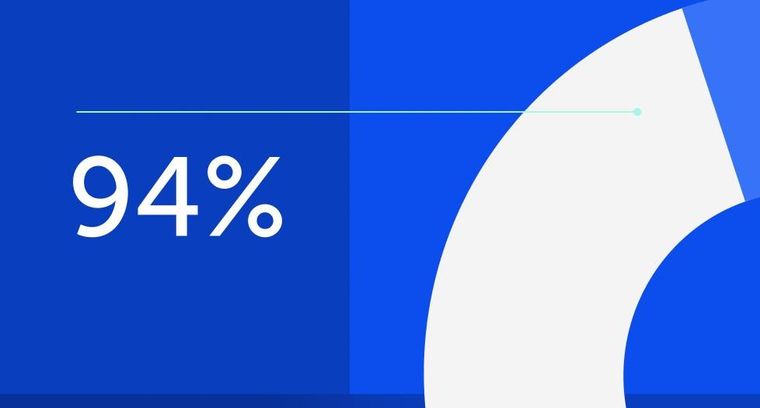
94% of researchers rate our articles as excellent or good
Learn more about the work of our research integrity team to safeguard the quality of each article we publish.
Find out more
ORIGINAL RESEARCH article
Front. Vet. Sci., 24 July 2023
Sec. Veterinary Epidemiology and Economics
Volume 10 - 2023 | https://doi.org/10.3389/fvets.2023.1127819
This article is part of the Research TopicLivestock and its role in the emergence, spread, and evolution of antimicrobial resistance: Animal-to-human or animal-to-environment transmissionView all 10 articles
Bacteria are the dominant particulate matter in livestock houses and can threaten animal and public health. Antimicrobial resistance (AMR) is a crucial concern worldwide, and nationwide measures established based on the One Health approach are being implemented in many countries. This requires multidisciplinary perspectives and collaboration among the human, animal, and environmental sectors. However, information on the AMR risk in livestock house aerosol is limited, especially its association with antimicrobial usage (AMU). Therefore, this study was conducted to reveal the AMR profile of Staphylococcus, the major bacterial genus in the aerosol of the piggeries of Japanese farms, and the association between farm-level AMU and AMR. The investigation at 10 farrow-to-finish pig farms revealed that regardless of the sampling season and the piggery group, the resistance rate of isolated staphylococci for oxacillin, erythromycin, and lincomycin was more than 40% of the median and tended to be higher than that for other antimicrobials. The AMU adjusted by the defined daily dose (DDD-adjusted AMU) in the fattening piggery group was significantly higher than that in the sow piggery group (p < 0.05). Finally, for the fattening piggery group, the generalized linear mixed model revealed that the AMR rate for oxacillin, erythromycin, tetracycline, and chloramphenicol was positively associated with the corresponding class-based DDD-adjusted AMU of penicillins (odds ratio (OR) = 2.63, p = 0.03), macrolides (OR = 6.89, p = 0.0001), tetracyclines (OR = 2.48, p = 0.04), and amphenicols (OR = 3.22, p = 0.03), respectively. These significant positive associations observed in this study imply that the resistance rate for these antimicrobials may decrease by reducing the corresponding antimicrobials’ use. In addition, the resistance rates for erythromycin and chloramphenicol also displayed a positive association with the AMU of antimicrobial classes other than macrolides and amphenicols, respectively. The mechanism underlying these phenomena is unclear; therefore, further evaluation will be needed. As limited studies have reported staphylococci in piggery aerosol and its AMR with quantitative AMU, these results based on on-farm investigations are expected to aid in establishing countermeasures for AMR of aerosol bacteria in pig farms.
Particulate matter in aerosol is an essential indicator of air pollution (1). Toxic and harmful substances, including microorganisms and bacteria, constitute air pollution (2, 3), dominating livestock farm aerosols (4). Therefore, it is rational that bacteria from the environment and animals threaten both animal and public health. For instance, the increased density of animals in piggeries under an intensive production system often results in poor air quality (5). This phenomenon increases the risk of various opportunistic infections, unless ventilation is appropriately managed. Moreover, pig house farmers are at higher risk of respiratory diseases than chicken, cattle, or sheep farmers (6, 7).
Moreover, as the world faces multiple health challenges, antimicrobial resistance (AMR) is a crucial concern listed among the top 10 global health threats (8, 9). A recent worldwide estimation revealed approximately 4.95 million deaths associated with AMR in 2019 (10). Excessive and inappropriate antimicrobial usage (AMU) has been primary reason; therefore, nationwide measures based on the action plan of each country, established based on the Global Action Plan with the One Health approach, are being taken (11). Thus, the human, animal, and environmental sectors need to have multidisciplinary perspectives and collaborate by sharing the insights obtained from each sector.
In Japan, the total quantity of antimicrobials based on the weight of active substances was 1,761.4 tons in 2018. Among those, 36.7 and 12.3% accounted for the livestock sector and feed additives, respectively. Moreover, 74.5% of those for the livestock sector were used in pig production, with tetracyclines, penicillins, sulfonamides, and macrolides as the major classes (12).
Although information on bacterial AMR in the piggery aerosol is available (13), that on its association with AMU is limited. Therefore, this study revealed the AMR characteristics of staphylococci, including animal and human pathogens. We also aimed to evaluate the association between farm-level AMU and AMR of staphylococci. This study’s findings would aid in establishing better countermeasures for AMR in piggeries for animal and public health.
With the cooperation of the field veterinarians from The Japanese Association of Swine Veterinarians,1 consent for participation in this observational study was obtained from ten farrow-to-finish pig farms on a convenient basis. Between November 2017 and July 2020, each farm was visited twice in the warm (spring and summer) and cold (autumn and winter) seasons, respectively, except for farm E (visited only once in the cold season). Brief descriptions of these farms are presented in Table 1 with the varied farm size of 70–1,790, based on sow number. At each visit, aerosol samples were collected from five pig houses of different life stages, including sow stall and farrowing houses as the sow piggery group and the weaners, growers, and finishers houses as the fattening piggery group. Using a commercial air sampler (CORIOLIS MICRO, Bertin Technologies SAS, France) placed at the center of each piggery, 3,000 L of air was passed into 10 mL of sterilized phosphate-buffered saline (PBS, Dulbecco’s PBS (−) “Nissui” Nissui Pharmaceutical Co., Ltd., Tokyo, Japan) for 10 min. These PBS samples were brought to the National Institute of Animal Health for laboratory investigations.
In total, 19 sampling visits were made in ten cold and nine warm seasons. Samples were obtained from both piggery groups during all nine warm season visits, meaning nine sow and nine fattening piggeries. However, during the ten cold season visits, due to the technical condition, samples were collected from only the fattening piggery group of Farm D, which meant nine sow and ten fattening piggeries were targeted. This sampling frame is summarized in Figure 1.
Figure 1. Flowchart of the sampling process in this study based on farm visits by season and piggery group.
Within 20 h after the on-farm sampling, 100 μL of the PBS sample obtained from each piggery was inoculated on 5% sheep blood in trypticase soy agar (TSA) (BD Trypticase Soy Agar with 5% Sheep Blood, Nippon Becton, Dickinson, and Company, Japan) and mannitol salt agar (MSA) (Mannitol Salt Agar “Nissui,” Nissui Pharmaceutical Co., Ltd., Tokyo, Japan) and aerobically cultured for 16–20 h at 37°C. MSA was used as gram-positive bacteria selective agar, especially, salt-tolerant bacteria, which included some members of the Staphylococcus genus. Then, 10 isolates were randomly selected from each medium and stored in 10% glycerol-added Muller Hinton broth (Difco; BD, New Jersey, United States) at −80°C until identification.
All the isolates were identified using species-specific PCR for staphylococci, assumed as the dominant genus by the authors, following previously established procedures (14, 15). For those not identified using this PCR, partial gap gene sequencing (16) or 16S rRNA partial sequencing using a commercial kit (Bacterial 16S rDNA PCR Kit, Takara Bio Inc., Shiga, Japan) was applied following the manufacturer’s instructions. In addition, sequence data were analyzed to determine the most likely species, referring to the EzBioCloud Database.2
The identified isolates’ distribution by genus was summarized. In particular, the Chi-square test statistically evaluated the proportion of staphylococci among all the bacterial isolates in each farm by the seasons and the piggery groups.
For all the isolated staphylococci, the minimum inhibitory concentration (MIC) values of the 11 antimicrobials below were determined using a commercial kit (Dry Plate “Eiken,” Eiken Chemical Co., Ltd., Japan). Antimicrobial phenotypes were interpreted based on the breakpoints provided by the CLSI guidelines: 0.5 μg/mL for oxacillin (OXA), 0.5 μg/mL for ampicillin (AMP), 8.0 μg/mL for cefazoline (CFZ), 16.0 μg/mL for kanamycin (KAN), 16.0 μg/mL for gentamycin (GEN), 8.0 μg/mL for erythromycin (ERY), 16.0 μg/mL for tetracycline (TET), 32.0 μg/mL for chloramphenicol (CHL), 32.0 μg/mL for vancomycin (VAN), 8.0 μg/mL for lincomycin (LCM), and 4.0 μg/mL for ciprofloxacin (CIP), respectively (17). In addition, the following quality control strains were also assessed: Staphylococcus aureus ATCC 29213, Enterococcus faecalis ATCC 29212, Escherichia coli ATCC 25923, and Pseudomonas aeruginosa ATCC 27853.
The resistance rate (%) for each antimicrobial was defined as the proportion of resistant staphylococci isolates among the staphylococci isolates analyzed using the antimicrobial susceptibility test and the distribution of each antimicrobial based on the sampling seasons [n = 19 for “cold”s, and 18 for “warm”s (Figure 1)] and piggery groups [n = 18 for “sow”s, and 19 for “fattening”s (Figure 1)] was compared using the Mann–Whitney U test.
Annual antimicrobial product purchases were recorded for the piggery groups in each farm. This study used the previous year’s volume as the reference AMU data for a farm visit between January and June. The current year’s data were adopted for visits between July and December. Then, the annual mean treatment days (head*day) were estimated as follows:
The defined daily dose (DDD) is the Japanese pig production-specific indicator established previously (18, 19), based on the original concept and definition by the World Health Organization (20). These annual mean treatment days were the annual DDD-adjusted usage of each commercial product and summed up by the antimicrobial classes, which were tetracyclines (TETs), amphenicols (APCs), penicillins (PENs), cephalosporins (CEPs), sulfonamides (SULs), pyrimidines (PMDs), macrolides (MCLs), lincosamides (LCMs), aminoglycosides (AGDs), quinolones (QUIs), polymixins (PMXs), and pleuromutilins (PLMs), respectively. The class-based annual DDD-adjusted AMU was statistically compared by the seasons [n = 19 of “cold”s, and 18 of “warm”s (Figure 1)] and piggery groups [n = 18 of “sow”s, and 19 of “fattening”s Figure 1)], respectively, using the Mann–Whitney U test.
Association between the resistance rate of staphylococci for each antimicrobial and class-based annual DDD-adjusted AMU was explored using the generalized linear mixed model on each piggery group (n = 18 for the sow group and n = 19 for the fattening group). Considering the difference in the number of staphylococci successfully obtained on each sampling visit, raw data used for resistance rate calculation were incorporated into the model as the dependent variable; both tested and resistant staphylococci isolates were directly employed. Moreover, with the various farms cooperating, as presented in Table 1, sampling season was forced into the model, and the farm was employed as the random effect. Therefore, the model is described as follows:
Where in logit () of the model outcome represents the resistance rate accounting for the tested and resistant staphylococci isolates, is the model intercept, is the dichotomous data of cold or warm season, is the fixed effect as the dichotomous data classified as “high” or “low” based on the median of the class-based annual DDD-adjusted AMU, is its coefficient, is the farm as the random effect, and is the binomially distributed residual term.
If the resistance rate to an antimicrobial revealed a positive and significant association with “high” class-based annual DDD-adjusted AMU of its class, a multivariable model for the associations with the AMU of other classes was also explored. The final model met the minimum Akaike’s Information Criterion (AIC), and statistical significance was set at p < 0.05 for the remaining independent variables with positive fixed effects.
The statistical modeling and other tests mentioned above were performed using R version 4.1.0.3 Primarily, the “glmmML” package version 1.1.34 was used for the generalized linear mixed model.
Animal ethics approval was not required for this study as the samples consisted of piggery aerosol and were collected in the presence of the veterinarians during their routine farm visits for veterinary care and consultation.
In total, 915 bacterial isolates were obtained from TSA, and the genus-level description is summarized in Figure 2. The most dominant genus was Staphylococcus (n = 610, 66.7%), followed by Aerococcus (n = 85, 9.3%) and Rothia (n = 50, 5.5%). Finally, 1,113 staphylococci isolated from TSA (n = 610) and MSA (n = 503) underwent the antimicrobial susceptibility test, respectively.
Figure 2. Genus description of bacterial isolates from the aerosol in ten pig farms (n = 915 obtained by trypticase soy agar).
The proportion of staphylococci exceeded 50% in most sampling visits. Farms A and H had over 70%. Apart from Farm E, which was visited once, no intra-farm significant seasonal difference was observed in the staphylococci proportion (Figure 3A, Chi-square test: p > 0.05). Sow and fattening piggeries had over 40% of staphylococci among the isolates. Farm A’s sow piggery group and both piggery groups of Farm H had over 80%. Only Farm C and I had significantly higher proportions of staphylococci in the fattening piggery group than in the sow piggery group (Figure 3B, Chi-square test: p = 0.02 and 0.03 for Farm C and Farm I, respectively).
Figure 3. The proportion (%) of staphylococci from the aerosol in ten pig farms by sampling season (A) and piggery group (B). The last letter of each item denotes the following: C: cold season, W: warm season, S: sow piggery group, and F: fattening piggery group. *: p < 0.05 as revealed by the Mann–Whitney U test.
Among the 1,113 staphylococci isolates, the most dominant specie was S. sciuri (which was renamed Mammaliicoccus sciuri in 2020) (n = 265, 23.8%), and others had <10% each (Table 2). The top five species S. sciuri, S. cohnii, S. saprophyticus, S. haemolyticus, and S. chromogenes dominated over 40% of each farm and some over 80% (data not shown).
Table 2. Species description of staphylococci isolated from the aerosol in ten pig farms (n = 1,113).
For 10 of the 11 tested antimicrobials (all the isolates were susceptible to VAN), datasets of the resistance rate of staphylococci by the seasons (Figure 4A and Supplementary file 1) and piggery groups (Figure 4B and Supplementary file 2) were obtained. Regardless of the seasons and piggery groups, resistance rates for OXA, ERY, and LCM were > 40% of the median and tended to be higher than those of other antimicrobials.
Figure 4. Distribution of the resistance rate for ten antimicrobials of staphylococci from the aerosol in ten pig farms by sampling season (A) and piggery group (B). The last letter of each item denotes the following: C: cold season, W: warm season, S: sow piggery group, and F: fattening piggery group. OXA, oxacillin; AMP, ampicillin; CFZ, cefazoline; KAN, kanamycin; GEN, gentamycin; ERY, erythromycin; TET, tetracycline; CHL, chloramphenicol; LCM, lincomycin; CIP, ciprofloxacin. *: p < 0.05 as revealed by the Mann–Whitney U test.
A significant seasonal difference was only identified in the resistance rate for OXA, with the median for the cold and warm seasons being 65.4 and 80.7%, respectively (Figure 4A and Supplementary file 1, p = 0.03 as revealed by the Mann–Whitney U test). In contrast, a significant between-piggery group difference was identified in OXA, AMP, ERY, and CHL. The resistance rates for these four antimicrobials in the fattening piggery group were significantly higher than those in the sow piggery group, with a median of 78.8 and 58.0% for OXA, 57.1 and 31.8% for AMP, 82.4 and 48.4% for ERY, and 45.5 and 21.1% for CHL for the fattening and sow piggery groups, respectively (Figure 4B and Supplementary file 2, all p < 0.05 as revealed by the Mann–Whitney U test).
Figure 5 illustrates the distribution of the class-based annual DDD-adjusted AMU. The AMU varied by farm; however, no intra-class difference was identified by season (Figure 5A and Supplementary file 3, all p > 0.45 as revealed by the Mann–Whitney U test). In contrast, a between-piggery group difference was identified in the AMU of all the classes, except PMXs and PLMs. Therefore, the fattening piggery group had a significantly higher AMU than the sow piggery group, with zero medians for all classes, except TETs and MCLs (Figure 5B and Supplementary file 4, all p < 0.05 as revealed by the Mann–Whitney U test).
Figure 5. Distribution of the defined-daily-dose-adjusted annual usage of 12 antimicrobial classes in ten pig farms by sampling season (A) and piggery group (B). The last letter of each item denotes the following: C: cold season, W: warm season, S: sow piggery group, and F: fattening piggery group. TETs, tetracyclines; APCs, amphenicols; PENs, penicillins; CEPs, cephalosporins; SULs, sulfonamides; PMDs, pyrimidines; MCLs, macrolides; LCMs, lincosamides; AGDs, aminoglycosides; QUIs, quinolones; PMXs, polymyxins; and PLMs, pleuromutilins. *: p < 0.05 as revealed by the Mann–Whitney U test.
Table 3 presents four final models obtained by statistical modeling from the datasets of the fattening piggery group for the association between the class-based annual DDD-adjusted AMU and resistance rate of staphylococci. Out of the 11 evaluated antimicrobials, the resistance rate for OXA, ERY, TET, and CHL was significantly associated with the AMU of the corresponding PENs, MCLs, TETs, and APCs, respectively.
Table 3. Final models of resistance rate for four antimicrobials of staphylococci from the aerosol in fattening piggeries of ten Japanese pig farms in association with the annual antimicrobial class-based usage.
Regarding OXA, the final model included only PENs, and its “high” usage was associated with a higher resistance rate for OXA [odds ratio (OR) and 95% confidence interval (CI)] = 2.36 (1.11, 5.05), p = 0.03). For ERY, the final model included MCLs, APCs, and LCMs. A “high” usage of these three antimicrobial classes was independently associated with a higher resistance rate for ERY (OR (95% CI) = 6.89 (2.53, 18.73), p = 0.0001 for MCLs, OR (95% CI) = 4.81 (1.17, 19.69), p = 0.03 for APCs, and OR (95% CI) = 20.91 (3.60, 121.51), p = 0.001 for LCMs, respectively). For TET, the final model included only TETs, and its “high” usage was associated with a higher resistance rate for TET (OR (95% CI) = 2.48 (1.03, 5.99), p = 0.04). In addition, for CHL, the final model included APCs and TETs. A “high” usage of these antimicrobial classes was independently associated with a higher resistance rate for CHL (OR (95% CI) = 3.22 (1.14, 9.12), p = 0.03 for APCs, and (OR (95% CI) = 3.39 (1.73, 6.62), p = 0.0004 for TETs, respectively). No significant interaction terms were identified in all the final models.
Conversely, analyses of the sow piggery group did not reveal any significantly positive association between the AMU and resistance rate. However, the resistance rates for KAN and TET had a marginally positive association with “high” AGDs and TETs use, respectively (p = 0.09 and 0.11, respectively, data not shown).
Previous studies on aerosol bacteria in piggeries have been limited thus far (21); therefore, White et al. (22) evaluated piggery staphylococci for their viability, capturability, inflammogenicity, and biofilm-forming capacity. Eisenlöffel et al. (23) and Tenzin et al. (24) revealed the impact of dust filtration and decontamination. These studies are relevant; however, once countermeasures are in operation, it is better to understand the extent of bacterial distribution and AMR status in these years to strengthen the rationale of the activities. However, few studies have targeted staphylococci AMR with quantitative AMU in Japan. Therefore, this study evaluated the bacterial profile of aerosol in Japanese piggeries, AMR characteristics, and the association between farm-level AMU and AMR, especially for staphylococci.
The aerobic culture using TSA revealed that most isolates were gram-positive bacteria (Figure 2), including the hazardous genus for animal and public health. The most dominant genus was Staphylococcus. In this study, staphylococci exceeded 40% and did not differ by sampling season and piggery group in each farm, with a few exceptions (Figure 3). Seasonal differences in sand dust in the general environment influence the bacterial community during aerosol pollution (25); nonetheless, the bacterial distribution stability observed in this study might be due to the relatively steady and closed state in the piggery based on the firm on-farm management system. These results imply the importance of staphylococci among aerosol bacteria and necessitate the maintenance or improvement of on-farm biosecurity levels, especially ventilation and humidity control in piggeries, to prevent clinical diseases in pigs. Further, workers need the shower-in and-out operation and change to washed and clean clothes and disinfected boots before they start their daily tasks. These procedures would promote animal and occupational health.
Among these staphylococci, the most dominant specie S. sciuri is a principally animal-associated bacterial species on the skin and mucosal surfaces of various pets and farm and wild animals. However, its clinical relevance in humans is increasing (26), and this bacterium is ubiquitous in human wound infection (27, 28). S. hyicus and S. aureus are occasionally involved in pig infections (29). Moreover, S. hyicus commonly occurs in the nares and on the hairy cutaneous areas of pigs; therefore, it sporadically induces exudative epidermitis in 5–60 d-old pigs along with other staphylococci, such as S. chromogenes and S. aureus (30). Livestock-associated methicillin-resistant S. aureus is more recognized as a public health concern, mainly associated with pigs. In Japan, its presence has been investigated using nasal swabs from slaughtered pigs (31). Given the present situation, there have been few evaluations on the environmental risks of each specie isolated from piggery aerosols. Therefore, a detailed species-based investigation is highly needed under the rational sampling frame in the future.
AMR was revealed for 11 antimicrobials. A high resistance rate of staphylococci was observed for OXA, ERY, and LCM (Figure 3). The influence of season on the resistance rate was not identified in all antimicrobials, except OXA (Figure 4A). The class-based annual DDD-adjusted AMU did not exhibit seasonal differences (Figure 5A). In contrast, the resistance rate in the fattening piggery group was significantly higher than that in the sow piggery group for OXA and AMP of PENs, ERY of MCLs, and CHL of APCs (Figure 4B) as the AMU of the 10 classes, including PENs, MCLs, and APCs, was also higher in the fattening piggery group (Figure 5B). These results indicated that the AMU of the corresponding class might influence some antimicrobials’ resistance compared with environmental conditions. Generally, bacterial survival relies on various factors, such as bacterial species and their burden (32, 33) and environmental conditions, including the type of surface materials, ambient temperature, UV radiation extent, and water and nutrient availability (34, 35). These factors may affect AMR regardless of the bacterial isolates.
From the statistical modeling of the fattening piggery group, the resistance rate for four antimicrobials, including OXA, ERY, TET, and CHL, was positively associated with the AMU of the corresponding class (Table 3). This implies that the resistance rate for these antimicrobials might be decreased by reducing the use of the corresponding antimicrobials.
Moreover, the modeling identified an association between the resistance rate for ERY and the AMU of APCs and LCMs, in addition to MCLs. A similar result was obtained in the association between the resistance rate to CHL and the AMU of TETs, in addition to APCs. The mechanism of these phenomena is unclear; however, Makita et al. (36) suggested that these issues were due to the natural, cross- or co-selection based on analyses of individual pig-originated Escherichia coli isolates and qualitative AMU. Further evaluation is strongly needed to validate our study.
In contrast, no significant association between the resistance rate and AMU in the dataset of the sow piggery group was identified. The possible reasons could be the relatively lower AMU in this group, which might be insufficient to establish antimicrobial selection. Moreover, considering that the isolates were from the aerosol, they may include both environmental and pig-origin bacteria. Therefore, the AMR in this group was probably influenced by other factors along with the AMU. However, the resistance rate to KAN and TET displayed a marginally positive association with AGDs and TETs. Among these, TETs with relatively high AMUs in the sow piggery group could be the reason.
Some limitations should be considered in interpreting this study’s results. First, as mentioned above, the AMR of aerosol-origin bacteria is influenced by both the AMU and other factors. Therefore, evaluating the pig-origin (including healthy and diseased ones) staphylococci will help better understand the piggery’s AMR risk. Second, this study’s statistical modeling was performed using aggregated data on the resistance rate and AMU, which could have an ecological fallacy (37). However, antimicrobials are administered on a herd basis in the general pig industry; hence, this is the best way to assess the on-farm situation quantitatively. Based on these results, it is essential to further evaluate the effect of the countermeasures aimed at decreasing the resistance rate for single antimicrobials at the farm level and clarifying multidrug resistance. Lastly, all the evaluations on the association between the resistance rate and AMU were performed on a genus basis to provide an overview of staphylococci. Therefore, detailed investigations focusing on each species will be more useful for the species-level measures.
In conclusion, the aerosol bacteria in Japanese pig farms included those that could threaten public and animal health, mostly staphylococci. Staphylococci resistance to some antimicrobials was associated with using the corresponding antimicrobial class, implying that reducing such antimicrobials would decrease resistance. These results should help establish countermeasures for the AMR of aerosol bacteria in pig farms.
The raw data supporting the conclusions of this article will be made available by the authors, without undue reservation.
KK conceptualized the study. KK and SK designed and performed the on-farm investigation, sample collection, and laboratory experiments. IY, YT-A, and SK contributed to the data management of antimicrobial usage. SK analyzed the data and drafted the manuscript in consultation with KK, YT-A, IY, and MK. All authors contributed to the article and approved the submitted version.
This study was conducted under the research project on “Regulatory Research Projects for Food Safety, Animal Health and Plant Protection (grant JPJ008617.17935699/22682153)” funded by the Ministry of Agriculture, Forestry and Fisheries of Japan.
The authors are grateful for the on-farm support by Katsumasa Kure, Hiromichi Ishikawa, and Mitsuo Kagawa from The Japanese Association of Swine Veterinarians as well as for the laboratory support by Yoshie Watanabe.
The authors declare that the research was conducted in the absence of any commercial or financial relationships that could be construed as a potential conflict of interest.
All claims expressed in this article are solely those of the authors and do not necessarily represent those of their affiliated organizations, or those of the publisher, the editors and the reviewers. Any product that may be evaluated in this article, or claim that may be made by its manufacturer, is not guaranteed or endorsed by the publisher.
The Supplementary material for this article can be found online at: https://www.frontiersin.org/articles/10.3389/fvets.2023.1127819/full#supplementary-material
1. Tang, Q, Huang, K, Liu, J, Jin, X, and Li, C. Distribution characteristics of bioaerosols inside pig houses and the respiratory tract of pigs. Ecotoxicol Environ Saf. (2021) 212:112006. doi: 10.1016/j.ecoenv.2021.112006
2. Gou, H, Lu, J, Li, S, Tong, Y, Xie, C, and Zheng, X. Assessment of microbial communities in PM1 and PM10 of Urumqi during winter. Environ Pollut. (2016) 214:202–10. doi: 10.1016/j.envpol.2016.03.073
3. Wang, Y, Li, W, Gao, W, Liu, Z, Tian, S, Shen, R, et al. Trends in particulate matter and its chemical compositions in China from 2013–2017. Sci China Earth Sci. (2019) 62:1857–71. doi: 10.1007/s11430-018-9373-1
4. Zucker, BA, Trojan, S, and Müller, W. Airborne gram-negative bacterial flora in animal houses. J Vet Med B Infect Dis Vet Public Health. (2000) 47:37–46. doi: 10.1046/j.1439-0450.2000.00308.x
5. White, JK, Nielsen, JL, and Madsen, AM. Microbial species and biodiversity in settling dust within and between pig farms. Environ Res. (2019) 171:558–67. doi: 10.1016/j.envres.2019.01.008
6. Radon, K, Danuser, B, Iversen, M, Jörres, R, Monso, E, Opravil, U, et al. Respiratory symptoms in European animal farmers. Eur Respir J. (2001) 17:747–54. doi: 10.1183/09031936.01.17407470
7. Vogelzang, PF, van der Gulden, JW, Preller, L, Heederik, D, Tielen, MJ, and van Schayck, CP. Respiratory morbidity in relationship to farm characteristics in swine confinement work: possible preventive measures. Am J Ind Med. (1996) 30:212–8. doi: 10.1002/(SICI)1097-0274(199608)30:2<212::AID-AJIM13>3.0.CO;2-#
8. World Health Organization (2019). Ten threats to global health in 2019. Available at: https://www.who.int/news-room/spotlight/ten-threats-to-global-health-in-2019 (Accessed November 29, 2022).
9. World Health Organization (2020). 10 global health issues to track in 2021. Available at: https://www.who.int/news-room/spotlight/10-global-health-issues-to-track-in-2021 (Accessed November 29, 2022).
10. Murray, CJ, Ikuta, KS, Sharara, F, Swetschinski, L, Robles Aguilar, G, Gray, A, et al. Global burden of bacterial antimicrobial resistance in 2019: a systematic analysis. Lancet. (2022) 399:629–55. doi: 10.1016/S0140-6736(21)02724-0
11. World Health Organization (2015). Global action plan on antimicrobial resistance. Available at: https://www.who.int/publications/i/item/9789241509763 (Accessed November 29, 2022).
12. Ministry of Health, Labour and Welfare (2021). Nippon AMR one health report (NAOR) 2020. Available at: https://www.mhlw.go.jp/stf/seisakunitsuite/bunya/0000120172.html (Accessed November 29, 2022).
13. Létourneau, V, Nehmé, B, Mériaux, A, Massé, D, Cormier, Y, and Duchaine, C. Human pathogens and tetracycline-resistant bacteria in bioaerosols of swine confinement buildings and in nasal flora of hog producers. Int J Hyg Environ Health. (2010) 213:444–9. doi: 10.1016/j.ijheh.2010.09.008
14. Shome, BR, Das Mitra, S, Bhuvana, M, Krithiga, N, Velu, D, Shome, R, et al. Multiplex PCR assay for species identification of bovine mastitis pathogens. J Appl Microbiol. (2011) 111:1349–56. doi: 10.1111/j.1365-2672.2011.05169.x
15. Campos-Peña, E, Martín-Nuñez, E, Pulido-Reyes, G, Martín-Padrón, J, Caro-Carrillo, E, Donate-Correa, J, et al. Multiplex PCR assay for identification of six different Staphylococcus spp. and simultaneous detection of methicillin and mupirocin resistance. J Clin Microbiol. (2014) 52:2698–701. doi: 10.1128/JCM.00918-14
16. Ghebremedhin, B, Layer, F, König, W, and König, B. Genetic classification and distinguishing of Staphylococcus species based on different partial gap, 16S rRNA, hsp60, rpoB, sodA, and tuf gene sequences. J Clin Microbiol. (2008) 46:1019–25. doi: 10.1128/JCM.02058-07
17. Clinical Laboratory Standards Institute. VET01S, performance standards for antimicrobial disk and dilution susceptibility tests for Bacteria isolated from animals. 3rd ed. PA, United States: Wayne (2015).
18. Takagi, H, Lei, Z, Yamane, I, Yamazaki, H, Kure, K, and Sugiura, K. Establishing DDD values for veterinary antimicrobial products in Japan for measuring antimicrobial use on pig farms. J Jpn Vet Med Assoc. (2020) 73:352–5.
19. Fujimoto, K, Kawasaki, M, Abe, R, Yokoyama, T, Haga, T, and Sugiura, K. Establishing defined daily doses (DDDs) for antimicrobial agents used in pigs, cattle and poultry in Japan and comparing them with European DDD values. PLoS One. (2021) 16:e0245105. doi: 10.1371/journal.pone.0245105
20. WHO Collaborating Centre for Drug Statistics and Methodology (2020). ATCvet Index 2020. Available at: http://www.whocc.no/atcvet/atcvet/ (Accessed November 29, 2022).
21. Cui, H, Zhang, C, Liu, J, Dong, S, Zhao, K, Chen, L, et al. The distribution characteristics of aerosol bacteria in different types of pig houses. Animals (Basel). (2022) 12:1540. doi: 10.3390/ani12121540
22. White, JK, Nielsen, JL, Larsen, CM, and Madsen, AM. Impact of dust on airborne Staphylococcus aureus' viability, culturability, inflammogenicity, and biofilm forming capacity. Int J Hyg Environ Health. (2020) 230:113608. doi: 10.1016/j.ijheh.2020.113608
23. Eisenlöffel, L, Reutter, T, Horn, M, Schlegel, S, Truyen, U, and Speck, S. Impact of UVC-sustained recirculating air filtration on airborne bacteria and dust in a pig facility. PLoS One. (2019) 14:e0225047. doi: 10.1371/journal.pone.0225047
24. Tenzin, S, Ogunniyi, AD, Khazandi, M, Ferro, S, Bartsch, J, Crabb, S, et al. Decontamination of aerosolised bacteria from a pig farm environment using a pH neutral electrochemically activated solution (Ecas4 anolyte). PLoS One. (2019) 14:e0222765. doi: 10.1371/journal.pone.0222765
25. Wei, M, Liu, H, Chen, J, Xu, C, Li, J, Xu, P, et al. Effects of aerosol pollution on PM2.5-associated bacteria in typical inland and coastal cities of northern China during the winter heating season. Environ Pollut. (2020) 262:114188. doi: 10.1016/j.envpol.2020.114188
26. Dakić, I, Morrison, D, Vuković, D, Savić, B, Shittu, A, Jezek, P, et al. Isolation and molecular characterization of Staphylococcus sciuri in the hospital environment. J Clin Microbiol. (2005) 43:2782–5. doi: 10.1128/JCM.43.6.2782-2785.2005
27. Shittu, A, Lin, J, Morrison, D, and Kolawole, D. Isolation and molecular characterization of multiresistant Staphylococcus sciuri and Staphylococcus haemolyticus associated with skin and soft-tissue infections. J Med Microbiol. (2004) 53:51–5. doi: 10.1099/jmm.0.05294-0
28. Kolawole, DO, and Shittu, AO. Unusual recovery of animal staphylococci from septic wounds of hospital patients in Ile-Ife, Nigeria. Lett Appl Microbiol. (1997) 24:87–90. doi: 10.1046/j.1472-765x.1997.00337.x
29. Kloos, WE. Natural populations of the genus Staphylococcus. Annu Rev Microbiol. (1980) 34:559–92. doi: 10.1146/annurev.mi.34.100180.003015
30. Frana, TS. Staphylococcosis In: JJ Zimmerman, LA Karriker, A Ramirez, KJ Schwartz, and GW Stevenson, editors. Diseases of swine. Oxford: Wiley-Blackwell (2012). 834–40.
31. Sasaki, Y, Yamanaka, M, Nara, K, Tanaka, S, Uema, M, Asai, T, et al. Isolation of ST398 methicillin-resistant Staphylococcus aureus from pigs at abattoirs in Tohoku region, Japan. J Vet Med Sci. (2020) 82:1400–3. doi: 10.1292/jvms.20-0184
32. Wendt, C, Dietze, B, Dietz, E, and Rüden, H. Survival of Acinetobacter baumannii on dry surfaces. J Clin Microbiol. (1997) 35:1394–7. doi: 10.1128/jcm.35.6.1394-1397.1997
33. Neely, AN, and Maley, MP. Survival of enterococci and staphylococci on hospital fabrics and plastic. J Clin Microbiol. (2000) 38:724–6. doi: 10.1128/JCM.38.2.724-726.2000
34. Hanczvikkel, A, and Tóth, Á. Quantitative study about the role of environmental conditions in the survival capability of multidrug-resistant bacteria. J Infect Public Health. (2018) 11:801–6. doi: 10.1016/j.jiph.2018.05.001
35. Katzenberger, RH, Rösel, A, and Vonberg, RP. Bacterial survival on inanimate surfaces: a field study. BMC Res Notes. (2021) 14:97. doi: 10.1186/s13104-021-05492-0
36. Makita, K, Goto, M, Ozawa, M, Kawanishi, M, Koike, R, Asai, T, et al. Multivariable analysis of the association between antimicrobial use and antimicrobial resistance in Escherichia coli isolated from apparently healthy pigs in Japan. Microb Drug Resist. (2016) 22:28–39. doi: 10.1089/mdr.2014.0311
Keywords: antimicrobial resistance, antimicrobial usage, staphylococci, aerosol, piggery
Citation: Kobayashi S, Tamamura-Andoh Y, Yamane I, Kusumoto M and Katsuda K (2023) The association between farm-level antimicrobial usage and resistance of Staphylococcus spp., as the major genus isolated from aerosol samples, in Japanese piggeries. Front. Vet. Sci. 10:1127819. doi: 10.3389/fvets.2023.1127819
Received: 20 December 2022; Accepted: 10 July 2023;
Published: 24 July 2023.
Edited by:
Eliana Guedes Stehling, University of São Paulo, Ribeirão Preto, BrazilReviewed by:
Timothy Frana, Boehringer Ingelheim, United StatesCopyright © 2023 Kobayashi, Tamamura-Andoh, Yamane, Kusumoto and Katsuda. This is an open-access article distributed under the terms of the Creative Commons Attribution License (CC BY). The use, distribution or reproduction in other forums is permitted, provided the original author(s) and the copyright owner(s) are credited and that the original publication in this journal is cited, in accordance with accepted academic practice. No use, distribution or reproduction is permitted which does not comply with these terms.
*Correspondence: Ken Katsuda, a2F0c3VkYUBhZmZyYy5nby5qcA==
Disclaimer: All claims expressed in this article are solely those of the authors and do not necessarily represent those of their affiliated organizations, or those of the publisher, the editors and the reviewers. Any product that may be evaluated in this article or claim that may be made by its manufacturer is not guaranteed or endorsed by the publisher.
Research integrity at Frontiers
Learn more about the work of our research integrity team to safeguard the quality of each article we publish.