- 1College of Animal Life Science, Kangwon National University, Chuncheon, Republic of Korea
- 2Hooin Ecobio Institute, Hongseong-gun, Chungcheongnam-do, Republic of Korea
In response to climate change, the use of digital livestock systems and probiotic mixtures as technological strategies to improve animal health and production is driving new innovations in the farm animal industry. However, there is little information available regarding the effects of digital livestock systems and probiotic mixtures (consisting of Bacillus subtillus, Streptomyces galilaeus, and Sphingobacteriaceae) on the growth performance of the growth-finishing swine. Thus, the objective of this study was to investigate the effects of digital livestock systems and probiotic mixtures on the immune function, cecal bacteria, short-chain fatty acids, nutrient digestibility, and growth performance of growth-finishing swine. A total of 64 crossbred male swine (Duroc × Landrace × Yorkshire, average body weight: 60.17 ± 1.25 kg) were randomly assigned to four treatment groups: CON (control group with a conventional livestock system without a probiotic mixture), CON0.4 (a conventional livestock system with a 0.4% probiotic mixture), DLSC (a digital livestock system without a probiotic mixture), and DLS0.4 (a digital livestock system with a 0.4% probiotic mixture). The swine were reared under standard environmental conditions until their average body weight reached 110 kg. The results indicated that the growth performance of the swine improved with an increase in nutrient digestibility and immune function via modulation of blood immune markers in the group with a digital livestock system compared to the CON group, although the growth performance of the swine was similar between the DLSC and CON0.4 groups. Moreover, the application of the digital livestock system and the probiotic mixture maintained higher levels of Lactobacillus and balanced short-chain fatty acid profiles compared to the CON group. These results suggest that a digital livestock system and a probiotic mixture can improve the growth performance of swine by enhancing their nutrient digestibility, improving their immune function, and maintaining balanced cecal bacteria and short-chain fatty acids. Therefore, this study provides insights into the application of digital livestock systems and probiotic mixtures as a climate change response strategy to improve swine production.
Introduction
Climate change-induced global warming can negatively impact animal behavior and welfare through heat stress and greenhouse gas (GHG) emissions, which can ultimately result in economic animal crises and food supply problems. Thus, preemptive responses are required. A digital livestock system has been developed to resolve the farming animal crisis due to climate change and to maintain sustainable livestock production (1, 2). The development of a digital livestock system for precision livestock farming (PLF) has led to the implementation of remote monitoring systems using cameras, microphones, and accelerometer sensors. Rapid progress has been made to improve animal welfare and production, and various systems managed by high-tech equipment have been introduced to livestock farming (1–3). Digital livestock systems, including feeding, weighing, and sorting systems, wearable sensors, and climate control systems, have been implemented in swine farming in an effort to improve swine production, animal behavior and welfare, sound detection, and 3D camera application information (4, 5).
The FAO has predicted that the world's population will reach 9.2 billion by 2050, resulting in a 50–60% increase in food consumption compared to current levels. Global animal food production will increase by 2/3 from 334 million tons in 2015 to 498 million tons in 2050 (6, 7). By 2050, the consumption of meat, eggs, and dairy products is predicted to increase by 73, 64, and 58%, respectively, in comparison with the present situation. Thus, sustainable livestock production is necessary to secure food supplies. The use of precision livestock farming (PLF) as a digital livestock system is being increasingly adopted to improve the swine industry. This climate-smart livestock system has recently been developed in response to the rapid increase in pork meat consumption (8, 9).
In the digital era, an intelligent PLF and ICT (information and communication technology)-based climate-smart livestock system (hereinafter referred to as a digital livestock system) is being commercialized as future megatrend strategies using big data converged with the 4th industrial revolution's ICT, Internet of Things (IoT), wireless biosensors, mobile phones, artificial intelligence (AI), automatic systems, and drone technology (1, 2, 10–12). A digital livestock system is a new technology developed for the animal livestock industry that can improve humans' and animals' lives through ICT convergence, where the demand for information across the supply and food chains is the source of power for the development and expansion of digital agriculture (13–15). A digital livestock system, such as PLF, is a groundbreaking innovation that can play a significant role in addressing climate change issues and improving feeding management, ventilation, and animal welfare by enhancing the quality of livestock production and animal food. It allows for young livestock populations to be reared in rural communities, thus promoting sustainable livestock farming. It can control, adjust, and monitor the ventilation, heating, and cooling of livestock farms through a central PC. It can also remotely control feeding management using a smartphone or tablet PC. Error messages are sent via SMS or email. The system is integrated with an air cleaner (14–17). In particular, it can remove specks of dust, ammonia, and odors that are generated from livestock farms during the summer to provide a comfortable climate for livestock. In addition, it can improve livestock production while reducing the environmental impact of the livestock industry, saving energy, and improving animal welfare. The use of a digital livestock system as a technological strategy to improve animal health and production can lead to new innovations in the economic animal industry. It is known to improve economic animals' welfare and growth performance by automating feeding and environmental management systems. However, little is known about its effect on the growth performance of growth-finishing swine (1, 2, 18–20).
Probiotics are living organisms that are part of the normal intestinal microflora of animals. Maintaining optimal levels of intestinal bacteria in swine through the use of probiotics can improve animal production and boost immune function (21–23). Previous studies have reported that a probiotic mixture (Bacillus subtillus, Streptomyces galilaeus, and Sphingobacteriaceae) can improve growth performance and egg and chicken meat quality by enhancing caecal microbial balance and immune function. The probiotic mixture can also reduce the odor in poultry farms for laying hens and broilers exposed to heat stress (22, 23). However, little is known about the effect of using digital livestock systems and probiotic mixtures on the growth performance of growth-finishing swine (1, 2, 16, 18–20).
This study was conducted to compare the effects of a conventional livestock system, a digital livestock system, and both livestock systems with a probiotic mixture on growth performance, immune function, cecal bacteria, cecal short-chain fatty acid, and nutrient digestibility in growth-finishing swine.
Materials and methods
Ethical approval
This study was conducted according to the guidelines for scientific and ethical procedures provided in the European Experimental Animal Handling License (SCT-w94058) and European Union Council Directive 2008/120/EC. It was approved by the Institutional Animal Care and Use Committee of Kangwon National University, Republic of Korea (No. KNU-20197).
Animals and experimental design
The animal experiment was conducted in swine research farms of the Hooin Ecobio Institute (Hongseong, Chungcheongnamdo), Republic of Korea. A total of 64 crossbred male swine (Duroc × Landrace × Yorkshire; average body weight: 60.17 ± 1.25 kg) were reared until their body weights reached ~110 kg. Regarding the blocking factor for the experiment, the livestock system and probiotic mixtures were treatment variables. The swine were assigned to one of the following four groups (16 replicate pens per group) based on a randomized complete block design: CON (control group with a conventional livestock system without a probiotic mixture), CON0.4 (a conventional livestock system with a 0.4% probiotic mixture), DLSC (digital livestock system without a probiotic mixture), and DLS0.4 (a digital livestock system with a 0.4% probiotic mixture). Mixed probiotics were prepared by mixing 2% of Bacillus subtillus, Streptomyces galilaeus, and Sphingobacteriaceae (isolated from earthworm cast) with 98% of the earthworm cast. These products were provided by HooinEcobio Co., Ltd. (Korean Patent No. 0092670). Each strain contained at least 3.5 × 108 colony-forming units (CFU/g of product) (22, 23). The level of probiotic mixture added was determined based on preliminary results, demonstrating plateau levels without further increasing growth performance under standard environmental conditions for swine research farms.
Digital livestock system
The digital livestock system used in this study incorporated ICT convergence, big data, and mobile phones. It was equipped with various features such as automatic feeding and drinking dispensers, radio frequency identification, temperature and humidity sensors, an odor removal system, cooling pads, an air conditioner, livestock farm monitoring through CCTV, feed bins, and a mobile imaging system for live weight measurement (1, 2, 16).
Diets and feeding management
This study used experimental basal diets formulated based on the nutritional requirements for growth-finishing swine recommended by the NRC (24). The probiotic mixture was added to the yellow corn grain. Ingredients and chemical compositions of the basal diet are shown in Table 1. The swine were reared under the same standard environmental conditions (a breeding area of 0.45–0.8 m2 per animal, a temperature of 25°C, and a relative humidity of 50–60%) for both livestock systems. Through an installed ventilation controller, minimum ventilation and maximum ventilation were adjusted to 20–30% and 60–70%, respectively, to minimize changes in the internal environment in line with changes in the external environment. All animals were provided free access to water and feed. After feeding basal diets for 45 days, a total of 24 animals (six replicates per group, four groups) were moved to metabolic cages to measure nutrient digestibility. The remaining 40 animals (10 replicates per group, four groups) were kept to investigate growth performance.
Growth performance and spleen weight
The growth performance was measured based on average daily weight gain (ADG), average daily feed intake (ADF), and the feed conversion ratio (FCR) (weight gain/feed intake) at the beginning and end of animal experiments, respectively. After finishing the animal feeding experiment, the swine were euthanized using electrical stunning and exsanguination. The weights of their spleens (immune organ; as % BW, the relative weight to body weight) were then measured.
Nutrient digestibility
The digestibility of nutrients was measured for six replicates per group in four groups. The animals were moved into metabolic cages for fecal collection. They were fed different diets mixed with 0.30% chromic oxide as an indigestible marker to measure their nutrient digestibility. The animals had an adaptation period of 1 week. Total feces from each metabolic cage were collected for 3 days. After collection, fecal samples were oven-dried at 70°C and milled through a 40-mesh sieve prior to chemical analyses. Nutrient compositions and chromic oxide in diets and fecal samples were analyzed following the AOAC testing methods (25). Nutrient digestibility was calculated as a percentage: (the amount of marker in the diet/amount of marker in the feces) × (amount of nutrient in the feces/amount of nutrient in the diet) (26).
Immune markers
To evaluate the immune markers of animals at the end of the experiment, we collected 10 mL of blood from the jugular vein of each animal and placed it into a clot activator vacuum tube (Becton Dickinson Vacutainer Systems, Franklin Lakes, NJ, USA). Immunoglobulin G (IgG) concentration in serum was measured using a swine IgG ELISA Kit (E101-104, Bethyl Laboratories, USA). The serum concentration of cortisol, a stress hormone, was quantified using a swine cortisol ELISA Kit (EKC31445-BM, BioCat GmbH, Germany) according to the manufacturer's protocol. Three blood smears per animal were taken immediately after blood collection, and the slides were stained with the May-Grunwald-Giemsa stain after air drying. Heterophils (H) and lymphocytes (L) were differentiated through light microscopy. After 100 leukocytes per slide and 200 cells per animal were counted, the H/L ratio was then calculated (27).
Cecal bacteria counts
Lactobacillus, E. coli, coliform bacteria, and total aerobic bacteria from cecal contents were cultured on MRS agar (Difco), violet red bile agar with MUG (Difco), and nutrient agar (Difco), respectively. After aerobic culturing at 37°C for 24 h, the count of colonies for each strain was determined using a colony counter. Data were presented as log10 colony forming units (CFU) per gram of cecal content (28).
Cecal short-chain fatty acids
Cecum samples were collected from each swine to analyze short-chain fatty acids (SCFAs) and bacteria counts. The cecum was quickly frozen in liquid nitrogen and then stored at −80°C for further analysis. Cecal SCFAs were measured using a gas chromatographic system (model GC-15A, Shimadzu Corp., Kyoto, Japan) equipped with a glass column filled with 10% SP-1000/1% H3PO4 (180 cm × 4 mm; Supelco, Inc., Bellefonte, PA, US) and attached to a flame ionization detector. After adding 25% H3PO4 solution to the supernatant, the mixture was homogenized, maintained on ice for more than 30 min, and then centrifuged at 3,000 rpm for 10 min at 4°C (29).
Statistical analysis
All obtained data were checked for normal distribution using the Shapiro–Wilk normality test prior to analysis. They were analyzed using the generalized linear model (GLM) procedure of SAS (SAS 9.4 Institute Inc., Cary, NC, USA) as a randomized complete block design with a repeat pen serving as the experimental unit. The effects of the digital livestock system, conventional livestock system, and probiotic mixtures were analyzed with an individual animal taken as an experimental unit. A linear effect analysis was performed using polynomial orthogonal contrasts. Differences among treatments were separated using Tukey's multiple-range test. Probability values < 0.05 were considered significant (P < 0.05).
Results
Growth performance
The results revealed that the applied digital livestock system greatly improved the growth performance of swine compared to the conventional livestock system without a probiotic mixture. However, although the DLSC and CON0.4 groups showed the same results (Table 2), ADG was significantly increased in the order of the DLS0.4, DLSC = CON0.4, and CON groups (P < 0.05). ADF was significantly higher in the order of the CON, CON0.4 = DLSC, and DLS0.4 groups (P < 0.05). FCR was significantly lower in the order of the DLS0.4, DLSC = CON0.4, and CON groups due to increased ADF (P < 0.05).
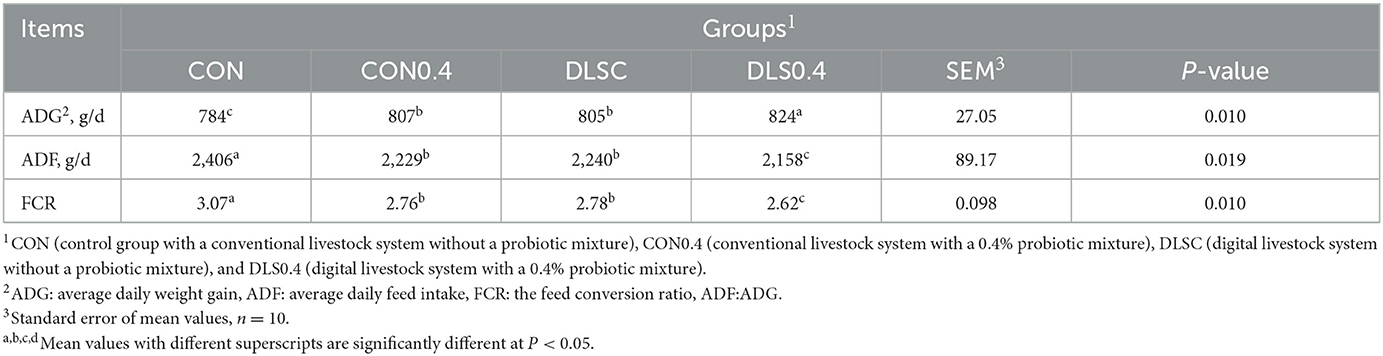
Table 2. Growth performance of growth-finishing swine reared under digital livestock system (60–110 kg body weight).
Nutrient digestibility
The use of the digital livestock system significantly increased nutrient digestibility compared to the conventional livestock system without a probiotic mixture, although the DLSC and CON0.4 groups showed a similar trend (Table 3). Regarding the digestibility of dry matter, crude protein was significantly increased in the order of the DLS0.4, DLSC = CON0.4, and CON groups. The ether extract was higher in the order of the DLS0.4, DLSC, CON0.4, and CON groups, and crude ash was significantly increased in the order of the DLS0.4, DLSC = CON0.4, and CON groups (P < 0.05).
Immune markers
The digital livestock system greatly improved the immune function of animals by modulating serum immune markers compared to the conventional livestock system without a probiotic mixture, although the DLSC and CON0.4 groups showed a similar trend (Table 4). Serum immune markers, IgG, cortisol, lymphocytes (L), and the H/L ratio were significantly increased in the order of the CON, CON0.4 = DLSC, and DLS0.4 groups (P < 0.05). Heterophil (H) was significantly higher in order of the CON, DLSC, CON0.4, and DLS0.4 groups (P < 0.05). The weights of the spleen (ranging from 0.11–0.13 % BW) as an immune organ did not differ between the groups.
Short-chain fatty acid
The digital livestock system maintained balanced short-chain fatty acid (SCFA) profiles compared to the conventional livestock system without a probiotic mixture, although the DLSC and CON0.4 groups showed a similar trend (Table 5). In comparison to the SCFAs of the CON and DLSC groups, the SCFAs of the DLS0.4 and CON0.4 groups exhibited a significant increase in acetic acid and propionic acid but not in butyric acid, isobutyric acid, valeric acid, or isovaleric acid. Cecal total SCFA was significantly increased in the order of the DLS0.4, CON0.4, and DLSC = CON groups (P < 0.05). Acetic acid and propionic acid were significantly increased in the order of the DLS0.4 = CON0.4, DLSC, and CON groups (P < 0.05). Butyric acid was significantly higher in the CON group than in the other groups (P < 0.05). Isobutyric acid was significantly increased in the order of the CON, DLSC, and DLS0.4 = CON0.4 groups (P < 0.05). Valeric acid and isovaleric acid were significantly higher in the CON group than in other groups (P < 0.05).
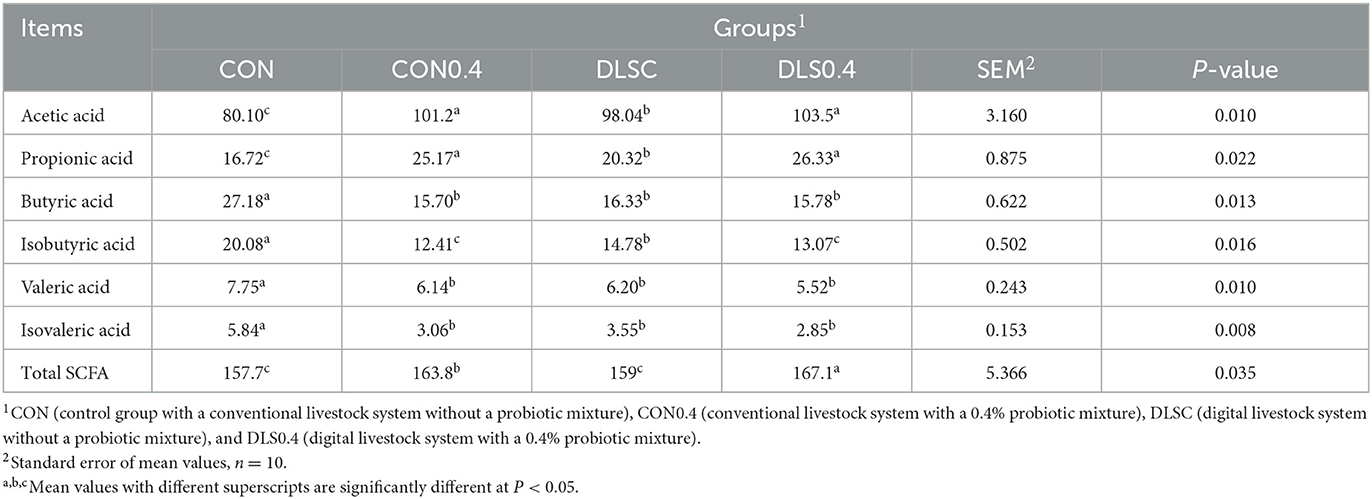
Table 5. Cecal short-chain fatty acid in growth-finishing swine reared under a digital livestock system (SCFA, μmol/g of cecal content).
Cecal bacteria
The digital livestock system increased Lactobacillus but decreased E. coli counts compared to the conventional livestock system without a probiotic mixture (Table 6). Cecal Lactobacillus counts were significantly lower in the CON group than in other groups (P < 0.05), but they showed no significant differences among the DLSC, DLSC0.4, and CON0.4 groups. E. coli counts were significantly increased in the order of the CON, DLSC, CON0.4, and DLS0.4 groups (P < 0.05). Coliform bacteria counts were significantly increased in the order of the CON, DLSC, CON0.4, and DLS0.4 groups (P < 0.05), although they were not significantly different between the CON0.4 and DLS0.4 groups. Total aerobic bacteria counts were significantly increased in the order of the CON, DLSC = CON0.4, and DLS0.4 groups (P < 0.05), although they were not significantly different between the DLSC and CON0.4 groups (P < 0.05).
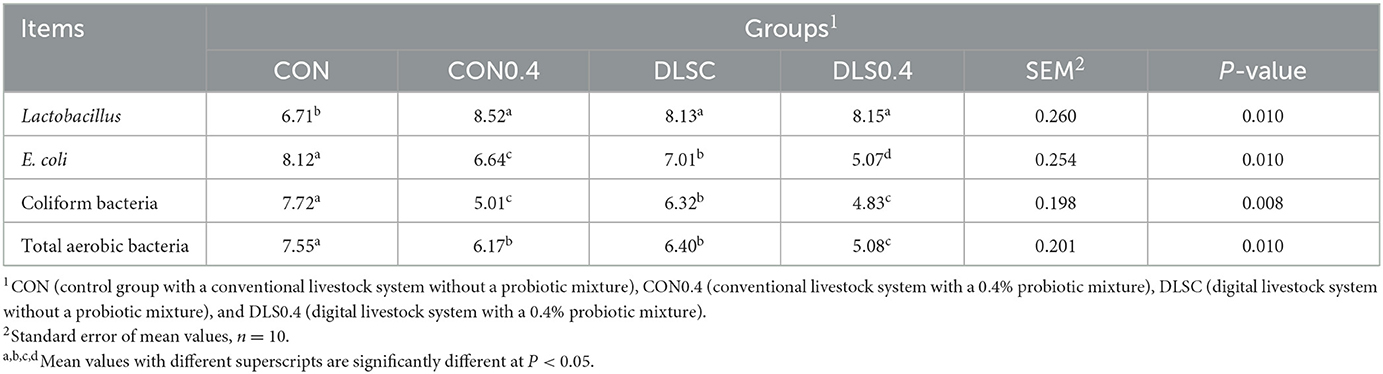
Table 6. Cecal bacteria counts in growth-finishing swine reared under a digital livestock system (log cfu/g of fresh cecal content).
Discussion
Our results revealed a new fact: the growth performance of swine could be greatly improved by using a digital livestock system compared to a conventional livestock system without a probiotic mixture. Both the DLSC and CON0.4 groups showed a similar trend. Our results also showed that the digital livestock system can improve the growth performance of swine by improving their welfare, reducing stress, and promoting their overall health through the convenience provided by automatic feeding and environmental management, which streamlines animal care. The digital livestock system can also modulate immune markers, increase nutrient digestibility, and balance cecal bacteria and SCFA. However, it is important to note that butyrate SFCA was significantly reduced in the CON0.4, DLSC, and DLS0.4 groups compared to the CON group. This reduction may have negative implications, as butyrate is a crucial SCFA involved in many physiological pathways. Similarly, IgG was significantly decreased in the CON0.4, DLSC, and DLS0.4 groups, and it may be seen as a negative immune response in these groups (1, 5, 17, 19). The fact that DLS0.4 improved the growth performance of swine could be considered a combined effect of the digital livestock system and the probiotic mixture in SCFA (1, 2, 16) (Table 2). CON0.4 and DLS0.4 groups consumed the same level of probiotics, with DLSC and CON0.4 groups showing the same results, suggesting that swine production could be significantly improved by the digital livestock system with the probiotic mixture. However, few such studies have been reported. The digestibility of dry matter, crude protein, crude fat, and crude fiber were higher in the digital livestock system than in the conventional livestock system without a probiotic mixture, although the DLSC and CON0.4 groups showed similar trends, demonstrating improved growth performance in the DLSC group. The digital livestock system resulted in better nutrient digestibility because animal stress was alleviated due to an automatic environment and feeding management control in the digital swine production system, leading to improved nutritional and metabolic capability (1, 16) (Table 3). Animal stresses occur due to various factors, including environmental conditions. These stresses can degrade an animal's nutritional and metabolic capabilities, resulting in decreased digestibility, reduced feed intake, and impaired growth performance (18, 27–29). This supports the results of this study. The fact that the DLS0.4 group showed the highest nutrient digestibility might be due to the possible interaction between the probiotic mixture and the digital livestock system, which ultimately contributed to the improvement of swine production. The supply of probiotics to swine is known to improve nutrient digestibility and increase animal growth performance and production (22, 30, 31).
In the hematological evaluation of animal stress, leukocyte counts (leukocyte profiles) from blood smears, that is, the H/L ratio as an immunological marker, are important. Various stressors, including animal environment and management, can increase blood IgG, H/L ratio, and cortisol concentration as immune markers, enabling it to cope with stress (27, 29, 32). Although spleen weight was not significantly different between treatment groups, the modulation of immune markers in the DLS0.4 group animals could be due to the combined effect of the probiotic mixture and digital livestock systems. This system can promote immune function by increasing nutrient digestibility through feeding intake stimulation and balancing cecal bacteria with the help of probiotics.
In the field of poultry farming, implementing a digital livestock system has been shown to improve animal growth performance and animal welfare while relieving animal stress by modulating immune markers (1, 16). Based on this result, modulated immune markers in the DLS0.4 group might be due to a combined effect of the probiotic mixture and the digital livestock system, thus improving the growth performance of the swine. The growth performance of animals could be improved by modulating immune markers by DLS compared to those of the CON group, although the spleen weight was similar between treatment groups (32, 33) (Table 4). When animals are exposed to poor welfare conditions, poor feeding management, and environmental stress, their blood IgG, H/L ratio, and cortisol concentration rapidly increase as a response. Therefore, proper maintenance of immune markers is crucial to ensure optimal immune function (16, 22, 32). One of the most important roles of the microorganisms found in probiotics is to stimulate immune function in host animals. Various types of probiotics, including normal microorganisms in the digestive tract, are known to stimulate the immune system in animals (21, 22, 30, 34).
It was confirmed that the digital livestock system could maintain balanced cecal SCFA profiles compared to a conventional livestock system without a probiotic mixture, although similar results were observed between the CON0.4 and DLS0.4 groups (Table 5). Probiotics have been known to increase swine production through competition with other intestinal bacteria for nutrients, production of antibacterial substances, enhancement of immune markers, SCFA production, and nutrient digestibility (22, 30, 31). For this reason, it can be seen that the SCFA results between the CON0.4 and DLS0.4 groups showed similar trends.
The obtained data indicated that cecal total short-chain fatty acids, acetic acid, and propionic acid were increased in the DLS0.4 and CON0.4 groups compared to those in the CON and DLSC groups, whereas butyric acid, isobutyric acid, valeric acid, and isovaleric acid showed a higher tendency in the CON group than in other groups, eventually stimulating immune cell development and maintaining balanced levels of immune markers. The results for SCFA of both probiotic mixture groups presented in Table 5 will ultimately contribute to improved swine production through improved immune function and gut health, a reduction in stress, and an improvement in animal welfare (1, 28, 29, 35) (Table 5). Probiotics are known to improve growth performance and immune function by modulating the level of SCFA in the cecum of animals (1, 36–38). However, the combined effect of the digital livestock system and probiotic mixture has not been reported until now.
Our results showed that the digital livestock system could lead to higher cecal Lactobacillus counts compared to the conventional livestock system without a probiotic mixture because the CON0.4, DLSC, and DLS0.4 groups displayed the same results. Lactobacillus, a good cecal bacteria, can improve the growth performance of animals and maintain the gut environment by balancing cecal bacteria and promoting the stability of indigenous microbes against animal stressors. The digital livestock system can help maintain balanced cecal bacteria by increasing Lactobacillus and reducing E. coli in the digestive tract of animals, thereby reducing stress and improving the animal's nutritional and metabolic capabilities (1, 16) (Table 6). E. coli is known to form a colony of harmful bacteria in intestinal villous cells, generating toxins that can trigger a swine's digestive tract to secrete intestinal fluids, resulting in diarrhea (16, 21, 31). In an environment where animals are exposed to various stressors, unexpected disorders or illnesses will occur if the stability of the intestinal microbial ecosystem is threatened (1, 21, 29, 31). For this reason, in this study, the growth performance of the swine was improved with the digital livestock system.
Conclusion
In conclusion, our study demonstrated that the use of a digital livestock system can improve the growth performance of swine compared to both a conventional livestock system without a probiotic mixture and a conventional system with a probiotic mixture. This study's findings provide new insights into the action mechanism through which the digital livestock system and probiotic mixture can improve the growth performance of swine by reducing stress, increasing nutrient digestibility, promoting a balanced cecal bacterial community and SCFA production, and modulating immune function. The implementation of a digital livestock system can improve animal welfare, animal feeding, and environmental management through automation, wireless mobile phones, and sensing application technology that incorporates ICT convergence. Our results also showed that the growth performance of swine in a digital livestock system without a probiotic mixture and a conventional livestock system with a probiotic mixture were the same. This might be due to the digital livestock system's ability to increase nutrient digestibility, modulate immune function, and balance cecal bacteria and short-chain fatty acid profiles. Immune markers such as IgG, cortisol, and the heterophils (H)/lymphocytes (L) were higher in the digital livestock system than in the conventional livestock system without a probiotic mixture, indicating that the digital livestock system could modulate immune function in swine. Moreover, the digital livestock system modulated the balanced cecal SCFA profiles and bacteria counts, such as Lactobacillus and E. coli, compared to conventional livestock systems without probiotics. These findings suggest that the digital livestock system could play an important role in maintaining the immune function of swine. The study's results provide valuable insights into the application of a digital livestock system and probiotic mixtures in farm animals as a climate change response strategy, especially in the swine industry.
Data availability statement
The original contributions presented in the study are included in the article/supplementary material, further inquiries can be directed to the corresponding author.
Ethics statement
The animal study was reviewed and approved by Institutional Animal Care and Use Committee of Kangwon National University, Republic of Korea (No: KNU-20197). Written informed consent was obtained from the owners for the participation of their animals in this study.
Author contributions
S-OP designed and conducted the experiments, wrote the original draft, and revised the manuscript. K-HS helped with the animal experiments. S-OP and K-HS analyzed the data. All authors have read and approved the final version of the manuscript.
Funding
This study was funded by the Basic Research Project of the National Research Foundation of the Republic of Korea (NRF) grant funded by the government of the Republic of Korea (Ministry of Education), 2019 (No. 018RIDIA3B07047548).
Acknowledgments
The authors thank the NRF Republic of Korea for research funding and the Institute of Animal Life Sciences at Kangwon National University for the experimental design and excellent cooperation.
Conflict of interest
The authors declare that the research was conducted in the absence of any commercial or financial relationships that could be construed as a potential conflict of interest.
Publisher's note
All claims expressed in this article are solely those of the authors and do not necessarily represent those of their affiliated organizations, or those of the publisher, the editors and the reviewers. Any product that may be evaluated in this article, or claim that may be made by its manufacturer, is not guaranteed or endorsed by the publisher.
References
1. Park SO. Application strategy for sustainable livestock production with farm animal algorithms in response to climate change up to 2050: a review. Czech J Anim Sci. (2022) 67:425–41. doi: 10.17221/172/2022-CJAS
2. Baker D, Jackson EL, Cook S. Perspectives of digital agriculture in diverse types of livestock supply chain systems. Making sense of uses and benefits. Front Vet Sci. (2022) 9:992882. doi: 10.3389/fvets.2022.992882
3. Morrone S, Dimauro C, Gambella F, Cappai MG. Industry 4.0 and precision livestock farming (PLF): an up to date overview across animal productions. Sensors. (2022) 22:4319–44. doi: 10.3390/s22124319
4. Arulmozhi E, Bhujel A, Moon BE, Kim H. The application of cameras in precision pig farming: an overview for swine-keeping professionals. Animals. (2021) 11:2343–66. doi: 10.3390/ani11082343
5. Halachmi I, Guarino M, Bewley J, Pastell M. Smart animal agriculture: application of real-time sensors to improve animal well-being and production. Ann Rev Anim Biosci. (2019) 7:403–25. doi: 10.1146/annurev-animal-020518-114851
6. FAO. Food and Agriculture Organization of the United Nations. Information and Communication Technology (ICT) in Agriculture. A Report to the G20 Agricultural Deputies. Rome: FAO (2017).
7. FAO. World Agriculture: Towards 2030/2050. Global Perspectivestudies Unit. Interim Report. Rome: FAO (2006).
8. Delgado C. Rising consumption of meat and milk in developing countries has created a new food revolution. J Nutr. (2003) 133:3907–10S. doi: 10.1093/jn/133.11.3907S
9. Tilman D, Cassman KG, Matson PA, Naylor R, Polasky S. Agricultural sustainability and intensive production practices. Nature. (2002) 418:671–7. doi: 10.1038/nature01014
10. Wolfert S, Ge L, Verdouw C, Boogaardt MJ. Big data in smart farming–A review. Agric Syst. (2017) 153:69–80. doi: 10.1016/j.agsy.2017.01.023
11. Astill J, Dara RA, Fraser EDG, Roberts B, Sharif S. Smart poultry management: smart snsors, big data, and the internet of things. Comput Electron Agric. (2020) 170:105291–402. doi: 10.1016/j.compag.2020.105291
12. Germani L, Mecarelli V, Baruffa G, Rugini L, Frescura F. An IoT architecture for continuous livestock monitoring using LoRa LPWAN. Electronics. (2019) 8:1435–63. doi: 10.3390/electronics8121435
13. Dawkins MS. Does smart farming improve or damage animal welfare? Technology and what animals want. Front Anim Sci. (2021) 2:736536–5545. doi: 10.3389/fanim.2021.736536
14. Neethirajan S. The role of sensors, big data and machine learning in modern animal farming. Sensing Biosensing Res. (2020) 29:100367–75. doi: 10.1016/j.sbsr.2020.100367
15. Xu X, Liang W, Xu Z. Remote monitoring cost minimization for an unreliable sensor network with guaranteed network throughput. Inf Process Agric. (2014) 1:83–94. doi: 10.1016/j.inpa.2014.10.001
16. Zammit VA, Park SO. Effect of smart poultry on growth performance, blood biochemistry parameters and cecal fermentation indices of broiler chickens. Anim Nutr Feed Technol. (2020) 20:419–32. doi: 10.5958/0974-181X.2020.00037.2
17. Groher T, Heitkamper K, Umstaetter C. Digital technology adoption in livestock production with a special focus on ruminant farming. Animals. (2020) 14:2404–13. doi: 10.1017/S1751731120001391
18. Dopelt K, Radon P, Davidovitch N. Environmental effects of the livestock industry: the relationship between knowledge, attitudes, and behavior among students in Israel. Int J Environ Res Public Health. (2019) 16:1359–75. doi: 10.3390/ijerph16081359
19. Hoste R, Suh H, Kortstee H. Smart farming in pig production and greenhouse horticulture; an inventory in the Netherlands. Wageningen University & Research Report 2017-097. Wageningen, Netherlands. (2017). p. 1–38.
20. Mohamed ES, Belal AA, Abd-Elmabod SK, El-Shirbeny MA, Gad A, Zahran MB. Smart farming for improving agricultural management. Egypt J Remote Sens Space Sci. (2021) 24:971–091. doi: 10.1016/j.ejrs.2021.08.007
21. Giang HH, Viet TQ, Ogle B, Lindberg JE. Effects of supplementation of probiotics on the performance, nutrient digestibility and faecal microflora in growing-finishing pigs. Asian Australas J Anim Sci. (2011) 24:655–61. doi: 10.5713/ajas.2011.10238
22. Song YH, Goh YG, Hwan KH, Park BS. Effects of dietary probiotic mixture on growth performance, caecal microorganism and immune response in broiler chickens under heat stress. J Korean Appl Sci Technol. (2018) 35:807–15.
23. Shin JS, Um KH, Park BS. Effect of a probiotic mixture on egg quality and egg production in laying hens. J Korean Appl Sci Technol. (2019) 36:748–57.
26. Ouweltjes W, Verschuren LMG, Pijlman J, Bergsma R, Schokker D, Knol EF, et al. The repeatability of individual nutrient digestibility in pigs. Livestock Sci. (2018) 207:63–7. doi: 10.1016/j.livsci.2017.11.013
27. Davis AK, Maney DL, Maerz JC. Review: the use of leukocyte profiles to measure stress in vertebrates. A review for ecologists. Funct Ecol. (2008) 22:760–72. doi: 10.1111/j.1365-2435.2008.01467.x
28. Park SO, Zammit VA. Effect of feed restriction with betaine and ascorbic acid supplementation on caecal bacteria, short chain fatty acid, blood biomarker, duodenal morphology and growth performance of meat ducks under heat stress. Eur Poult Sci. (2019) 83:1–13. doi: 10.1399/eps.2019.267
29. Park BS, Park SO. Effects of feeding time with betaine diet on growth performance, blood markers, and short chain fatty acids in meat ducks exposed to heat stress. Livestock Sci. (2017) 199:31–6. doi: 10.1016/j.livsci.2017.03.003
30. Salim HM, Kang HK, Akter N, Kim DW, Kim JH, Kim MJ. Supplementation of direct fed microbials as an alternative to antibiotic on growth performance, immune responses, cecal microbial population, and ileal morphology of broiler chickens. Poult Sci. (2013) 92:2084–90. doi: 10.3382/ps.2012-02947
31. Yu Y, Li Q, Zeng X, Xu Y, Jin K, Liu J, et al. Effects of probiotics on the growth performance, antioxidant functions, immune responses, and caecal microbiota of broilers challenged by lipopolysaccharide. Front Vet Sci. (2022) 9:846649–84661. doi: 10.3389/fvets.2022.846649
32. Lentfer TL, Pendl H, Gebhardt-Henrich SG, Fröhlich EK, Von Borell E. H/L ratio as a measurement of stress in laying hens-methodology and reliability. Br Poult Sci. (2015) 56:157–63. doi: 10.1080/00071668.2015.1008993
33. Scanes CG. Biology of stress in poultry with emphasis on glucocorticoids and the heterophil to lymphocyte ratio. Poult. Sci. (2016) 95:2208–15. doi: 10.3382/ps/pew137
34. Barba-Vidala E, Martín-Orúea S, Castillejos L. Practical aspects of the use of probiotics in pig production: a review. Livestock Sci. (2019) 223:84–96. doi: 10.1016/j.livsci.2019.02.017
35. Liu L, Li Q, Yang Y, Guo A. Biological function of short-chain fatty acids and its regulation on intestinal health of poultry. Front Vet Sci. (2021) 8:736739. doi: 10.3389/fvets.2021.736739
36. Andretta I, Remus A, Garcia-Launay F, Hauschild L, Kipper M. Editorial: strategies for mitigating the environmental impacts of pig and poultry production. Front Vet Sci. (2022) 9:892340. doi: 10.3389/fvets.2022.892340
37. Zhong X, Zhang Z, Wang S, Cao L, Zhou L, Sun A. Microbial-driven butyrate regulates jejunal homeostasis in piglets during the weaning stage. Front Microbiol. (2019) 9:3335. doi: 10.3389/fmicb.2018.03335
Keywords: immune marker, growth performance, digital livestock system, probiotics, swine
Citation: Park S-O and Seo K-H (2023) Digital livestock systems and probiotic mixtures can improve the growth performance of swine by enhancing immune function, cecal bacteria, short-chain fatty acid, and nutrient digestibility. Front. Vet. Sci. 10:1126064. doi: 10.3389/fvets.2023.1126064
Received: 20 December 2022; Accepted: 23 February 2023;
Published: 24 March 2023.
Edited by:
Guillermo Tellez-Isaias, University of Arkansas, United StatesReviewed by:
Jaime Andres Angel Isaza, National University of Colombia, ColombiaSantiago Uribe-Diaz, University of Arkansas, United States
Copyright © 2023 Park and Seo. This is an open-access article distributed under the terms of the Creative Commons Attribution License (CC BY). The use, distribution or reproduction in other forums is permitted, provided the original author(s) and the copyright owner(s) are credited and that the original publication in this journal is cited, in accordance with accepted academic practice. No use, distribution or reproduction is permitted which does not comply with these terms.
*Correspondence: Sang-O Park, YnNwYXJrQGthbmd3b24uYWMua3I=