- Baker Institute for Animal Health, College of Veterinary Medicine, Cornell University, Ithaca, NY, United States
Ruminant livestock, including cattle, sheep, goat, and buffalo, are essential for global food security and serve valuable roles in sustainable agricultural systems. With the limited availability of embryonic stem cells (ESCs) from these species, ruminant induced pluripotent stem cells (iPSCs) and iPSC-like cells provide a valuable research tool for agricultural, veterinary, biomedical, and pharmaceutical applications, as well as for the prospect of translation to human medicine. iPSCs are generated by reprogramming of adult or fetal cells to an ESC-like state by ectopic expression of defined transcription factors. Despite the slow pace the field has evolved in livestock species compared to mice and humans, significant progress has been made over the past 15 years in using different cell sources and reprogramming protocols to generate iPSCs/iPSC-like cells from ruminants. This mini review summarizes the current literature related to the derivation of iPSCs/iPSC-like cells from domesticated ruminants with a focus on reprogramming protocols, characterization, associated limitations, and potential applications in ruminant basic science research and production.
Introduction
The world population is projected to reach 9.8 billion by 2050 and 11.2 billion by 2100 (1). As a result, the demand for livestock commodities to support global food security is expected to double by 2050 (2). In both industrialized and developing agricultural systems, current livestock production practices are insufficient to fulfill projected world needs. To address this issue, the genetics of animal development, conformation, and disease resistance are being studied with the goal of improving the efficiency of animal food production. In addition, knowledge of livestock genetics has the potential to refine veterinary practices and contribute to biomedical and pharmaceutical applications that may be translatable to human medicine.
Embryonic stem cells (ESCs) are pluripotent cells typically derived from the inner cell mass of blastocyst-stage embryos (3). Ruminant ESCs can (i) provide material for genomic testing, (ii) be used to select desirable genetic traits, and (iii) be engineered to improve desirable genetic traits, each of which has the potential to expand our current knowledge of livestock genetics. Ruminant ESCs, however, are difficult to obtain and have proven hard to maintain in culture for research purposes.
Induced pluripotent stem cells (iPSCs) are pluripotent cells created by reprogramming differentiated cells. The creation of iPSCs from mouse (4), and shortly thereafter from humans (5, 6), opened new avenues for basic science research while also significantly improving the feasibility of producing and analyzing stem cells from other mammalian species. iPSCs have been produced from a wide range of eutherian mammals, including several types of domesticated ruminant species such as cattle, sheep, goats, and buffalo. With the limited availability of bona fide ESCs from these ruminants, iPSCs, which closely resemble ESCs, provide a practically limitless source of pluripotent stem cells.
The production of iPSCs from domesticated ruminants has the potential to benefit both agriculture and biotechnology. Here, we describe the methods utilized to create, characterize, and maintain, ruminant pluripotent cell lines. This mini review refers to these cell lines as iPSCs regardless of the extent to which they have been characterized (Table 1), and thus, represent authentic iPSCs. Moreover, we discuss the limitations of these cells and explore possibilities for enhancing livestock production.
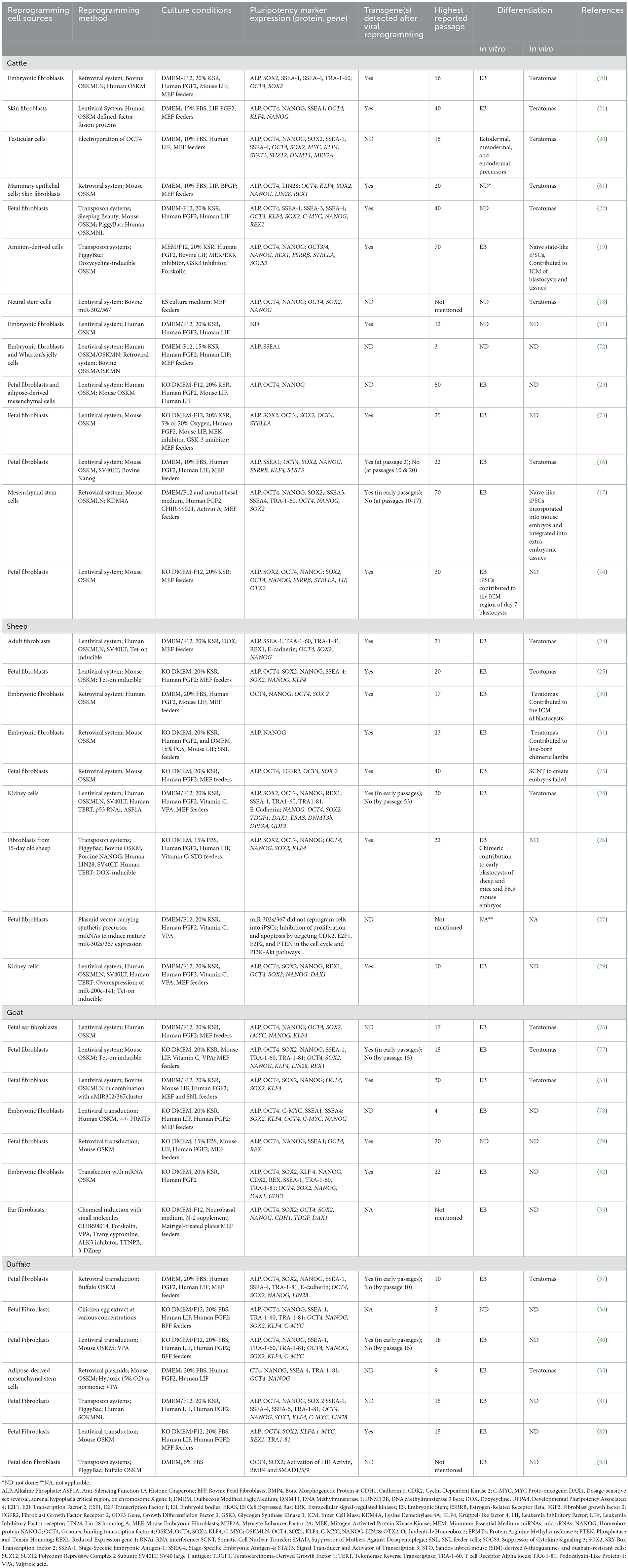
Table 1. Summary of induced pluripotent stem cells (iPSCs) generated from cattle, sheep, goat, and buffalo.
Limited availability of embryonic stem cells from domesticated ruminants
The derivation and maintenance of stable ESCs from domesticated ruminants is challenging and complicated. Over the years, there have been numerous and contradictory reports of the successful generation of ESCs from domesticated ruminants (7). However, stable, well-characterized ruminant ESCs are extremely limited in supply. The poor success rates in developing and maintaining ESCs from ruminants compared to mice or humans can be attributed to the differences in these animals' developmental processes and the need for specific culture conditions. Fundamental biological differences between species, the time point differences utilized to isolate ESCs, differences in the genes and molecular pathways that govern the pluripotency network, and poorly defined pluripotency states (naïve vs. prime) in ruminant species, may necessitate protocols designed specifically for handling ESCs from each species. In addition, the long-term culture of ruminant ESCs while maintaining full pluripotency is challenging and requires further refinements.
Nevertheless, efforts have been made to establish (putative) ruminant ESCs by applying standard or modified culture systems developed for murine and human ESCs. For instance, a culture system containing fibroblast growth factor 2 (FGF2) and an inhibitor of the canonical Wnt-signaling pathway, which was successfully used to create human ESCs, was employed to derive pluripotent cell lines from cow blastocysts with stable morphology, karyotype, pluripotency marker expression and epigenetic features (8). Likewise, ovine ESCs have been derived using similar conditions (9). The generation of caprine ESCs (10), caprine ESC-like cells (11), buffalo ESCs (12, 13) and buffalo ESC-like cells (14), has been reported as well. However, most of these cells did not maintain robust self-renewal capacity and did not develop into bona fide ESC lines capable of undergoing germline transmission.
Generation and characterization of induced pluripotent stem cells from domesticated ruminants
Since the first reports were published in 2011, numerous studies describe the production of ruminant iPSCs and iPSC-like cells using a variety of cell sources, reprogramming systems, reprogramming factor combinations, and culture conditions. Moreover, these cultures have been characterized in vitro and/or in vivo to various degrees, and are referred to as iPSCs in this review, regardless of the extent to which they have been characterized and, thus, to what extent they represent authentic iPSCs. Table 1 illustrates variations and similarities in the generation and characterization of domesticated ruminant iPSCs across studies.
General criteria to characterize induced pluripotent stem cells
Measuring pluripotency is a fundamental component of every stem cell-based study. Assays testing pluripotency in vitro include relative quantification of the expression of pluripotency genes at the mRNA level and immunocytochemistry to detect specific pluripotency markers at the protein level. Moreover, embryoid body (EB) formation assays to test the ability of the cells to form three embryonic germ-layers can be conducted. Teratoma formation in immunodeficient mice is widely used as an index of pluripotency, as it assesses the capability of the cells to differentiate into the three embryonic germ layers in vivo, and it provides a reliable and comprehensive validation of the functional pluripotency of the cells (15).
Descriptions of the assays used to characterize each ruminant cell line discussed are detailed in Table 1.
Induced pluripotent stem cells from cattle
Several groups have reprogrammed bovine cells from various developmental stages and tissues, including non-conventional cell sources such as amnion-derived cells, Wharton's jelly cells, and multipotent stem cells such as neural stem cells and mesenchymal stromal cells (MSCs). These groups primarily relied on bovine, human, or murine reprogramming factors consisting of POU class 5 homeobox1 (OCT4), SRY-box transcription factor 2 (SOX)2, KLF transcription factor 4 (KLF4) and MYC proto-oncogene (c-MYC) (OSKM) or OSKM plus Lin-28 homolog A (LIN28) and Nanog homeobox (NANOG) (OSKMNL) (Table 1). Variations such as overexpression of Lysine demethylase 4A (KDM4A) or forced expression of SV40 large T antigen, together with reprogramming factors (16, 17) or antigen reprogramming with micro RNAs (18), have also been employed. Generally, reprogramming elements were delivered via viral vectors, but the use of transposon systems encoding reprogramming factors (19) and electroporation of plasmid DNA containing a single reprogramming gene have been explored as well (20). The majority of bovine iPSCs were maintained in a dual-factor culture medium consisting of both FGF2 and leukemia inhibitory factor (LIF) for proliferation in an undifferentiated state.
Characterization of these bovine iPSCs demonstrated the expression of endogenous pluripotency factors such as ZFP42 Zinc Finger Protein (REX1), Estrogen related receptor beta (ESRRB) and Developmental pluripotency associated 3 (STELA) at the transcript level, and ALP, Fucosyltransferase 4 (SSEA-1) and LIN28 at the protein level. Two studies demonstrated bovine iPSC longevity by maintaining cultures for more than 40 passages (21, 22). Both groups supplemented the culture medium with FGF2 and LIF, and one added kinase inhibitors (22). Another group reported the maintenance of bovine iPSCs for over 50 passages using FGF2 and LIF supplemented culture medium (23). In addition, bovine iPSCs cultured for over 70 passages was achieved with a culture medium containing doxycycline, histone methyltransferase, and WNT inhibitors (17, 19). Additionally, few groups demonstrated epigenetic validation of iPSCs by showing demethylation of NANOG and OCT4 promoter regions in the host cells' genomes (17, 18, 21). Finally, many cultures were not subjected to experimentation to demonstrate differentiation into EBs in vitro and/or formation of teratomas in vivo, measures of functional pluripotency (Table 1). While most studies did not focus on the pluripotency state, two research groups reported the generation of bovine naïve-like iPSCs (16, 19), and both naïve state-like and primed state-like status of these cells was achieved using culture conditions for mouse and human iPSCs (17, 19).
Induced pluripotent stem cells from sheep
Two independent groups published the first reports of ovine iPSCs in 2011 (24, 25). They used viral vectors to introduce OSKM or OSKM plus additional pluripotency genes into fibroblasts collected at different stages of development and showed that the generated iPSCs expressed multiple pluripotency markers and formed EB and teratomas. Since these two initial reports, additional groups have introduced pluripotency genes into ovine cells using a PiggyBac transposon system (26) and microRNAs (27). In addition to ovine fibroblasts, kidney cells have also been used for reprogramming (28, 29). A variety of culture media has been used across ovine iPSC studies, and different pluripotency markers have been assessed (Table 1). Most ovine iPSC cultures could form EB, some formed teratomas, and other cultures were found to contribute to early blastocysts (26, 30) and live-born chimeric lambs (31).
Induced pluripotent stem cells from goats
All caprine iPSCs produced thus far were derived from fetal, embryonic, or adult fibroblasts. In most of the cases, lentiviral or retroviral vectors containing OSKM or OSKM plus additional pluripotency factors were utilized (Table 1). Caprine iPSCs have also been produced via mRNA transduction (32) and chemical induction using small molecules (33). Many different culture conditions have been used, which either included LIF or FGF2, or both. Most of the caprine iPSC cultures displayed a colony morphology like mouse iPSCs, stained positively for alkaline phosphatase (ALP), and exhibited goat-specific pluripotency markers at the transcriptional and/or protein level. Although most caprine iPSC cultures could develop into EBs, very few were able to induce teratomas in vivo. One study showed that directed differentiation of caprine iPSCs resulted in the in vitro production of trophoblast-like cells, yolk-sac endoderm-like cells and neuronal cells (34).
Induced pluripotent stem cells from buffalo
Almost all buffalo iPSC cultures have been generated using fetal fibroblasts as the reprogramming cell source, except for one study which used adipose-derived MSCs (Table 1). In addition to viral or non-viral delivery of buffalo, mouse, or human reprogramming factors (OSKM or OSKMNL), the epigenetic modifier valproic acid has been used to enhance the reprogramming efficiency (35). Chicken egg extract added to the culture medium was also shown to be adequate to generate putative buffalo iPSCs colonies (36). Although most of these buffalo iPSC cultures were able to differentiate into EBs, only one group reported generation of in vivo teratomas and epigenetically validated buffalo iPSCs (35, 37).
Limitations of ruminant induced pluripotent stem cells
Technical barriers to iPSC generation and maintenance, safety concerns when using iPSC in vivo, and the cost of creating and sustaining iPSC lines for therapeutic use, all contribute to the slow rate of progress in the field of iPSC research across species.
Technically, the core genes required for the establishment of pluripotency are different between mammals and are expressed at different stages of development (38). As a result, pluripotency factors other than OSKMNL need to be tested and timing of the introduction of pluripotency genes must be optimized, to determine the best methods for establishing pluripotent cell lines from ruminants. When pluripotent iPSC lines are created successfully, permanent expression of viral transgenes can interfere with differentiation into desired cell types (39). Non-viral methods of introducing pluripotency factors to target cells might circumvent this issue, but such methods have not been well-explored in ruminants to date.
For the in vivo use of iPSCs, safety concerns are at the forefront, primarily the risk of (i) harmful immune reactions to allogeneic cells, (ii) random integration of transduction material into the recipient's genome and (iii) differentiation of iPSCs leading to tumorigenesis. Immune reactions may be avoided by using iPSCs derived from autologous cells or altering MHC genes in iPSCs to make them less immunogenic (40). However, each of these strategies has its drawbacks. Autologous cells are not practical for large-scale, commercial treatments, and administering foreign cells that can completely avoid the host immune response introduces a risk of unchecked, inappropriate cell growth. Non-viral methods to induce pluripotency would avert the random integration of transgenes, but as mentioned above, these methods are not yet well-developed. The risk of tumor development could be reduced by differentiating iPSCs in vitro before administering them as a treatment (41) or by introducing a drug-inducible “suicide” gene, that can be turned on to prevent tumor growth (42). Such methods, however, are not fully optimized and currently not used in vivo.
The cost of generating and maintaining iPSCs for research is not trivial. The expenses required to create marketable iPSC-derived meat and other animal products in controlled laboratory environments (43), as well as those involved in commercializing iPSC-based therapies (44), are tremendous. Governments and private companies must be assured that ruminant iPSCs are a useful resource worth investing in if progress is to be made in the fields of food production, and veterinary, biomedical, and/or pharmaceutical applications.
Potential applications of ruminant induced pluripotent stem cells for research and enhancing livestock production
iPSCs derived from ruminant somatic cells have the potential to (i) improve agriculture, (ii) enhance veterinary, biomedical, and pharmaceutical practices, and (iii) provide knowledge that may be translatable to human medicine (Figure 1).
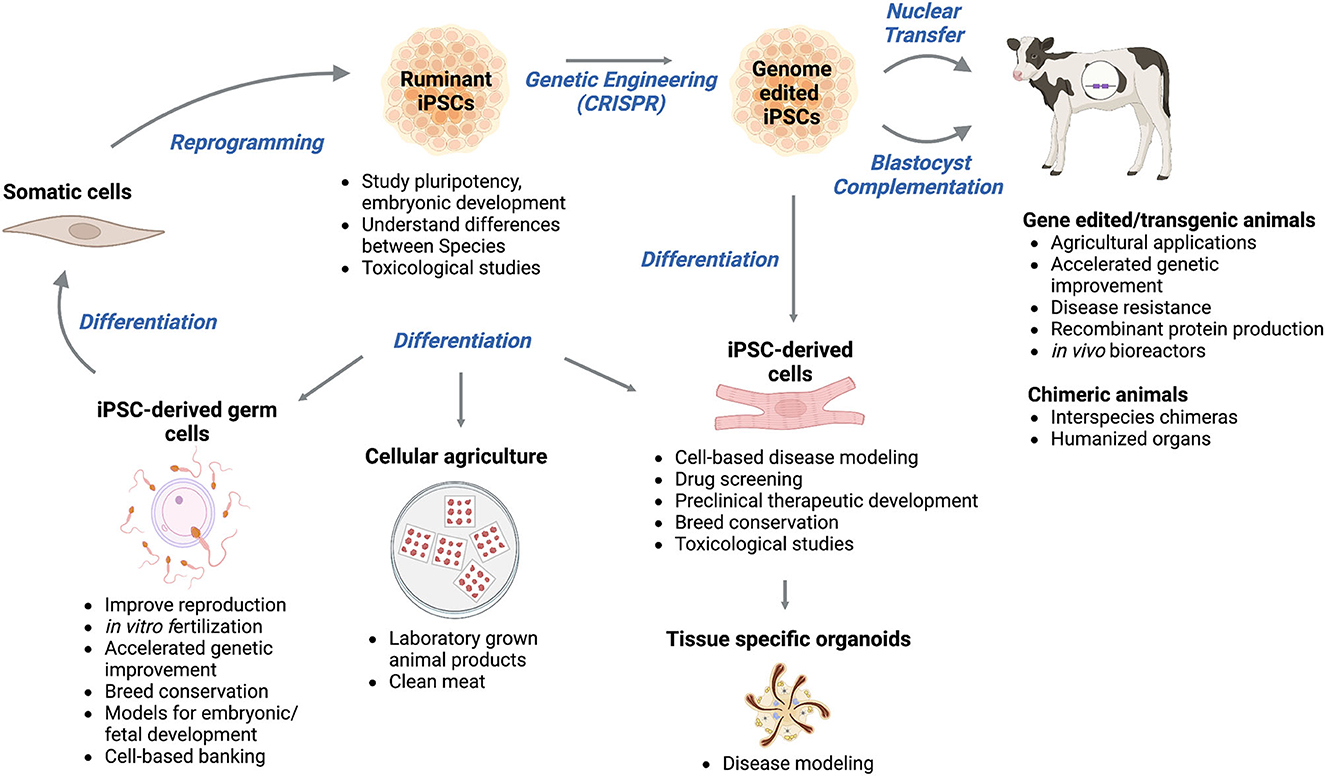
Figure 1. Applications and future usages of induced pluripotent stem cells (iPSCs) from ruminant livestock. Ruminant iPSCs are generated by the reprogramming of somatic cells. Ruminant iPSCs are a useful tool for studies of pluripotency, embryonic development, and understanding species differences, and can be genetically modified to create transgenic animals for various agricultural, veterinary, biomedical, and pharmaceutical applications. Ruminant iPSCs and/or gene-edited iPSCs have the potential to be used for the generation of interspecies chimeras and humanized organs. They can be the basis for disease modeling, drug screening, preclinical therapeutic development, and toxicological studies. Tissue organoids derived from ruminant iPSCs can be used to model diseases and identify effective treatment options. Ruminant iPSCs can also contribute to cellular agriculture as a source of laboratory-grown animal products. Successful generation of ruminant iPSC-derived germ cells can lead to improved reproduction, genetic improvement, breed conversation, and can be used as tools to study embryonic and/or fetal development. Figure was created using Biorender.com.
Cellular agriculture
Finding alternatives to conventional farming practices is crucial, given the rising reliance on animal products for human nutrition. Compared to plant-based food sources, conventionally produced animal-based material has a larger environmental footprint, requires more soil and water, and leads to the emission of more greenhouse gases (45). Moreover, antibiotic overuse in livestock farming results in the emergence of antimicrobial-resistant bacterial strains, a significant human health concern (46). Cellular agriculture, defined as the production of animal-sourced food from cultured cells, has the potential to replace traditional farming with more environmentally friendly practices. The production of meat in vitro using iPSCs is proposed as a clean and prominent alternative to reduce the global burden of the livestock industry (47). In 2012, meat derived from bovine stem cells was used to create the first lab-made hamburger (48). Moreover, a commercial meat producer in the UK reported the first lab-made strips of bacon and pork belly in 2020 (49). In addition, production of cell-based seafood from fish cells and tissue cultures is also becoming popular to address the challenges associated with industrial aquaculture systems and marine capture (50). More recently, laboratory-grown meat, slaughter-free chicken, received clearance from U.S. Food and Drug Administration for human consumption (51). Additionally, generation of other iPSC-derived animal products, such as skin and fur, could reduce our dependence on industrial farming and minimize the associated environmentally harmful effects.
Genetically modified (transgenic) ruminant livestock
The recent advancements in iPSC generation along with targeted genome editing technologies, especially the CRISPR-Cas9 system (52), have facilitated the introduction of desired genetic modifications and, combined with somatic cell nuclear transfer (SCNT) or blastocyst complementation, represent a powerful platform for transgenic animal production (53, 54).
Genetically modifying ruminants can enhance growth rates and production, improve nutrients in animal products, increase disease resistance, and enhance reproductive efficiency and fecundity. Moreover, transgenic ruminant livestock, especially those animals used for milk production such as cattle, buffalo, and goats, that are generated by genetically modified iPSCs could be used as bioreactors to produce therapeutic proteins of pharmaceutical interest. To this end, cloned transgenic cattle, which produce recombinant proteins in milk such as human coagulation factor IX has been reported (55). Additionally, transgenic ruminant livestock has the potential to significantly reduce the environmental footprint of livestock husbandry by increasing productivity and efficiency through transgenesis, which results in reduced use of land and water resources while safeguarding the soil and groundwater.
Reproduction and conservation
Significant paradigm changes in reproduction have been made possible by prominent developments in stem cell biology. Germ cells have been derived successfully from mouse stem cells (56) and although protocols for differentiating buffalo embryonic stem cells into germ cells (57) and animal embryo-stem cell livestock laboratory breeding systems have been proposed (58), the differentiation of ruminant iPSCs to functional gametes in vitro has not been achieved yet.
Derivation of ruminant iPSCs may open the possibility of in vitro breeding. For example, selected cell lines could be differentiated to create functional gametes, which would then be used to create a new generation of embryos through in vitro fertilization. Such breeding schemes could substantially reduce generation intervals, enhance selection intensity, achieve more genetic gain, and preserve rare ruminant breeds and highly valuable genotypes. In addition, these cells could be expanded for the banking of genetic material and be used as donor cells for SCNT.
Disease modeling
Ruminant diseases are widespread and have detrimental consequences on the herd and consumer health (59). The lack of appropriate ruminant disease models hampers the study of disease pathogenesis and the development of strategies to control these diseases. iPSCs are valuable tools for tissue and disease modeling, as well as preclinical therapeutic development in both human and mouse models (60). However, the use of iPSCs for ruminant disease modeling is currently limited, in part because differentiating ruminant iPSCs into clinically relevant lineages has not been well explored. One study demonstrated the potential of using iPSC technology for generating bovine mammary tissue in vitro (61). In this study, bovine iPSCs were successfully generated from the bovine mammary epithelium, and mammary epithelium-derived-iPSCs were differentiated back to a mammary phenotype characterized by epithelial cells expressing cytokeratin 14, cytokeratin 18, and smooth muscle actin, after treatment with progesterone (61). These studies could be complemented by generating iPSC-derived mammary organoids that can be used to explore the pathogenesis and prevention of important bovine udder diseases. Additionally, rare genetic disorders such as bovine citrullinemia and bovine leukocyte adhesion deficiency found in Holstein-Friesian cattle (62), and Chediak-Higashi syndrome found in Hereford, Brangus, and Japanese black cattle (63), may be studied using bovine iPSCs models, based on the unique advantages that iPSC cultures have shown in the modeling of rare human genetic disorders (64).
Toxicology studies
Endocrine-disrupting chemicals (EDCs) may significantly impact the reproductive functioning of ruminant livestock, which greatly impacts agricultural production (65). Bovine iPSCs have been used to study the effects of phthalate esters, synthetic organic chemicals used in the plastic industry (20). These esters were found to significantly downregulate androgen receptors on iPSCs, which supported apoptosis (20). Ruminant iPSCs may also be used in toxicological studies investigating how pharmaceuticals, potential toxins, teratogens, and EDCs affect livestock species and humans.
Chimera formation and growth of human organs
A composite organism of at least two genetically distinct cell populations is called a chimera. With the use of iPSC technology, the production of chimeric ruminants would allow for the genetic engineering of farm animals to improve traits of agricultural importance and the generation of biomedical models. Reports on interspecies ruminant chimeras such as sheep-goat (66) and cattle-buffalo (67) are already available. Moreover, human-ruminant chimeras could be created for use as models to study human organ development and disease pathogenesis, as well as to meet the increasing demand for and reduce the shortage of human organs. For example, human iPSCs have been engrafted in cattle pre-implantation blastocysts (68). However, enormous technical challenges and complex ethical issues must be considered and overcome before producing human organs in ruminants or any other mammals becomes feasible. The risks of human consciousness, human traits, and the creation of human gametes by such chimeras are the primary ethical concerns (69), and any attempt to create human-ruminant chimeras must be thoroughly risk-assessed, technically evaluated, and closely supervised.
Conclusions
This mini review summarizes the work carried out to generate, maintain, and characterize iPSCs and iPSC-like cells derived from somatic cells of domesticated ruminants. Despite their undeniable potential in agriculture, conservation biology, biotechnology, and as models for preclinical research, iPSC cultures from ruminant livestock species have yet to be fully optimized. Developing uniform (i) reprogramming protocols, (ii) characterization criteria, and (iii) methods for the long-term maintenance of ruminant iPSCs needs to be prioritized to establish stable, well-defined ruminant iPSC lines that can be used to improve animal and human well-being.
Author contributions
PW and RH: original draft preparation and editing. GV: conceptualization and editing. All authors contributed to the article and approved the submitted version.
Conflict of interest
The authors declare that the research was conducted in the absence of any commercial or financial relationships that could be construed as a potential conflict of interest.
Publisher's note
All claims expressed in this article are solely those of the authors and do not necessarily represent those of their affiliated organizations, or those of the publisher, the editors and the reviewers. Any product that may be evaluated in this article, or claim that may be made by its manufacturer, is not guaranteed or endorsed by the publisher.
References
1. Mohammad Fakhrul Islam S, Karim Z. World's Demand for Food and Water: The Consequences of Climate Change. In:Desalination - Challenges and Opportunities. Hossein Davood Abadi Farahani M, Vatanpour V, Hooshang Taheri A, , editors. IntechOpen. (2020). Available online at: https://www.intechopen.com/books/desalination-challenges-and-opportunities/world-s-demand-for-food-and-water-the-consequences-of-climate-change (accessed November 6, 2022).
2. Rojas-Downing MM, Nejadhashemi AP, Harrigan T, Woznicki SA. Climate change and livestock: Impacts, adaptation, and mitigation. Clim Risk Manag. (2017) 16:145–63. doi: 10.1016/j.crm.2017.02.001
3. Hou DR, Jin Y, Nie XW, Zhang ML, Ta N, Zhao LH, et al. Derivation of porcine embryonic stem-like cells from in vitro-produced blastocyst-stage embryos. Sci Rep. (2016) 6:25838. doi: 10.1038/srep25838
4. Takahashi K, Yamanaka S. Induction of pluripotent stem cells from mouse embryonic and adult fibroblast cultures by defined factors. Cell. (2006) 126:663–76. doi: 10.1016/j.cell.2006.07.024
5. Takahashi K, Tanabe K, Ohnuki M, Narita M, Ichisaka T, Tomoda K, et al. Induction of pluripotent stem cells from adult human fibroblasts by defined factors. Cell. (2007) 131:861–72. doi: 10.1016/j.cell.2007.11.019
6. Yu J, Vodyanik MA, Smuga-Otto K, Antosiewicz-Bourget J, Frane JL, Tian S, et al. Induced pluripotent stem cell lines derived from human somatic cells. Science. (2007) 318:1917–20. doi: 10.1126/science.1151526
7. Yuan Y. Capturing bovine pluripotency. Proc Natl Acad Sci USA. (2018) 115:1962–3. doi: 10.1073/pnas.1800248115
8. Bogliotti YS, Wu J, Vilarino M, Okamura D, Soto DA, Zhong C, et al. Efficient derivation of stable primed pluripotent embryonic stem cells from bovine blastocysts. Proc Natl Acad Sci USA. (2018) 115:2090–5. doi: 10.1073/pnas.1716161115
9. Vilarino M, Alba Soto D, Soledad Bogliotti Y, Yu L, Zhang Y, Wang C, et al. Derivation of sheep embryonic stem cells under optimized conditions. Reproduction. (2020) 160:761–72. doi: 10.1530/REP-19-0606
10. Behboodi E, Bondareva A, Begin I, Rao K, Neveu N, Pierson JT, et al. Establishment of goat embryonic stem cells from in vivo produced blastocyst-stage embryos: goat embryonic stem cells. Mol Reprod Dev. (2011) 78:202–11. doi: 10.1002/mrd.21290
11. De AK, Garg S, Singhal DK, Malik H, Mukherjee A, Jena MK, et al. Derivation of goat embryonic stem cell-like cell lines from in vitro produced parthenogenetic blastocysts. Small Ruminant Research. (2013) 113:145–53. doi: 10.1016/j.smallrumres.2013.01.018
12. Muzaffar M, Selokar NL, Singh KP, Zandi M, Singh MK, Shah RA, et al. Equivalency of buffalo (Bubalus Bubalis) embryonic stem cells derived from fertilized, parthenogenetic, and hand-made cloned embryos. Cell Reprogram. (2012) 14:267–79. doi: 10.1089/cell.2011.0090
13. Shah SM, Saini N, Ashraf S, Singh MK, Manik R, Singla SK, et al. Development of buffalo (Bubalus bubalis) embryonic stem cell lines from somatic cell nuclear transferred blastocysts. Stem Cell Res. (2015) 15:633–9. doi: 10.1016/j.scr.2015.10.010
14. Anand T, Kumar D, Singh M, Shah R, Chauhan M, Manik R, et al. Buffalo (Bubalus bubalis) embryonic stem cell-like cells and preimplantation embryos exhibit comparable expression of pluripotency-related antigens: pluripotency-related surface antigens in buffalo stem cells and embryos. Reprod Domest Anim. (2011) 46:50–8. doi: 10.1111/j.1439-0531.2009.01564.x
15. Zhang W. Teratoma formation: A tool for monitoring pluripotency in stem cell research. In: StemBook. (2014). Available online at: http://www.stembook.org/node/723 (accessed December 9, 2022).
16. Pillai VV, Koganti PP, Kei TG, Gurung S, Butler WR, Selvaraj V. Efficient induction and sustenance of pluripotent stem cells from bovine somatic cells. Biology Open. (2021) 10:bio058756. doi: 10.1242/bio.058756
17. Su Y, Wang L, Fan Z, Liu Y, Zhu J, Kaback D, et al. Establishment of bovine-induced pluripotent stem cells. IJMS. (2021) 22:10489. doi: 10.3390/ijms221910489
18. Bai C, Li X, Gao Y, Yuan Z, Hu P, Wang H, et al. Melatonin improves reprogramming efficiency and proliferation of bovine-induced pluripotent stem cells. J Pineal Res. (2016) 61:154–67. doi: 10.1111/jpi.12334
19. Kawaguchi T, Tsukiyama T, Kimura K, Matsuyama S, Minami N, Yamada M, et al. Generation of naïve bovine induced pluripotent stem cells using piggybac transposition of doxycycline-inducible transcription factors. PLoS ONE. (2015) 10:e0135403. doi: 10.1371/journal.pone.0135403
20. Wang SW, Wang SSW, Wu DC, Lin YC, Ku CC, Wu CC, et al. Androgen receptor-mediated apoptosis in bovine testicular induced pluripotent stem cells in response to phthalate esters. Cell Death Dis. (2013) 4:e907–e907. doi: 10.1038/cddis.2013.420
21. Cao H, Yang P, Pu Y, Sun X, Yin H, Zhang Y, et al. Characterization of bovine induced pluripotent stem cells by lentiviral transduction of reprogramming factor fusion proteins. Int J Biol Sci. (2012) 8:498–511. doi: 10.7150/ijbs.3723
22. Talluri TR, Kumar D, Glage S, Garrels W, Ivics Z, Debowski K, et al. Derivation and characterization of bovine induced pluripotent stem cells by transposon-mediated reprogramming. Cell Reprogram. (2015) 17:131–40. doi: 10.1089/cell.2014.0080
23. Bressan FF, Bassanezze V, de Figueiredo Pessôa LV, Sacramento CB, Malta TM, Kashima S, et al. Generation of induced pluripotent stem cells from large domestic animals. Stem Cell Res Ther. (2020) 11:247. doi: 10.1186/s13287-020-01716-5
24. Bao L, He L, Chen J, Wu Z, Liao J, Rao L, et al. Reprogramming of ovine adult fibroblasts to pluripotency via drug-inducible expression of defined factors. Cell Res. (2011) 21:600–8. doi: 10.1038/cr.2011.6
25. Li Y, Cang M, Lee AS, Zhang K, Liu D. Reprogramming of sheep fibroblasts into pluripotency under a drug-inducible expression of mouse-derived defined factors. PLoS ONE. (2011) 6:e15947. doi: 10.1371/journal.pone.0015947
26. Liu M, Zhao L, Wang Z, Su H, Wang T, Yang G, et al. Generation of sheep induced pluripotent stem cells with defined dox-inducible transcription factors via piggybac transposition. Front Cell Dev Biol. (2021) 9:785055. doi: 10.3389/fcell.2021.785055
27. Wu X, Tong R, Chen X, Jiang X, He X, Ma L. The miR-302s/367 cluster inhibits the proliferation and apoptosis in sheep fetal fibroblasts via the cell cycle and Pi3k-akt pathways. Mamm Genome. (2021) 32:183–94. doi: 10.1007/s00335-021-09873-5
28. Shi H, Fu Q, Li G, Ren Y, Hu S, Ni W, et al. Roles of p53 and ASF1A in the reprogramming of sheep kidney cells to pluripotent cells. Cell Reprogram. (2015) 17:441–52. doi: 10.1089/cell.2015.0039
29. Zhang Y, He Y, Wu P, Hu S, Zhang Y, Chen C. miR-200c-141 enhances sheep kidney cell reprogramming into pluripotent cells by targeting ZEB1. IJSC. (2021) 14:423–33. doi: 10.15283/ijsc21080
30. Liu J, Balehosur D, Murray B, Kelly JM, Sumer H, Verma PJ. Generation and characterization of reprogrammed sheep induced pluripotent stem cells. Theriogenology. (2012) 77:338–46.e1. doi: 10.1016/j.theriogenology.2011.08.006
31. Sartori C, DiDomenico AI, Thomson AJ, Milne E, Lillico SG, Burdon TG, et al. Ovine-induced pluripotent stem cells can contribute to chimeric lambs. Cell Reprogram. (2012) 14:8–19. doi: 10.1089/cell.2011.0050
32. Chen H, Zuo Q, Wang Y, Song J, Yang H, Zhang Y, et al. Inducing goat pluripotent stem cells with four transcription factor mRNAs that activate endogenous promoters. BMC Biotechnol. (2017) 17:11. doi: 10.1186/s12896-017-0336-7
33. Li L, Zhang D, Ren Y, Ye S, Zheng B, Liu S, et al. The modification of mitochondrial energy metabolism and histone of goat somatic cells under small molecules compounds induction. Reprod Dom Anim. (2019) 54:138–49. doi: 10.1111/rda.13304
34. Sandmaier SES, Nandal A, Powell A, Garrett W, Blomberg L, Donovan DM, et al. Generation of induced pluripotent stem cells from domestic goats: iPSC from domestic goats. Mol Reprod Dev. (2015) 82:709–21. doi: 10.1002/mrd.22512
35. Deng Y, Huang G, Chen F, Testroet ED Li H, Li H, et al. Hypoxia enhances buffalo adipose-derived mesenchymal stem cells proliferation, stemness, and reprogramming into induced pluripotent stem cells. J Cell Physiol. (2019) 234:17254–68. doi: 10.1002/jcp.28342
36. Mahapatra PS, Bag S. Reprogramming of buffalo (Bubalus bubalis) foetal fibroblasts with avian egg extract for generation of pluripotent stem cells. Res Vet Sci. (2014) 96:292–8. doi: 10.1016/j.rvsc.2014.02.008
37. Deng Y, Liu Q, Luo C, Chen S, Li X, Wang C, et al. Generation of induced pluripotent stem cells from buffalo (Bubalus bubalis) fetal fibroblasts with buffalo defined factors. Stem Cells Dev. (2012) 21:2485–94. doi: 10.1089/scd.2012.0018
38. Bernardo AS, Jouneau A, Marks H, Kensche P, Kobolak J, Freude K, et al. Mammalian embryo comparison identifies novel pluripotency genes associated with the naïve or primed state. Biology Open. (2018) 1:bio.033282. doi: 10.1242/bio.033282
39. Sommer CA, Christodoulou C, Gianotti-Sommer A, Shen SS, Sailaja BS, Hezroni H, et al. Residual expression of reprogramming factors affects the transcriptional program and epigenetic signatures of induced pluripotent stem cells. PLoS ONE. (2012) 7:e51711. doi: 10.1371/journal.pone.0051711
40. Xu H, Wang B, Ono M, Kagita A, Fujii K, Sasakawa N, et al. Targeted disruption of HLA genes via CRISPR-Cas9 generates iPSCs with enhanced immune compatibility. Cell Stem Cell. (2019) 24:566–578.e7. doi: 10.1016/j.stem.2019.02.005
41. Bavin EP, Atkinson F, Barsby T, Guest DJ. Scleraxis is essential for tendon differentiation by equine embryonic stem cells and in equine fetal tenocytes. Stem Cells Dev. (2017) 26:441–50. doi: 10.1089/scd.2016.0279
42. Liang Q, Monetti C, Shutova MV, Neely EJ, Hacibekiroglu S, Yang H, et al. Linking a cell-division gene and a suicide gene to define and improve cell therapy safety. Nature. (2018) 563:701–4. doi: 10.1038/s41586-018-0733-7
43. Ben-Arye T, Levenberg S. Tissue engineering for clean meat production. Front Sustain Food Syst. (2019) 3:46. doi: 10.3389/fsufs.2019.00046
44. Dashtban M, Panchalingam KM, Shafa M, Ahmadian Baghbaderani B. Addressing Manufacturing Challenges for Commercialization of iPSC-Based Therapies. In:Stem Cells and Good Manufacturing Practices. Turksen K, , editor. New York, NY: Springer US. (2020). p. 179–98. Available online at: http://link.springer.com/10.1007/7651_2020_288 (accessed November 28, 2022).
45. Richie H, Roser M. Environmental Impacts of Food Production. (2020). Available online at: OurWorldInData.org
46. Manyi-Loh C, Mamphweli S, Meyer E, Okoh A. Antibiotic use in agriculture and its consequential resistance in environmental sources: potential public health implications. Molecules. (2018) 23:795. doi: 10.3390/molecules23040795
47. Stephens N, Di Silvio L, Dunsford I, Ellis M, Glencross A, Sexton A. Bringing cultured meat to market: technical, socio-political, and regulatory challenges in cellular agriculture. Trends Food Sci Technol. (2018) 78:155–66. doi: 10.1016/j.tifs.2018.04.010
48. Post MJ. Cultured meat from stem cells: challenges and prospects. Meat Sci. (2012) 92:297–301. doi: 10.1016/j.meatsci.2012.04.008
49. Edwards C. High Steaks. Lab-Grown Bacon Strips Pork Belly Created by UK Food Engineers From Pig Cells. The US Sun. (2020). Available online at: https://www.the-sun.com/lifestyle/tech-old/1185714/lab-grown-bacon-pork-belly-uk/#:~:text=HIGH%20STEAKS-Lab%2Dgrown%20BACON%20strips%20and%20pork%20belly%20created%20by,food%20engineers%20from%20pig%20cells&text=PORK%20belly%20and%20bacon%20strips,testing%20event%20later%20this%20year (accessed December 11, 2022).
50. Rubio N, Datar I, Stachura D, Kaplan D, Krueger K. Cell-based fish: a novel approach to seafood production and an opportunity for cellular agriculture. Front Sustain Food Syst. (2019) 3:43. doi: 10.3389/fsufs.2019.00043
51. Newburger, Emma. FDA says lab-grown meat is safe for human consumption. In: CNBC. (2022). Available online at: https://www.cnbc.com/2022/11/17/fda-says-lab-grown-meat-is-safe-for-human-consumption.html (accessed December 11, 2022).
52. Ran FA, Hsu PD, Wright J, Agarwala V, Scott DA, Zhang F. Genome engineering using the CRISPR-Cas9 system. Nat Protoc. (2013) 8:2281–308. doi: 10.1038/nprot.2013.143
53. Hockemeyer D, Jaenisch R. Induced pluripotent stem cells meet genome editing. Cell Stem Cell. (2016) 18:573–86. doi: 10.1016/j.stem.2016.04.013
54. Valenti MT, Serena M, Carbonare LD, Zipeto D. CRISPR/Cas system: an emerging technology in stem cell research. WJSC. (2019) 11:937–56. doi: 10.4252/wjsc.v11.i11.937
55. Monzani PS, Sangalli JR, de Bem THC, Bressan FF, Fantinato-Neto P, Pimentel JRV, et al. Breeding of transgenic cattle for human coagulation factor IX by a combination of lentiviral system and cloning. Genet Mol Res. (2013) 12:3675–88. doi: 10.4238/2013.February.28.25
56. Hayashi M, Kawaguchi T, Durcova-Hills G, Imai H. Generation of germ cells from pluripotent stem cells in mammals. Reprod Med Biol. (2018) 17:107–14. doi: 10.1002/rmb2.12077
57. Shah SM, Singla SK, Palta P, Manik RS, Chauhan MS. Retinoic acid induces differentiation of buffalo (Bubalus bubalis) embryonic stem cells into germ cells. Gene. (2017) 631:54–67. doi: 10.1016/j.gene.2017.05.037
58. Hou Z, An L, Han J, Yuan Y, Chen D, Tian J. Revolutionize livestock breeding in the future: an animal embryo-stem cell breeding system in a dish. J Animal Sci Biotechnol. (2018) 9:90. doi: 10.1186/s40104-018-0304-7
59. Mcelwain TF, Thumbi SM. Animal pathogens and their impact on animal health, the economy, food security, food safety and public health: -EN- -FR- Les agents pathogènes d'origine animale et leur impact sur la santé animale, l'économie, la sécurité alimentaire, la sécurité sanitaire des aliments et la santé publique -ES- Los patógenos animales y su impacto en la sanidad animal, la economía, la seguridad alimentaria, la higiene de los alimentos y la salud pública. Rev Sci Tech OIE. (2017) 36:423–33. doi: 10.20506/rst.36.2.2663
60. Doss MX, Sachinidis A. Current challenges of iPSC-based disease modeling and therapeutic implications. Cells. (2019) 8:403. doi: 10.3390/cells8050403
61. Cravero D, Martignani E, Miretti S, Accornero P, Pauciullo A, Sharma R, et al. Generation of induced pluripotent stem cells from bovine epithelial cells and partial redirection toward a mammary phenotype in vitro. Cell Reprogram. (2015) 17:211–20. doi: 10.1089/cell.2014.0087
62. Akyüz B, Erturul O. Detection of bovine leukocyte adhesion deficiency (BLAD) in Turkish native and Holstein cattle. Acta Veterinaria Hungarica. (2006) 54:173–8. doi: 10.1556/AVet.54.2006.2.4
63. Mi S, Ogawa H, Ikeda M, Kawashima S, Ito K. Platelet dysfunction in chediak-higashi syndrome-affected cattle. J Vet Med Sci. (2002) 64:751–60. doi: 10.1292/jvms.64.751
64. Anderson RH, Francis KR. Modeling rare diseases with induced pluripotent stem cell technology. Mol Cell Probes. (2018) 40:52–9. doi: 10.1016/j.mcp.2018.01.001
65. Boerjan ML, Freijnagel S, Rhind SM, Meijer GAL. The potential reproductive effects of exposure of domestic ruminants to endocrine disrupting compounds. Anim Sci. (2002) 74:3–12. doi: 10.1017/S1357729800052164
66. Polzin VJ, Anderson DL, Anderson GB, BonDurant RH, Butler JE, Pashens RL, et al. Production of sheep-goat chimeras by inner cell mass transplantation. J Animal Sci. (1987) 65:325–30. doi: 10.2527/jas1987.651325x
67. Bain G, Qin Q, Feng G, Lu F, Shi D. A preliminary study on making interspecific chimeras between cattle and buffalo by aggregating blastomeres. In: China Animal Husbandry and Veterinary Medicine. Nanning: Animal Reproduction Institute, Guangxi University (2019).
68. Wu J, Platero-Luengo A, Sakurai M, Sugawara A, Gil MA, Yamauchi T, et al. Interspecies chimerism with mammalian pluripotent stem cells. Cell. (2017) 168:473–486.e15. doi: 10.1016/j.cell.2016.12.036
69. Bourret R, Martinez E, Vialla F, Giquel C, Thonnat-Marin A, De Vos J. Human–animal chimeras: ethical issues about farming chimeric animals bearing human organs. Stem Cell Res Ther. (2016) 7:87. doi: 10.1186/s13287-016-0345-9
70. Han X, Han J, Ding F, Cao S, Lim SS Dai Y, et al. Generation of induced pluripotent stem cells from bovine embryonic fibroblast cells. Cell Res. (2011) 21:1509–12. doi: 10.1038/cr.2011.125
71. Canizo JR, Vazquez Echegaray C, Klisch D, Aller JF, Paz DA, Alberio RH, et al. Exogenous human OKSM factors maintain pluripotency gene expression of bovine and porcine iPS-like cells obtained with STEMCCA delivery system. BMC Res Notes. (2018) 11:509. doi: 10.1186/s13104-018-3627-8
72. Pillai VV, Kei TG, Reddy SE, Das M, Abratte C, Cheong SH, et al. Induced pluripotent stem cell generation from bovine somatic cells indicates unmet needs for pluripotency sustenance. Anim Sci J. (2019) 90:1149–60. doi: 10.1111/asj.13272
73. Bessi BW, Botigelli RC, Pieri NCG, Machado LS, Cruz JB, de Moraes P, et al. Cattle in vitro induced pluripotent stem cells generated and maintained in 5 or 20% oxygen and different supplementation. Cells. (2021) 10:1531. doi: 10.3390/cells10061531
74. Botigelli RC, Pieri NCG, Bessi BW, Machado LS, Bridi A, de Souza AF, et al. Acquisition and maintenance of pluripotency are influenced by fibroblast growth factor, leukemia inhibitory factor, and 2i in bovine-induced pluripotent stem cells. Front Cell Dev Biol. (2022) 10:938709. doi: 10.3389/fcell.2022.938709
75. German SD, Campbell KHS, Thornton E, McLachlan G, Sweetman D, Alberio R. Ovine induced pluripotent stem cells are resistant to reprogramming after nuclear transfer. Cell Reprogram. (2015) 17:19–27. doi: 10.1089/cell.2014.0071
76. Song H, Li H, Huang M, Xu D, Gu C, Wang Z, et al. Induced pluripotent stem cells from goat fibroblasts: generation of goat iPSCs. Mol Reprod Dev. (2013) 80:1009–17. doi: 10.1002/mrd.22266
77. Tai D, Liu P, Gao J, Jin M, Xu T, Zuo Y, et al. Generation of arbas cashmere goat induced pluripotent stem cells through fibroblast reprogramming. Cell Reprogram. (2015) 17:297–305. doi: 10.1089/cell.2014.0107
78. Chu Z, Niu B, Zhu H, He X, Bai C, Li G, et al. PRMT5 enhances generation of induced pluripotent stem cells from dairy goat embryonic fibroblasts via down-regulation of p53. Cell Prolif. (2015) 48:29–38. doi: 10.1111/cpr.12150
79. Guo Y, Yu T, Lei L, Duan A, Ma X, Wang H. Conversion of goat fibroblasts into lineage-specific cells using a direct reprogramming strategy: direct reprogramming of goat fibroblasts. Anim Sci J. (2017) 88:745–54. doi: 10.1111/asj.12700
80. Mahapatra PS, Singh R, Kumar K, Sahoo NR, Agarwal P, Mili B, et al. Valproic acid assisted reprogramming of fibroblasts for generation of pluripotent stem cells in buffalo (Bubalus bubalis). Int J Dev Biol. (2017) 61:81–8. doi: 10.1387/ijdb.160006sb
81. Kumar D, Anand T, Vijayalakshmy K, Sharma P, Rajendran R, Selokar NL, et al. Transposon mediated reprogramming of buffalo fetal fibroblasts to induced pluripotent stem cells in feeder free culture conditions. Res Vet Sci. (2019) 123:252–60. doi: 10.1016/j.rvsc.2019.01.015
82. Rawat N, Singh MK, Sharma T, Vats P, Nagoorvali D, Palta P, et al. Media switching at different time periods affects the reprogramming efficiency of buffalo fetal fibroblasts. Animal Biotechnol. (2021) 32:155–68. doi: 10.1080/10495398.2019.1671435
Keywords: ruminants, cellular reprogramming, characterization, induced pluripotency, stem cells
Citation: Weeratunga P, Harman RM and Van de Walle GR (2023) Induced pluripotent stem cells from domesticated ruminants and their potential for enhancing livestock production. Front. Vet. Sci. 10:1129287. doi: 10.3389/fvets.2023.1129287
Received: 21 December 2022; Accepted: 31 January 2023;
Published: 20 February 2023.
Edited by:
Iris Maria Gerner, University of Veterinary Medicine Vienna, AustriaReviewed by:
Dharmendra Kumar, Central Institute for Research on Buffaloes (ICAR), IndiaYoung Tang, Northwest A&F University, China
Copyright © 2023 Weeratunga, Harman and Van de Walle. This is an open-access article distributed under the terms of the Creative Commons Attribution License (CC BY). The use, distribution or reproduction in other forums is permitted, provided the original author(s) and the copyright owner(s) are credited and that the original publication in this journal is cited, in accordance with accepted academic practice. No use, distribution or reproduction is permitted which does not comply with these terms.
*Correspondence: Gerlinde R. Van de Walle, Z3J2MjNAY29ybmVsbC5lZHU=