- Animal Biosciences and Biotechnology Laboratory, United States Department of Agriculture, Agricultural Research Service, Beltsville, MD, United States
The poultry industry has improved genetics, nutrition, and management practices, resulting in fast-growing chickens; however, disturbances during embryonic development may affect the entire production cycle and cause irreversible losses to broiler chicken producers. The most crucial time in the chicks' development appears to be the perinatal period, which encompasses the last few days of pre-hatch and the first few days of post-hatch. During this critical period, intestinal development occurs rapidly, and the chicks undergo a metabolic and physiological shift from the utilization of egg nutrients to exogenous feed. However, the nutrient reserve of the egg yolk may not be enough to sustain the late stage of embryonic development and provide energy for the hatching process. In addition, modern hatchery practices cause a delay in access to feed immediately post-hatch, and this can potentially affect the intestinal microbiome, health, development, and growth of the chickens. Development of the in ovo technology allowing for the delivery of bioactive substances into chicken embryos during their development represents a way to accommodate the perinatal period, late embryo development, and post-hatch growth. Many bioactive substances have been delivered through the in ovo technology, including carbohydrates, amino acids, hormones, prebiotics, probiotics and synbiotics, antibodies, immunostimulants, minerals, and microorganisms with a variety of physiological effects. In this review, we focused on the physiological effects of the in ovo delivery of these substances, including their effects on embryo development, gastrointestinal tract function and health, nutrient digestion, immune system development and function, bone development, overall growth performance, muscle development and meat quality, gastrointestinal tract microbiota development, heat stress response, pathogens exclusion, and birds metabolism, as well as transcriptome and proteome. We believe that this method is widely underestimated and underused by the poultry industry.
Introduction
Over the years, the poultry industry has improved genetics, nutrition, and management practices, resulting in fast-growing chickens that reach market weight faster than broilers raised decades ago (1). However, disturbances during embryonic development may affect the entire production cycle and cause irreversible losses to broiler chicken producers. Embryonic development accounts for more than 33% of the entire life of commercial broilers (2). Moreover, the most crucial time in the chicks' development appears to be the perinatal period, which encompasses the last few days of pre-hatch and the first few days of post-hatch (3). During this critical period, intestinal development occurs rapidly (4), and the chicks undergo metabolic and physiological shifts from the utilization of egg nutrients to exogenous feed (4, 5). The nutrients in the egg yolk may not be enough to sustain the late stage of embryonic development and provide energy for the hatching process. In addition, modern hatchery practices cause a delay in access to feed immediately post-hatch, and this can potentially affect the intestinal microbiome, health, development, and growth of the chickens (6–15). One of the technological advances that could help remedy the issue is in ovo delivery of bioactive substances. The biggest benefit of in ovo administration of bioactive substances during embryonic development is their long-term effects on avian physiology (16).
The in ovo methodology was developed in the early 1980s as a means of delivering Marek's disease vaccine (17). The development of this technology allows for the expansion of research beyond vaccine delivery. However, many issues have been indicated with the methodology, such as the lack of standardization/optimization of the method for delivery of various bioactive substances, including the age of the embryo, volume of the injection, site of the injection, or concentration of the bioactive substances (3). Automated delivery of bioactive substances has been developed over the years after the initial introduction of the method. The patent of Uni and Ferket (18) is the most widely used method for delivering in ovo injections. There are five locations for in ovo delivery in chickens, namely, the air cell, embryo, yolk sac, allantoic membrane, and amniotic fluid (3). The in ovo technology was adapted over time for in ovo feeding of probiotics or prebiotics on embryonic day (e)18 or synbiotics delivery on e12 (18–20). In general, in ovo delivery on e12 is referred to as in ovo stimulation, whereas delivery on e17–18 is known as in ovo feeding (21). According to the patent of Uni and Ferket (18), the optimal time and site for in ovo delivery for feeding purposes is late-term embryo and the amniotic fluid, respectively (20). When the embryo consumes the amniotic fluid, intestinal tissues are exposed to the injected substances (18). The in ovo procedure on e12 involves delivery of bioactive compounds into the egg's air cell. Prebiotics delivered to the air cell are transferred through the vascular system in the chorioallantoic membrane into the intestine, while probiotics in the air cell are available for the chicken embryo at the time of hatch (19). There is no consensus about the volume of injections. The volume used previously ranges from 50 μl (Marek's disease vaccine), 200 μl (prebiotics and synbiotics), 700 μl (carbohydrates), to 2,000 μl (electrolytes) (22–25). Many bioactive compounds have been studied for in ovo delivery, including vitamins, amino acids, carbohydrates, prebiotics, probiotics, synbiotics, hormones, minerals, CpG oligodeoxynucleotides, proteins, and immunostimulants (3, 21) (Figure 1).
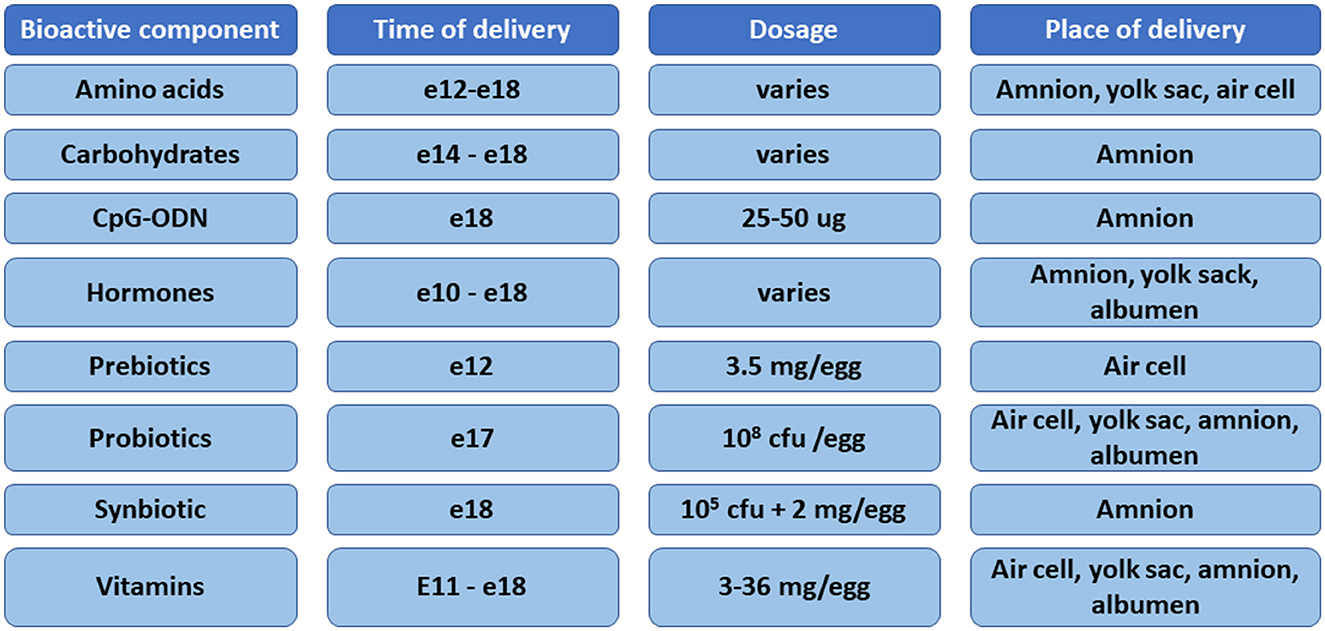
Figure 1. Summary of the bioactive compounds, time of delivery, dose, and sites of injection used for in ovo delivery in chickens.
In this review, we focused on the physiological effects of the in ovo delivery of these substances, including their effects on embryo development, gastrointestinal tract (GIT) function and health, nutrient digestion, immune system development and function, bone development, overall growth performance, muscle development and meat quality, heat stress response, GIT microbiota development, pathogens exclusion, and birds metabolism, as well as transcriptome and proteome (Figure 2). The aim of this study was to characterize the physiological changes caused by the in ovo delivery of bioactive substances and pinpoint directions where this technology could be used more efficiently to improve the overall growth and health of chickens.
Embryo development and hatching
Chicken embryonic development is divided into early, middle, and late phases. Organs and systems form during the early and middle phases, and they grow and mature during the late phase. The staging of embryonic development and hatching behavior have been reviewed (26). A successful embryonic development depends on the nutrient reserves of the egg and external factors that create the optimal environmental conditions for the embryo. The metabolism of fast-growing strains of chickens has created the need to supply the developing embryo with nutrients (27), and this has been done throughout the three embryonic phases depending on the objectives but most frequently at the late embryonic phase to increase nutrient reserves before hatch to support the hatching process and early growth post-hatch.
The in ovo supplementation targeting the early phase has showed that nanoparticles were able to cross the inner membrane and deliver glutamine into the developing embryo. In this study, diamond nanoparticles in combination with 0.3 ml of L-glutamine (50 mg/L) were injected on e1 in the air chamber. The authors showed that this technology may be able to influence energy metabolism because oxygen consumption was increased on e10, and the proliferation and differentiation of the pectoral muscle cells were enhanced (28).
The supplementation of a mixture of essential and non-essential amino acids solution in the yolk on e7 increased the amino acid contents of the embryo, yolk, albumen, allantois, and amniotic fluid (29). The supplementation during early embryonic development directly impacts organ and system formation; however, the supplementation during late embryonic development increases the energy reserve that will support the hatching process. The hatching process is a coordinated event in which the pipping muscle plays an important role (30). Glucose and glycogen reserves, as well as the weight of the pipping muscle, generally increase during the last 2 days of incubation (31). Uni et al. (32) have demonstrated that the supplementation of a solution containing maltose, sucrose, dextrin, and β-hydroxy-β-methylbutyrate (HMB) during e17.5 increased the energy reserve of the embryo that could be used in the hatching process.
Embryo development can be hindered by the presence of mycotoxins in fertile eggs. Mycotoxins contained in breeders' feed may contaminate eggs (33); cause oxidative stress; and damage organs, tissues, and skeletal muscle in the developing embryo (34, 35). Studies have evaluated the effects of bioactive substances against mycotoxicosis during embryonic development. The supplementation of 0.01 g of Arctostaphylos uv-aurs extract (containing phenols and flavonoids) in the amnion on e10 reduced the negative effects of aflatoxin B1 on tibia length and weight (36). Methionine supplementation at 5.90 mg reduced hepatic cell apoptosis caused by aflatoxin B1 toxicity in chicken embryos. However, methionine may have enhanced the oxidative stress caused by aflatoxin B1 as shown by increased superoxide dismutase (SOD), glutathione, glutathione peroxidase (GSH-Px), and catalase activities, despite the reduction in serum malondialdehyde (37). Further research is needed to clarify the effects of methionine and other bioactive substances on mycotoxicosis during incubation in chickens.
Gastrointestinal tract development
The intestinal development during embryonic stages and early post-hatch depends on the availability of nutrients in the egg. The GIT appears early in embryonic development and follows a sequential formation of its components, but it is completely formed by e12 (38). Morphological and physiological changes start by e15 and increase dramatically when the embryo starts swallowing the amniotic fluid on e17 (39). As a result, the proportion of intestinal to embryo weight increased from 1% on e17 to 3.5% at hatch (39). Physiological functions also appear early and continue to develop until the eggs hatch. Enzyme amylase activity appears on e6 and increases in stages until after e19 (40). Intestinal brush border membranes, or microvilli, increase rapidly in the last 2 days of incubation, thereby expanding the intestinal surface area and increasing the digestive and absorptive capacities (41). Brush border enzymes (maltase and aminopeptidase) and nutrients transporter sodium-glucose cotransporter (SGLT)-1 as well as the basolateral Na+K+ATPase activities increase progressively from e15 and reach their highest levels at hatch (39). Therefore, the intestine of the developing embryo has some but limited capacity to digest carbohydrates and proteins (39).
Embryonic development requires nutrients that are supplied by different substrates through metabolic pathways that are divided into three phases. Gastrointestinal development and metabolic pathways have been previously reviewed (2, 30, 42). Briefly, the embryonic development goes through the germ establishment, the embryonic completion, and the emergence phases. The germ establishment is characterized by anaerobic glycolysis. The embryonic completion is characterized by beta oxidation and the use of fatty acids. During the emergence phase, the embryo switches to glycogen reserves as an energy substrate. The glycogen reserve will be used by the embryo for the hatching process, maintenance, and tissue growth prior to hatch and during the post-hatch period. However, the energy reserve may be depleted, and this can hinder organ development post-hatch. As chicks transition from yolk nutrients to exogenous feed, intestinal development and maturation become crucial factors for survival and growth post-hatch (2, 30, 42). Nutritional strategies during embryonic development are used to improve energy reserves in the in ovo technology.
The in ovo supplementation of bioactive substances can be used to provide developing embryos with nutrients to improve intestinal development and function during the peri-hatch period. Substances including carbohydrates, amino acids, vitamins, minerals, prebiotics, probiotics, synbiotics, and plant extracts have been investigated and shown to have beneficial effects on intestinal development and digestive function. The supplementation of amino acids can improve intestinal morphology. A 3.5% threonine (0.6 ml) (43, 44) and 6 or 10 mg arginine (45–47) supplemented into the amniotic fluid on e17.5 increased villus height, villus height to crypt depth ratio, and surface area. The supplementation of a mixture of 10 amino acids, providing 16 g/L of total nitrogen in the yolk sac on e14, increased villus height, muscle layer thickness, and goblet density (48). Glutamine supplemented at 1% in the amniotic fluid on e17 increased total villus cell counts during the peri-hatch period (49). Glutamine serves as a fuel source for enterocytes, and this result confirms that it plays an important role in intestinal development.
Carbohydrates such as 10% and 20% dextrose, 0.1% mannan-oligosaccharide (MOS), 0.528 mg transgalacto-oligosaccharide (Bi2tos), and 4.5 mg raffinose increased villus height on either e12 or e17 (48, 50, 51). The supplementation of 0.1% MOS, 10% and 20% dextrose, 5% and 10% stachyose, and 1.76 mg inulin on either e12 or e17 increased villus surface area (48, 51, 52). In addition, 0.1% MOS increased the intestinal thickness (50), and 4.5 mg raffinose increased the villus height to crypt depth ratio (51). The supplementation of inulin reduced the crypt depth (53). Different synbiotics of 0.528 mg Bi2tos with 1,000 cfu of Lactobacillus lactis subsp. lactis IBBSL1; 1.76 mg inulin with 1,000 cfu Lactobacillus lactis subsp. cremoris IBB SC1 (53); and 0.5% inulin with 106 cfu Enterococcus feacium (54) increased the villus height.
Villus height, villus height to crypt depth ratio, goblet cell number, and density are morphological markers of intestinal health, and the supplementation of various bioactive substances can increase these markers, suggesting that these substances can improve intestinal development during the peri-hatch period. The significance of these markers generally reported in the literature is based on different sections of the intestine (duodenum, jejunum, and ileum) and different periods of incubation or post-hatch, and this makes the comparison between studies difficult to ascertain the effects of these bioactive substances on the intestine as a whole.
Immune system development
The immune system development of chickens starts early in the embryonic stage and progresses until hatching. The ontogeny of the immune system in chickens has been extensively reviewed (55, 56). The components of the gut-associated lymphoid tissue develop progressively during embryonic development, as evidenced by the presence of anlage of Peyer's patches and cecal tonsils as well as clusters of MHC class II+, IgM+, and Bu-1+ cells on e15 (57). Toll-like receptors were highly expressed in the heart, brain, intestine, and liver from e12 to e21 (58). Most of the development of the immune system is complete by the late embryonic phase (59); however, the maturation and response of the immune system to antigens increase with age post-hatch (60, 61). Providing substrates and antigens early in life can accelerate the development and maturation of the immune system of chickens. A previous study has shown that early feeding improves the immune system (62); however, due to delayed access to feed, supplying substances prior to hatch is more attractive.
The immunomodulatory effects of prebiotics and synbiotics supplemented in ovo have been reported. Prebiotic Bi2tos supplemented on e12 in the air sac increased cytokine levels (IL-1β, IL-10, and IL-12p40) in the jejunum, ileum, and cecum as well as avian beta defensin-1 and cathelicidin 2 in the cecum (63). Similarly, a synbiotic (1.9 mg raffinose with 3 × 108 cfu Lactobacillus lactis subsp. lactis IBB SL1) increased IL-4, IL-6, IFN-β, and IL-18 in the spleen of chickens (64). Not all immune-related genes are upregulated by the in ovo supplementation of bioactive substances. Prebiotic (1.76 mg inulin) and synbiotic (1.76 mg inulin with 1,000 cfu of Lactobacillus lactis subsp. lactis 2955) decreased IFN-β, IFN-γ, IL18, IL-4, IL-6, IL-12p40, IL-8, and CD80 during the post-hatch period, with greater magnitude toward day 35 post-hatch (65). For the authors of this study, the fast-growing strains may have prioritized energy for growth as they reached market weight. However, further studies including some disease challenges after in ovo supplementation may help shed light on the effects of these substances on the immune system.
Cell-mediated immunity can also be stimulated by the in ovo supplementation of bioactive substances. In a study where prebiotic (3.5 mg Bi2tos), probiotic (1 × 105 cfu Lactococcus lactis subsp. cremoris IBB477), and synbiotic (3.5 mg Bi2tos with 1 × 105 cfu Lactococcus lactis subsp. cremoris IBB477) were supplemented, the probiotic increased the number of CD4+ lymphocytes in the cecal tonsils on day 7 post-hatch while the prebiotic increased the number of CD4+ and CD8+ cells in the cecal tonsils and CD8+ cells in the spleen on day 21 post-hatch (66). Other synbiotics (103 cfu Lactococcus lactis subsp. cremoris IBBSL1 with 1.76 mg inulin or 0.528 mg Bi2tos) also increased TCRγδ+ lymphocytes in the spleen at days 21 and 35 post-hatch (67). The supplementation of minerals has been shown to enhance the cellular immune response. Minerals (500 μg Zn, 17.5 μg I, or 1.5 μg Se) supplemented on e14 in the yolk or amnion showed that IL-2 and IL-12 genes expression were increased by Zn, while that of inducible nitric oxide synthase (iNOS) was increased by Zn, Se, and I at day 14 post-hatch (68).
Other immune system parameters such as white blood cell counts, heterophil and lymphocyte ratios, and leucocyte phagocytic ability were mostly increased at day 21 post-hatch by the supplementation of inulin, Bi2tos, or synbiotics (inulin with Lactococcus lactis subsp. lactis or Bi2tos with Lactococcus lactis subsp. cremoris) (69). Other substances such as arginine increased total nitric oxide and iNOS at day 7 post-hatch (45), while vitamin D3 and vitamin K3 increased antibody production (70). The supplementation of soybean selenium proteinate and sodium selenium at e18 in the amniotic fluid increased the IL-6, IL-8, and iNOS genes expression in chickens that were co-infected with E. maxima (day 14 post-hatch) and C. perfringers (day 18 post-hatch) (71, 72). These results suggest that some prebiotics, probiotics, amino acids, minerals, and vitamins may improve the immune response.
Muscle development and meat quality
The skeletal muscle formation during embryonic development may influence muscle development and meat quality post-hatch. In general, skeletal muscle fibers are formed through the fusion of myoblasts, and this process is mostly completed by hatch. The fusion of myoblasts is performed by a membrane activator called myomaker, which is required for the formation of multinucleate fibers (73). In chickens, the myomaker gene is highly expressed from e12 to e15 and decreased to nearly zero at hatch, confirming that muscle fiber types are determined during the embryonic development and do not change during the post-hatch period (74). The fiber number and density contribute to muscle growth and weight during post-hatch (75). In addition, intramuscular fat accumulation during embryonic development increases from e17 to day 1 post-hatch and plays an important role in meat quality. Thus, the in ovo programming of muscle development and meat quality post-hatch may be possible.
The supplementation of Bi2tos with Lactobacillus salivarius (SYN1) or raffinose with Lactobacillus plantarum (SYN2) supplemented on e12 in the air chamber showed that SYN1 increased the number and diameter of capillaries and decreased fiber necrosis in the breast meat at day 45 post-hatch. Normal breast meat should have enough blood supply, which is increased by the number of capillaries surrounding muscle fibers. Fewer capillaries reduce blood supply to the fiber and can cause necrosis and affect meat quality (76).
Creatine pyruvate (12 mg) supplementation on e17.5 in the amniotic fluid increased pectoral muscle weight on e19 and days 3, 7, and 19 post-hatch. In addition, muscle glycogen was increased at day 7 post-hatch. Body weight was increased at day 21 post-hatch (77). The increase in glycogen reserve upon entering the hatching process may help improve body weight during the post-hatch period. However, not all bioactive substances have shown positive effects on muscle development or meat quality. In a study where raffinose (1.9 mg) was supplemented in the air chamber on e12, meat quality was negatively affected. In addition, raffinose increased peroxidation in the breast meat after slaughter, and this could negatively affect the quality and shelf life of the meat (78).
The supplementation of Lactococcus lactis subsp. cremoris (105 cfu/egg), Bi2tos (3.5 mg/egg), or Bi2tos (3.5 mg/egg) with Lactococcus lactis (105 cfu/egg) injected into the air chamber on e12 showed no effects on muscle microstructure (fiber diameter, normal fiber, fiber atrophy, giant fiber, change in fiber shape, necrotic fiber, and fiber splitting) (79). The supplementation of arginine at different concentrations (6.25, 25, and 100 mg/kg) on e16 stimulated myoblast differentiation but reduced the number of myofibers and the subsequent muscle growth during the post-hatch period (80). It appears that the supplementation of arginine has negative effects on muscle development and may not be of interest. However, the supplementation of diamond nanoparticles in combination with 0.3 ml of L-glutamine (50 mg/L) on e1 in the air chamber enhanced the proliferation and differentiation of pectoral muscle cells (28). Unfortunately, the authors did not go beyond the embryonic development stage; therefore, the impact of nanoparticles and L-glutamine on muscle development and growth are only limited to incubation time. The in ovo programming of muscle development may depend on the substances and the timing of bioactive substance supplementation during embryonic development.
Bones
The structural soundness is crucial for fast-growing strains of chickens that have been genetically selected for maximum muscle development because of potential negative effects on bone development during embryo development and the early post-hatch period. The bone development in chicken embryos has been reviewed elsewhere (81, 82). In brief, bone formation begins early in embryonic development (83). The skeleton has different structural elements, whose formation is not completed at the same time. The vertebral body formation begins early in embryonic development and continues with differentiation and mineralization until e19 (84). Wing and leg bones are partially mineralized by e12, but the mineralization is mostly completed by e16. For ribs and pelvic bones, mineralization is initiated at e12 (85). Tibia bone elongation and mineralization increase between e14 and e17, while those of the femur occur between e19 and e21 (86). Regardless of the sequence of bone formation and mineralization, minerals are exported from the egg yolk and eggshell at different periods. While the yolk supplies most minerals during early phase of embryo development, eggshell supplies a greater amount of minerals between e12 and e16 (85). Chicken bone formation and mineralization slow down during the late embryonic phase, and this coincides with the depletion of the egg mineral (Cu, Fe, Zn, P, and Mn) reserves (86, 87). In addition, the muscle development of fast-growing strains puts more pressure and load on their skeleton, resulting in all forms of bone deformities and leg problems (88). Therefore, the supplementation of minerals during the late embryonic phase could increase the mineral uptake by the developing embryo and increase mineral reserves to support the rapid bone development and mineralization during the post-hatch period. In addition, vitamin D and vitamin K are two principal actors that regulate the mobilization of minerals from the yolk and eggshell, and their supplementation in combination with minerals during embryonic development may increase mineral absorption, at least Ca and P, and improve bone development (70, 89).
The supplementation of organic trace minerals (P, Zn, Cu, and Mn), phosphate, and vitamin D; inorganic trace minerals (P, Zn, Cu, and Mn) and phosphate (90); and maltodextrin containing Fe, Zn, Mn, Ca, Cu, and P (87) on e17 in the amniotic fluid increased the Zn, Cu, and Mn contents of the yolk on e19 and at hatch. Unfortunately, these studies (87, 90) did not determine mineral content beyond hatch. Contrary to these results, Oliveira et al. (91) reported no effects of in ovo supplementation of Mn, Zn, and Cu on mineral (Ca, P, and Zn) content at days 1, 7, and 21 during the post-hatch period. There were no effects of in ovo supplementation with 25-hydroxycholecalciferol (vitamin D3) on bone mineral contents (92). These discrepancies in the literature regarding the effects of minerals and vitamin supplementations on bone mineral contents are likely due to the nature of the supplements, the organs used (yolk as opposed to bone), and the periods of sampling. Further research is needed to investigate the effects of the in ovo supplementation of minerals and vitamins covering at least the late embryonic phase and the life span of fast-growing strains of broiler chickens.
The main objective for supplying the developing embryo with minerals or vitamins is to reduce mineral deficiency during peri-hatch period and improve bone development and characteristics. The in ovo supplementation of minerals (Zn, Cu, Mn, and Ca) and vitamin D3 improved tibia and femur length, stiffness and thickness, load and work to fracture, breaking strength, and bone weight during the post-hatch period (90). Similarly, the supplementation of 0.6 μg of vitamin D3 and vitamin K3 in the amniotic fluid on e18 improved tibia fracture force, bone weight, and breaking strength of the bone while higher doses of vitamin D3 >0.6 μg reduced bone strength (70). Contrary to the studies (87, 90, 91) mentioned in the previous paragraph, others have reported no effects of in ovo supplementation of minerals and vitamins on bone characteristics. No treatment effects of supplemental minerals (Zn, Mn, and Cu) on bone Ca, P, Mg, Mn, and Zn concentrations despites increased % ash of the bone (93). In addition, no effects of vitamin D supplementation on breaking strength were reported by Bello et al. (92). It is worth mentioning that the dosage, type, and combination of these minerals with vitamins may explain the differences. Alternatively, higher dosages of minerals and vitamins may incur some toxicity or mineral imbalance that may have confounded the results of these studies.
Microbiota development
Chicken GIT microbiota is composed of bacteria, fungi, viruses, and protists and is characterized by commensal, symbiotic, and pathogenic relationships with its host (94). Bacterial species identified in the broiler chicken's GIT (95) play important roles in host nutrition, including feed digestion, nutrient absorption and metabolism, pathogen exclusion, endocrine activity, immune system development and functioning, and performance efficiency (96). In broiler chickens, the symbiotic relationships between the host and the microbiota have been characterized by nutrient exchange, modulation of the immune system, GIT physiology, and pathogen exclusion (6, 95, 97–99). Microbiota composition and function can be affected by many factors, including age; host genotype and sex; diet composition and form; dietary ingredients such as probiotics, prebiotics, synbiotics, phytobiotics; and bacteriophages, stress, antibiotics, and location in the GIT (97, 99, 100). Colonization of the newly hatched GIT occurs through a process known as ecological succession (101). The GIT microbiota reaches the mature community state between weeks 2 and 3 post-hatch (102). Early colonization of beneficial bacteria in the GIT can prevent not only intestinal disorders caused by pathogenic bacteria but also promote the maturity and integrity of the GIT (103). Any improvement in early GIT maturation and digestive ability will positively impact the growth and production performance of chickens (50). In ovo injection has the possibility to change bacterial composition in GIT short-term and long-term and influence its developmental process. Most of the studies published and summarized below focus on the effects of probiotic delivery on GIT colonization and microbiota balance.
Pedroso et al. (104) showed that in ovo administration on e18 of a probiotic competitive exclusion product derived from adult chicken microbiota influenced the development of broilers microbiota in both fast-growing and heritage-breed chickens. They showed that the early administration of microbiota increased the diversity and taxonomic composition of recipient microbiota (104). Additionally, in heritage birds, the abundance of undesirable bacteria phylum such as Proteobacteria was reduced by in ovo treatment (104). Injection of Bacillus subtilus, Entrococcus faecium, or Pediococcus acidilactici (107 cfu) on e18 into the amnion decreased the population of E. coli and increased the abundance of lactic acid bacteria during the first week post-hatch (105). Supplementation of lactic acid bacteria probiotic, Flora-Max B11 (104 cfu/egg into the amnion), on e18 influenced the microbiota composition in the GIT by increasing the abundance of lactic acid bacteria post-hatch (106). Additionally, in ovo delivery of Flora-Max-B11 reduced the salmonella incidence in comparison to non-treated birds (106). Injection of different Bifidobacteriun spp. (2 × 108 cfu of B. bifidum, B. animalis, B. longum, or B. infantis) into the yolk sac on e17 increased the abundance of lactic acid bacteria and decreased the total counts of bacteria and coliform in ileum (107). Wilson et al. (108) have shown that supplementation with 102 cfu of lactic acid bacteria such as Citrobacter freundii or Citrobacter spp. on e18 influenced the initial microbiota of the ceca. Moreover, changes in ileal microbiota diversity were observed after in ovo inoculation of Citrobacter freundii, Citrobacter spp., or lactic acid bacteria mixture on e18 into the air cell (102 cfu/egg) at days 3 and 10 post-hatch (109). Lactic acid bacteria supplementation increased the colonization of butyrate-producing bacteria and decreased the level of Enterococcaceae (109). Another study has shown that inoculation of embryos with lactic acid bacteria isolated from adult hen on e19 (107 cfu/ml) induced variation in cecal microbiota as shown on day 7 post-hatch. This variation was characterized by a reduction in Enterobacteriaceae, an increase in the abundance of Ruminococcaceae, and an enrichment of Proteus, Butyricicoccus, Lachnospiraceae, and Erysipelotrichaceae (110).
Probiotics and prebiotics functions are different in GIT; the prebiotics stimulate the development of microbiota and improve the microbiota balance, whereas probiotics colonize the environment (111, 112). Supplementation with two different synbiotics (105 cfu L. salivarius and 2 mg GOS or 105 L. plantarum and 2 mg of raffinose-family oligosaccharides per egg) on e12 resulted in changes in microbiota composition in ileum and ceca on day 21 post-hatch as determined by fluorescent in situ hybridization (113). Both synbiotics decreased the abundance of Lactobacillus spp. and Enterococcus spp. in the ileum, while in the cecum, Bacteroides-Prevotella cluster was lowered and Eubacterium rectale cluster was increased in comparison to non-injected chickens (113). GOS (3.5 mg/egg) supplementation on e12 (air cell) significantly decreased the relative abundance of Lactobacillus spp. in the ileum and increased the level of Bifidobcterium spp. in the cecum at 42 days post-hatch (63).
Other studies have shown that in ovo supplementation with chito-oligosaccharide and chlorella polysaccharide (5 or 20 mg/egg) on e12.5 led to changes in cecal microbiota later in life (day 21 post-hatch) (114). The relative abundance of polysaccharide-utilizing bacteria such as Lactobacillus johnsonii, Bacteroides coprocola, and Bacteroides salanitronis was higher in supplemented birds. At the same time, the abundance of opportunistic pathogenic bacteria was decreased due to the supplementation (114). Interestingly, the supplementation did not influence the development of microbiota, since no changes in taxonomic composition were observed at 3 days post-hatch (114). Injection of the essential amino acid, i.e., arginine (0.5–1%) into the amniotic fluid on e14 increased the abundance of Lactobacillus and decreased the levels of Coliform and E. coli bacteria (115).
Besides synbiotics, probiotics, and prebiotics, microbiota development has also been shown to be influenced by other bioactive substances, such as host-defense peptides or antioxidants. Cuperus et al. (116) showed that in ovo injection of the host-defense peptide—chicken cathelicidin-2 (1 mg/kg of body weight, e18 into the amnion) altered the GIT microbiota by reducing the abundance of Ruminococcaceae and Butyricicoccus in the ceca and Escherichia/Shigella in the ileum and ceca at 7 days post-hatch. Intraamniotic administration of isoflavone genistein (1.25 and 2.5%) on e17 positively altered the composition and function of the intestinal microbiota by increasing the abundance of Bifidobacterium spp., Lactobacillus spp., and Clostridium spp. and decreasing the level of E. coli (117).
Metabolism
Adequate energy reserve in the embryo of fast-growing chickens is the basis for later successful growth performance. The metabolic pathways in poultry embryos prior to hatch have been reviewed elsewhere (2, 42). During the late embryonic phase, the developing embryo increases its energy reserves through gluconeogenesis and glycogenesis using protein from the amniotic fluid and tissue reserve. Gluconeogenesis occurs mainly in the yolk sac, but also in the liver during late embryonic development (118). The synthesized glycogen is then stored in the liver, muscles, and yolk (118). These energy reserves will be used for the hatching process and during the early post-hatch period for maintenance and development (2, 42, 119). However, the yolk nutrient reserves are depleted during the late embryonic phase, and the muscle of the developing embryo becomes the main source of amino acids for gluconeogenesis. In addition, after hatching, chicks rely on the residual nutrients of the yolk as they transition to exogenous nutrient sources (32). As modern chicken strains have been selected to grow about twice as fast with liver maturing earlier in heritage lines (120), embryonic development has become a crucial phase to prepare and equip newly hatched chicks for this fast-growing process. In the last two decades, studies have focused on supplying various nutrient substrates to the developing embryo, more specifically, during the late embryonic phase between e14 and e18, to increase energy reserve and other nutrients that will support organs and structural development post-hatch.
A mixture of 1 g/L β-hydroxy-β-methylbutyrate, 25 g/L maltose, 25 g/L sucrose, and 200 g/L dextrin supplemented on e17.5 in the amniotic fluid increased liver and pectoral muscle glycogen at e20, hatch, and days 10 and 25 post-hatch (32). Similarly, 15 mg creatine monohydrate in combination with 62.5 mg glucose injected in the amniotic fluid on e17.5 increased muscle glycogen on e19 (121). The supplementation of 12 mg creatine pyruvate injected in the amniotic fluid on e17.5 also increased liver glycogen reserve on e19 and at hatch (77).
Various substances can be used to increase energy reserves; however, it is important that these substances or the end products of their digestion or hydrolysis can serve as substrates for gluconeogenesis. It is possible that the increase in energy reserve has spared muscle catabolisms resulting in increased body weight and organ development at hatch which were sustained during the post-hatch period (32, 77, 121). The in ovo injection of nucleosides on e18 (25–100 mg/egg) led to significantly higher metabolizable energy in chickens on day 14 post-hatch (122). Nucleosides, the materials for nucleic acid synthesis, are suggested to play an important role in the development of the GIT and immune system (122). Supplementation of creatine pyruvate (12 mg/egg) on e17.5 increased energy reserves in broilers (123). The same dose of creatine pyruvate increased plasma creatine and pyruvate concentration, liver pyruvate, glycogen and glucose concentration, and mRNA expression of gluconeogenesis and glycogenesis enzymes in the liver before hatch or early post-hatch (124).
Furthermore, in ovo stimulation of embryos with Lactobacillus-based synbiotics on e12 increased glucose, insulin, glucagon, and leptin levels in plasma and influenced the expression of metabolic genes in muscle (125). However, more changes in metabolic gene expression were seen early post-hatch (day 7) vs. the end of the production cycle (day 42). Among the metabolic genes, follistatin, pyruvate dehydrogenase kinase, isozyme 4, CCAAT/enhancer binding protein beta, phosphorylase kinase regulatory subunit beta, AMP-activated protein kinase, and gamma 3 subunit were significantly upregulated due to synbiotic delivery (125).
Gastrointestinal health
Intestinal health is a complex and dynamic interaction involving the intestinal mucosa and microbiota, the immune system, and feed utilization. This section will mainly focus on the effects of in ovo supplementation with bioactive substances on the intestinal mucosa. The epithelium is the mucosal layer that forms the direct interface between the body and the intestinal lumen. It is formed by a single cell layer made of different cell types, including enterocytes, goblet cells, enteroendocrine cells, Paneth cells, and tuft cells (126). The intestinal barrier formed by the epithelial cells is guarded by a complex structure including the tight junction, adherent junction, gap junction, and desmosome that spans the paracellular space. The most apical component, the tight junction, comprises the transmembrane proteins claudins (CDN), occludin (OCLN), and the scaffolding molecules of the cytoskeleton, zonula occludens (ZO). The tight junction regulates paracellular transport through the intestinal barrier by selectively preventing harmful molecules and pathogens from entering the body while allowing the passage of smaller molecules and nutrients (127). The intestinal mucosa is completely formed at hatch, but morphological and physiological changes continue during the post-hatch period (41). During early post-hatch when chicks transition from using nutrients from the residual yolk to exogenous feed, their immature intestine is more vulnerable to opportunistic pathogens and toxins from the feed (128). Although, early feeding during post-hatch to provide nutrients to hatchlings has been developed, in ovo programming of intestinal barrier functions has gained attraction recently.
The in ovo supplementation of bioactive substances can provide nutrients not only for energy reserve to support early life but also to improve the development of the intestinal mucosa and its barrier components. In general, tight junction proteins are assessed through the expression levels of their respective genes. Arginine supplementation (6 or 10 mg) increased CDN-1, ZO-1, and ZO-2 mRNA expression levels (47). Despites the crucial role of tight junctions in intestinal health, limited reports exist on the effects of in ovo supplementation of bioactive substances on its different components. Further research is warranted to assess the effects of in ovo supplementation not only on gene expression of the tight junction proteins but also on the expression of their protein levels in the intestinal mucosa.
In addition to the tight junctions, the intestinal epithelium is covered by a protective mucus layer, which plays a crucial role in the intestinal defense mechanism. The mucus layer is formed by the gel-forming mucins (MUC) produced by the goblet cells. Different types of mucins exist, but the MUC2 gene expression level has been extensively used as a marker of intestinal health in poultry. Because the intestine is still immature at hatch and undergoing morphological and physiological changes (41), the presence of the mucus layer is critical for its protection against pathogens and toxins present in feed. The in ovo supplementation of bioactive substances can modulate the gene expression levels of MUC2. The gene expression level of MUC2 was increased by the in ovo supplementation of threonine, arginine, and methionine (43, 44, 47, 129). The supplementation of 107 cfu of B. subtilis on e18 increased MUC2 gene expression in intestinal mucosa at e21 and day 3 post-hatch (105). Goblet cell numbers and density were increased when methionine, glutamine, and synbiotics (inulin + Lactobacillus lactis subsp. lactis IBBSL1) were supplemented in ovo in chickens (53, 129, 130). Although the MUC2 gene expression has been extensively used in poultry as a marker of intestinal health, gene expression may be transient and may not reflect the actual protein level of the mucin. In addition to the gene expression, the mucus thickness may be of interest since the mucus layer may not cover the entirety of the intestine as reported in rats (131). Further research needs to determine mucin protein levels and mucus thickness to expand our knowledge of in ovo supplementation on intestinal mucosa barrier function.
Digestion
The small intestine plays an important role in the digestion and absorption of nutrients. Although the intestine is completely formed and functional at hatch, it continues to develop and mature as the chick transitions to exogenous nutrient sources (132). The main accessory digestive organ, the pancreas, also continues to develop, as evidenced by its histogenesis during the post-hatch period (133). The activity of digestive enzymes secreted by the pancreas, including amylase, trypsin, lipase, and chymotrypsin, has been shown to increase during the post-hatch period (134). Because the intestine is immature at hatch and fast-growing birds in the modern poultry industry have been genetically selected to reach market weight by 6–8 weeks after hatch, the intestine needs to reach its full functional digestive and absorptive capacities quickly. Luminal digestion can be improved through in ovo supplementation of bioactive substances that enhance digestive enzyme activities. In this regard, the in ovo supplementation of 1% arginine increased maltase and sucrase activities at day 7 post-hatch (45). Synbiotics, 0.528 mg Bi2tos with 1,000 cfu of Lactobacillus lactis subsp. lactis IBBSL1 and 1.76 mg inulin with 1,000 cfu Lactobacillus lactis subsp. cremoris IBB SC1, injected into the air sac on e12 enhanced amylase, lipase, and trypsin activities post-hatch (135).
In addition to enzymatic activity in the intestinal lumen, nutrient digestion is completed with other enzymes at the brush border membrane of enterocytes (136). Reicher and Uni (41) recently reported that the development and maturation of the intestinal digestive and absorptive functions depend on intestinal mucosa growth and surface expansion, both of which take more time during the post-hatch period. Structural changes can still occur over 7 days post-hatch when the intestinal mucosa reaches its maximum growth rate (41). This suggests that the newly hatched chicks have limited capacities to complete the digestion and absorption of carbohydrates, di-, and tripeptides in their early days post-hatch. Improving intestinal digestive and absorptive capacities is nowadays possible by increasing brush border membrane enzyme activities and nutrient transporters early during embryonic development (5). The in ovo supplementation of 0.1% MOS increased the aminopeptidase and sucrase-isomaltase activities of the brush border membrane (50). Prebiotics (5% and 10% of raffinose and stachyose) upregulated the mRNA expression of aminopeptidase and sucrase-isomaltase genes (52). A 3.5 % threonine in ovo supplementation increased mRNA expression of aminopeptidase N gene (43). Furthermore, in ovo supplementation of bioactive substances can improve nutrient transporter activities. For example, glutamine increased the differentiated di- and tri-peptide transporter 1 (PepT-1) region on intestinal villi (49). In ovo supplementation of threonine increased the mRNA expression of SGLT-1, glucose transporter 2, and alanine, serine, cysteine, threonine (ASCT) transporter 1 (137) and the expression of PepT-1 (43, 137). Prebiotics (5% and 10% of raffinose and stachyose) upregulated the mRNA expression of SGLT-1 (52). Intestinal luminal and mucosal digestive and mucosal absorptive capacities can be improved by in ovo supplementation of various bioactive substances.
Birds' performance
Current broiler lines are characterized by rapid growth, high body weight at slaughter, and a reduced feed conversion ratio. The in ovo supplementation of bioactive substances has been shown to influence chicken performance parameters. Body weight at hatch or during the post-hatch period was increased by in ovo supplementation of Bi2tos (24, 138), Bacillus-based probiotics (139); lactic acid bacteria-based probiotics (110); a mixture of Lactobacillus acidophilus, Lactobacillus casei, and Bifidobacterium bifidum (140); inulin; and Lactococcus lactis spp. lactis 2955 (24). Furthermore, arginine (47) and 60 mg Zn injected on e18 in the amniotic fluid (141) increased body weight and feed intake and decreased feed conversion ratio (46, 47). Production parameter reports are not always consistent in the literature. Recent reports have highlighted the fact that some bioactive substances have had unintended results by increasing feed intake and feed conversion ratio. Some synbiotics, such as raffinose with Lactococcus lactis ssp. cremoris IBB SC1, lactose with Lactobacillus acidophilus and Streptococcus faecium (142), and Lactobacillus plantarum with Astragalus polysaccharide, or probiotics, such as Lactobacillus plantarum with an increased feed conversion ratio (143). These conflicting results are likely due to the types of bioactive substances, dosages, environmental conditions, and management differences. In addition, the periods considered for feed intake, body weight gain, and feed conversion ratio are not always the same across studies. To advance our knowledge of the impacts of bioactive substances on production parameters, further research needs to take these factors into consideration and develop standards that can facilitate the transfer of results and the adoption of these new in ovo supplementation technologies by the poultry industry.
Epigenetic regulation
Epigenetic modification of gene expression works through the methylation of DNA. This process can be modulated by nutritional and stress factors as well as intestinal microbiota (144–146). Dunislawska at al. (147) showed that in ovo delivery of Lactobacillus-based synbiotics on e12 significantly changed methylation pattern of several hepatic genes related to metabolism. Similarly, microbiota modulation by prebiotics such as GOS and inulin, or Lactobacillus-based synbiotics have shown an ability to modulate the methylation pattern of splenic genes in fast-growing broilers and native chickens (148). However, the epigenetic changes depended on the chicken genotype and the bioactive substances delivered in ovo (148). It has been suggested that the mechanisms of negative changes in gene expression in the liver of broiler chickens after Lactobacillus-based synbiotic injections are probably related to changes in DNA methylation patterns and miRNA activity (147, 149). Additionally, Zhu et al. (150) have shown that in ovo delivery of vitamin C on e11 resulted in changes in the mRNA expression of genes related to DNA methylation such as DNA methyltransferase, DNA demethylation, growth arrest, and DNA-damage inducible protein beta, thymine-DNA glycosylase, and methyl-CpG-binding domain protein 4 at day 21 or 42 post-hatch.
Transcriptome
The in ovo delivery of bioactive compounds has been shown to affect gene expression in several tissues in developing embryos and post-hatch chickens. Injection in ovo of prebiotics and synbiotics on e12 influenced the transcriptomic profile of the spleen, cecal tonsils, and large intestine post-hatch (151). The most differentially expressed genes were observed in the cecal tonsils, and galacto-oligosaccharides (GOS) were the most potent stimulators of the host transcriptome (151). Moreover, lymphocyte proliferation, activation, and differentiation, as well as cytokine production, were the most affected gene ontology categories in cecal tonsils (151). Previous studies from the same group have shown that early delivery of synbiotics composed of raffinose family oligosaccharides and Lactococcus lactis upregulated and downregulated interleukin mRNA expression in the spleen and cecal tonsils, respectively (64). Downregulation of the expression of immune-related genes in the spleen and the cecal tonsils was observed after injecting the chicken embryos on e12 with inulin or GOS together with Lactococcus lactis (65). Synbiotics, Lactobacillus salivarius plus GOS and Lactobacillus plantarum plus raffinose family oligosaccharides, injected in ovo on e12 decreased expression of two incretins: glucagonlike peptide 1 (GLP-1) and glucose-dependent insulinotropic peptide (GIP) mRNA in the duodenum and GLP-1 receptor mRNA in pancreas post-hatch (152). Both incretins are involved in many biological functions (153). The study by Kolodziejski et al. (152) suggests that synbiotics can be directly or indirectly involved in incretin secretion and action. Injection of synbiotics also resulted in transcriptome changes in the spleen, jejunum, cecal tonsils, and liver on day 35 post-hatch (154). These authors showed that the synbiotic based on Lactobacillus salivarius was involved in the activation of pathways related to fat and carbohydrate metabolism, cell adhesion, and immune response, while the synbiotic based on Lactobacillus plantarum was involved in the upregulation of expression of genes involved in metabolic pathways (154). In another study, Bertocchi et al. (155) showed that in ovo supplementation with prebiotics (GOS) did not result in any differentially expressed genes in the jejunum and cecum post-hatch; however, gene set enrichment analysis (GSEA) showed 11 significantly enriched gene sets related to energy metabolism and oxidation in the jejunum. In contrast, very few changes in enriched gene sets were observed in the cecum after in ovo injections of GOS (155). The supplementation of GOS on e12 (3.5 mg/egg) attenuated the negative effects of acute and chronic heat stress post-hatch by decreasing the mRNA expression of IL-4, IL-12p40, and SOD in comparison to non-injected birds (156). Additionally, it has been shown that administration of Lactobacillus-based synbiotics affects the metabolism and development-related gene expression in the liver in broiler chicken (154), while in ovo administration of inulin (1.76 mg/egg) as a prebiotic or inulin and Lactococcus lactis (1.76 mg + 1,000 cfu/egg, respectively) on e12 led to changes in immune gene expression in peripheral tissues, cecal tonsils, and spleen (65). Besides synbiotics having wide effects on gene expression in multiple tissues, probiotics alone have been shown to influence mRNA levels. Embryonic injection of multi-strain lactobacilli mixture composed of Lactobacillus salivarius, L. reuteri, L. crispatus, and L. johnsonii on e18 (105-107 cfu/egg) led to changes in cytokines mRNA expression in cecal tonsils, bursa of Fabricius, and spleen at 5 and 10 days post-hatch (157). Upregulation of cytokines (IFN-α, IFN-β, IFN-γ, IL-8, and IL-12) expression was observed in the spleen, while cecal tonsils were characterized by downregulation of IL-6, IL-2, and IL-8 post-hatch. In the bursa of Fabricius, only IL-13 expression was affected by Lactobacillus in ovo delivery (157).
Although several studies utilized synbiotics or probiotics, transcriptome has also been shown to be influenced by the delivery of other bioactive substances. For example, intra-amniotic zinc-methionine administration on e17 increased zinc exporter mRNA expression in the small intestine as well as the expression of brush border enzymes and transporter genes, suggesting its role in intestinal development enhancement and improved functionality (158). Zinc plays an important role in many biological processes, and it is required for enzyme function, nucleic acid synthesis, and as a cofactor in many proteins (159–162).
Other studies have shown that perinatal administration of 2 mM quinine on e17 affected the expression level of gustatory (palate) and extra-gustatory (duodenum) mRNA for the three bitter taste receptors ggTas2r1, ggTas2r2, and ggTas2r7 and their signaling components alpha-gustducin, phospholipase, and transient receptor potential melastatin 5 (163). Amino acids have also been implicated in gene expression regulation. Threonine injection (from 17.5 to 70 mg/ml) into amniotic fluid on e17 resulted in increased mRNA levels of the MUC2 gene and PepT-1 at hatch, suggesting the role of threonine in the functional development of intestinal mucosa (43).
Zhao et al. (123) showed that creatine pyruvate (12 mg/egg) in ovo injection on e17.5 increased the expression level of myogenic differentiation 1, myogenin, and paired box 7 at day 3 post-hatch, leading to enhanced muscle growth and increased satellite cells activity (123). Moreover, injection of creatine pyruvate affected the expression level of gluconeogenesis and glycogenesis enzymes in the liver during the late embryonic phase and early post-hatch indicating that in ovo delivery of creatine pyruvate may increase energy reserves in the liver in broiler chickens early post-hatch (124).
Few studies have also shown the effects of growth factors and vitamins on transcriptomics. Epidermal growth factor (EGF) has been shown to be involved in the stimulation of proliferation, differentiation, and maturation of neonatal intestinal cells in mammals (164, 165). In birds, in ovo delivery of EGF (160–640 μg/egg, e17) increased EGF receptor mRNA expression only during the end of embryonic development and had no effects post-hatch (166). Supplementation of vitamin C (3 mg/egg into amnion on e11) regulated the expression of inflammatory cytokines while the same dose injected on e18 into the air sac decreased the expression of proinflammatory cytokines such as IL-1β, IL-6, and TNF-α in the spleen and increased expression of antioxidant genes such as GSH-Px and SOD (150, 167).
Proteome
Information addressing the changes in proteome due to in ovo delivery is limited. Dunislawska et al. (168) have shown that synbiotics based on Lactobacillus plantarum and raffinose family oligosaccharides administered in ovo on e12 led to changes in the expression of the liver proteome in chicken post-hatch. Injection of 200 μl of a synbiotic, 2 mg/egg of oligosaccharides, and 105 cfu of Lactobacillus plantarum into the air chamber resulted in 16 differentially expressed proteins (5 downregulated and 11 upregulated) in chicken liver at 21 days post-hatch. Analysis of the differentially expressed proteins determined that they belong to mitochondrial, cytoskeleton, cytoplasmic, and cytoskeleton organizing proteins (168). The authors concluded that Lactobacillus-based synbiotics have the ability to accelerate the energy-yielding metabolic pathway in the liver of 21-day-old broilers (168).
Wilson et al. (169) have found that in ovo administration of 102 cfu (200 μl volume, into amnion) of Citrobacter freundii, Citrobacter spp., or mixed of Lactobacillus salivarius and Pediococcus spp. on e18 resulted in the changes in 107, 39, and 78 proteins level in the gastrointestinal tract at hatch. These proteomic changes were associated with antioxidant capacity, gluconeogenesis, cellular oxidative stress, and inflammatory stimulus (169). In another study, supplementation of pathogenic Enterobacteriaceae isolates and lactic acid bacteria in ovo (102 cfu, Citrobacter spp., and L. salivarius and Pediococcus ssp., e18, amnion) were associated with activation and balance function of the innate and adaptive immune systems (lactic acid bacteria) and attenuation of processes related to the development of the immune system and dysregulation of immunological mechanisms (Enterobacteriaceae) at hatch (170). Besides the early post-hatch effects of probiotic inoculation on proteomic, Rodrigues et al. (171) have shown activation of inflammation pathway in chicks inoculated with lactic acid bacteria or Citrobacter freundii (e18, 102 cfu, into amnion) 10 days post-hatch in ileum. Moreover, in ovo delivery of lactic acid bacteria was associated with the activation and trafficking of immune cells and skeletal growth. Exposure to Enterobacteriaceae (C. freundii) was related to the inhibition of biological function associated with immune cell migration and the inflammatory response (171).
Pathogen exclusion
The defense mechanism of newly hatched chicks is minimally developed and limited to the innate immune system and maternal antibodies (44). During early post-hatch development, chicks are exposed to many organisms, and due to a low level of colonization of their GIT, they are susceptible to pathogen colonization (44, 104, 172, 173). There are a few mechanisms of competitive exclusion proposed, and they involve the creation of an unfavorable environment for invading bacteria, utilization of receptor sites by commensal bacteria, production of antimicrobial substances, and competition for nutrients allowing for the selection of certain strains of bacteria (174–176). In chicken, administration of 3.5% threonine into the amniotic fluid on e17.5 reduced Salmonella enteritidis colonization and ameliorated the negative effects of Salmonella infection, including intestinal morphology and integrity, and performance (44). The proposed mechanism of action of threonine involves increased expression of MUC2 gene coding for intestinal mucin and level of mucosal antibodies IgA, and accelerated maturation of the GIT (44). Another study has shown that administration of Bi2tos (3.5 mg/egg) on e12 resulted in reduced negative effects of natural Eimeria infections, including intestinal lesions and oocyte excretion in Kuroiler chickens (177). Arreguin-Nava et al. (110) showed that injection of defined lactic acid bacteria (107 cfu/200 μl) isolated from adult hens on e19 decreased the Enterobacteriaceae colonization after hatch in chickens challenged with virulent E. coli in horizontal infection model. Reduction in Enterobacteriaceae colonization was accompanied by improved body weight gain, reduced mortality at day 7 post-hatch, and variation in cecal microbiota (110).
Heat stress response
Heat stress negatively affects poultry welfare and productivity (178). Severe physiological changes caused by heat stress include impaired thermotolerance, acid-base imbalance, oxidative stress, and reduced immunocompetence. Behavioral and physiological changes used by chickens to adapt to elevated temperature result in increased mortality, reduced feed intake and body weight gain, and reduced meat quality (179). Management (cooling systems) and nutritional (high-fat diet, supplementation of osmolytes, vitamins, and minerals) strategies have been implemented to mitigate these negative impacts of heat stress on poultry (179). Recent studies have investigated the use of in ovo supplementation of bioactive substances to mitigate the negative impacts of heat stress in poultry, especially in broilers due to their fast-growing body and increased metabolic rate (156, 180–183).
The in ovo supplementation of L-leucine on e14 and e19 at 25 mm depth into the egg was followed by exposure of the chicks to heat stress at day 9 post-hatch for 3 h (181). The L-leucine supplementation reduced heat shock proteins 70 and 90 in heat-stressed chickens (181). In the same study, heat stress reduced liver arginine and lysine and increased liver glutamine, aspartic acid, and citrulline. Heat stress negatively affected amino acid metabolism; however, the in ovo supplementation of L-leucine was not able to mitigate these effects in the heat-stressed chickens (181). Furthermore, the supplementation of 10% γ-aminobutyric acid in ovo on e17.5 in the amniotic fluid did not reduce the negative effects of heat stress on various parameters, including rectal temperature, average daily feed intake, average daily gain, and antioxidant balance (180). However, the supplementation of 3.5 mg of Bi2tos on e12 downregulated the expression of cytokines IL-4 and IL-12p40. This downregulation could be a result of reduced activation of the immune system. Heat stress is known to damage the intestine and increase the translocation of antigens that can trigger the immune system. It is possible that the downregulation of IL-4 and IL-12p40 is related to intestinal health. Further study needs to clarify this hypothesis (156). In addition, the supplementation of 3.5 mg Bi2tos on e12 did not reduce the negative effects of heat stress including breast muscle weight loss, increased feed conversion ratio, and reduced weight gain (182, 183). Although in ovo supplementation has shown promise in improving various parameters during the post-hatch period, heat stress is a multifactorial problem and further research is needed to comprehensively define substances that can be used to mitigate the negative consequences of heat stress in chickens.
In ovo effects in other avian species
Most studies related to in ovo delivery have been focused on broiler chickens. Information addressing other species is somehow limited in comparison to broilers, but in ovo administration of different bioactive substances has been characterized also in other avian species such as turkey, pigeon, quail, and duck.
The in ovo supplementation of bioactive substances, including fatty acids, amino acids, and carbohydrates has been investigated in turkeys. The supplementation of branched-chain amino acids (leucine, valine, and isoleucine) increased yolk sac and pancreas weight at hatch and breast muscle weight on e24 and hatch (184). Butyric acid (10, 20, or 30 mg/egg) injected into the yolk sac on e7 improved hatching weight and increased villus height in the duodenum, jejunum, and ileum at day 21 post-hatch. In addition, the feed conversion ratio was reduced, and body weight was increased at hatch and days 21–42 post-hatch (185). Earlier studies have shown that β-hydroxy-β-methyl-butyrate (HMB) and arginine have the potential to improve the development of intestinal digestive and absorptive functions. Foye et al. (186) demonstrated that the interaction between HMB (0 and 0.1%) and arginine (0 and 0.7%) injected on e23 into the amniotic fluid increased the expression of sucrase-isomaltase gene on e25 and those of PEPT-1 and SGLT-1 at hatch. Furthermore, the interaction of HMB and arginine increased jejunal sucrase, maltase, and leucine aminopeptidase activities at e25 and day 14 post-hatch (187). The supplementation of a mixture of dextrin and iodinated casein (75 μg/ml) and dextrin (18% maltodextrin and 10% potato starch dextrin) increased poults' weight at hatch and day 7 post-hatch (188). These results show that in ovo supplementation of bioactive substances has the potential to improve intestinal development and function and growth performance post-hatch.
Research on in ovo supplementation of bioactive substances in domestic pigeons is limited. The supplementation of 2.5% maltose and 2.5% sucrose on e14.5 in the amniotic fluid increased body weight and pectoral muscle at hatch (189) and showed that in ovo supplementation of carbohydrates can enhance intestinal development and digestive functions through increased villus surface area, enhanced brush border enzymes (maltase and sucrase) activities, and upregulation of nutrient transporter genes (SGLT-1 and GLUT-2) (190). Arginine (1%) injected on e13 in the amniotic fluid increased body weight at hatch and day 13 post-hatch, while breast and leg meat yields were increased at day 7 post-hatch (191). Contrary to arginine, histidine supplementation did not affect breast weight (192). In addition, the supplementation of a mixture of amino acids (arginine, lysine, and histidine) at 0.1, 1, or 10% in the amniotic fluid on e13 increased body weight and the relative weight of the heart, kidney, liver, and small intestine at hatch (193). These results suggest that in ovo supplementation of amino acids and carbohydrates may help improve embryo development.
Administration of leptin (0.1 or 1 ug per egg) on e5 into the albumen in quail embryos resulted in an earlier hatch and higher post-hatch body weight in treated animals. Moreover, leptin treatment led to changes in endocrine and metabolic parameters such as thyroid hormones and total lipids or triacylglycerol concentrations during post-hatch development (194). Kermanshahi et al. have shown that threonine (5 mg/ml) in ovo injection into quail eggs (e11, under the air sac) modulated the MUC2 gene expression post-hatch. At the same time, the injection has no effects on digestive enzyme activity (195). Another study from the same group determined that the lower dose of threonine (5 mg/ml, 50 μl injection volume) increased the mRNA expression level of IgA at hatch (196). The in ovo injection of medical plant extracts (1% solution, e5, air cell) such as garlic, ginger, oregano, or cinnamon has been shown to influence the time of sexual maturity and the quality of early eggs laid by Japanese quails (197). The importance of all-trans retinoic acid, a metabolite of vitamin A in adipose tissue development has been emphasized by its in ovo injection (300 nM) on e9 into quail embryos (198). Additionally, in ovo injection of this metabolite increased mRNA expression of adipogenic markers and decreased expression of preadipocyte markers, suggesting the promotion of adipocyte differentiation and decrease population of preadipocytes (198). In another study, Karagecili and Babacanoglu demonstrated that in ovo injection of vitamin E and ascorbic acid into the yolk sac or amniotic fluid on e5 of quail eggs did not impact hatchability and quail post-hatch development, but it affected the residual yolk sac absorption, total carotene and concentration of vitamin E derivatives with antioxidant characteristics in the newly hatched quails (199).
In ducks, administration of glutamine, β-hydroxy-β-methylbutyrate, and carbohydrates on e23 into the amniotic fluid has been shown to improve small intestine weight, sucrase and maltase activity post-hatch, and increased pectoral muscle weight at e25 (200, 201). In another study, in ovo supplementation of disaccharides and alanyl-glutamine dipeptide on e23 increased plasma glucose concentration post-hatch, liver glycogen content pre-hatch and at hatch, and pectoral glycogen content pre-hatch (202). Additionally, Tangara et al. have shown that in ovo carbohydrates and arginine feeding on e23 led to increased body weight of post-hatch ducklings as well as enhanced liver and muscle glycogen storage pre-hatch (203). The in ovo administration of IGF-1 (100 ng/embryo, e12, into the albumen) resulted in increased body weight, muscle fiber diameter, muscle cross-sectional area, and the number of myofibers per unit area as well as the number of activated satellite cells and mitotic nuclei in leg and breast muscle of post-hatch ducklings. Moreover, the expression level of MyoD and Myf5 was increased in IGF-1 supplemented ducklings (204). In a second study, Liu et al. have shown that e12 supplementation of IGF-1 into the albumen affected the expression level of myogenic transcription factors such as MyoD and MRF4, muscle fiber parameters, and muscle weight during the late stage of duck embryonic development (205). The same researchers also found that in ovo supplementation of follistatin (100 ng/egg, e12) to ducks' embryos affected the expression level of muscle development-related genes. Mainly, Pax7 mRNA expression was upregulated in breast and leg muscle, MyoD mRNA was increased only in leg muscle while Myf5 mRNA was upregulated only in breast muscle. Moreover, the expression of myostatin was downregulated in both muscle types (206). The effects of vitamin C on duck embryos' hatchability were investigated by Nowaczewski et al. (207). They have found that 4 and 8 mg of vitamin C/egg delivered on e20 into the air cell significantly improved the ducks' hatchability by decreasing the number of dead or unhatched embryos (207). Recent data indicate that in ovo feeding of vitamin A, L-carnitine, or folic acid (1 mg/egg, e0, air sac) has positive effects on the embryonic development of duck embryos and the health of newly hatched ducklings (208). Primarily, the embryo weight, residual yolk weight, and hatchability percentage were improved in in ovo supplemented animals, while body weight, blood parameters, and plasma hormone levels were increased at hatch (208).
Discussion and conclusion
As characterized in this review, in ovo supplementation has been extensively studied in broiler chickens. Regarding other avian species, such as turkey, qual, or duck, the current data are much more limited in comparison to broiler chicken studies. Much more research is needed in those species to obtain the full benefits of this technology. Further research is needed in particular to advance our understanding of in ovo technology, particularly the effects of prebiotics and probiotics on intestinal morphological, function, and microbiome development in turkey, duck, quail, and domestic pigeon. Many bioactive substances have been used for injection, with a wide range of physiological responses. The majority of the early studies focused on in ovo feeding to supply the embryo with nutrients to facilitate the hatching process and early post-hatch growth, particularly during the time of delayed access to feed. Only in the past few years, there has been an increase in research, focusing on in ovo stimulation using mostly prebiotics and synbiotics. Even though it seems that the technology has been researched extensively, many factors still remain inconclusive. For example, the site and timing of injections as well as the volume of injections are not standardized for each bioactive substance, and very often, different methodologies lead to inconclusive or unreproducible results. Therefore, standardization efforts should be made in order to provide a bulletproof methodology for in ovo supplementation.
The most efficient way to deliver bioactive substances would be during Marek's disease in ovo vaccination. The data on co-delivery are extremely limited. A study by Beck et al. (209) showed no effect of co-delivery of the Marek's disease vaccine (HVT) and probiotics (Lactobacillus animalis or Enterococcus faecium, e18, amnion, 106 cfu/50 μl volume) on hatchability, performance data, or gastrointestinal parameters (209). The volume of injection as well as the timing and site of delivery need to be taken into consideration for co-delivery. Marek's disease vaccine delivery volume is 50 μl, but for most of the biological substances, much higher volumes are utilized.
Embryo growth, development, and hatchability are affected by many environmental factors and incubation conditions, including egg storage time, incubation temperature, humidity, gas exchange, turning, and light (210, 211). It is clear that any environmental factors compromising embryo development and hatchability will affect the physiological effects of in ovo delivery of biological substances. However, we were unable to locate any specific literature addressing the effect of incubation and hatchability conditions together with in ovo effects. At the same time, in ovo procedure can be one of the factors affecting embryo development and hatching performance (212). However, in most cases, this impact depends on the type of bioactive substances or injection location (213–215). The negative effects are mostly due to the injection process, which leads to a compromised shell and membrane of the egg, allowing for pathogenic bacteria and environmental factors affecting the egg and embryo. The sanitary condition of the injection may lead to compromised eggs and embryos (209). Moghaddam at al. have shown that in ovo injection of saline or bioactive substance (royal jelly) significantly decreased hatchability in comparison to the control group (216). Data from de Oliveira et al. (214) suggest only a 10% decrease in hatchability due to inoculation with probiotics. A decrease in hatchability and embryo mortality was also observed by Melo et al. (217). At the same time, there are numerous data indicating no negative in ovo effects on hatchability and mortality (115, 218–220), decreased embryo mortality (218), or improved hatchability (221, 222).
Clearly, in ovo supplementation with bioactive substances can influence embryonic and post-hatching chick growth, nutrient digestibility, bone development, immune system development, GIT health, GIT microbiota development, heat stress response, and overall health. Even though in ovo supplementation has many advantages, the industry use of the method is solely for vaccine delivery right now. There are many reasons why the poultry industry is currently not interested in this methodology, one of which is the lack of a standardized method for in ovo feeding and stimulation being one of them. Moreover, more research is needed to show the long-term effects of in ovo supplementation and economic advantages for poultry producers. In conclusion, we believe that this method is widely underestimated and underused by the poultry industry. Furthermore, adaptation to commercial settings will require more research efforts and collaborations between researchers and industry partners.
Author contributions
All authors listed have made a substantial, direct, and intellectual contribution to the work and approved it for publication.
Funding
The study was funded by the in-house CRIP project # 8042-31000-114-00D.
Conflict of interest
The authors declare that the research was conducted in the absence of any commercial or financial relationships that could be construed as a potential conflict of interest.
Publisher's note
All claims expressed in this article are solely those of the authors and do not necessarily represent those of their affiliated organizations, or those of the publisher, the editors and the reviewers. Any product that may be evaluated in this article, or claim that may be made by its manufacturer, is not guaranteed or endorsed by the publisher.
References
1. Zuidhof MJ, Schneider BL, Carney VL, Korver DR, Robinson FE. Growth, efficiency, and yield of commercial broilers from 1957, 1978, and 2005. Poult Sci. (2014) 93:2970–82. doi: 10.3382/ps.2014-04291
2. Givisiez PEN, Moreira Filho ALB, Santos MRB, Oliveira HB, Ferket PR, Oliveira CJB, et al. Chicken embryo development: metabolic and morphological basis for in ovo feeding technology. Poult Sci. (2020) 99:6774–82. doi: 10.1016/j.psj.2020.09.074
3. Roto SM, Kwon YM, Ricke SC. Applications of in ovo technique for the optimal development of the gastrointestinal tract and the potential influence on the establishment of its microbiome in poultry. Front Vet Sci. (2016) 3:63. doi: 10.3389/fvets.2016.00063
4. Ferket PR. Embryo Epigenetic Response to Breeder Management and Nutrition. Salvador: World's Poult Congress (2012).
5. Iji PA, Saki A, Tivey DR. Body and intestinal growth of broiler chicks on a commercial starter diet. 1. Intestinal weight and mucosal development. Br Poult Sci. (2001) 42:505–13. doi: 10.1080/00071660120073151
6. de Jong IC, van Riel J, Bracke MBM, van den Brand H. A 'meta-analysis' of effects of post-hatch food and water deprivation on development, performance and welfare of chickens. PLoS ONE. (2017) 12:e0189350. doi: 10.1371/journal.pone.0189350
7. van de Ven LJ, van Wagenberg AV, Debonne M, Decuypere E, Kemp B, van den Brand H. Hatching system and time effects on broiler physiology and posthatch growth. Poult Sci. (2011) 90:1267–75. doi: 10.3382/ps.2010-00876
8. van de Ven LJ, van Wagenberg AV, Decuypere E, Kemp B, van den Brand H. Perinatal broiler physiology between hatching and chick collection in 2 hatching systems. Poult Sci. (2013) 92:1050–61. doi: 10.3382/ps.2012-02534
9. van de Ven LJ, van Wagenberg AV, Uitdehaag KA, Groot Koerkamp PW, Kemp B, van den Brand H. Significance of chick quality score in broiler production. Animal. (2012) 6:1677–83. doi: 10.1017/S1751731112000663
10. Wang Y, Li Y, Willems E, Willemsen H, Franssens L, Koppenol A, et al. Spread of hatch and delayed feed access affect post hatch performance of female broiler chicks up to day 5. Animal. (2014) 8:610–7. doi: 10.1017/S175173111400007X
11. Careghi C, Tona K, Onagbesan O, Buyse J, Decuypere E, Bruggeman V. The effects of the spread of hatch and interaction with delayed feed access after hatch on broiler performance until seven days of age. Poult Sci. (2005) 84:1314–20. doi: 10.1093/ps/84.8.1314
12. Proszkowiec-Weglarz M, Miska KB, Ellestad LE, Schreier LL, Kahl S, Darwish N, et al. Delayed access to feed early post-hatch affects the development and maturation of gastrointestinal tract microbiota in broiler chickens. BMC Microbiol. (2022) 22:206. doi: 10.1186/s12866-022-02619-6
13. Proszkowiec-Weglarz M, Schreier LL, Kahl S, Miska KB, Russell B, Elsasser TH. Effect of delayed feeding post-hatch on expression of tight junction- and gut barrier-related genes in the small intestine of broiler chickens during neonatal development. Poult Sci. (2020) 99:4714–29. doi: 10.1016/j.psj.2020.06.023
14. Proszkowiec-Weglarz M, Schreier LL, Miska KB, Angel R, Kahl S, Russell B. Effect of early neonatal development and delayed feeding post-hatch on jejunal and ileal calcium and phosphorus transporter genes expression in broiler chickens. Poult Sci. (2019) 98:1861–71. doi: 10.3382/ps/pey546
15. Qu Y, Kahl S, Miska KB, Schreier LL, Russell B, Elsasser TH, et al. The effect of delayed feeding post-hatch on caeca development in broiler chickens. Br Poult Sci. (2021) 62:731–48. doi: 10.1080/00071668.2021.1912291
16. Shehata AM, Paswan VK, Attia YA, Abdel-Moneim A-ME, Abougabal MS, Sharaf M, et al. Managing gut microbiota through in ovo nutrition influences early-life programming in broiler chickens. Animals. (2021) 11:3491. doi: 10.3390/ani11123491
17. Sharma JM, Burmester BR. Resistance of Marek's disease at hatching in chickens vaccinated as embryos with the turkey herpesvirus. Avian Dis. (1982) 26:134–49. doi: 10.2307/1590032
18. Uni Z, Ferket PR. Enhancement of Development of Oviparous Species by in ovo Feeding. (2003). Patent number US 6592878 b2.
19. Dunislawska A, Slawinska A, Siwek M, Bednarczyk M. Epigenetic changes in poultry due to reprogramming of the gut microbiota. Anim Front. (2021) 11:74–82. doi: 10.1093/af/vfab063
20. Uni Z, Ferket RP. Methods for early nutrition and their potential. World's Poult Sci J. (2004) 60:101–11. doi: 10.1079/WPS20038
21. Das R, Mishra P, Jha R. In ovo feeding as a tool for improving performance and gut health of poultry: a review. Front Vet Sci. (2021) 8:754246. doi: 10.3389/fvets.2021.754246
22. McGruder BM, Zhai W, Keralapurath MM, Bennett LW, Gerard PD, Peebles ED. Effects of in ovo injection of electrolyte solutions on the pre- and posthatch physiological characteristics of broilers. Poult Sci. (2011) 90:1058–66. doi: 10.3382/ps.2010-00893
23. Zhai W, Rowe DE, Peebles ED. Effects of commercial in ovo injection of carbohydrates on broiler embryogenesis. Poult Sci. (2011) 90:1295–301. doi: 10.3382/ps.2010-01130
24. Dankowiakowska A, Bogucka J, Sobolewska A, Tavaniello S, Maiorano G, Bednarczyk M. Effects of in ovo injection of prebiotics and synbiotics on the productive performance and microstructural features of the superficial pectoral muscle in broiler chickens. Poult Sci. (2019) 98:5157–65. doi: 10.3382/ps/pez202
25. Bednarczyk M, Stadnicka K, Kozłowska I, Abiuso C, Tavaniello S, Dankowiakowska A, et al. Influence of different prebiotics and mode of their administration on broiler chicken performance. Animal. (2016) 10:1271–9. doi: 10.1017/S1751731116000173
26. Tong Q, Romanini CE, Exadaktylos V, Bahr C, Berckmans D, Bergoug H, et al. Embryonic development and the physiological factors that coordinate hatching in domestic chickens. Poult Sci. (2013) 92:620–8. doi: 10.3382/ps.2012-02509
27. Uni Z, Yadgary L, Yair R. Nutritional limitations during poultry embryonic development. J Appl Poult Res. (2012) 21:175–84. doi: 10.3382/japr.2011-00478
28. Grodzik M, Sawosz F, Sawosz E, Hotowy A, Wierzbicki M, Kutwin M, et al. Nano-nutrition of chicken embryos. The effect of in ovo administration of diamond nanoparticles and l-glutamine on molecular responses in chicken embryo pectoral muscles. Int J Mol Sci. (2013) 14:23033–44. doi: 10.3390/ijms141123033
29. Ohta Y, Kidd MT, Ishibashi T. Embryo growth and amino acid concentration profiles of broiler breeder eggs, embryos, and chicks after in ovo administration of amino acids. Poult Sci. (2001) 80:1430–6. doi: 10.1093/ps/80.10.1430
30. Moran ET Jr. Nutrition of the developing embryo and hatchling. Poult Sci. (2007) 86:1043–9. doi: 10.1093/ps/86.5.1043
31. Pulikanti R, Peebles ED, Keirs RW, Bennett LW, Keralapurath MM, Gerard PD. Pipping muscle and liver metabolic profile changes and relationships in broiler embryos on days 15 and 19 of incubation. Poult Sci. (2010) 89:860–5. doi: 10.3382/ps.2009-00531
32. Uni Z, Ferket PR, Tako E, Kedar O. In ovo feeding improves energy status of late-term chicken embryos. Poult Sci. (2005) 84:764–70. doi: 10.1093/ps/84.5.764
33. Prelusky DB, Hamilton RM, Trenholm HL. Transmission of residues to eggs following long-term administration of 14c-labelled deoxynivalenol to laying hens. Poult Sci. (1989) 68:744–8. doi: 10.3382/ps.0680744
34. Wang Y, Quan H, Li X, Li Q, Haque MA, Shi Q, et al. Contamination with fumonisin b and deoxynivalenol is a threat to egg safety and contributes to gizzard ulcerations of newborn chickens. Front Microbiol. (2021) 12:676671. doi: 10.3389/fmicb.2021.676671
35. Oznurlu Y, Celik I, Sur E, Ozaydin T, Oguz H, Altunbaş K. Determination of the effects of aflatoxin b1 given in ovo on the proximal tibial growth plate of broiler chickens: histological, histometric and immunohistochemical findings. Avian Pathol. (2012) 41:469–77. doi: 10.1080/03079457.2012.712673
36. Elwan H, Mohamed ASA, Dawood DH, Elnesr SS. Modulatory effects of arctostaphylos uva-urs extract in ovo injected into broiler embryos contaminated by aflatoxin b1. Animals. (2022) 12:2042. doi: 10.3390/ani12162042
37. Elwan H, Xie C, Miao LP, Dong X, Zou XT, Mohany M, et al. Methionine alleviates aflatoxinb1-induced broiler chicks embryotoxicity through inhibition of caspase-dependent apoptosis and enhancement of cellular antioxidant status. Poult Sci. (2021) 100:101103. doi: 10.1016/j.psj.2021.101103
38. Alcântara D, Rodrigues MN, Franciolli AL, Da Fonseca ET, Silva FM, Carvalho RC, et al. Embryonic development of endoderm in chicken (Gallus gallus domesticus). Microsc Res Tech. (2013) 76:803–10. doi: 10.1002/jemt.22232
39. Uni Z, Tako E, Gal-Garber O, Sklan D. Morphological, molecular, and functional changes in the chicken small intestine of the late-term embryo. Poult Sci. (2003) 82:1747–54. doi: 10.1093/ps/82.11.1747
40. Ikeno T, Ikeno K. Amylase activity increases in the yolk of fertilized eggs during incubation in chickens. Poult Sci. (1991) 70:2176–9. doi: 10.3382/ps.0702176
41. Reicher N, Uni Z. Intestinal brush border assembly during the peri-hatch period and its contribution to surface area expansion. Poult Sci. (2021) 100:101401. doi: 10.1016/j.psj.2021.101401
42. De Oliveira JE, Uni Z, Ferket PR. Important metabolic pathways in poultry embryos prior to hatch. World's Poult Sci J. (2008) 64:488–99. doi: 10.1017/S0043933908000160
43. Moreira Filho ALdB, Ferket PR, Malheiros RD, Oliveira CJB, Aristimunha PC, Wilsmann DE, et al. Enrichment of the amnion with threonine in chicken embryos affects the small intestine development, ileal gene expression and performance of broilers between 1 and 21 days of age. Poult Sci. (2019) 98:1363–70. doi: 10.3382/ps/pey461
44. Moreira Filho ALdB, Oliveira CJ, Freitas Neto OC, de Leon CM, Saraiva M, Andrade MF, et al. Intra-amnionic threonine administered to chicken embryos reduces enteritidis cecal counts and improves posthatch intestinal development. J Immunol Res. (2018) 2018:9795829. doi: 10.1155/2018/9795829
45. Gao T, Zhao MM Li YJ, Zhang L, Li JL Yu LL, et al. Effects of in ovo feeding of l-arginine on the development of digestive organs, intestinal function and post-hatch performance of broiler embryos and hatchlings. J Anim Physiol Anim Nutr. (2018) 102:e166–e75. doi: 10.1111/jpn.12724
46. Dai D, Wu SG, Zhang HJ Qi GH, Wang J. Dynamic alterations in early intestinal development, microbiota and metabolome induced by in ovo feeding of l-arginine in a layer chick model. J Anim Sci Biotechnol. (2020) 11:19. doi: 10.1186/s40104-020-0427-5
47. Gao T, Zhao M, Zhang L, Li J, Yu L, Gao F, et al. In ovo feeding of L-arginine regulates intestinal barrier functions of posthatch broilers by activating the MTOR signaling pathway. J Sci Food Agric. (2018) 98:1416–25. doi: 10.1002/jsfa.8609
48. Nazem MN, Amiri N, Tasharrofi S. Effect of in ovo feeding of amino acids and dextrose solutions on hatchability, body weight, intestinal development and liver glycogen reserves in newborn chicks. Vet Res Forum. (2019) 10:323–31. doi: 10.30466/vrf.2018.69536.1956
49. Reicher N, Melkman-Zehavi T, Dayan J, Wong EA, Uni Z. Nutritional stimulation by in-ovo feeding modulates cellular proliferation and differentiation in the small intestinal epithelium of chicks. Anim Nutr. (2022) 8:91–101. doi: 10.1016/j.aninu.2021.06.010
50. Cheled-Shoval SL, Amit-Romach E, Barbakov M, Uni Z. The effect of in ovo administration of mannan oligosaccharide on small intestine development during the pre- and posthatch periods in chickens. Poult Sci. (2011) 90:2301–10. doi: 10.3382/ps.2011-01488
51. Berrocoso JD Kida R, Singh AK, Kim YS, Jha R. Effect of in ovo injection of raffinose on growth performance and gut health parameters of broiler chicken. Poult Sci. (2017) 96:1573–80. doi: 10.3382/ps/pew430
52. Pacifici S, Song J, Zhang C, Wang Q, Glahn RP, Kolba N, et al. Intra amniotic administration of raffinose and stachyose sffects the intestinal brush border functionality and alters gut microflora populations. Nutrients. (2017) 9:304. doi: 10.3390/nu9030304
53. Bogucka J, Dankowiakowska A, Elminowska-Wenda G, Sobolewska A, Szczerba A, Bednarczyk M. Effects of prebiotics and synbiotics delivered in ovo on broiler small intestine histomorphology during the first days after hatching. Folia Biol. (2016) 64:131–43. doi: 10.3409/fb64_3.131
54. Calik A, Ceylan A, Ekim B, Adabi SG, Dilber F, Bayraktaroglu AG, et al. The effect of intra-amniotic and posthatch dietary synbiotic administration on the performance, intestinal histomorphology, cecal microbial population, and short-chain fatty acid composition of broiler chickens. Poult Sci. (2017) 96:169–83. doi: 10.3382/ps/pew218
55. Alkie TN, Yitbarek A, Hodgins DC, Kulkarni RR, Taha-Abdelaziz K, Sharif S. Development of innate immunity in chicken embryos and newly hatched chicks: a disease control perspective. Avian Pathol. (2019) 48:288–310. doi: 10.1080/03079457.2019.1607966
56. Panda AK, Bhanja SK, Sunder GS. Early post hatch nutrition on immune system development and function in broiler chickens. World's Poult Sci J. (2015) 71:285–96. doi: 10.1017/S004393391500029X
57. Kajiwara E, Shigeta A, Horiuchi H, Matsuda H, Furusawa S. Development of Peyer's patch and cecal tonsil in gut-associated lymphoid tissues in the chicken embryo. J Vet Med Sci. (2003) 65:607–14. doi: 10.1292/jvms.65.607
58. Kannaki TR, Reddy MR, Verma PC, Shanmugam M. Differential toll-like receptor (TLR) mRNA expression patterns during chicken embryological development. Anim Biotechnol. (2015) 26:130–5. doi: 10.1080/10495398.2014.939658
59. Eren U, Kum S, Nazligul A, Gules O, Aka E, Zorlu S, et al. The several elements of intestinal innate immune system at the beginning of the life of broiler chicks. Microsc Res Tech. (2016) 79:604–14. doi: 10.1002/jemt.22674
60. Reemers SS, van Leenen D, Koerkamp MJ, van Haarlem D, van de Haar P, van Eden W, et al. Early host responses to avian influenza a virus are prolonged and enhanced at transcriptional level depending on maturation of the immune system. Mol Immunol. (2010) 47:1675–85. doi: 10.1016/j.molimm.2010.03.008
61. Bar-Shira E, Friedman A. Development and adaptations of innate immunity in the gastrointestinal tract of the newly hatched chick. Dev Comp Immunol. (2006) 30:930–41. doi: 10.1016/j.dci.2005.12.002
62. Dibner J, Knight C, Kitchell M, Atwell C, Downs A, Ivey F. Early feeding and development of the immune system in neonatal poultry. J Appl Poult Res. (1998) 7:425–36. doi: 10.1093/japr/7.4.425
63. Slawinska A, Dunislawska A, Plowiec A, Radomska M, Lachmanska J, Siwek M, et al. Modulation of microbial communities and mucosal gene expression in chicken intestines after galactooligosaccharides delivery in ovo. PLoS ONE. (2019) 14:e0212318. doi: 10.1371/journal.pone.0212318
64. Sławinska A, Siwek MZ, Bednarczyk MF. Effects of synbiotics injected in ovo on regulation of immune-related gene expression in adult chickens. Am J Vet Res. (2014) 75:997–1003. doi: 10.2460/ajvr.75.11.997
65. Płowiec A, Sławińska A, Siwek MZ, Bednarczyk MF. Effect of in ovo administration of inulin and Lactococcus lactis on immune-related gene expression in broiler chickens. Am J Vet Res. (2015) 76:975–82. doi: 10.2460/ajvr.76.11.975
66. Madej JP, Skonieczna J, Siwek M, Kowalczyk A, Łukaszewicz E, Slawinska A. Genotype-dependent development of cellular and humoral immunity in the spleen and cecal tonsils of chickens stimulated in ovo with bioactive compounds. Poult Sci. (2020) 99:4343–50. doi: 10.1016/j.psj.2020.05.048
67. Szczypka M, Suszko-Pawłowska A, Kuczkowski M, Gorczykowski M, Lis M, Kowalczyk A, et al. Effects of selected prebiotics or synbiotics administered in ovo on lymphocyte subsets in bursa of the fabricius, thymus, and spleen in non-immunized and immunized chicken broilers. Animals. (2021) 11:476. doi: 10.3390/ani11020476
68. Goel A, Bhanja SK, Mehra M, Mandal A, Pande V. In ovo trace element supplementation enhances expression of growth genes in embryo and immune genes in post-hatch broiler chickens. J Sci Food Agric. (2016) 96:2737–45. doi: 10.1002/jsfa.7438
69. Stefaniak T, Madej JP, Graczyk S, Siwek M, Łukaszewicz E, Kowalczyk A, et al. Selected prebiotics and synbiotics administered in ovo can modify innate immunity in chicken broilers. BMC Vet Res. (2019) 15:105. doi: 10.1186/s12917-019-1850-8
70. Abbasi T, Shakeri M, Zaghari M, Kohram H. Growth performance parameters, bone calcification and immune response of in ovo injection of 25-hydroxycholecalciferol and vitamin k(3) in male ross 308 broilers. Theriogenology. (2017) 90:260–5. doi: 10.1016/j.theriogenology.2016.12.016
71. Lee SH, Lillehoj HS, Jang SI, Jeong M, Kim DK, Xu S, et al. Immune and anti-oxidant effects of in ovo selenium proteinate on post-hatch experimental avian necrotic enteritis. Vet Parasitol. (2014) 206:115–22. doi: 10.1016/j.vetpar.2014.10.025
72. Lee SH, Lillehoj HS, Jang SI, Jeong MS, Xu SZ, Kim JB, et al. Effects of in ovo injection with selenium on immune and antioxidant responses during experimental necrotic enteritis in broiler chickens. Poult Sci. (2014) 93:1113–21. doi: 10.3382/ps.2013-03770
73. Millay DP, O'Rourke JR, Sutherland LB, Bezprozvannaya S, Shelton JM, Bassel-Duby R, et al. Myomaker is a membrane activator of myoblast fusion and muscle formation. Nature. (2013) 499:301–5. doi: 10.1038/nature12343
74. Gu S, Wen C, Li J, Liu H, Huang Q, Zheng J, et al. Temporal expression of myogenic regulatory genes in different chicken breeds during embryonic development. Int J Mol Sci. (2022) 23:10115. doi: 10.3390/ijms231710115
75. Scheuermann GN, Bilgili SF, Hess JB, Mulvaney DR. Breast muscle development in commercial broiler chickens. Poult Sci. (2003) 82:1648–58. doi: 10.1093/ps/82.10.1648
76. Bogucka J, Dankowiakowska A, Stanek M, Stadnicka K, Kirkiłło-Stacewicz K. Effect of synbiotics administered in ovo on microvascularization and histopathological changes in pectoral muscle and the biochemical profile of broiler chicken blood. Poult Sci. (2022) 101:101628. doi: 10.1016/j.psj.2021.101628
77. Zhao MM, Gao T, Zhang L, Li JL, Lv PA Yu LL, et al. Effects of in ovo feeding of creatine pyruvate on the hatchability, growth performance and energy status in embryos and broiler chickens. Animal. (2017) 11:1689–97. doi: 10.1017/S1751731117000374
78. Stadnicka K, Bogucka J, Stanek M, Graczyk R, Krajewski K, Maiorano G, et al. Injection of raffinose family oligosaccharides at 12 days of egg incubation modulates the gut development and resistance to opportunistic pathogens in broiler chickens. Animals. (2020) 10:592. doi: 10.3390/ani10040592
79. Stasiak K, Slawinska A, Bogucka J. Effects of probiotics, prebiotics and synbiotics injected in ovo on the microstructure of the breast muscle in different chicken genotypes. Animals. (2021) 11:2944. doi: 10.3390/ani11102944
80. Li Y, Wang Y, Willems E, Willemsen H, Franssens L, Buyse J, et al. In ovo L-arginine supplementation stimulates myoblast differentiation but negatively affects muscle development of broiler chicken after hatching. J Anim Physiol Anim Nutr. (2016) 100:167–77. doi: 10.1111/jpn.12299
81. Torres CA, Korver DR. Influences of trace mineral nutrition and maternal flock age on broiler embryo bone development. Poult Sci. (2018) 97:2996–3003. doi: 10.3382/ps/pey136
82. Olsen BR, Reginato AM, Wang W. Bone development. Ann Rev Cell Dev Biol. (2000) 16:191–220. doi: 10.1146/annurev.cellbio.16.1.191
83. Pechak DG, Kujawa MJ, Caplan AI. Morphological and histochemical events during first bone formation in embryonic chick limbs. Bone. (1986) 7:441–58. doi: 10.1016/8756-3282(86)90004-9
84. Shapiro F. Vertebral development of the chick embryo during days 3–19 of incubation. J Morphol. (1992) 213:317–33. doi: 10.1002/jmor.1052130305
85. Halgrain M, Bernardet N, Crepeau M, Même N, Narcy A, Hincke M, et al. Eggshell decalcification and skeletal mineralization during chicken embryonic development: defining candidate genes in the chorioallantoic membrane. Poult Sci. (2022) 101:101622. doi: 10.1016/j.psj.2021.101622
86. Yair R, Uni Z, Shahar R. Bone characteristics of late-term embryonic and hatchling broilers: bone development under extreme growth rate. Poult Sci. (2012) 91:2614–20. doi: 10.3382/ps.2012-02244
87. Yair R, Uni Z. Content and uptake of minerals in the yolk of broiler embryos during incubation and effect of nutrient enrichment. Poult Sci. (2011) 90:1523–31. doi: 10.3382/ps.2010-01283
88. Dibner JJ, Richards JD, Kitchell ML, Quiroz MA. Metabolic challenges and early bone development. J Appl Poult Res. (2007) 16:126–37. doi: 10.1093/japr/16.1.126
89. Tuan RS, Ono T. Regulation of extraembryonic calcium mobilization by the developing chick embryo. J Embryol Exp Morphol. (1986) 97:63–74. doi: 10.1242/dev.97.1.63
90. Yair R, Shahar R, Uni Z. In ovo feeding with minerals and vitamin d3 improves bone properties in hatchlings and mature broilers. Poult Sci. (2015) 94:2695–707. doi: 10.3382/ps/pev252
91. Oliveira TF, Bertechini AG, Bricka RM, Hester PY, Kim EJ, Gerard PD, et al. Effects of in ovo injection of organic trace minerals and post-hatch holding time on broiler performance and bone characteristics. Poult Sci. (2015) 94:2677–85. doi: 10.3382/ps/pev249
92. Bello A, Bricka RM, Gerard PD, Peebles ED. Effects of commercial in ovo injection of 25-hydroxycholecalciferol on broiler bone development and mineralization on days 0 and 21 posthatch. Poult Sci. (2014) 93:1053–8. doi: 10.3382/ps.2013-03608
93. Oliveira TFB, Bertechini AG, Bricka RM, Kim EJ, Gerard PD, Peebles ED. Effects of in ovo injection of organic zinc, manganese, and copper on the hatchability and bone parameters of broiler hatchlings. Poult Sci. (2015) 94:2488–94. doi: 10.3382/ps/pev248
94. Dietz MW, Salles JF, Hsu BY, Dijkstra C, Groothuis TGG, van der Velde M, et al. Prenatal transfer of gut bacteria in rock pigeon. Microorganisms. (2019) 8:61. doi: 10.3390/microorganisms8010061
95. Stanley D, Hughes RJ, Moore RJ. Microbiota of the chicken gastrointestinal tract: influence on health, productivity and disease. Appl Microbiol Biotechnol. (2014) 98:4301–10. doi: 10.1007/s00253-014-5646-2
96. Schokker D, Veninga G, Vastenhouw SA, Bossers A, de Bree FM, Kaal-Lansbergen LM, et al. Early life microbial colonization of the gut and intestinal development differ between genetically divergent broiler lines. BMC Genom. (2015) 16:418. doi: 10.1186/s12864-015-1646-6
97. Clavijo V, Florez MJV. The gastrointestinal microbiome and its association with the control of pathogens in broiler chicken production: a review. Poult Sci. (2018) 97:1006–21. doi: 10.3382/ps/pex359
98. Chambers JR, Gong J. The intestinal microbiota and its modulation for salmonella control in chickens. Food Res Int. (2011) 44:3149–59. doi: 10.1016/j.foodres.2011.08.017
99. Pan D, Yu Z. Intestinal microbiome of poultry and its interaction with host and diet. Gut Microbes. (2014) 5:108–19. doi: 10.4161/gmic.26945
100. Zhao L, Wang G, Siegel P, He C, Wang H, Zhao W, et al. Quantitative genetic background of the host influences gut microbiomes in chickens. Sci Rep. (2013) 3:1163. doi: 10.1038/srep01163
101. Oakley BB, Lillehoj HS, Kogut MH, Kim WK, Maurer JJ, Pedroso A, et al. The chicken gastrointestinal microbiome. FEMS Microbiol Lett. (2014) 360:100–12. doi: 10.1111/1574-6968.12608
102. Huang P, Zhang Y, Xiao K, Jiang F, Wang H, Tang D, et al. The chicken gut metagenome and the modulatory effects of plant-derived benzylisoquinoline alkaloids. Microbiome. (2018) 6:211. doi: 10.1186/s40168-018-0590-5
103. Lan Y, Verstegen MWA, Tamminga S, Williams BA. The role of the commensal gut microbial community in broiler chickens. World's Poult Sci J. (2005) 61:95–104. doi: 10.1079/WPS200445
104. Pedroso AA, Batal AB, Lee MD. Effect of in ovo administration of an adult-derived microbiota on establishment of the intestinal microbiome in chickens. Am J Vet Res. (2016) 77:514–26. doi: 10.2460/ajvr.77.5.514
105. Majidi-Mosleh A, Sadeghi AA, Mousavi SN, Chamani M, Zarei A. Ileal muc2 gene expression and microbial population, but not growth performance and immune response, are influenced by in ovo injection of probiotics in broiler chickens. Br Poult Sci. (2017) 58:40–5. doi: 10.1080/00071668.2016.1237766
106. Teague KD, Graham LE, Dunn JR, Cheng HH, Anthony N, Latorre JD, et al. In ovo evaluation of floramax(r)-b11 on marek's disease HVT vaccine protective efficacy, hatchability, microbiota composition, morphometric analysis, and salmonella enteritidis infection in broiler chickens. Poult Sci. (2017) 96:2074–82. doi: 10.3382/ps/pew494
107. Abdel-Moneim A-ME, Elbaz AM, Khidr RE-S, Badri FB. Effect of in ovo inoculation of Bifidobacterium spp. On growth performance, thyroid activity, ileum histomorphometry, and microbial enumeration of broilers. Prob Antimicrob Prot. (2020) 12:873–82. doi: 10.1007/s12602-019-09613-x
108. Wilson KM, Rodrigues DR, Briggs WN, Duff AF, Chasser KM, Bielke LR. Evaluation of the impact of in ovo administered bacteria on microbiome of chicks through 10 days of age. Poult Sci. (2019) 98:5949–60. doi: 10.3382/ps/pez388
109. Rodrigues DR, Winson E, Wilson KM, Briggs WN, Duff AF, Chasser KM, et al. Intestinal pioneer colonizers as drivers of ileal microbial composition and diversity of broiler chickens. Front Microbiol. (2020) 10:2858. doi: 10.3389/fmicb.2019.02858
110. Arreguin-Nava MA, Graham BD, Adhikari B, Agnello M, Selby CM, Hernandez-Velasco X, et al. In ovo administration of defined lactic acid bacteria previously isolated from adult hens induced variations in the cecae microbiota structure and enterobacteriaceae colonization on a virulent Escherichia coli horizontal infection model in broiler chickens. Front Vet Sci. (2020) 7:489. doi: 10.3389/fvets.2020.00489
111. Fuller R. Probiotics in man and animals. J Appl Bacteriol. (1989) 66:365–78. doi: 10.1111/j.1365-2672.1989.tb05105.x
112. Gibson GR, Roberfroid MB. Dietary modulation of the human colonic microbiota: introducing the concept of prebiotics. J Nutr. (1995) 125:1401–12. doi: 10.1093/jn/125.6.1401
113. Dunislawska A, Slawinska A, Stadnicka K, Bednarczyk M, Gulewicz P, Jozefiak D, et al. Synbiotics for broiler chickens-in vitro design and evaluation of the influence on host and selected microbiota populations following in ovo delivery. PLoS ONE. (2017) 12:e0168587. doi: 10.1371/journal.pone.0168587
114. Zhang J, Cai K, Mishra R, Jha R. In ovo supplementation of chitooligosaccharide and chlorella polysaccharide affects cecal microbial community, metabolic pathways, and fermentation metabolites in broiler chickens. Poult Sci. (2020) 99:4776–85. doi: 10.1016/j.psj.2020.06.061
115. Omidi S, Ebrahimi M, Janmohammadi H, Moghaddam G, Rajabi Z, Hosseintabar-Ghasemabad B. The impact of in ovo injection of l-arginine on hatchability, immune system and caecum microflora of broiler chickens. J Anim Physiol Anim Nutr. (2020) 104:178–85. doi: 10.1111/jpn.13222
116. Cuperus T, Kraaij MD, Zomer AL, van Dijk A, Haagsman HP. Immunomodulation and effects on microbiota after in ovo administration of chicken cathelicidin-2. PLoS ONE. (2018) 13:e0198188. doi: 10.1371/journal.pone.0198188
117. Cheng J, Kolba N, Sisser P, Turjeman S, Even C, Koren O, et al. Intraamniotic administration (Gallus gallus) of genistein alters mineral transport, intestinal morphology, and gut microbiota. Nutrients. (2022) 14:3473. doi: 10.3390/nu14173473
118. Yadgary L, Uni Z. Yolk sac carbohydrate levels and gene expression of key gluconeogenic and glycogenic enzymes during chick embryonic development. Poult Sci. (2012) 91:444–53. doi: 10.3382/ps.2011-01669
119. van der Wagt I, de Jong IC, Mitchell MA, Molenaar R, van den Brand H. A review on yolk sac utilization in poultry. Poult Sci. (2020) 99:2162–75. doi: 10.1016/j.psj.2019.11.041
120. Schmidt CJ, Persia ME, Feierstein E, Kingham B, Saylor WW. Comparison of a modern broiler line and a heritage line unselected since the 1950s. Poult Sci. (2009) 88:2610–9. doi: 10.3382/ps.2009-00055
121. Zhang L, Zhu XD, Wang XF Li JL, Gao F, Zhou GH. Individual and combined effects of in-ovo injection of creatine monohydrate and glucose on somatic characteristics, energy status, and post-hatch performance of broiler embryos and hatchlings. Poult Sci. (2016) 95:2352–9. doi: 10.3382/ps/pew130
122. Gopi M, Manojkumar V, Verma AK, Singh P, Rokade JJ, Pearlin BV, et al. In ovo administration of nucleosides improved the performance, apparent metabolizable energy and gut development in broiler chickens. Front Vet Sci. (2020) 7:583748. doi: 10.3389/fvets.2020.583748
123. Zhao MM, Gao T, Zhang L, Li JL, Lv PA Yu LL, et al. In ovo feeding of creatine pyruvate alters energy reserves, satellite cell mitotic activity and myogenic gene expression of breast muscle in embryos and neonatal broilers. Poult Sci. (2017) 96:3314–23. doi: 10.3382/ps/pex150
124. Zhao MM, Gong DQ, Gao T, Zhang L, Li JL, Lv PA, et al. In ovo feeding of creatine pyruvate modulates growth performance, energy reserves and mRNA expression levels of gluconeogenesis and glycogenesis enzymes in liver of embryos and neonatal broilers. J Anim Physiol Anim Nutr. (2018) 102:E758–E67. doi: 10.1111/jpn.12831
125. Dunislawska A, Siwek M, Slawinska A, Lepczynski A, Herosimczyk A, Kolodziejski PA, et al. Metabolic gene expression in the muscle and blood parameters of broiler chickens stimulated in ovo with synbiotics. Animals. (2020) 10:687. doi: 10.3390/ani10040687
126. Martens EC, Neumann M, Desai MS. Interactions of commensal and pathogenic microorganisms with the intestinal mucosal barrier. Nat Rev Microbiol. (2018) 16:457–70. doi: 10.1038/s41579-018-0036-x
127. Lee B, Moon KM, Kim CY. Tight junction in the intestinal epithelium: its association with diseases and regulation by phytochemicals. J Immunol Res. (2018) 2018:2645465. doi: 10.1155/2018/2645465
128. Jha R, Singh AK, Yadav S, Berrocoso JFD, Mishra B. Early nutrition programming (in ovo and post-hatch feeding) as a strategy to modulate gut health of poultry. Front Vet Sci. (2019) 6:82. doi: 10.3389/fvets.2019.00082
129. Nazem MN, Sajjadian SM, Kheirandish R, Mohammadrezaei H. Histomorphometric analysis of the small intestine of broiler chick embryos injected in ovo with methionine. Anim Prod Sci. (2019) 59:133–9. doi: 10.1071/AN17269
130. Wang JG, Lin J, Wang J, Wu SG Qi GH, Zhang HJ, et al. Effects of in ovo feeding of n-acetyl-l-glutamate on early intestinal development and growth performance in broiler chickens. Poult Sci. (2020) 99:3583–93. doi: 10.1016/j.psj.2020.04.003
131. Szentkuti L, Lorenz K. The thickness of the mucus layer in different segments of the rat intestine. Histochem J. (1995) 27:466–72. doi: 10.1007/BF02388803
132. Uni Z. Early development of small intestinal function. Avian Gut Funct Health Dis. (2006) 28:29–42. doi: 10.1079/9781845931803.0029
133. da Silva ABS, Fonseca CMB, Cavalcante M, de Oliveira IM, Ferraz MS, Viana FJC, et al. Histomorphometry of pancreas development in hybrid chicken (Galus galus) embryo and fetus. Microsc Res Tech. (2018) 81:614–23. doi: 10.1002/jemt.23016
134. Nir I, Nitsan Z, Mahagna M. Comparative growth and development of the digestive organs and of some enzymes in broiler and egg type chicks after hatching. Br Poult Sci. (1993) 34:523–32. doi: 10.1080/00071669308417607
135. Pruszynska-Oszmalek E, Kolodziejski PA, Stadnicka K, Sassek M, Chalupka D, Kuston B, et al. In ovo injection of prebiotics and synbiotics affects the digestive potency of the pancreas in growing chickens. Poult Sci. (2015) 94:1909–16. doi: 10.3382/ps/pev162
136. Holmes R, Lobley RW. Intestinal brush border revisited. Gut. (1989) 30:1667–78. doi: 10.1136/gut.30.12.1667
137. Andrade MDFDS, Moreira Filho ALDB, Alves da Silva EF, Silva JHVD, Freitas Neto OCD, de Oliveira CJB, et al. In ovo threonine supplementation affects ileal gene expression of nutrient transporters in broilers inoculated post-hatch with salmonella enteritidis. J Anim Physiol Anim Nutr. (2022) 106:395–402. doi: 10.1111/jpn.13672
138. Bogucka J, Dankowiakowska A, Elminowska-Wenda G, Sobolewska A, Jankowski J, Szpinda M, et al. Performance and small intestine morphology and ultrastructure of male broilers injected in ovo with bioactive substances. Ann Anim Sci. (2017) 17:179–95. doi: 10.1515/aoas-2016-0048
139. Arreguin-Nava MA, Graham BD, Adhikari B, Agnello M, Selby CM, Hernandez-Velasco X, et al. Evaluation of in ovo Bacillus spp. based probiotic administration on horizontal transmission of virulent Escherichia coli in neonatal broiler chickens. Poult Sci. (2019) 98:6483–91. doi: 10.3382/ps/pez544
140. Pender CM, Kim S, Potter TD, Ritzi MM, Young M, Dalloul RA. In ovo supplementation of probiotics and its effects on performance and immune-related gene expression in broiler chicks. Poult Sci. (2017) 96:1052–62. doi: 10.3382/ps/pew381
141. Kim HJ, Kang HK. Effects of in ovo injection of zinc or diet supplementation of zinc on performance, serum biochemical profiles, and meat quality in broilers. Animals. (2022) 12:630. doi: 10.3390/ani12050630
142. Maiorano G, Sobolewska A, Cianciullo D, Walasik K, Elminowska-Wenda G, Slawinska A, et al. Influence of in ovo prebiotic and synbiotic administration on meat quality of broiler chickens. Poult Sci. (2012) 91:2963–9. doi: 10.3382/ps.2012-02208
143. Duan AY, Ju AQ, Zhang YN, Qin YJ, Xue LG, Ma X, et al. The effects of in ovo injection of synbiotics on the early growth performance and intestinal health of chicks. Front Vet Sci. (2021) 8:658301. doi: 10.3389/fvets.2021.658301
144. Zhang N. Epigenetic modulation of DNA methylation by nutrition and its mechanisms in animals. Anim Nutr. (2015) 1:144–51. doi: 10.1016/j.aninu.2015.09.002
145. Hing B, Braun P, Cordner ZA, Ewald ER, Moody L, McKane M, et al. Chronic social stress induces DNA methylation changes at an evolutionary conserved intergenic region in chromosome x. Epigenetics. (2018) 13:627–41. doi: 10.1080/15592294.2018.1486654
146. Ansari I, Raddatz G, Gutekunst J, Ridnik M, Cohen D, Abu-Remaileh M, et al. The microbiota programs DNA methylation to control intestinal homeostasis and inflammation. Nat Microbiol. (2020) 5:610–9. doi: 10.1038/s41564-019-0659-3
147. Dunislawska A, Slawinska A, Siwek M. Hepatic DNA methylation in response to early stimulation of microbiota with lactobacillus synbiotics in broiler chickens. Genes. (2020) 11:579. doi: 10.3390/genes11050579
148. Dunislawska A, Slawinska A, Gryzinska M, Siwek M. Interaction between early in ovo stimulation of the gut microbiota and chicken host-splenic changes in gene expression and methylation. J Anim Sci Biotechnol. (2021) 12:73. doi: 10.1186/s40104-021-00602-1
149. Sikorska M, Siwek M, Slawinska A, Dunislawska A. Mirna profiling in the chicken liver under the influence of early microbiota stimulation with probiotic, prebiotic, and synbiotic. Genes. (2021) 12:685. doi: 10.3390/genes12050685
150. Zhu Y, Li S, Duan Y, Ren Z, Yang X, Yang X. Effects of in ovo feeding of vitamin c on post-hatch performance, immune status and DNA methylation-related gene expression in broiler chickens. Br J Nutr. (2020) 124:903–11. doi: 10.1017/S000711452000210X
151. Slawinska A, Plowiec A, Siwek M, Jaroszewski M, Bednarczyk M. Long-term transcriptomic effects of prebiotics and synbiotics delivered in ovo in broiler chickens. PLoS ONE. (2016) 11:e0168899. doi: 10.1371/journal.pone.0168899
152. Kolodziejski PA, Sassek M, Chalupka D, Leciejewska N, Nogowski L, Mackowiak P, et al. Glp1 and gip are involved in the action of synbiotics in broiler chickens. J Anim Sci Biotechnol. (2018) 9:13. doi: 10.1186/s40104-017-0227-8
153. Seino Y, Fukushima M, Yabe D. Gip and glp-1, the two incretin hormones: similarities and differences. J Diabetes Investig. (2010) 1:8–23. doi: 10.1111/j.2040-1124.2010.00022.x
154. Dunislawska A, Slawinska A, Bednarczyk M, Siwek M. Transcriptome modulation by in ovo delivered lactobacillus synbiotics in a range of chicken tissues. Genes. (2019) 698:27–33. doi: 10.1016/j.gene.2019.02.068
155. Bertocchi M, Zampiga M, Luise D, Vitali M, Sirri F, Slawinska A, et al. In ovo injection of a galacto-oligosaccharide prebiotic in broiler chickens submitted to heat-stress: impact on transcriptomic profile and plasma immune parameters. Animals. (2019) 9:1067. doi: 10.3390/ani9121067
156. Pietrzak E, Dunislawska A, Siwek M, Zampiga M, Sirri F, Meluzzi A, et al. Splenic gene expression signatures in slow-growing chickens stimulated in ovo with galactooligosaccharides and challenged with heat. Animals. (2020) 10:474. doi: 10.3390/ani10030474
157. Alizadeh M, Shojadoost B, Astill J, Taha-Abdelaziz K, Karimi SH, Bavananthasivam J, et al. Effects of in ovo inoculation of multi-strain lactobacilli on cytokine gene expression and antibody-mediated immune responses in chickens. Front Vet Sci. (2020) 7:105. doi: 10.3389/fvets.2020.00105
158. Tako E, Ferket PR, Uni Z. Changes in chicken intestinal zinc exporter mRNA expression and small intestinal functionality following intra-amniotic zinc-methionine administration. J Nutr Biochem. (2005) 16:339–46. doi: 10.1016/j.jnutbio.2005.01.002
159. Vallee BL, Auld DS. Zinc coordination, function, and structure of zinc enzymes and other proteins. Biochemistry. (1990) 29:5647–59. doi: 10.1021/bi00476a001
160. Vallee BL, Falchuk KH. The biochemical basis of zinc physiology. Physiol Rev. (1993) 73:79–118. doi: 10.1152/physrev.1993.73.1.79
161. Gaither LA, Eide DJ. Eukaryotic zinc transporters and their regulation. Biometals. (2001) 14:251–70. doi: 10.1023/A:1012988914300
162. Rhodes D, Klug A. Zinc fingers. Sci Am. (1993) 268:56–9, 62–5. doi: 10.1038/scientificamerican0293-56
163. Cheled-Shoval SL, Behrens M, Meyerhof W, Niv MY, Uni Z. Perinatal administration of a bitter tastant influences gene expression in chicken palate and duodenum. J Agric Food Chem. (2014) 62:12512–20. doi: 10.1021/jf502219a
164. Jaeger LA, Lamar CH, Cline TR, Cardona CJ. Effect of orally administered epidermal growth factor on the jejunal mucosa of weaned pigs. Am J Vet Res. (1990) 51:471–4.
165. Playford RJ, Wright NA. Why is epidermal growth factor present in the gut lumen? Gut. (1996) 38:303–5. doi: 10.1136/gut.38.3.303
166. Kim E, Akhtar N, Li J, Hui Q, Dong B, Yang C, et al. In ovo feeding of epidermal growth factor: embryonic expression of intestinal epidermal growth factor receptor and posthatch growth performance and intestinal development in broiler chickens. Poult Sci. (2020) 99:5736–43. doi: 10.1016/j.psj.2020.07.029
167. El-Senousey HK, Chen B, Wang JY, Atta AM, Mohamed FR, Nie QH. In ovo injection of ascorbic acid modulates antioxidant defense system and immune gene expression in newly hatched local Chinese yellow broiler chicks. Poult Sci. (2018) 97:425–9. doi: 10.3382/ps/pex310
168. Dunislawska A, Herosimczyk A, Ozgo M, Lepczynski A, Ciechanowicz AK, Bednarczyk M, et al. Proteome changes upon in ovo stimulation with lactobacillus synbiotic in chicken liver. Poult Sci. (2021) 100:101449. doi: 10.1016/j.psj.2021.101449
169. Wilson KM, Rodrigues DR, Briggs WN, Duff AF, Chasser KM, Bottje WG, et al. Impact of in ovo administered pioneer colonizers on intestinal proteome on day of hatch. Poult Sci. (2020) 99:1254–66. doi: 10.1016/j.psj.2019.10.017
170. Rodrigues DR, Wilson KM, Bielke LR. Proper immune response depends on early exposure to gut microbiota in broiler chicks. Front Physiol. (2021) 12:758183. doi: 10.3389/fphys.2021.758183
171. Rodrigues DR, Wilson KM, Trombetta M, Briggs WN, Duff AF, Chasser KM, et al. A proteomic view of the cross-talk between early intestinal microbiota and poultry immune system. Front Physiol. (2020) 11:20. doi: 10.3389/fphys.2020.00020
172. Juricova H, Videnska P, Lukac M, Faldynova M, Babak V, Havlickova H, et al. Influence of Salmonella enterica serovar enteritidis infection on the development of the cecum microbiota in newly hatched chicks. Appl Environ Microbiol. (2013) 79:745–7. doi: 10.1128/AEM.02628-12
173. Bar-Shira E, Cohen I, Elad O, Friedman A. Role of goblet cells and mucin layer in protecting maternal IgA in precocious birds. Dev Comp Immunol. (2014) 44:186–94. doi: 10.1016/j.dci.2013.12.010
174. Ricke SC, Pillai SD. Conventional and molecular methods for understanding probiotic bacteria functionality in gastrointestinal tracts. Crit Rev Microbiol. (1999) 25:19–38. doi: 10.1080/10408419991299176
175. Schleifer JH. A review of the efficacy and mechanism of competitive exclusion for the control of salmonella in poultry. World's Poult Sci J. (1985) 41:72–83. doi: 10.1079/WPS19850007
176. Edens FW, Parkhurst CR, Casas IA, Dobrogosz WJ. Principles of ex ovo competitive exclusion and in ovo administration of Lactobacillus reuteri. Poult Sci. (1997) 76:179–96. doi: 10.1093/ps/76.1.179
177. Angwech H, Tavaniello S, Ongwech A, Kaaya AN, Maiorano G. Efficacy of in ovo delivered prebiotics on growth performance, meat quality and gut health of Kuroiler chickens in the face of a natural coccidiosis challenge. Animals. (2019) 9:876. doi: 10.3390/ani9110876
178. Uyanga VA, Oke EO, Amevor FK, Zhao J, Wang X, Jiao H, et al. Functional roles of taurine, L-theanine, L-citrulline, and betaine during heat stress in poultry. J Anim Sci Biotechnol. (2022) 13:23. doi: 10.1186/s40104-022-00675-6
179. Wasti S, Sah N, Mishra B. Impact of heat stress on poultry health and performances, and potential mitigation strategies. Animals. (2020) 10:1266. doi: 10.3390/ani10081266
180. Ncho C-M, Goel A, Jeong C-M, Gupta V, Choi Y-H. Effects of in ovo feeding of γ-aminobutyric acid on growth performances, plasma metabolites, and antioxidant status in broilers exposed to cyclic heat stress. Sustainability. (2021) 13:11032. doi: 10.3390/su131911032
181. Han G, Yang H, Wang Y, Zhang R, Tashiro K, Bungo T, et al. Effects of in ovo feeding of l-leucine on amino acids metabolism and heat-shock protein-70, and−90 mRNA expression in heat-exposed chicks. Poult Sci. (2019) 98:1243–53. doi: 10.3382/ps/pey444
182. Tavaniello S, Slawinska A, Prioriello D, Petrecca V, Bertocchi M, Zampiga M, et al. Effect of galactooligosaccharides delivered in ovo on meat quality traits of broiler chickens exposed to heat stress. Poult Sci. (2020) 99:612–9. doi: 10.3382/ps/pez556
183. Tavaniello S, Slawinska A, Sirri F, Wu M, De Marzo D, Siwek M, et al. Performance and meat quality traits of slow-growing chickens stimulated in ovo with galactooligosaccharides and exposed to heat stress. Poult Sci. (2022) 101:101972. doi: 10.1016/j.psj.2022.101972
184. Kop-Bozbay C, Ocak N. In ovo injection of branched-chain amino acids: embryonic development, hatchability and hatching quality of Turkey poults. J Anim Physiol Anim Nutr. (2019) 103:1135–42. doi: 10.1111/jpn.13111
185. Salmanzadeh MH, Shahryar A, Lotfi A. Effect of in ovo feeding of butyric acid on hatchability, performance and small intestinal morphology of Turkey poults. Kafkas Univ Vet Fak Derg. (2015) 21:19–25.
186. Foye OT, Ashwell C, Uni Z, Ferket PR. The effects of intra-amnionic feeding of arginine and/or ß-hyroxy-ß-methylbutyrate on jejunal gene expression in the turkey embryo and hatchling. Int J Poult Sci. (2009) 8:437–45. doi: 10.3923/ijps.2009.437.445
187. Foye OT, Ferket PR, Uni Z. The effects of in ovo feeding arginine, beta-hydroxy-beta-methyl-butyrate, and protein on jejunal digestive and absorptive activity in embryonic and neonatal turkey poults. Poult Sci. (2007) 86:2343–9. doi: 10.3382/ps.2007-00110
188. Bottje W, Wolfenden A, Ding L, Wolfenden R, Morgan M, Pumford N, et al. Improved hatchability and posthatch performance in turkey poults receiving a dextrin-iodinated casein solution in ovo. Poult Sci. (2010) 89:2646–50. doi: 10.3382/ps.2010-00932
189. Dong XY, Jiang YJ, Wang MQ, Wang YM, Zou XT. Effects of in ovo feeding of carbohydrates on hatchability, body weight, and energy status in domestic pigeons (Columba livia). Poult Sci. (2013) 92:2118–23. doi: 10.3382/ps.2013-03091
190. Dong XY, Wang YM, Song HH, Zou XT. Effects of in ovo injection of carbohydrate solution on small intestine development in domestic pigeons (Columba livia). J Anim Sci. (2013) 91:3742–9. doi: 10.2527/jas.2013-6400
191. Zhang XY, Wan XP, Miao LP, Zou XT, Dong XY. Effects of in ovo feeding of l-arginine on hatchability, hatching time, early posthatch development, and carcass traits in domestic pigeons (Columba livia). J Anim Sci. (2017) 95:4462–71. doi: 10.2527/jas2017.1776
192. Xu QQ, Zhang XY, Zou XT, Dong XY. Effects of in ovo injection of l-histidine on hatch performance and post-hatch development in domestic pigeons (Columba livia). Poult Sci. (2019) 98:3194–203. doi: 10.3382/ps/pez046
193. Zhang XY Li LL, Miao LP, Zhang NN, Zou XT. Effects of in ovo feeding of cationic amino acids on hatchability, hatch weights, and organ developments in domestic pigeon squabs (Columba livia). Poult Sci. (2018) 97:110–7. doi: 10.3382/ps/pex260
194. Lamosová D, Mácajová M, Zeman M, Mózes S, Jezová D. Effect of in ovo leptin administration on the development of Japanese quail. Physiol Res. (2003) 52:201–9.
195. Kermanshahi H, Daneshmand A, Emami NK, Tabari DG, Doosti M, Javadmanesh A, et al. Effect of in ovo injection of threonine on mucin2 gene expression and digestive enzyme activity in Japanese quail (Coturnix japonica). Res Vet Sci. (2015) 100:257–62. doi: 10.1016/j.rvsc.2015.03.023
196. Kermanshahi H, Ghofrani Tabari D, Khodambashi Emami N, Daneshmand A, Ibrahim SA. Effect of in ovo injection of threonine on immunoglobulin a gene expression in the intestine of Japanese quail at hatch. J Anim Physiol Anim Nutr. (2017) 101:10–4. doi: 10.1111/jpn.12543
197. Al-Shammari KIA, Batkowska J, Drabik K, Gryzińska MM. Time of sexual maturity and early egg quality of Japanese quails affected by in ovo injection of medicinal plants. Arch Anim Breed. (2019) 62:423–30. doi: 10.5194/aab-62-423-2019
198. Kim DH, Lee J, Kim S, Lillehoj HS, Lee K. Hypertrophy of adipose tissues in quail embryos by in ovo injection of all-trans retinoic acid. Front Physiol. (2021) 12:681562. doi: 10.3389/fphys.2021.681562
199. Karageçili MR, Babacanoglu E. Influence of in-ovo vitamin e and ascorbic acid injections on chick development, hatching performance and antioxidant content in different tissues of newly-hatched quail chicks. Br Poult Sci. (2022) 63:840–6. doi: 10.1080/00071668.2022.2094221
200. Chen W, Wang R, Wan HF, Xiong XL, Peng P, Peng J. Influence of in ovo injection of glutamine and carbohydrates on digestive organs and pectoralis muscle mass in the duck. Br Poult Sci. (2009) 50:436–42. doi: 10.1080/00071660903114341
201. Chen W, Wang R, Xiong XL, Wan HF, Xu J, Peng J. Influence of in ovo injection of disaccharides, glutamine and β-hydroxy-β-methylbutyrate on the development of small intestine in duck embryos and neonates. Br Poult Sci. (2010) 51:592–601. doi: 10.1080/00071668.2010.520533
202. Chen W, Xu J, Tangara M, Peng J. Effects of in ovo injecting disaccharides and alanyl-glutamine dipeptide on the energy status in duck embryos and neonates. Anim Reprod Sci. (2010) 122:29–35. doi: 10.1016/j.anireprosci.2010.06.009
203. Tangara M, Chen W, Xu J, Huang FR, Peng J. Effects of in ovo feeding of carbohydrates and arginine on hatchability, body weight, energy metabolism and perinatal growth in duck embryos and neonates. Br Poult Sci. (2010) 51:602–8. doi: 10.1080/00071668.2010.520303
204. Liu HH, Wang JW, Zhang RP, Chen X, Yu HY, Jin HB, et al. In ovo feeding of igf-1 to ducks influences neonatal skeletal muscle hypertrophy and muscle mass growth upon satellite cell activation. J Cell Physiol. (2012) 227:1465–75. doi: 10.1002/jcp.22862
205. Liu HH, Wang JW, Chen X, Zhang RP, Yu HY, Jin HB, et al. In ovo administration of rhigf-1 to duck eggs affects the expression of myogenic transcription factors and muscle mass during late embryo development. J Appl Physiol. (2011) 111:1789–97. doi: 10.1152/japplphysiol.00551.2011
206. Liu H, Zhang R, Li X, Sun L, Wang H, Yang C, et al. Influence of recombinant duck follistatin protein on embryonic muscle development and gene expressions. J Anim Physiol Anim Nutr. (2014) 98:522–9. doi: 10.1111/jpn.12104
207. Nowaczewski S, Kontecka H, Krystianiak S. Effect of in ovo injection of vitamin c during incubation on hatchability of chickens and ducks. Folia Biol. (2012) 60:93–7. doi: 10.3409/fb60_1-2.93-97
208. Gouda A, Tolba SA, Mahrose KM. Influences of vitamin a, l-carnitine, and folic acid in ovo feeding on embryo and hatchling characteristics and general health status in ducks. Anim Biotechnol. (2022) 33:150–8. doi: 10.1080/10495398.2020.1864389
209. Beck CN, McDaniel CD, Wamsley KGS, Kiess AS. The potential for inoculating Lactobacillus animalis and Enterococcus faecium alone or in combination using commercial in ovo technology without negatively impacting hatch and post-hatch performance. Poult Sci. (2019) 98:7050–62. doi: 10.3382/ps/pez441
210. Yalcin S, Özkan S, Shah T. Incubation temperature and lighting: effect on embryonic development, post-hatch growth, and adaptive response. Front Physiol. (2022) 13:899977. doi: 10.3389/fphys.2022.899977
211. Decuypere E, Tona K, Bruggeman V, Bamelis F. The day-old chick: a crucial hinge between breeders and broilers. World's Poult Sci J. (2001) 57:127–38. doi: 10.1079/WPS20010010
212. Tona K, Voemesse K, N'Nanlé O, Oke OE, Kouame YAE, Bilalissi A, et al. Chicken incubation conditions: role in embryo development, physiology and adaptation to the post-hatch environment. Front Physiol. (2022) 13:895854. doi: 10.3389/fphys.2022.895854
213. Cox NA, Bailey JS, Blankenship LC, Gildersleeve RP. Research note: in ovo administration of a competitive exclusion culture treatment to broiler embryos. Poult Sci. (1992) 71:1781–4. doi: 10.3382/ps.0711781
214. de Oliveira JE, van der Hoeven-Hangoor E, van de Linde IB, Montijn RC, van der Vossen JMBM. In ovo inoculation of chicken embryos with probiotic bacteria and its effect on posthatch salmonella susceptibility. Poult Sci. (2014) 93:818–29. doi: 10.3382/ps.2013-03409
215. Triplett MD, Zhai W, Peebles ED, McDaniel CD, Kiess AS. Investigating commercial in ovo technology as a strategy for introducing probiotic bacteria to broiler embryos. Poult Sci. (2018) 97:658–66. doi: 10.3382/ps/pex317
216. Moghaddam AA, Borji M, Komazani D. Hatchability rate and embryonic growth of broiler chicks following in ovo injection royal jelly. Br Poult Sci. (2014) 55:391–7. doi: 10.1080/00071668.2014.921664
217. Melo LD, Cruz FGG, Rufino JPF, Melo RD, Feijó JDC, Andrade PGC, et al. In ovo feeding of creatine monohydrate increases performances of hatching and development in breeder chicks. Anim Biotechnol. (2022) 2022:1–11. doi: 10.1080/10495398.2022.2126368
218. Mousstaaid A, Fatemi SA, Elliott KEC, Alqhtani AH, Peebles ED. Effects of the in ovo injection of l-ascorbic acid on broiler hatching performance. Animals. (2022) 12:1020. doi: 10.3390/ani12081020
219. Dang DX, Zhou H, Lou Y, Li D. Effects of in ovo feeding of disaccharide and/or methionine on hatchability, growth performance, blood hematology, and serum antioxidant parameters in geese. J Anim Sci. (2022) 100:ska014. doi: 10.1093/jas/skac014
220. Fatemi SA, Elliott KEC, Bello A, Durojaye OA, Zhang H, Peebles ED. Effects of source and level of in ovo-injected vitamin d(3) on the hatchability and serum 25-hydroxycholecalciferol concentrations of ross 708 broilers. Poult Sci. (2020) 99:3877–84. doi: 10.1016/j.psj.2020.04.030
221. Al-Shamery N, Al-Shuhaib MB. Effect of in ovo injection of various nutrients on the hatchability, mortality ratio and weight of the broiler chickens. IOSR J Agric Vet Sci. (2015) 8:2319–72. doi: 10.9790/2380-08123033
Keywords: in ovo, nutrition, growth, microbiota, gastrointestinal tract, chicken
Citation: Kpodo KR and Proszkowiec-Weglarz M (2023) Physiological effects of in ovo delivery of bioactive substances in broiler chickens. Front. Vet. Sci. 10:1124007. doi: 10.3389/fvets.2023.1124007
Received: 14 December 2022; Accepted: 14 February 2023;
Published: 16 March 2023.
Edited by:
Daniel Hernandez-Patlan, Key Laboratory for Feed Biotechnology, National Autonomous University of Mexico, MexicoReviewed by:
Haijun Zhang, Feed Research Institute (CAAS), ChinaAli Daneshmand, University of New England, Australia
Margarita A. Arreguin Nava, Independent Researcher, Fayetteville, AR, United States
Copyright © 2023 Kpodo and Proszkowiec-Weglarz. This is an open-access article distributed under the terms of the Creative Commons Attribution License (CC BY). The use, distribution or reproduction in other forums is permitted, provided the original author(s) and the copyright owner(s) are credited and that the original publication in this journal is cited, in accordance with accepted academic practice. No use, distribution or reproduction is permitted which does not comply with these terms.
*Correspondence: Monika Proszkowiec-Weglarz, bW9uaWthLndlZ2xhcnpAdXNkYS5nb3Y=