCoV-vaccinated cows had BCoV antibodies and drinking this milk helped people acquire SARS-CoV-2 heterologous antibodies and thus develop passive immunity against COVID-19 (2). Milk antibodies also conferred protection against rotavirus, Shigella flexneri, Escherichia coli, Clostridium difficile, Streptococcus mutans, Cryptosporidium parvum, and Helicobacter pylori (3, 4).
Despite broad consumption of milk and milk products, mastitis in dairy cows, typically incited by bacteria (5), raises many concerns about milk quality. Mastitis can be divided into 3 stages: invasion, infection (colonization) and inflammation (6). Mastitis is classified as subclinical or clinical, based on whether clinical signs are absent of present. Subclinical mastitis causes some changes in the milk, including a white blood cell count > 500,000/mL (7), whereas cows with clinical mastitis may exhibit milk clots, udder swelling and systemic symptoms to varying degrees (8). Mastitis caused by infectious pathogens, including Staphylococcus aureus, Streptococcus agalactiae and Mycoplasma bovis, is infectious (9) (Figure 1), whereas mammary infections caused by environmental pathogens such as E. coli, Klebsiella pneumoniae and coagulase-negative staphylococci are called environmental mastitis (10, 11).
Figure 1. Mastitis in dairy cows. The circled numbers are to guide the reader through the sequence of mastitis. “*” represents the main causes of mastitis in cows (Created with BioRender.com).
Current mastitis treatment relies on antibiotics and is the most important reason for antibiotic use in dairy cows. However, emergence of drug-resistant strains is threatening viability of antibiotics for mastitis treatment. Antimicrobial resistance (AMR) occurs when pathogens are able to overcome effects of antibiotics that were originally effective. It was reported that AMR was first detected in penicillin resistance of Streptococcus pneumoniae, and the isolation rate of drug-resistant strains increased by 11 percent over the following decade (12). Genes responsible for drug resistance can be transferred between bacteria of different taxonomic and ecological groups by mobile genetic elements such as phages, plasmids, naked DNA or transposons (13). Thus, resistant strains of animal origin and resistant strains of human origin may interact and transfer resistance.
With emergence of drug-resistant strains signals, it is clear that antibiotics will no longer be fully effective against mastitis. This is attributed to decades of antimicrobial use and misuse in human and veterinary medicine (13). Consequently, there is a global focus on finding alternatives to treat bacterial diseases. Finland substantially reduced macrolide use, resulting in nearly a 50% decrease in erythromycin resistance (14). This was proof of concept that reducing antibiotic use can reduce AMR.
In a study conducted on 40 large United States dairy farms, antibiotic treatment of dry cows and clinical mastitis cases accounted for > 75% of all antibiotic usage (15) (Figure 2). On dairy farms, direct costs of mastitis treatment include: drug treatment costs, veterinary service fees, and the value of discarded milk containing antibiotics or other ingredients (16). As antibiotics and veterinary fees account for 24% of financial losses from clinical mastitis (17–19), reducing antibiotic use could save considerable money. Combined with the presence of drug-resistant strains that prolong treatment, there is also a potential mortality and morbidity impact (13). In addition, as withdrawal of antibiotics for treating and preventing bovine mastitis presents substantial challenges for farmers, it is essential to provide viable alternatives.
Figure 2. Annual antibiotic usage on dairy farms in the United States. Reprinted from de Campos et al. (15) under CC-BY-NC-ND.
Antimicrobial treatment of mastitis in dairy cows is generally regarded as necessary to maintain a balance among economics, animal welfare, and udder health (20). However, emergence of AMR strains is becoming one of the biggest threats to global health, food security, and societal development (21). Many mastitis-derived pathogens from various countries are resistant to common antibiotics (Table 1). Furthermore, common mastitis pathogens collected by our research team (Table 2) had a greater prevalence of AMR than mastitis pathogens from Europe (5, 36), confirming that mastitis caused by multi-drug resistant strains is a problem in large Chinese dairy herds (5).
Table 2. Comparison of drug resistance in common antimicrobial resistance (AMR) strains from bovine mastitis in China and Europe.
Given the urgency to reduce antibiotic use in the dairy industry, we review options for mastitis treatment and prevention, with goals of reducing emergence of antibiotic-resistant strains and minimizing financial losses. In this paper, we review clinical effects and application prospects of non-steroidal anti-inflammatory drugs (NSAIDS), herbal medicines, antimicrobial peptides (AMPs), bacteriophages (and phage endolysins), vaccination and other emerging therapies for treatment of bovine mastitis. Vaccination, herbal medicines, and AMPs can prevent mastitis by regulating the immune system. In addition, herbal medicines and AMPs can also act directly on bacteria to produce therapeutic effects. Phage (and phage endolysins) and NSAIDS are more effective on treatment. It is worth mentioning that although they can prevent and/or treat mastitis, the immature clinical application means that they are still an alternative to the prevention and treatment of mastitis.
Not all cases of clinical mastitis benefit from antibiotics, as 10–40% of cultures in clinical mastitis cases have no bacterial growth and do not require antibiotic therapy, and another 40% of positive cultures (mainly gram-negative bacteria and yeasts) are not sensitive to antibiotics approved for intramammary use (8). Intramammary antibiotic therapy is generally recommended only for infections caused by gram-positive bacteria such as S. aureus, S. agalactiae and environmental Streptococci spp. (37). In contrast, most Gram-negative infections are cleared by the cow’s own immune system (38). Therefore, antibiotics approved for use in the udder of dairy cows are effective in only 20–50% of clinical mastitis (8).
The specific mechanism of action of NSAIDs is inhibition of cyclooxygenase (COX), reducing production of prostaglandins (an inflammatory mediator) (39). COX has 2 isoforms, COX-1 and COX-2; the former is naturally expressed in all tissues, and has a role in maintaining normal physiological functions, whereas the latter is induced by inflammatory stimuli and cytokines (40). NSAIDs that are more selective inhibitors of COX-2 have greater therapeutic effects, whereas those that are highly selective inhibitors of COX-1 have more side effects, including an increased risk of retained placenta, uterine inflammation, and gastric irritation (41) (Figure 3).
Figure 3. Mechanisms of action of non-steroidal anti-inflammatory drugs (NSAIDs) and commonly used NSAIDs (Created with BioRender.com).
The NSAIDs used to treat bovine mastitis include flunixin meglumine, meloxicam, ketoprofen, and carprofen. Flunixin meglumine, the only NSAID approved by FDA in the US for dairy cows to control fever associated with mastitis and endotoxemia associated with E. coli mastitis, is commonly used as an analgesic in US food animals (42, 43). It inhibits both COX-1 and COX-2, but is more selective for COX-1, thereby increasing risk of retained placenta and digestive disorders (41). However, using only a single dose of flunixin meglumine can reduce these side effects (44). In cows with lipopolysaccharide-induced mastitis, flunixin meglumine increased feeding time and rumination during the first 9 and 12 h, and improved ruminal activity (45, 46). In addition, flunixin meglumine decreased blood nonesterified fatty acids and Isop concentrations in cows with E. coli mastitis, indicating a reduced inflammatory response (45).
Meloxicam is a more selective inhibitor of COX-2, greatly avoiding side effects associated with COX-1 inhibition (47). In a randomized trial on 2,653 cows from 20 herds, 1 mg/kg meloxicam orally at calving reduced the incidence of subclinical mastitis, increased feed intake and milk production, and reduced systemic inflammation (48). Furthermore, meloxicam alleviated the pain of LPS-induced clinical mastitis, mitigated udder edema, and reduced rectal temperature (49). When meloxicam was used to treat mild to moderate mastitis in the first 120 days of lactation, calving interval of infected cows were reduced, and the conception rate of infected cows was improved, which had positive benefits for pasture-based dairy production (50).
Ketoprofen inhibits both COX-1 and COX-2 (51) and has been used for treatment of bovine mastitis due to its rapid onset of action, short plasma half-life, low toxicity, and no milk withdrawal. It has been approved for use in Canada, Brazil and other countries (52). Intramammary administration of ketoprofen reduced SCC and damage to the blood-milk barrier, decreasing concentrations of IgG in milk during LPS-induced mastitis (53). Ketoprofen alone had positive effects on chronic mastitis (54), although effects on acute mastitis were less clear (52, 55).
Carprofen, like meloxicam, is a COX-2 selective, single-dose, long-acting NSAID to treat bovine mastitis (42, 56). In cows with mastitis, carprofen reduced heart rate, rectal temperature and udder swelling (57). In cows with E. coli mastitis, carprofen reduced rectal temperature and promoted ruminal motility (58).
There is a growing recognition of NSAIDs to manage inflammation, pain and endotoxin production in cows with mastitis (59). In Denmark, 72% of veterinarians use NSAIDs alone for mastitis, especially if caused by gram-negative bacteria (60). Some NSAIDs synergize with antibiotics in treatment of mastitis, such as meloxicam or ketoprofen plus gentamicin (59). In addition, some NSAIDs (e.g., meloxicam) can block virulence genes, prevent hemolysis, downregulate expression of genes related to biofilm formation, and inhibit S. aureus growth (59). We inferred that NSAIDs have potential to fully substitute for antibiotics in treating mastitis in cows in the absence of bacterial growth or for most gram-negative infections. Furthermore, since the primary mechanism of action for NSAIDs against bovine mastitis is non-bacterial, resistant strains should not affect efficacy.
Herbal medicines are derived from natural plants and have a long history of medicinal value, with limited or no side effects compared to antibiotics. The medicinal value of herbs are often due to their metabolites (e.g., phenolic acids, alkaloids, flavonoids, terpenoids, and volatile oils) that have antibacterial, antioxidant, and anti-inflammatory capabilities (61).
Many herbal medicines have antibacterial ability. For example, Red ginger had good bactericidal effects on Staph epidermidis, S. aureus, and S. agalactiae derived from bovine mastitis (62); the bactericidal mechanism is curcumin and gingerol that kill bacteria by disrupting their extracellular membrane (62). Biofilm is a key virulence factor to increase resistance of mastitis-derived methicillin-resistant S. aureus (MRSA); however, Maize whiskers significantly inhibited biofilm production by MRSA strains (63). Essential oils are secondary metabolites of plants with antimicrobial properties that do not stimulate drug resistance with prolonged use (64). Essential oils (Oregano essential, Thyme essential, Carvacrol essential, and Thymol) killed more than 30 species of Staphylococci (64). Several other herbal medicines and their extracts, including Terminalia Chebula, Purslane and Dandelion also had bactericidal activity against various mastitis pathogens (65).
Mastitis occurs when the immune system of the mammary gland fails to defend against bacterial invasion; therefore, it is very important to enhance immune activity to prevent and treat mastitis. Dandelion has free radical scavenging, antioxidant, antibacterial, and anti-inflammatory functions (66) and in a murine mammary gland infection model with S. aureus, Dandelion downregulated the inflammatory response (67). Vitexin treatment increased T-AOC, SOD, GSH-PX, CAT enzyme activity during S. aureus infection, both in vitro and in vivo (68). Baicalin, the bioactive component of Scutellaria baicalensis georgi, reduced expression of inflammatory factors and apoptosis of bMECs in cows with LPS-induced mastitis. Baicalein protected the mammary gland, reducing mastitis-induced damage (69, 70). The curative effect of mangostin on LPS-induced mastitis was attributed to suppression of inflammatory cytokine production, particularly the NF-κB and NLRP3 inflammasome (71). Geniposide anpolydatin was anti-inflammatory by interfering with expression of TLR4 and TLR2 and reducing expression of TNF-α, IL1β, and IL-6 (72, 73).
Immunity has a decisive role in occurrence, development and clearance of mastitis. Cows with robust immunity are often able to clear pathogenic bacteria during invasion of the udder. In addition to their powerful antibacterial influence, essential oils can be used as an alternative to antibiotics to improve feed efficiency, nutrient use, and animal health (64, 74). Dietary supplementation with black seed oil, chamomile oil, or cretian origanum oil starting 8 weeks before calving enhanced immunity in dairy cows (74). Furthermore, addition of essential oils to cow diets improved milk production, milk quality, udder health, and immunity (74). A Chinese herbal preparation containing 18 herbal medicines, including Astmgali radix, Platycladi cacumen, Crataegi fructus, and Chuanxiong, greatly promoted productivity in late-lactation cows exposed to heat stress (75).
In summary, herbal medicines contain bioactive components with great value in preventing and treating bovine mastitis, with mechanisms of action similar to antibiotics, but without the presence of antibiotic residues in milk (76). However, some bacteria are naturally resistant to herbal compounds and others develop resistance over time (77–79). Moreover, few herbal medicines have been approved by the FDA for clinical use, mainly due to the complexity of their composition and the difficulty to accurately assess efficacy and safety (80), although at least some of these issues can be readily addressed.
Antimicrobial peptides are another promising replacement for antibiotics. Most cells produce naturally occurring antibiotic-like molecules, known as AMPs, key components of innate immunity (81). Their antimicrobial activity is attributed to net charge, hydrophobicity, and amphiphilicity (82). As of December 2022, the continuously updated Antimicrobial Peptide Database (APD, https://aps.unmc.edu/home) included 3,425 AMPs from 6 kingdoms, 147 human host defense peptides, 385 bacteriocins/peptide antibiotics isolated/predicted from bacteria, 5 from archaea, 8 from protozoa, 25 from fungi, 368 from plants, and 2,489 from animals, including some synthetic peptides.
Nisin, a natural antimicrobial peptide produced by Lactococcus lactis, had excellent antimicrobial activity against gram-positive bacteria isolated from mastitis in dairy cows (83). In a bovine mastitis trial, there was no difference between Nisin and an antibiotic group for rates of bacteriological or clinical cure (84). An isolate of S. aureus from mastitis that was resistant to a variety of antibiotics was readily killed by Nisin (84). For treatment of subclinical mastitis, Nisin not only reduced somatic cell count, but also had good bacteriological cure rates against S. agalactiae, S. aureus, and coagulase-negative Staphylococci (CNS) (85).
Polybia MP-1, a 14-amino acid AMP from wasp venom, was bactericidal against multidrug-resistant S. aureus, E. coli and K. pneumoniae strains from bovine mastitis (25, 35). Esculentin 1–21, an AMP from frog skin, had broad-spectrum antimicrobial activity (86), particularly against Pseudomonas aeruginosa, E. coli, and S. agalactiae in vivo and in vitro (87). In a clinical trial, Esculentin 1–21 had a 100% improvement rate after 5 days without side effects (87). Although an increasing number of mastitis-derived strains have multidrug resistance, AMPs had good bactericidal ability against them.
AMPs secreted in the mammary tissue of cows include β-defensins, psoriasin, cathelicidins, and lactoferrin (88). Bactericidal and therapeutic functions of AMPs secreted by mammary gland of cows, especially β-defensins (89), have been studied. Tracheal antimicrobial peptide (TAP), a cationic β-defensin, can be produced by bMECs (90). In both in vitro and in vivo infection models, TAP effectively killed S. aureus and reduced induced apoptosis of bMECs (91). Plectasin, a cationic AMP with 40 amino acids isolated from fungus, has low cytotoxicity (92). MP1102 is similar to plectasin and had strong antibacterial activity against MRSA, even inside bMECs (66, 93). Recently, a series of specific and targeted antimicrobial peptides based on the pheromone and cell-penetrating peptides of S. agalactiae were produced and designated cell-penetrating selective antimicrobial peptides L1–L12 (94). L1, L2, and L11 killed S. agalactiae by membrane disruption, whereas L2 and L10 entered cells and activated endocytosis (94).
Although AMPs secreted directly from mammary tissue have tissue homology and high bactericidal efficiency, purification methods, production, and in vitro preservation stability restrict clinical applications (95). Therefore, future research should use eukaryotic expression vectors or genetic engineering to develop novel AMPs based on natural AMPs. Bacteria can become resistant to AMPs, and potential cross-resistance between AMPs and conventional antibiotics has been reported (23). Gram-negative bacteria can resist effects of AMPs by surface remodeling, biofilm structure, efflux pumps, interception (binding and isolation of antimicrobial peptides so that they cannot act on the bacterial membrane), proteolytic degradation, and modulation of cationic antimicrobial peptides expression (96).
Bacteriophages are viruses that can lyse bacteria; based on their life cycle, they are classified as either lytic or lysogenic (temperature) phages (97). Lytic phages usually attach an adsorption structure to a specific receptor on the surface of the bacterium, inject DNA into the host bacterium through the tail structure, lyse the host, and release a large number of phages (98). Unlike lytic phages, lysogenic phages assemble their own genes in the host bacterial genome and coexist without causing host bacterial lysis (98) (Figure 4). Due to their direct bactericidal effect, lytic phages are preferred for treatment of bacterial infectious diseases. In contrast, lysogenic phages are usually used as vectors to transmit genes encoding inhibition of bacterial virulence, to develop small molecular proteins of simulated bacteriophage derivatives for bacterial virulence, and to design vaccines (99). Phages are usually safe, effective, non-residual, highly specific, and without effect on non-target bacteria, with great potential to replace antibiotic therapy for mastitis in cows. Two strains of S. aureus phages, SAJK-IND and MSP, were isolated from mastitis milk and environmental sewage and were 100% bactericidal against 120 S. aureus strains (100). In another study, 36 MRSA strains were isolated from milk and teat skin of cows with subclinical mastitis and had 100% susceptibility to S. aureus phages (22). Teng et al. (101) isolated S. aureus phage 4086-1 from mastitis milk, which efficiently killed MRSA in the murine mammary gland and had a good therapeutic effect. S. aureus phages ΦSA012 and ΦSA039 had broad lytic spectrums (102). In vivo, phage ΦSA012 removed S. aureus from the murine mammary gland, suppressing the inflammatory response and tissue damage (102).
Figure 4. Mechanism of phage lysis of host bacteria and published types of mastitis pathogenic bacteria targeted by phages. 1. Phage attaches to host bacterium and injects DNA. 2. Phage DNA enters the lytic or lysogenic cycle. 3a. DNA and protein synthesis followed by assembly of new phages. 4a. Lysis of the host bacterium, releasing a large number of new phages. 3b. Phage DNA is integrated into the host bacterium chromosome. 4b. Lysogenic bacterial have normal reproduction. 5. Under specific conditions, the prophage is isolated from the host bacterium genome and enters the lysis cycle (Created with BioRender.com).
Regarding the use of phages to control other pathogens causing bovine mastitis, Bai et al. (103) isolated a S. agalactiae phage JX01, reported its complete genomic sequence, and determined it can lyse 65.3% of bovine S. agalactiae with no killing effect on human or fish strains (i.e., high specificity). The T4 phage vB_EcoM-UFV13, a novel E. coli phage with a broad host range, decreased the bacterial load by 90% in murine mammary glands and had a positive result on E. coli-induced mastitis in dairy cows (104). PAJD-1, a phage isolated from sewage samples on a dairy farm, lysed 80% of P. aeruginosa strains (105). The edema and hemorrhagic response of mouse mammary tissues caused by P. aeruginosa was greatly alleviated by the action of PAJD-1 in vivo, similar to antibiotics (105). Our research team isolated 5 strains of K. pneumoniae phages from the sewage samples of dairy farms, and conducted biological identification, genome sequencing and therapeutic research (106–108). We reported that K. pneumoniae phages mitigated K. pneumoniae-induced inflammation in bMECs and reduced structural damage and inflammatory responses of murine mammary gland tissue (107, 108).
The law of survival of the fittest suggests that the coexistence of phages and bacteria for millions of years results from their co-evolution, i.e., the phage cannot completely eliminate the host bacterium because there is always a portion of the host bacterium that has evolved into a mutant strain that is unaffected by the phage (109). Notwithstanding the superior lytic competence of phages on pathogenic bacteria, even strains in the biofilm state, there is no shortage of phage mutants (110). In that regard, it was stated that E. coli can develop resistance to phages within a short interval (111). Experiments by Pires et al. (112) also noted development of resistance. Furthermore, after 24 h of phage action, P. aeruginosa developed two strains of bacteriophage-insensitive mutants (BIM). The co-evolutionary nature of phages and bacteria coupled with the abundance and diversity of phages in nature may be a critical solution to addressing bacterial resistance to phages (113). One method is to replace the phage to which the bacteria have developed resistance, and another approach is to use a cocktail of multiple phages with different receptors and complementary hosts (110). Phage cocktails can not only expand the scope of response but diminish emergence of phage-mutant strains (114). Phage cocktails are mixtures of phages that broaden the host range and minimize production of phage-resistant bacteria (114). By mixing 3 strains of phages, Garcia et al. (115) demonstrated the bactericidal power of the cocktail was significantly enhanced. In treatment of mastitis, an E. coli cocktail consisting of phages (SYGD1, SYGE1 and SYGMH1) had more powerful bactericidal activity and clinical therapeutic effect than a single phage (34).
Endolysins encoded by phages also have strong potential for clinical application due to broad lyase spectrum, safety and stability (116). PlySs2 and PlySs9, 2 bacteriocins from Streptococcus suis prophage, had broad lytic activity against Streptococcus uberis isolated from bovine mastitis (117). LysRODI, encoded by the Staphylococcal phage phiIPLA-RODI, had superior lysis capacity against Staphylococci strains from dairy farms and decreased mammary tissue damage caused by Staphylococcus infection in mice (118).
In recent years, more and more animal models and clinical trials have been conducted to evaluate the therapeutic effects of phages, and some phage products have been approved for clinical treatment. However, there are still many difficulties in using phages as first-line agents, due to: (i) lack of chemotaxis, preventing phages from dispersing and reaching sites of infection (102); (ii) intravenous administration of phages is limited by the body’s immune system and focused on direct action at the site of infection, with deep tissues and intracellular bacteria being less accessible (119); (iii) phages are replication-competent nucleoprotein complexes, and their “pharmacology,” e.g., dose, is not well understood (120); (iv) the safety of phage products is affected by many elements, e.g., purity and sterility (121); (v) phages have not yet reached a gold standard for double-blind efficacy assays (122); and (vi) phage therapies do not yet have a dedicated legal regulatory framework and have only been implemented in a few countries (121, 123).
Effective vaccines can reduce the incidence of mastitis, thereby effectively reducing antibiotic use. Vaccines have been developed for some pathogens causing clinical mastitis, e.g., E. coli, S. aureus, and Streptococcus spp. Among them, J5 mutant strains-based vaccines represent a breakthrough in E. coli vaccine development (124). In clinical trials, E. coli J5 vaccination reduced the incidence of gram-negative mastitis in dairy cows, with protection lasting up to the third month of lactation (125). In another study, J5 vaccination failed to reduce the incidence of E. coli mastitis, although it mitigated severity (126). Vaccines for controlling S. aureus mastitis consist of either whole cells (autologous vaccines) or subunits (recombinant proteins and bacterial surface extracts) (124). Small colony variants of S. aureus have potential for development of a live vaccine capable of preventing mastitis in dairy cows. Côté-Gravelet et al. (127) developed a novel attenuated mutant by knocking out the hemB and vraG genes and demonstrating its potential as an attenuated vaccine for ameliorating udder infections caused by S. aureus. An experimental vaccine based on S. aureus surface-associated protein had promise, enhancing serum-associated protein titers and maintaining efficacy for ~ 4 months (128). Another study used recombinant protein technology to confirm that genes associated with iron acquisition had good immunogenicity in both rabbits and cattle. 54 strains of S. aureus were screened for 5 iron acquisition system-related genes: isd, feo, sir, sst and fhu. IsdH protein from the Isd system induced a long-lasting immune response when inoculated in cattle, implying IsdH was a good candidate for a S. aureus mastitis vaccine (129). Streptococci species closely associated with mastitis in dairy cows are primarily S. uberis, and also S. agalactiae and Streptococcus dysgalactiae (130, 131). By using the strain of S. uberis that formed the greatest biofilm as the source of the vaccine, Collado et al. (132) evaluated a subunit vaccination based on lipophosphatidic acid (LTA) for S. uberis against experimental intra-dairy heterozygous strains of infection in dairy cows. Protection was incomplete, but vaccination significantly reduced clinical signs and hastened recovery of the milk compared to the control group (132). Cows given live S. uberis via subcutaneous injection had higher serum antibody titers and less severe clinical signs compared to unvaccinated cows (133). However, this vaccine was effective against homologous but not heterologous strains (133).
Vaccines have much potential for preventing mastitis in dairy cows. However, it is evident that the number of pathogenic bacteria causing mastitis in cows far exceeds bacteria targeted by existing vaccine development. Furthermore, pathways and mechanisms of infection for these pathogenic bacteria are not uniform, posing challenges to developing effective vaccines for mastitis in cows. Additionally, there are numerous constraints, such as timing of administration and duration of effect.
That intestinal flora can induce bovine mastitis through endogenous paths highlights potential to use probiotics to treat mastitis in dairy cows (134). Feeding Bacillus subtilis to heifers and transitional cows subtilis for 3 weeks before calving and throughout lactation reduced the incidence of clinical mastitis, SCC, and days of discarded milk (135). Furthermore, Lactobacilli, Yeast, and LAB (a mixture of Lactobacilli and maltodextrin) optimized the mammary microbiota and increased mammary resistance of dairy cows (136). Lactobacillus casei, a probiotic that regulates the digestive system, can adhere to and internalize into bMECs without altering cell viability and morphology, but prohibiting S. aureus infection (137). Moreover, L. casei activated innate immunity of bMECs and reduced susceptibility to infection (138). A commercial post-dip solution containing L. casei, L. brucei, and L. paracasei has been used on dairy farms and reduced the incidence of mastitis (139).
Mesenchymal stem cells (MSCs) are non-specialized pluripotent cells capable of self-renewal and differentiation into specific cell types, with potential for tissue regeneration. As they are easily accessible, their therapeutic competence is of interest (140). MSCs from fetal bovine bone marrow (BM-MSC) and adipose tissue (AT-MSC) reduced growth of S. aureus in vitro (141). Intramammary administration of AT-MSC in dairy cows killed S. aureus in the udder without side effects (142). A recent study used MSCs from umbilical cords and their extracellular vesicles to treat subclinical mastitis (143). MSCs may have an immunomodulatory role by releasing bioactive components and promoting repair of damaged tissues in dairy cows with mastitis (142, 143).
Nanotechnology-based drug delivery enables drugs to be deposited, sustained and slowly released at target locations, thereby overcoming some limitations of conventional drugs, including antibiotic resistance (144). Self-assembly tilmicosin nanogel was used on cows with S. aureus mastitis and had a higher cure rate compared to a conventional treatment group (145). Cinnamon oil and silver nanoparticles were bactericidal against S. agalactiae (146). Polyherbal nanocolloids from Dandelion, Cinnamon, Phyllanthus emblica, Terminalia, and Citronella had efficient, dose-dependent antibacterial ability against mastitis-derived pathogens (147).
Photodynamic therapy (PDT) has much potential for treating bovine mastitis (148). A non-toxic photosensitizer is activated to produce ROS that kills bacteria by altering its cell membranes and DNA (149). In cows with subclinical mastitis, PDT was bactericidal against S. uberis and coagulase negative S. aureus (CNS) (150). Furthermore, in sheep with mastitis, PDT reduced CNS, Streptococcus spp. And E. coli within udders (151). Though PDT has much promise to treat mastitis, the method is still in initial research stages. Improvements in the photosensitizer, light sources and oxygen supply are needed to strengthen the effectiveness of action and reduce adverse side effects (152).
Acoustic pulse therapy (APT) is another antibiotic-free strategy to treat bovine mastitis. Cows with mastitis can be treated by APT devices using low-power acoustic pulses to penetrate deep tissue and disperse pressure waves over a broad region of udders (153). In addition, APT can activate immune cells and repair damaged tissue (153). Similarly, APT was more effective for treating mastitis caused by E. coli compared to Streptococcus (154), with APT-treated cows producing an addition 500 L milk in a 305-day lactation (154).
Commercial dairy farms are likely to have ongoing issues with mastitis. At present, antibiotic therapy is the first line of treatment, but there is much concern about emergence of multi-drug resistant strains on dairy farms and the potential for that resistance to be spread to pathogens affecting humans. Therefore, there is a great impetus to identify alternatives for treating mastitis in dairy cows.
From our perspective, NSAIDs, herbal medicines, AMPs, bacteriophages and vaccination have much potential for easing the plight of antibiotic resistance. Many veterinarians already use NSAIDs as adjunctive therapy for mastitis. Furthermore, some practitioners are using NSAIDs alone for treating mastitis. The advantages of herbal medicines are undeniable, but much effort is needed to produce commercially viable products. Although AMPs can also have positive effects, their ability to damage eukaryotic cells needs to be addressed. Phages are well known for their selective effects on target bacteria, making them the most prospective successor to antibiotics for bovine mastitis. In fact, they have already contributed to save many lives in human infection. Vaccines can prevent mastitis, limit the severity of clinical signs, and hasten cure. However, due to the wide range of mastitis-causing organisms, especially environmental pathogens that are becoming dominant, vaccine control of mastitis faces many challenges. Some other possibilities were also briefly described in the review. Probiotics may work by modulating the intestinal flora, with a proof of concept of the direct effect of probiotics on mastitis in dairy cows. Although at the initial stage of research, nanotechnology has great application potential in the treatment of mastitis in combination with other antibacterial substances due to their good drug-carrying capacity. PDT and APT are emerging as potential approaches in the treatment of mastitis in dairy cows, but more research is needed to make them practical and effective.
XL wrote the manuscript with support from CX, BL, JK, BH, XT, and JG. All authors contributed to the article and approved the submitted version.
This study was financially supported by the Beijing-Tianjin-Hebei Collaborative Innovation Community Project (21346601D) and the National Natural Science Foundation of China (32273082 and U21A20262).
We thank Professor Yuxiang Shi from Hebei University of Engineering for providing the current status of antibiotic use on many dairy farms.
The authors declare that the research was conducted in the absence of any commercial or financial relationships that could be construed as a potential conflict of interest.
All claims expressed in this article are solely those of the authors and do not necessarily represent those of their affiliated organizations, or those of the publisher, the editors and the reviewers. Any product that may be evaluated in this article, or claim that may be made by its manufacturer, is not guaranteed or endorsed by the publisher.
AMP, antimicrobial peptides; AMR, antimicrobial resistance; APT, acoustic pulse therapy; BIM, bacteriophage-insensitive mutants; bMEC, bovine mammary epithelial cell; CNS, coagulase-negative Staphylococci; COX, cyclooxygenase; MRSA, methicillin-resistant S. aureus; MSC, BM-MSC, AT-MSC, mesenchymal stem cells, or fetal bovine bone marrow, or adipose tissue; NSAID, non-steroidal anti-inflammatory drug; PDT, photodynamic therapy; SCC, somatic cell count.
1. Marangoni, F, Pellegrino, L, Verduci, E, Ghiselli, A, Bernabei, R, Calvani, R, et al. Cow’s milk consumption and health: a health professional’s guide. J Am Coll Nutr. (2019) 38:197–208. doi: 10.1080/07315724.2018.1491016
2. Arenas, A, Borge, C, Carbonero, A, Garcia-Bocanegra, I, Cano-Terriza, D, Caballero, J, et al. Bovine coronavirus immune milk against COVID-19. Front Immunol. (2021) 12:637152. doi: 10.3389/fimmu.2021.637152
3. Ebina, T, Ohta, M, Kanamaru, Y, Yamamoto-Osumi, Y, and Baba, K. Passive immunizations of suckling mice and infants with bovine colostrum containing antibodies to human rotavirus. J Med Virol. (1992) 38:117–23. doi: 10.1002/jmv.1890380209
4. Korhonen, HJ, and Marnila, P. 10 - Bovine milk immunoglobulins against microbial human diseases. In: Dairy-Derived Ingredients. ed. M. Corredig (Sawston: Woodhead Publishing). (2009), 269–89.
5. Cheng, J, Qu, W, Barkema, HW, Nobrega, DB, Gao, J, Liu, G, et al. Antimicrobial resistance profiles of 5 common bovine mastitis pathogens in large Chinese dairy herds. J Dairy Sci. (2019) 102:2416–26. doi: 10.3168/jds.2018-15135
6. Ruegg, PL. A 100-year review: mastitis detection, management, and prevention. J Dairy Sci. (2017) 100:10381–97. doi: 10.3168/jds.2017-13023
7. Plastridge, WN. Bovine mastitis: a review. J Dairy Sci. (1958) 41:1141–81. doi: 10.3168/jds.S0022-0302(58)91071-3
8. Roberson, JR. Establishing treatment protocols for clinical mastitis. Vet Clin North Am Food Anim Pract. (2003) 19:223–34, viii. doi: 10.1016/s0749-0720(02)00071-3
9. Ashraf, A, and Imran, M. Causes, types, etiological agents, prevalence, diagnosis, treatment, prevention, effects on human health and future aspects of bovine mastitis. Anim Health Res Rev. (2020) 21:36–49. doi: 10.1017/S1466252319000094
10. Bogni, C, Odierno, L, Raspanti, C, Giraudo, J, Larriestra, A, Reinoso, E, et al. War against mastitis: current concepts on controlling bovine mastitis pathogens In: A Me’ndez-Vilas, editor. Science against microbial pathogens: Communicating current research and technological advances. Singapore: World Scientific (2011). 483–94.
11. De Visscher, A, Supré, K, Haesebrouck, F, Zadoks, RN, Piessens, V, Van Coillie, E, et al. Further evidence for the existence of environmental and host-associated species of coagulase-negative staphylococci in dairy cattle. Vet Microbiol. (2014) 172:466–74. doi: 10.1016/j.vetmic.2014.06.011
12. Appelbaum, PC. Antimicrobial resistance in Streptococcus pneumoniae: an overview. Clin Infect Dis. (1992) 15:77–83. doi: 10.1093/clinids/15.1.77
13. Levy, SB, and Marshall, B. Antibacterial resistance worldwide: causes, challenges and responses. Nat Med. (2004) 10:S122–9. doi: 10.1038/nm1145
14. Seppälä, H, Klaukka, T, Vuopio-Varkila, J, Muotiala, A, Helenius, H, and Lager, K. The effect of changes in the consumption of macrolide antibiotics on erythromycin resistance in group a streptococci in Finland. N Engl J Med. (1997) 337:441–6. doi: 10.1056/nejm199708143370701
15. de Campos, JL, Kates, A, Steinberger, A, Sethi, A, Suen, G, Shutske, J, et al. Quantification of antimicrobial usage in adult cows and preweaned calves on 40 large Wisconsin dairy farms using dose-based and mass-based metrics. J Dairy Sci. (2021) 104:4727–45. doi: 10.3168/jds.2020-19315
16. Blosser, TH. Economic losses from and the national research program on mastitis in the United States. J Dairy Sci. (1979) 62:119–27. doi: 10.3168/jds.S0022-0302(79)83213-0
17. Cha, E, Bar, D, Hertl, JA, et al. The cost and management of different types of clinical mastitis in dairy cows estimated by dynamic programming. J Dairy Sci. (2011) 94:4476–87. doi: 10.3168/jds.2010-4123
18. Huijps, K, Lam, TJ, and Hogeveen, H. Costs of mastitis: facts and perception. J Dairy Res. (2008) 75:113–20. doi: 10.1017/S0022029907002932
19. Rollin, E, Dhuyvetter, KC, and Overton, MW. The cost of clinical mastitis in the first 30 days of lactation: an economic modeling tool. Prev Vet Med. (2015) 122:257–64. doi: 10.1016/j.prevetmed.2015.11.006
20. Krömker, V, and Leimbach, S. Mastitis treatment— reduction in antibiotic usage in dairy cows. Reprod Dom Anim. (2017) 52:21–9. doi: 10.1111/rda.13032
22. Varela-Ortiz, DF, Barboza-Corona, JE, González-Marrero, J, León-Galván, MF, Valencia-Posadas, M, Lechuga-Arana, AA, et al. Antibiotic susceptibility of Staphylococcus aureus isolated from subclinical bovine mastitis cases and in vitro efficacy of bacteriophage. Vet Res Commun. (2018) 42:243–50. doi: 10.1007/s11259-018-9730-4
23. Bennett, S, Ben Said, L, Lacasse, P, Malouin, F, and Fliss, I. Susceptibility to nisin, bactofencin, pediocin and reuterin of multidrug resistant Staphylococcus aureus, Streptococcus dysgalactiae and Streptococcus uberis causing bovine mastitis. Antibiotics (Basel). (2021) 10:1418. doi: 10.3390/antibiotics10111418
24. Gogoi, P, Shrivastava, S, Shah, P, Saxena, S, Srivastava, S, and Gaur, GK. Linear and branched forms of short antimicrobial peptide-irk inhibit growth of multi drug resistant Staphylococcus aureus isolates from mastitic cow milk. Int J Pept Res Ther. (2021) 27:2149–59. doi: 10.1007/s10989-021-10243-7
25. Shah, P, Shrivastava, S, Gogoi, P, Saxena, S, Srivastava, S, Singh, RJ, et al. Wasp venom peptide (polybia mp-1) shows antimicrobial activity against multi drug resistant bacteria isolated from mastitic cow milk. Int J Pept Res Ther. (2022) 28:44. doi: 10.1007/s10989-021-10355-0
26. Mohammadian, F, Rahmani, HK, Bidarian, B, and Khoramian, B. Isolation and evaluation of the efficacy of bacteriophages against multidrug-resistant (MDR), methicillin-resistant (MRSA) and biofilm-producing strains of Staphylococcus aureus recovered from bovine mastitis. BMC Vet Res. (2022) 18:406. doi: 10.1186/s12917-022-03501-3
27. Saeed, SI, Mat Yazid, KA, Hashimy, HA, Dzulkifli, SK, Nordin, F, Nik Him, NA, et al. Prevalence, antimicrobial resistance, and characterization of Staphylococcus aureus isolated from subclinical bovine mastitis in East Coast Malaysia. Animals (Basel). (2022) 12:1680. doi: 10.3390/ani12131680
28. Dias, JA, de Menezes, CA, Brito, MAVP, and Lange, CC, Queiroz RBde. Antimicrobial resistance profile of Staphylococcus spp. isolates in cattle herds from Western Amazon. Semin Cienc Agrar. (2022) 43: 1355–1364. doi: 10.5433/1679-0359.2022v43n3p1355
29. da Costa, GM, Ribeiro, NA, Gonçalves, MS, da Silva, JR, da Custódio, DA, and Mian, GF. Antimicrobial susceptibility profile of Streptococcus agalactiae strains isolated from bovine mastitis. Braz J Vet Res Anim Sci. (2021) 58:e178109. doi: 10.11606/issn.1678-4456.bjvras.2021.178109
30. Monistero, V, Barberio, A, Cremonesi, P, Castiglioni, B, Morandi, S, Lassen, DCK, et al. Genotyping and antimicrobial susceptibility profiling of Streptococcus uberis isolated from a clinical bovine mastitis outbreak in a dairy farm. Antibiotics (Basel). (2021) 10:644. doi: 10.3390/antibiotics10060644
31. Pereira de Oliveira, R, Francelino Dias, RF, Da Silva, RA, Bandeira de Melo, RP, Cabral de Araujo, CAS, Nogueira, JF, et al. Bovine mastitis caused by multidrug-resistant Nocardia farcinica. Acta Sci Vet. (2020) 48:520. doi: 10.22456/1679-9216.101045
32. Bag, MAS, Khan, MSR, Sami, MDH, Begum, F, Islam, MS, Rahman, MM, et al. Virulence determinants and antimicrobial resistance of E. coli isolated from bovine clinical mastitis in some selected dairy farms of Bangladesh. Saudi. J Biol Sci. (2021) 28:6317–23. doi: 10.1016/j.sjbs.2021.06.099
33. Ghallache, L, Mohamed-Cherif, A, China, B, Mebkhout, F, Boilattabi, N, Bouchemal, A, et al. Antibiotic resistance profile of Escherichia coli isolated from bovine subclinical mastitis of dairy farms in Algeria from 2017 to 2019. World’s Vet J. (2021) 11:402–15. doi: 10.54203/scil.2021.wvj52
34. Guo, M, Gao, Y, Xue, Y, Liu, Y, Zeng, X, Cheng, Y, et al. Bacteriophage cocktails protect dairy cows against mastitis caused by drug resistant Escherichia coli infection. Front Cell Infect Microbiol. (2021) 11:690377. doi: 10.3389/fcimb.2021.690377
35. Shah, P, Shrivastava, S, Singh, RJ, Gogoi, P, Saxena, S, Srivastava, S, et al. Synthetic antimicrobial peptide polybia mp-1 (mastoparan) inhibits growth of antibiotic resistant Pseudomonas aeruginosa isolates from mastitic cow milk. Int J Pept Res Ther. (2021) 27:2471–86. doi: 10.1007/s10989-021-10266-0
36. de Jong, A, Garch, FE, Simjee, S, Moyaert, H, Rose, M, Youala, M, et al. Monitoring of antimicrobial susceptibility of udder pathogens recovered from cases of clinical mastitis in dairy cows across Europe: vet path results. Vet Microbiol. (2018) 213:73–81. doi: 10.1016/j.vetmic.2017.11.021
37. Lago, A, Godden, SM, Bey, R, Ruegg, PL, and Leslie, K. The selective treatment of clinical mastitis based on on-farm culture results: I. effects on antibiotic use, milk withholding time, and short-term clinical and bacteriological outcomes. J Dairy Sci. (2011) 94:4441–56. doi: 10.3168/jds.2010-4046
38. Pyörälä, S, Kaartinen, L, Käck, H, and Rainio, V. Efficacy of two therapy regimens for treatment of experimentally induced Escherichia coli mastitis in cows. J Dairy Sci. (1994) 77:453–61. doi: 10.3168/jds.S0022-0302(94)76973-3
39. Ricciotti, E, and Fitz Gerald, GA. Prostaglandins and inflammation. Arterioscler Thromb Vasc Biol. (2011) 31:986–1000. doi: 10.1161/ATVBAHA.110.207449
40. Vane, JR. Introduction: mechanism of action of NSAIDs. Br J Rheumatol. (1996) 35:1–3. doi: 10.1093/rheumatology/35.suppl_1.1
41. Trimboli, F, Ragusa, M, Piras, C, Lopreiato, V, and Britti, D. Outcomes from experimental testing of nonsteroidal anti-inflammatory drug (NSAID) administration during the transition period of dairy cows. Animals (Basel). (2020) 10:1832. doi: 10.3390/ani10101832
42. Smith, GW, Davis, JL, Tell, LA, Webb, AI, and Riviere, JE. Extralabel use of nonsteroidal anti-inflammatory drugs in cattle. J Am Vet Med Assoc. (2008) 232:697–701. doi: 10.2460/javma.232.5.697
43. Wagner, BK, Nixon, E, Robles, I, Baynes, RE, Coetzee, JF, and Pairis-Garcia, MD. Non-steroidal anti-inflammatory drugs: pharmacokinetics and mitigation of procedural-pain in cattle. Animals (Basel). (2021) 11:282. doi: 10.3390/ani11020282
44. Giammarco, M, Fusaro, I, Vignola, G, Manetta, AC, Gramenzi, A, Fustini, M, et al. Effects of a single injection of flunixin meglumine or carprofen postpartum on haematological parameters, productive performance and fertility of dairy cattle. Anim Prod Sci. (2018) 58:322–31. doi: 10.1071/an16028
45. Walker, CCF, Brester, JL, and Sordillo, LM. Flunixin meglumine reduces milk isoprostane concentrations in Holstein dairy cattle suffering from acute coliform mastitis. Antioxidants (Basel). (2021) 10:834. doi: 10.3390/antiox10060834
46. Yeiser, EE, Leslie, KE, McGilliard, ML, and Petersson-Wolfe, CS. The effects of experimentally induced Escherichia coli mastitis and flunixin meglumine administration on activity measures, feed intake, and milk parameters. J Dairy Sci. (2012) 95:4939–49. doi: 10.3168/jds.2011-5064
47. Newby, NC, Renaud, D, Tremblay, R, and Duffield, TF. Evaluation of the effects of treating dairy cows with meloxicam at calving on retained fetal membranes risk. Can Vet J. (2014) 55:1196–9.
48. Shock, DA, Renaud, DL, Roche, SM, Poliquin, R, Thomson, R, and Olson, ME. Evaluating the impact of meloxicam oral suspension administered at parturition on subsequent production, health, and culling in dairy cows: a randomized clinical field trial. PLoS One. (2018) 13:e0209236. doi: 10.1371/journal.pone.0209236
49. Fitzpatrick, CE, Chapinal, N, Petersson-Wolfe, CS, DeVries, TJ, Kelton, DF, Duffield, TF, et al. The effect of meloxicam on pain sensitivity, rumination time, and clinical signs in dairy cows with endotoxin-induced clinical mastitis. J Dairy Sci. (2013) 96:2847–56. doi: 10.3168/jds.2012-5855
50. van Soest, FJS, Abbeloos, E, McDougall, S, and Hogeveen, H. Addition of meloxicam to the treatment of bovine clinical mastitis results in a net economic benefit to the dairy farmer. J Dairy Sci. (2018) 101:3387–97. doi: 10.3168/jds.2017-12869
51. Breen, J. The importance of non-steroidal anti-inflammatory drugs (NSAIDs) in mastitis therapeutics. Livestock. (2017) 22:182–5. doi: 10.12968/live.2017.22.4.182
52. Latosinski, GS, Amzalak, MJ, and Pantoja, JCF. Efficacy of ketoprofen for treatment of spontaneous, culture-negative, mild cases of clinical mastitis: a randomized, controlled superiority trial. J Dairy Sci. (2020) 103:2624–35. doi: 10.3168/jds.2019-17504
53. Dan, D, Bruckmaier, RM, and Wellnitz, O. Ketoprofen affects the mammary immune response in dairy cows in vivo and in vitro. J Dairy Sci. (2018) 101:11321–9. doi: 10.3168/jds.2018-15034
54. Zecconi, A, Frosi, S, Cipolla, M, and Gusmara, C. Effects of chronic mastitis and its treatment with ketoprofen on the milk ejection curve. J Dairy Res. (2018) 85:50–2. doi: 10.1017/S0022029917000863
55. Shpigel, NY, Chen, R, Winkler, M, Saran, A, Ziv, G, and Longo, F. Anti-inflammatory ketoprofen in the treatment of field cases of bovine mastitis. Res Vet Sci. (1994) 56:62–8. doi: 10.1016/0034-5288(94)90197-x
56. Suojala, L, Kaartinen, L, and Pyörälä, S. Treatment for bovine Escherichia coli mastitis—an evidence-based approach. J Vet Pharmacol Ther. (2013) 36:521–31. doi: 10.1111/jvp.12057
57. Lohuis, JA, van Werven, T, Brand, A, van Miert, AS, Rohde, E, Ludwig, B, et al. Pharmacodynamics and pharmacokinetics of carprofen, a non-steroidal anti-inflammatory drug, in healthy cows and cows with Escherichia coli endotoxin-induced mastitis. J Vet Pharmacol Ther. (1991) 14:219–29. doi: 10.1111/j.1365-2885.1991.tb00830.x
58. Vangroenweghe, F, Duchateau, L, Boutet, P, Lekeux, P, Rainard, P, Paape, MJ, et al. Effect of carprofen treatment following experimentally induced Escherichia coli mastitis in primiparous cows. J Dairy Sci. (2005) 88:2361–76. doi: 10.3168/jds.S0022-0302(05)72914-3
59. Muzammil, I, Ijaz, M, Saleem, MH, and Ali, MM. Drug repurposing strategy: an emerging approach to identify potential therapeutics for treatment of bovine mastitis. Microb Pathog. (2022) 171:105691. doi: 10.1016/j.micpath.2022.105691
60. Wilm, J, Svennesen, L, Østergaard Eriksen, E, Halasa, T, and Krömker, V. Veterinary treatment approach and antibiotic usage for clinical mastitis in Danish dairy herds. Antibiotics (Basel). (2021) 10:189. doi: 10.3390/antibiotics10020189
61. Lopes, TS, Fontoura, PS, Oliveira, A, Rizzo, FA, Silveira, S, and Streck, AF. Use of plant extracts and essential oils in the control of bovine mastitis. Res Vet Sci. (2020) 131:186–93. doi: 10.1016/j.rvsc.2020.04.025
62. Poeloengan, M. The effect of red ginger (zingiber officinale roscoe) extract on the growth of mastitis causing bacterial isolates. Afr J Microbiol Res. (2011) 5:382–8. doi: 10.5897/AJMR10.776
63. Shang, F, Li, L, Yu, L, Ni, J, Chen, X, and Xue, T. Effects of stigmata maydis on the methicillin resistant Staphylococus aureus biofilm formation. Peer J. (2019) 7:e6461. doi: 10.7717/peerj.6461
64. Dal Pozzo, M, Santurio, DF, Rossatto, L, Vargas, AC, Alves, SH, Loreto, ES, et al. Activity of essential oils from spices against Staphylococcus spp. isolated from bovine mastitis. Arq Bras Med Vet Zootec. (2011) 63:1229–32. doi: 10.1590/S0102-09352011000500026
65. Kher, MN, Sheth, NR, and Bhatt, VD. In vitro antibacterial evaluation of Terminalia chebula as an alternative of antibiotics against bovine subclinical mastitis. Anim Biotechnol. (2019) 30:151–8. doi: 10.1080/10495398.2018.1451752
66. Li, XH, He, XR, Zhou, YY, Zhao, HY, Zheng, WX, Jiang, ST, et al. Taraxacum mongolicum extract induced endoplasmic reticulum stress associated-apoptosis in triple-negative breast cancer cells. J Ethnopharmacol. (2017) 206:55–64. doi: 10.1016/j.jep.2017.04.025
67. Ge, BJ, Zhao, P, Li, HT, Sang, R, Wang, M, Zhou, HY, et al. Taraxacum mongolicum protects against Staphylococcus aureus-infected mastitis by exerting anti-inflammatory role via TLR2-NF-κB/MAPKs pathways in mice. J Ethnopharmacol. (2021) 268:113595. doi: 10.1016/j.jep.2020.113595
68. Chen, Y, Yang, J, Huang, Z, Yin, B, Umar, T, Yang, C, et al. Vitexin mitigates Staphylococcus aureus-induced mastitis via regulation of ROS/ER stress/NF-κB/MAPK pathway. Oxidative Med Cell Longev. (2022) 2022:7977433. doi: 10.1155/2022/7977433
69. He, X, Wei, Z, Zhou, E, Chen, L, Kou, J, Wang, J, et al. Baicalein attenuates inflammatory responses by suppressing TLR4 mediated NF-κB and MAPK signaling pathways in LPS-induced mastitis in mice. Int Immunopharmacol. (2015) 28:470–6. doi: 10.1016/j.intimp.2015.07.012
70. Yang, W, Li, H, Cong, X, Wang, X, Jiang, Z, Zhang, Q, et al. Baicalin attenuates lipopolysaccharide induced inflammation and apoptosis of cow mammary epithelial cells by regulating NF-κB and HSP72. Int Immunopharmacol. (2016) 40:139–45. doi: 10.1016/j.intimp.2016.08.032
71. Qu, S, Wang, W, Li, D, Li, S, Zhang, L, Fu, Y, et al. Mangiferin inhibits mastitis induced by LPS via suppressing NF-ĸB and NLRP3 signaling pathways. Int Immunopharmacol. (2017) 43:85–90. doi: 10.1016/j.intimp.2016.11.036
72. Jiang, KF, Zhao, G, Deng, GZ, Wu, HC, Yin, NN, Chen, XY, et al. Polydatin ameliorates Staphylococcus aureus-induced mastitis in mice via inhibiting TLR2-mediated activation of the p 38 MAPK/NF-κB pathway. Acta Pharmacol Sin. (2017) 38:211–22. doi: 10.1038/aps.2016.123
73. Song, X, Zhang, W, Wang, T, Jiang, H, Zhang, Z, Fu, Y, et al. Geniposide plays an anti-inflammatory role via regulating TLR4 and downstream signaling pathways in lipopolysaccharide-induced mastitis in mice. Inflammation. (2014) 37:1588–98. doi: 10.1007/s10753-014-9885-2
74. Salem, A, El-Awady, H, Tagel-Dein, M, and Eisa, D. Effect of supplementation of aromatic plants oils on immunity, udder health and milk production of friesian cows. SVR [Internet]. (2019):56. doi: 10.26873/SVR-790-2019
75. Zhao, S, Shan, C, Wu, Z, Feng, M, Song, L, Wang, Y, et al. Fermented Chinese herbal preparation: impacts on milk production, nutrient digestibility, blood biochemistry, and antioxidant capacity of late-lactation cows under heat stress. Anim Feed Sci Technol. (2022) 292:115448. doi: 10.1016/j.anifeedsci.2022.115448
76. Yin, B, Li, W, Qin, H, Yun, J, and Sun, X. The use of Chinese skullcap (Scutellaria baicalensis) and its extracts for sustainable animal production. Animals (Basel). (2021) 11:1039. doi: 10.3390/ani11041039
77. Khan, R, Islam, B, Akram, M, Shakil, S, Ahmad, A, Ali, SM, et al. Antimicrobial activity of five herbal extracts against multi drug resistant (MDR) strains of bacteria and fungus of clinical origin. Molecules. (2009) 14:586–97. doi: 10.3390/molecules14020586
78. Singh, BR, Singh, V, Singh, RK, et al. Antimicrobial activity of lemongrass (Cymbopogon citratus) oil against microbes of environmental, clinical and food origin. Int Res J Pharm Pharmacol. (2011) 1:228–36.
79. Vadhana, P, Singh, BR, Bharadwaj, M, et al. Emergence of herbal antimicrobial drug resistance in clinical bacterial isolates. Pharm Anal Acta. (2015) 6:434. doi: 10.4172/2153-2435.1000434
80. You, L, Liang, K, An, R, and Wang, X. The path towards FDA approval: a challenging journey for traditional Chinese medicine. Pharmacol Res. (2022) 182:106314. doi: 10.1016/j.phrs.2022.106314
81. Zhang, LJ, and Gallo, RL. Antimicrobial peptides. Curr Biol. (2016) 26:14–9. doi: 10.1016/j.cub.2015.11.017
82. Tan, P, Fu, H, and Ma, X. Design, optimization, and nanotechnology of antimicrobial peptides: from exploration to applications. Nano Today. (2021) 39:101229. doi: 10.1016/j.nantod.2021.101229
83. Sears, PM, Smith, BS, Stewart, WK, Gonzalez, RN, Rubino, SD, Gusik, SA, et al. Evaluation of a nisin-based germicidal formulation on teat skin of live cows. J Dairy Sci. (1992) 75:3185–90. doi: 10.3168/jds.S0022-0302(92)78083-7
84. Cao, LT, Wu, JQ, Xie, F, Hu, SH, and Mo, Y. Efficacy of nisin in treatment of clinical mastitis in lactating dairy cows. J Dairy Sci. (2007) 90:3980–5. doi: 10.3168/jds.2007-0153
85. Wu, J, Hu, S, and Cao, L. Therapeutic effect of nisin Z on subclinical mastitis in lactating cows. Antimicrob Agents Chemother. (2007) 51:3131–5. doi: 10.1128/AAC.00629-07
86. Simmaco, M, Mignogna, G, Barra, D, and Bossa, F. Antimicrobial peptides from skin secretions of Rana esculenta. Molecular cloning of cDNAs encoding esculentin and brevinins and isolation of new active peptides. J Biol Chem. (1994) 269:11956–61. doi: 10.1016/S0021-9258(17)32666-2
87. Islas-Rodrìguez, AE, Marcellini, L, Orioni, B, Barra, D, Stella, L, and Mangoni, ML. Esculentin 1-21: a linear antimicrobial peptide from frog skin with inhibitory effect on bovine mastitis-causing bacteria. J Pept Sci. (2009) 15:607–14. doi: 10.1002/psc.1148
88. Tetens, J, Friedrich, JJ, Hartmann, A, Schwerin, M, Kalm, E, and Thaller, G. The spatial expression pattern of antimicrobial peptides across the healthy bovine udder. J Dairy Sci. (2010) 93:775–83. doi: 10.3168/jds.2009-2729
89. Gurao, A, Kashyap, SK, and Singh, R. β-defensins: an innate defense for bovine mastitis. Vet World. (2017) 10:990–8. doi: 10.14202/vetworld.2017.990-998
90. López-Meza, JE, Gutiérrez-Barroso, A, and Ochoa-Zarzosa, A. Expression of tracheal antimicrobial peptide in bovine mammary epithelial cells. Res Vet Sci. (2009) 87:59–63. doi: 10.1016/j.rvsc.2008.12.005
91. Zhang, Z, Chen, D, Lu, X, Zhao, R, Chen, Z, Li, M, et al. Directed expression of tracheal antimicrobial peptide as a treatment for bovine-associated Staphylococcus aureus-induced mastitis in mice. Front Vet Sci. (2021) 8:700930. doi: 10.3389/fvets.2021.700930
92. Mygind, PH, Fischer, RL, Schnorr, KM, Hansen, MT, Sönksen, CP, Ludvigsen, S, et al. Plectasin is a peptide antibiotic with therapeutic potential from a saprophytic fungus. Nature. (2005) 437:975–80. doi: 10.1038/nature04051
93. Zhang, Y, Teng, D, Wang, X, Mao, R, Cao, X, Hu, X, et al. In vitro and in vivo characterization of a new recombinant antimicrobial peptide, MP1102, against methicillin-resistant Staphylococcus aureus. Appl Microbiol Biotechnol. (2015) 99:6255–66. doi: 10.1007/s00253-015-6394-7
94. Li, J, Shang, L, Lan, J, Chou, S, Feng, X, Shi, B, et al. Targeted and intracellular antibacterial activity against S. agalactiae of the chimeric peptides based on pheromone and cell-penetrating peptides. ACS Appl Mater Interfaces. (2020) 12:44459–74. doi: 10.1021/acsami.0c12226
95. Shao, C, Zhu, Y, Lai, Z, Tan, P, and Shan, A. Antimicrobial peptides with protease stability: progress and perspective. Future Med Chem. (2019) 11:2047–50. doi: 10.4155/fmc-2019-0167
96. Band, VI, and Weiss, DS. Mechanisms of antimicrobial peptide resistance in gram-negative bacteria. Antibiotics (Basel). (2015) 4:18–41. doi: 10.3390/antibiotics4010018
97. Röhrig, C, Huemer, M, Lorgé, D, Luterbacher, S, Phothaworn, P, Schefer, C, et al. Targeting hidden pathogens: cell-penetrating enzybiotics eradicate intracellular drug-resistant Staphylococcus aureus. MBio. (2020) 11:e00209–20. doi: 10.1128/mBio.00209-20
98. Kortright, KE, Chan, BK, Koff, JL, and Turner, PE. Phage therapy: a renewed approach to combat antibiotic-resistant bacteria. Cell Host Microbe. (2019) 25:219–32. doi: 10.1016/j.chom.2019.01.014
99. Schroven, K, Aertsen, A, and Lavigne, R. Bacteriophages as drivers of bacterial virulence and their potential for biotechnological exploitation. FEMS Microbiol Rev. (2021) 45:fuaa041. doi: 10.1093/femsre/fuaa041
100. Ganaie, MY, Qureshi, S, Kashoo, Z, Wani, SA, Hussain, MI, Kumar, R, et al. Isolation and characterization of two lytic bacteriophages against Staphylococcus aureus from India: newer therapeutic agents against bovine mastitis. Vet Res Commun. (2018) 42:289–95. doi: 10.1007/s11259-018-9736-y
101. Teng, F, Xiong, X, Zhang, S, Li, G, Wang, R, Zhang, L, et al. Efficacy assessment of phage therapy in treating Staphylococcus aureus-induced mastitis in mice. Viruses. (2022) 14:620. doi: 10.3390/v14030620
102. Iwano, H, Inoue, Y, Takasago, T, Kobayashi, H, Furusawa, T, Taniguchi, K, et al. Bacteriophage ΦSA012 has a broad host range against Staphylococcus aureus and effective lytic capacity in a mouse mastitis model. Biology (Basel). (2018) 7:8. doi: 10.3390/biology7010008
103. Bai, Q, Zhang, W, Yang, Y, Tang, F, Nguyen, X, Liu, G, et al. Characterization and genome sequencing of a novel bacteriophage infecting Streptococcus agalactiae with high similarity to a phage from Streptococcus pyogenes. Arch Virol. (2013) 158:1733–41. doi: 10.1007/s00705-013-1667-x
104. da Silva, DV, Dias, RS, Kropinski, AM, Campanaro, S, Treu, L, Siqueira, C, et al. Genomic analysis and immune response in a murine mastitis model of vB_EcoM-UFV13, a potential biocontrol agent for use in dairy cows. Sci Rep. (2018) 8:6845. doi: 10.1038/s41598-018-24896-w
105. Wang, Z, Xue, Y, Gao, Y, Guo, M, Liu, Y, Zou, X, et al. Phage vB_PaeS-PAJD-1 rescues murine mastitis infected with multidrug-resistant Pseudomonas aeruginosa. Front Cell Infect Microbiol. (2021) 11:689770. doi: 10.3389/fcimb.2021.689770
106. Liang, B, Zhao, W, Han, B, Barkema, HW, Niu, YD, Liu, Y, et al. Biological and genomic characteristics of two bacteriophages isolated from sewage, using one multidrug-resistant and one non-multidrug-resistant strain of Klebsiella pneumoniae. Front Microbiol. (2022) 13:943279. doi: 10.3389/fmicb.2022.943279
107. Shi, Y, Zhao, W, Liu, G, Ali, T, Chen, P, Liu, Y, et al. Bacteriophages isolated from dairy farm mitigated Klebsiella pneumoniae-induced inflammation in bovine mammary epithelial cells cultured in vitro. BMC Vet Res. (2021) 17:37. doi: 10.1186/s12917-020-02738-0
108. Zhao, W, Shi, Y, Liu, G, Yang, J, Yi, B, Liu, Y, et al. Bacteriophage has beneficial effects in a murine model of Klebsiella pneumoniae mastitis. J Dairy Sci. (2021) 104:3474–84. doi: 10.3168/jds.2020-19094
109. Monteiro, R, Pires, DP, Costa, AR, and Azeredo, J. Phage therapy: going temperate? Trends Microbiol. (2019) 27:368–78. doi: 10.1016/j.tim.2018.10.008
110. McCallin, S, and Oechslin, F. Bacterial resistance to phage and its impact on clinical therapy In: A Górski, R Międzybrodzki, and J Borysowski, editors. Phage Therapy: A Practical Approach. Cham: Springer (2019)
111. Costa, P, Pereira, C, Gomes, ATPC, and Almeida, A. Efficiency of single phage suspensions and phage cocktail in the inactivation of Escherichia coli and Salmonella Typhimurium: an in vitro preliminary study. Microorganisms. (2019) 7:94. doi: 10.3390/microorganisms7040094
112. Pires, D, Sillankorva, S, Faustino, A, and Azeredo, J. Use of newly isolated phages for control of Pseudomonas aeruginosa PAO1 and ATCC 10145 biofilms. Res Microbiol. (2011) 162:798–806. doi: 10.1016/j.resmic.2011.06.010
113. Rohde, C, Wittmann, J, and Kutter, E. Bacteriophages: a therapy concept against multi-drug-resistant bacteria. Surg Infect. (2018) 19:737–44. doi: 10.1089/sur.2018.184
114. Pires, DP, Costa, AR, Pinto, G, Meneses, L, and Azeredo, J. Current challenges and future opportunities of phage therapy. FEMS Microbiol Rev. (2020) 44:684–700. doi: 10.1093/femsre/fuaa017
115. García, P, Madera, C, Martínez, B, Rodríguez, A, and Evaristo, SJ. Prevalence of bacteriophages infecting Staphylococcus aureus in dairy samples and their potential as biocontrol agents. J Dairy Sci. (2009) 92:3019–26. doi: 10.3168/jds.2008-1744
116. Love, MJ, Bhandari, D, Dobson, RCJ, and Billington, C. Potential for bacteriophage endolysins to supplement or replace antibiotics in food production and clinical care. Antibiotics (Basel). (2018) 7:17. doi: 10.3390/antibiotics7010017
117. Vander Elst, N, Linden, SB, Lavigne, R, Meyer, E, Briers, Y, and Nelson, DC. Characterization of the bacteriophage-derived endolysins PlySs2 and PlySs9 with in vitro lytic activity against bovine mastitis Streptococcus uberis. Antibiotics (Basel). (2020) 9:621. doi: 10.3390/antibiotics9090621
118. Gutiérrez, D, Garrido, V, Fernández, L, Portilla, S, Rodríguez, A, Grilló, MJ, et al. Phage lytic protein Lys RODI prevents staphylococcal mastitis in mice. Front Microbiol. (2020) 11:7. doi: 10.3389/fmicb.2020.00007
119. Bassetti, M, Poulakou, G, Ruppe, E, Bouza, E, Van Hal, SJ, and Brink, A. Antimicrobial resistance in the next 30 years, humankind, bugs and drugs: a visionary approach. Intensive Care Med. (2017) 43:1464–75. doi: 10.1007/s00134-017-4878-x
120. Dąbrowska, K, and Abedon, ST. Pharmacologically aware phage therapy: pharmacodynamic and pharmacokinetic obstacles to phage antibacterial action in animal and human bodies. Microbiol Mol Biol Rev. (2019) 83:e00012–9. doi: 10.1128/MMBR.00012-19
121. Furfaro, LL, Payne, MS, and Chang, BJ. Bacteriophage therapy: clinical trials and regulatory hurdles. Front Cell Infect Microbiol. (2018) 8:376. doi: 10.3389/fcimb.2018.00376
122. Chan, BK, Abedon, ST, and Loc-Carrillo, C. Phage cocktails and the future of phage therapy. Future Microbiol. (2013) 8:769–83. doi: 10.2217/fmb.13.47
123. Pirnay, JP, Verbeken, G, Ceyssens, PJ, Huys, I, De Vos, D, Ameloot, C, et al. The Magistral phage. Viruses. (2018) 10:64. doi: 10.3390/v10020064
124. Rainard, P, Gilbert, FB, Germon, P, and Foucras, G. Invited review: a critical appraisal of mastitis vaccines for dairy cows. J Dairy Sci. (2021) 104:10427–48. doi: 10.3168/jds.2021-20434
125. González, RN, Cullor, JS, Jasper, DE, Farver, TB, Bushnell, RB, and Oliver, MN. Prevention of clinical coliform mastitis in dairy cows by a mutant Escherichia coli vaccine. Can J Vet Res. (1989) 53:301–5.
126. Wilson, DJ, Grohn, YT, Bennett, GJ, González, RN, Schukken, YH, and Spatz, J. Comparison of J5 vaccinates and controls for incidence, etiologic agent, clinical severity, and survival in the herd following naturally occurring cases of clinical mastitis. J Dairy Sci. (2007) 90:4282–8. doi: 10.3168/jds.2007-0160
127. Côté-Gravel, J, Brouillette, E, Obradović, N, Ster, C, Talbot, BG, and Malouin, F. Characterization of a vraG mutant in a genetically stable Staphylococcus aureus small-colony variant and preliminary assessment for use as a live-attenuated vaccine against intrammamary infections. PLoS One. (2016) 11:e0166621. doi: 10.1371/journal.pone.0166621
128. Vidlund, Jessica J. Staphylococcal surface proteins as vaccine candidates for the control of Staphylococcal mastitis in dairy cows. Master’s thesis, university of tennessee, (2022). Available at: https://trace.tennessee.edu/utk_gradthes/7061
129. Ster, C, Beaudoin, F, Diarra, MS, Jacques, M, Malouin, F, and Lacasse, P. Evaluation of some Staphylococcus aureus iron-regulated proteins as vaccine targets. Vet Immunol Immunopathol. (2010) 15:311–8. doi: 10.1016/j.vetimm.2010.03.010
130. Kabelitz, T, Aubry, E, van Vorst, K, Amon, T, and Fulde, M. The role of Streptococcus spp. in bovine mastitis. Microorganisms. (2021) 9:1497. doi: 10.3390/microorganisms9071497
131. Klaas, IC, and Zadoks, RN. An update on environmental mastitis: challenging perceptions. Transbound Emerg Dis. (2018) 65:166–85. doi: 10.1111/tbed.12704
132. Collado, R, Montbrau, C, Sitjà, M, and Prenafeta, A. Study of the efficacy of a Streptococcus uberis mastitis vaccine against an experimental intramammary infection with a heterologous strain in dairy cows. J Dairy Sci. (2018) 101:10290–302. doi: 10.3168/jds.2018-14840
133. Finch, JM, Winter, A, Walton, AW, and Leigh, JA. Further studies on the efficacy of a live vaccine against mastitis caused by Streptococcus uberis. Vaccine. (1997) 15:1138–43. doi: 10.1016/s0264-410x(96)00307-6
134. Luo, S, Wang, Y, Kang, X, Liu, P, and Wang, G. Research progress on the association between mastitis and gastrointestinal microbes in dairy cows and the effect of probiotics. Microb Pathog. (2022) 173:105809. doi: 10.1016/j.micpath.2022.105809
135. Urakawa, M, Zhuang, T, Sato, H, Takanashi, S, Yoshimura, K, Endo, Y, et al. Prevention of mastitis in multiparous dairy cows with a previous history of mastitis by oral feeding with probiotic Bacillus subtilis. Anim Sci J. (2022) 93:e13764. doi: 10.1111/asj.13764
136. Gao, J, Liu, YC, Wang, Y, Li, H, Wang, XM, Wu, Y, et al. Impact of yeast and lactic acid bacteria on mastitis and milk microbiota composition of dairy cows. AMB Express. (2020) 10:22. doi: 10.1186/s13568-020-0953-8
137. Bouchard, DS, Rault, L, Berkova, N, Le Loir, Y, and Even, S. Inhibition of Staphylococcus aureus invasion into bovine mammary epithelial cells by contact with live Lactobacillus casei. Appl Environ Microbiol. (2013) 79:877–85. doi: 10.1128/AEM.03323-12
138. Souza, RFS, Rault, L, Seyffert, N, Azevedo, V, Le Loir, Y, and Even, S. Lactobacillus casei BL23 modulates the innate immune response in Staphylococcus aureus-stimulated bovine mammary epithelial cells. Benef Microbes. (2018) 9:985–95. doi: 10.3920/BM2018.0010
139. Alawneh, JI, James, AS, Phillips, N, Fraser, B, Jury, K, Soust, M, et al. Efficacy of a Lactobacillus-based teat spray on udder health in lactating dairy cows. Front Vet Sci. (2020) 7:584436. doi: 10.3389/fvets.2020.584436
140. Yousefi Dehbidi, M, Goodarzi, N, Azhdari, MH, and Doroudian, M. Mesenchymal stem cells and their derived exosomes to combat Covid-19. Rev Med Virol. (2022) 32:e2281. doi: 10.1002/rmv.2281
141. Cahuascanco, B, Bahamonde, J, Huaman, O, Jervis, M, Cortez, J, Palomino, J, et al. Bovine fetal mesenchymal stem cells exert antiproliferative effect against mastitis causing pathogen Staphylococcus aureus. Vet Res. (2019) 50:25. doi: 10.1186/s13567-019-0643-1
142. Peralta, OA, Carrasco, C, Vieytes, C, Tamayo, MJ, Muñoz, I, Sepulveda, S, et al. Safety and efficacy of a mesenchymal stem cell intramammary therapy in dairy cows with experimentally induced Staphylococcus aureus clinical mastitis. Sci Rep. (2020) 10:2843. doi: 10.1038/s41598-020-59724-7
143. Ghai, S, Saini, S, Ansari, S, Verma, V, Chopra, S, Sharma, V, et al. Allogenic umbilical cord blood-mesenchymal stem cells are more effective than antibiotics in alleviating subclinical mastitis in dairy cows. Theriogenology. (2022) 187:141–51. doi: 10.1016/j.theriogenology.2022.05.001
144. Ferreira, M, Ogren, M, Dias, JNR, Silva, M, Gil, S, Tavares, L, et al. Liposomes as antibiotic delivery dystems: a promising nanotechnological strategy against antimicrobial resistance. Molecules. (2021) 26:2047. doi: 10.3390/molecules26072047
145. Zhou, K, Wang, X, Chen, D, Yuan, Y, Wang, S, Li, C, et al. Enhanced treatment effects of tilmicosin against Staphylococcus aureus cow mastitis by self-assembly sodium alginate-chitosan nanogel. Pharmaceutics. (2019) 11:524. doi: 10.3390/pharmaceutics11100524
146. Abd El-Aziz, NK, Ammar, AM, El-Naenaeey, EYM, El Damaty, HM, Elazazy, AA, Hefny, AA, et al. Antimicrobial and antibiofilm potentials of cinnamon oil and silver nanoparticles against Streptococcus agalactiae isolated from bovine mastitis: new avenues for countering resistance. BMC Vet Res. (2021) 17:136. doi: 10.1186/s12917-021-02842-9
147. Ranjani, S, Priya, PS, Veerasami, M, and Hemalatha, S. Novel polyherbal nanocolloids to control bovine mastitis. Appl Biochem Biotechnol. (2022) 194:246–65. doi: 10.1007/s12010-021-03748-w
148. Sperandio, FF, Huang, YY, and Hamblin, MR. Antimicrobial photodynamic therapy to kill gram-negative bacteria. Recent Pat Antiinfect Drug Discov. (2013) 8:108–20. doi: 10.2174/1574891x113089990012
149. Hamblin, MR, and Hasan, T. Photodynamic therapy: a new antimicrobial approach to infectious disease? Photochem Photobiol Sci. (2004) 3:436–50. doi: 10.1039/b311900a
150. Moreira, LH, de Souza, JCP, de Lima, CJ, Salgado, MAC, Fernandes, AB, Andreani, DIK, et al. Use of photodynamic therapy in the treatment of bovine subclinical mastitis. Photodiagn Photodyn Ther. (2018) 21:246–51. doi: 10.1016/j.pdpdt.2017.12.009
151. Silva, LO, da Silva Souza, KL, de Jesus, BL, Neto, WMR, Núñez, SC, and Frias, DFR. Use of photodynamic therapy and photobiomodulation as alternatives for microbial control on clinical and subclinical mastitis in sheep. Lasers Med Sci. (2022) 37:2305–10. doi: 10.1007/s10103-022-03506-2
152. Yang, D, Lei, S, Pan, K, Chen, T, Lin, J, Ni, G, et al. Application of photodynamic therapy in immune-related diseases. Photodiagn Photodyn Ther. (2021) 34:102318. doi: 10.1016/j.pdpdt.2021.102318
153. Leitner, G, Zilberman, D, Papirov, E, and Shefy, S. Assessment of acoustic pulse therapy (APT), a non-antibiotic treatment for dairy cows with clinical and subclinical mastitis. PLoS One. (2018) 13:e0199195. doi: 10.1371/journal.pone.0199195
Keywords: dairy cows, bovine mastitis, NSAIDs, herbal medicines, antimicrobial peptides, bacteriophages, vaccination
Citation: Li X, Xu C, Liang B, Kastelic JP, Han B, Tong X and Gao J (2023) Alternatives to antibiotics for treatment of mastitis in dairy cows. Front. Vet. Sci. 10:1160350. doi: 10.3389/fvets.2023.1160350
Received: 07 February 2023; Accepted: 26 May 2023;
Published: 19 June 2023.
Edited by:
Cristina Carresi, University Magna Graecia of Catanzaro, ItalyReviewed by:
Mahmoud Mohamed Fayez, Veterinary Serum and Vaccine Research Institute, EgyptCopyright © 2023 Li, Xu, Liang, Kastelic, Han, Tong and Gao. This is an open-access article distributed under the terms of the Creative Commons Attribution License (CC BY). The use, distribution or reproduction in other forums is permitted, provided the original author(s) and the copyright owner(s) are credited and that the original publication in this journal is cited, in accordance with accepted academic practice. No use, distribution or reproduction is permitted which does not comply with these terms.
*Correspondence: Jian Gao, Z2FvamlhbjIwMTZAY2F1LmVkdS5jbg==
Disclaimer: All claims expressed in this article are solely those of the authors and do not necessarily represent those of their affiliated organizations, or those of the publisher, the editors and the reviewers. Any product that may be evaluated in this article or claim that may be made by its manufacturer is not guaranteed or endorsed by the publisher.
Research integrity at Frontiers
Learn more about the work of our research integrity team to safeguard the quality of each article we publish.