- 1Faculty of Medicine and Health Sciences, School of Veterinary Medicine and Science, University of Nottingham, Nottingham, United Kingdom
- 2Institute of Fundamental Medicine and Biology, Kazan Federal University, Kazan, Russia
- 3Faculty of Medicine and Health Science, Biodiscovery Institute, University of Nottingham, Nottingham, United Kingdom
- 4Comparative Pathology Platform (COMPATH), Institute of Animal Pathology, University of Bern, Bern, Switzerland
Cancer is a leading cause of non-communicable morbidity and mortality throughout the world, similarly, in dogs, the most frequent cause of mortality is tumors. Some types of cancer, including osteosarcoma (OSA), occur at much higher rates in dogs than people. Dogs therefore not only require treatment themselves but can also act as an effective parallel patient population for the human disease equivalent. It should be noted that although there are many similarities between canine and human OSA, there are also key differences and it is important to research and highlight these features. Despite progress using chorioallantoic membrane models, 2D and 3D in vitro models, and rodent OSA models, many more insights into the molecular and cellular mechanisms, drug development, and treatment are being discovered in a variety of canine OSA patient populations.
Introduction
In both human and canine patients the predominant bone cancer diagnosis is OSA (1, 2). Sarcomas are tumors originating in tissues derived from the mesoderm, affecting bone, cartilage and connective tissue (3). Osteosarcoma produces malignant bone or osteoid tissue, but a unifying feature is that all types of OSA histologically produce tumor osteoid (4). Archetypal OSA consists of a primary tumor, usually originating within the medullary cavity and spreading to the surface of the bone, but they can be extra-osseous (5). Typically the tumor grows, proliferates, invades, and left unchecked frequently metastasises to the lungs (6). OSA subtypes include osteoblastic (bony), chondroblastic (cartilaginous), and fibroblastic (resemble atypical fibroblasts), with a range of rare types, and those not originating in the medullary cavity (5), see also a previous review (7).
Overview of experimental OSA models
Rodent and chorioallantoic membrane (CAM) models have been utilized in OSA studies, in addition to a variety of in vitro methods, however each has a number of limitations. Early rodent models represent OSA well-histologically, but do not represent the true etiology of the disease (8). Immunocompromised mice inoculated with human OSA cell lines or grafts have served well for studying metastasis, drug screening, and helped toward identifying activation pathways, but have limited capacity in understanding OSA development and immune system interactions, although advances in this area are continuing (9–11). P53 and Rb mutation transgenic mouse studies have an overall relatively high cost and difficulties relating to breeding and development of non-OSA cancers, but have shown similarities to the human disease (12–15).
Although CAM models have been used for over a century, an avian environment does not always replicate the mammalian tumor environment or immune system. Although angiogenesis in this model can assist with looking at invasion, drug development, and metastasis, it has not been widely used for OSA models (16, 17). Indeed many of the models developed for OSA failed to produce a tumor and/or osteoid, a key component of OSA (18). The advancements in 3D in vitro models over 2D ones, represents a step forward in understanding microenvironment interactions and mechanisms, with fewer limitations than traditional culturing models (19, 20). Examples using liquid overlays (21) and ultra-low binding plates to develop spheroid formation have been used, the latter helping identify a potential role of miR-335 in OSA (21). Hanging drop methods have also been used, especially alongside 2D cell cultures to investigate VEGF expression, vital for angiogenesis (22).
Unfortunately, none of these methods perfectly recreate the tumor microenvironment, or replicate growth and development of the cancer. While these methods have improved prevention, diagnosis, and treatment of a range of diseases, OSA cure rates and survival times have not improved significantly in decades (23, 24). What is really required is a model or parallel patient population that accurately recapitulates the clinical, biological and molecular aspects of human/pediatric OSA.
OSA in dogs and people–parallel patient populations
Given the spontaneous nature of OSA in dogs, and the clinical relevance of canine to human OSA, these natural models might be better described as parallel patient populations (7, 25). Naturally occurring parallel patient populations allow researchers access to additional cases of disease without inducing disease.
Current understanding of OSA disease processes and treatments is largely based on studying affected individuals compared to unaffected individuals, or assessing differing types of OSA, with computer simulations/bioinformatics playing an increasing role (26, 27). The development and progression of OSA is frequently influenced by a combination of environmental and genetic risk factors. Understanding the basis of disease and development of new treatments via animal models, particularly within naturally occurring animal populations, is crucial, however care must be taken to ensure phenotypes are representative of the disease.
The overall canine population is genetically heterogeneous, however breeds can be comparatively homogeneous which further enhances their value for comparing genetic mechanisms of disease (28). Some breeds are at increased risk of developing OSA (1), making them a valuable parallel patient population. Human diseases may progress over a number of years, and spontaneously occurring canine OSA reflects this progression in contrast to laboratory models which are often investigated over much shorter periods of time. Indeed many human disease phenotypes are closely matched to canine disease phenotypes, exhibiting similar pathologies, progression, treatment options, and prognosis (29–31), this includes OSA (7). The canine and human OSA biological and histological similarities, alongside treatment trials and comparisons have been evidenced through numerous studies across the decades. More recently, the molecular and cellular comparisons undertaken between the two species, as detailed within this review, have provided crucial steps toward understanding both the limitations and benefits of studying canine OSA as a parallel population.
Similarities and differences in OSA incidence, risk factors and survival rates between people and dogs
Dogs naturally have a higher OSA incidence than people. Human population studies have shown there are roughly 0.89 cases of bone cancer per 100,000 people/annum (32, 33). In a population of 394,061 insured dogs, 764 (0.19%) developed a bone tumor (1), representing an incidence rate of 27.2 dogs per 100,000/annum, a much higher rate than in people. The higher incidence rate of canine OSA makes the pet dog population an ideal parallel patient population for investigating the disease in humans. In people, there is increasing evidence of variation in the incidence rate between families and different populations (2, 34, 35). Interestingly, OSA in dogs is highly influenced by breed, with Irish wolfhounds displaying the highest levels (12.3% of the population), with some other breeds mostly unaffected (1, 36).
Osteosarcoma is bimodal in people peaking in the young (< 20 years old) and elderly (>60 years old) (2, 32, 33, 37, 38). Although widely reported as a bimodal occurrence in canines, with peaks at 1.5–3 and 7–9 years, this bimodal observation has not been shown all studies (39–43). An additional important difference between the species is that OSA is more prominent in the older dog range (7–9 years), whereas in people the incidence is highest within the pediatric population. The first cross-species genomic analysis between canine and human OSA indicated that there were very strong gene expression similarities between the two species (25). Hierarchical clustering showed branching between OSA and normal tissues but showed no distinct branching of canine and human OSA. This study specifically compared pediatric tumors from children against canine (ages not stated) OSA and was able to draw the conclusion regarding the similarities between canine and pediatric OSA but did not test adult human tumors (25). Notably a later study looked at both juvenile and adult canine tumors indicated that the adult dog was a good model regarding genomic features and clinical characteristics (44). This supports the data indicating clinical presentation and diagnosis, histological presentation and treatment similarities between canine and juvenile human OSA (45, 46).
Despite a general trend of improving 5-year-event-free-survival rates across all cancer types in people (24, 47), OSA has not shown comparable improvements in mortality rates (2, 47, 48). The 5-year-event-free-survival for individuals with metastatic tumors at diagnosis was reported to be 27.4%, increasing to 70% in individuals with no metastases at diagnosis (2, 6). The 1-year survival rate for canines is typically < 45% (49–51). It is worth noting that for appendicular OSA, the 1 and 2-year survival rates have been published at just 11.5 and 2%, respectively for dogs receiving amputation only as a treatment option (52). These similarities in presentation not only support the rationale for the dog as a parallel patient population for studying OSA but also highlight the urgent need to develop improved treatments and cures.
The common risk factors associated with OSA development in both humans and canines include sex, growth, puberty (2, 34, 53), in addition to population/breed and a range of molecular associations. Growth has been associated with the development of OSA in both people and dogs (1, 36). In people, age of onset frequently coincides with rapid bone growth during puberty, tumor sites are most frequently situated at the end of bones where active growth occurs (2). In canines there is not as much evidence linking to growth, given the later onset of OSA in general, however OSA predominantly occurs in weight-bearing bones and adjacent to late-closing physes (1, 36). Large dog breeds make up the majority of canine OSA cases reflecting the human population where affected individuals are more likely to be taller than average (1, 34, 54).
In the canine population, as with the human population, there appears to be a skewed sex ratio with males typically more affected by OSA, and at younger ages, than females (1, 2, 32, 34, 55). Additionally, neutering status, although less relevant in the human context, appears to contribute to risk with neutered dogs more likely to develop OSA than non-neutered counterparts (36). The neutering effect, combined with the association with puberty, indicates that sex hormone signaling may play complex roles in OSA.
Presently there are over 544 canine “potential models for human traits” listed in OMIA (Online Mendelian Inheritance in Animals), more than any other species (29, 30). Dogs are typically treated as family members and so inhabit the same environment as their owners, alongside many of the environmental and other risk factors impacting disease risk, initiation and progression. Pet dogs also frequently benefit from high quality medical care, such that illnesses are detected and treated promptly, similar in a way to people (56). This also means that the amount of information being collected by veterinary clinics, researchers, and insurance companies expands the data available. These canine population characteristics represent a valuable resource for modeling human disease. Although understanding diseases and developing novel treatments in companion animals exhibiting occurring disease is less contentious than inducing disease in experimental animals, ethical concerns regarding treatment of individuals and gaining informed consent from owners remain (57).
Similarities and differences between OSA molecular mechanisms in people and dogs
Developing new treatments is expensive and time consuming. Only 4.1% of potential new compounds progress from preclinical discovery to patient use, taking on average 13.5 years (58, 59). In order to create targeted pharmaceuticals in shorter time frames, understanding the genetic mechanisms behind diseases are critical (60). Indeed, parallel animal patient populations of disease, including OSA, play crucial roles in identifying genetic loci associations and biomarkers, which may lead to target identification, to help determine appropriate drugs, leading through to target validation (61–63). Much of the molecular work, underpinning early drug development and repurposing, is facilitated by the conservation of many fundamental biological pathways between species (64–66).
Pedigree breeds in dogs are generally fairly closed populations, ancestry can often be traced for many generations, and even back to the breed's founding members (28, 67, 68). Although this restricts genetic diversity within breeds, it facilitates understanding the mode of inheritance of traits and diseases (67). Both the founder effects and later inbreeding within canine pedigree breeds have led to divergent allele frequencies between breeds, resulting in some breeds exhibiting higher disease frequencies (28, 69). As a result, differing breeds have homologs of numerous human conditions, making them ideal for identifying potential genetic loci associated with disease for both canine and human benefit.
Some cases of human OSA have been associated with heritable cancer syndromes, and the genetic basis of these has been established (70–72). Despite this, most human OSA cases are not considered to be heritable. Some somatic mutations in tumor suppressor genes have been identified in individuals with heritable cancer syndromes such as Li-Fraumeni syndrome, and other mutations have been identified in OSA tissue compared to normal tissue (70, 73–75). Interesting, to date, only two somatic genetic mutations have been specifically associated with OSA (53). Despite the lack of heritability and somatic genetic mutations, over 900 genes are associated with human OSA (76). These associations have been identified due to differences in expression, or identification of mutations, that have arisen in the tumor compared to the non-tumor tissue (77–79). Mutations within OSA tumor tissue may exist as a cause, or as a result, of the tumor. Differential expression, and mutations, may also exist via genomic and chromosomal instability, which in itself is a reported factor in many types of cancer progression (80, 81). Osteosarcoma in people has been shown to display chromosomal instability associated with mutations in the TP53 gene (82). Aneuploidy can occur as a consequence of chromosomal instability, which can lead to the gene overexpression in affected malignant cells, causing disruption to the normal cell processes (83). Although mutations in TP53 look likely to be associated with chromosomal instability, the gene itself is not over expressed following aneuploidy (82, 83). TP53 has also been implicated in canine OSA with whole genome sequencing and whole exome sequencing (WES) indicating frequent TP53 mutations in canine OSA tumors, at rates of up to 83%, specific mutation rates were variable between breeds (44, 84, 85). TP53 mutations have featured heavily in many of canine OSA studies, however findings still differ between these studies overall. For example it was found that TP53 missense mutations in dogs who had amputation followed by chemotherapy were associated with a longer DFI than wild type of null tumor samples investigated (85). Although similar results have not yet been observed in human OSA, other cancer types and mutant cell lines have shown improved treatment responses (86, 87).
Gene expression following treatment has also highlighted key similarities between people and dogs. Studies identifying gene expression in canine patients responding, and not responding, to chemotherapy treatment, were later found to be similar in people, indicating the value of the dog as a parallel patient population for human OSA (88). It should also be noted that gene expression variations have been observed on some occasions, despite the often-high similarities in many other studies (25), thus indicating a potential limitation of canine comparisons with human OSA.
In canine OSA patients, 33 loci have been associated with the disease across three breeds, and an additional single locus is associated in Deerhounds (89, 90). None of these loci are consistently associated across breeds, suggesting there may be a difference between breeds regarding genetic predisposition to developing OSA (89, 90). In addition to the 34 genetic loci identified, genes have been identified as differentially expressed in canine OSA compared to non-tumor tissue, many of which have implications for growth and metastasis, and are potential drug targets (55, 91–96). These genes have been identified utilizing canine OSA tumor tissue, and/or canine OSA cell lines. Some proteins of interest have also had histological work undertaken to start identifying their presence and relevance in OSA (55, 95). There has also been variation in the expression of genes within tumors associated with survival time in canine OSA (97–100).
Shared genes and proteins of interest for development of future treatments
In both humans and dogs, effective treatment for OSA involves surgery to remove primary tumors 27, 32], often combined with neoadjuvant and/or adjuvant radiotherapy and chemotherapy [33, 34]. The type of surgery rarely has an impact on survival for most human tumors [27], more important prognostic factors are how the tumor responds to chemotherapy and the presence of metastases prior to surgery [27]. In order to advance the treatments available, genomics and drug discovery are providing potential new treatments. Increasingly, comparisons between results from human and canine OSA studies are showing shared genes of interest between the two species. Many of these studies also highlight the need for further testing in relation to potential therapeutic agents.
Comparative transcriptional profiling of dogs and human OSAs has highlighted the similarities between the tumor tissues in the two species. One example was OSA tissue cluster analysis undertaken on 265 orthologous transcripts on pediatric human OSA compared to canine (age not stated) OSA (25). The conclusion was that it was not possible to differentiate between canine and pediatric human OSA tissues yet normal tissues from both species did branch (25). Similar outcome predictions for specific genes in both humans and dogs were also observed. Examples of these include interleukin-8 (IL-8) and solute carrier family 1 (glial high affinity glutamate transporter), member 3 (SLC1A3). Increased expression levels of IL-8 and SLC1A3 predicted poor clinical outcomes in tissues from both species, a result initially identified in canine samples, then followed up and confirmed using human OSA data and both human and canine OSA cell lines (25). Interestingly, increased expression of SLC2A1 (GLUT1) within tumors also resulted in poorer prognosis and a shorter disease free interval in people (101). SLC2A1/GLUT1 (see Figure 1) levels were also significantly increased in naturally occurring canine OSA tissue compared to normal bone tissue (55, 95). Inhibition of SLC2A1 and cellular glucose transport has been achieved by a number of pharmaceuticals, however, as with the MMP3 inhibitors, these have yet to be utilized in OSA trials in either people or dogs (102–105). Monoclonal IL-8 antibody therapy could also be of interest given the importance of this chemo-attractant angiogenic factor, which has been implicated in a number of cancers (106, 107). Although not yet tested in OSA, clinical trials using IL-8 monoclonal antibodies in other cancers types are ongoing (108) and provide an interesting target given the links between increased IL-8 expression and doxorubicin resistance (109, 110).
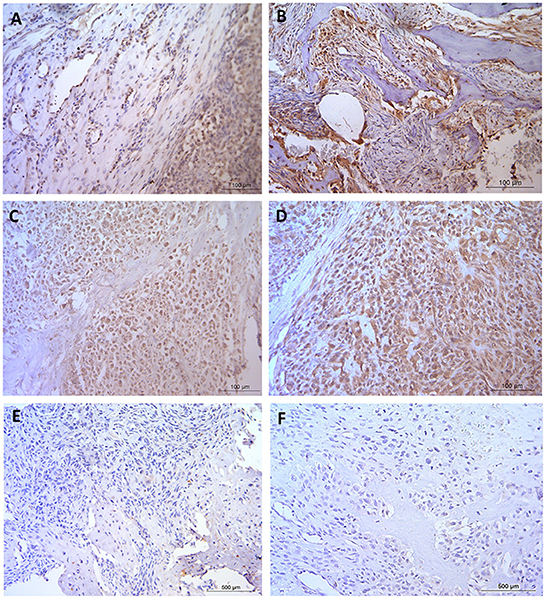
Figure 1. Immunohistochemical staining in naturally occurring canine osteosarcoma tissue. (A,B) GLUT1 and (C,D) MMP3, both staining patterns expressing positive nuclear, cytoplasmic and vascular tissue with negative staining observed in the osteoid. (E,F) Negative control. Scale bars represent (A–D) 100 μm, (E,F) 500 μm. Staining was conducted with ethics, techniques and tissues as previously published (95).
Another good example of comparative OSA highlighted the role of MMP3, with increased expression linked with a poor prognosis in OSA, and to formation of metastases (23, 111). Tsai et al. (112) and Huang et al. (113) identified higher expression of MMP3 in human OSA compared to normal bone. Additionally Adiguzel et al. (114) reported on MMP3 polymorphisms associated with OSA in people. Naturally occurring OSA was also associated with increased MMP3 levels in canine patients (55), and work was later undertaken to show expression patterns (Figure 1) of the protein in tissue (95). Despite the increasing evidence regarding MMP3, neither the selective inhibitor of MMP3 (UK370106) (115) or the generic MMP inhibitor (marimastat) (116) have been assessed in relation to restricting primary tumors or metastatic tumor growth in canine or human OSA, despite some trials in other tumor types.
The Dickkopf proteins are differentially expressed in a number of cancers, and inhibit Wnt signaling which, in turn, is aberrant in many cancers (117–119). Reduced DKK3 expression in human breast, endometrial, and cervical cancer, has implicated it as a tumor suppressor (120–123). DKK3 expression within OSA has resulted in conflicting reports. In human OSA cell lines, and in xenograft mice, DKK3 expression was reduced, however subsequent restoration of DKK3 expression resulted in reduced tumor and metastatic growth (124). In contrast, DKK3 was more highly expressed in human OSA cells overexpressing NKD2 and in tumor tissue (125), and also in tumor tissue compared to non-affected bone in naturally occurring canine OSA (55). Despite differences compared to some cancers, this outcome agreed with DKK3 knockdown in cells overexpressing NKD2 which exhibited increased proliferation, indicating a possible mechanism of NKD2 induced metastasis, although the authors noted more work into the mechanisms was required (125). With a lack of drugs available acting on DKK3, development in this direction could prove useful for OSA in both people and dogs. Although these examples represent just a small number of the genes and proteins of interest in both human and canine OSA, it helps show the benefits of using the dog as a parallel patient population for this cancer, especially in relation to drug development. Table 1 provides a summary of the genes, proteins and pathways detailed in this review.
Whole genome and exome sequencing have also discovered not only where mutations within pathways such as PI3K and MAPK are similar between human and canine OSA, but have also identified novel aberrations in canines, such as those in SETD and DMD, which have not yet been reported in people (44). Although these aberrations have not yet been found in human OSA cases, despite the high sequence homology between the two species, it is known that dysregulation of SETD2 has been implied in human OSA (25, 133). Although the DMD gene encoding dystrophin is more commonly associated with Duchene and Becker muscular dystrophy in both species, other studies have shown somatic DMD variants in human OSA patients (129). Comparative canine and human transcriptomic studies have also identified annotations and pathways unique to particular cancers. For example, annotations unique to bone material synthesis, including COL5A2, COL6A3, and COL12A, were discovered in OSA in both species but were not present in melanoma, pulmonary carcinoma, or B- and T-cell lymphoma (130). Considerable insights into possible pathways and biomarkers can be provided by such studies. Often potential biomarkers, or targets that have known chemistries presently available, including examples such as COL16A1 and KDELR2 (130), are highlighted as areas needing more research.
Shared MicroRNAs in comparative studies
Recent reviews outlining the potential comparative values for investigating vasculogenic mimicry molecular pathways and microRNAs (miRNA) in the dog, highlight how little work has been conducted in this species compared to humans (134, 135). They contain detailed discussions around miRNAs and lncRNAs and provide interesting reading around these areas, especially in relation to vasculogenic mimicry in canines in comparison to people, which is not therefore covered in the present review. In both dogs and people decreased expression of miR-1, miR-133b and miR-196A have been shown to be involved in proliferation-invasion, miR-34 with proliferation and 14q32 locus (including mir-544, miR-396-3p, miR134 and miR-382) with proliferation-apoptosis in OSA (135). Additionally increased miR-9 has been associated with invasion and increases in miR-106b cluster have been associated with proliferation (135). Comparative examples such as the dysregulation of the 14q32 miRNA cluster in both dogs and people, not only identified a potentially conserved mechanism related to the aggressive and invasive biological behavior of OSA in both species, but yet again emphasize the similarities between the two species (136). Another example is the discovery of miR-1 and miR-133b which showed lower expression levels in canine OSA compared to normal tissue, yet increased expression of their targets MET and MCL1 (137). Interestingly a previous study had shown that both miR-1 and miR-133b were differentially expressed in human OSA affected tissue compared to non-OSA bone (138). MiR-34a looks especially promising given its links to both human and canine OSA, its anti-proliferation and metastasis inhibition activities and the research relating to a genetically engineered pre-microRNA-34a prodrug (139–141). Work published after the recent review (135) compared 19 miRNA candidates expressing differential expression in OSA samples compared to non-affected tissue in both people and dogs were also assessed (142). This research showed that expression miR-223 increases and in let-7b and miR-130a decreases were associated with increased risk and a shorter disease free interval. These were highlighted as potential targets and/or biomarkers for OSA.
Translational drug development studies
In addition to the molecular studies highlighting potential targets of interest, a number of canine OSA trials have assessed treatment regimens which were primarily designed to increase survival times. Early evidence showed that while amputation alone increased canine survival by around 2.5 months, addition of liposome-encapsulated muramyl tripeptide (L-MTP-PE) administration following amputation prolonged survival by an additional 5 months, primarily by reducing OSA metastasis development (143). Later randomized canine trials showed the outcomes of combining differing protocols of L-MTP-PE and cisplatin chemotherapy treatment. L-MTP-PE exhibited antimetastatic activity when administered post amputation, increasing survival times to around 14.4 months when cisplatin was administered after L-MTP-PE (144). The survival advantages observed following L-MTP-PE alone were not observed when cisplatin and L-MTP-PE were administered concurrently rather than sequentially, indicating that treatment timing is crucial. Trials of this drug in children with OSA revealed an 8% improvement in survival (145), but anti-tumor effects and increased survival times were also noted when treating human OSA patients with L-MTP-PE, especially when chemotherapy was administered (146–148). For example, a 24-week treatment with L-MTP-PE increased median time to relapse from 4.5 months for the control group to 9 months for the treatment group (146, 147). It was also noted that plasma levels of cytokines including IL-8, TNF-α and IL-6 reduced following treatment, all of which may play roles in monocyte-mediated tumor cell death (146, 147). This work followed the smaller phase II trial indicating histological changes to pulmonary metastases in OSA patients (148).
HER2/neu, a tyrosine kinase receptor within the epidermal growth factor receptor family, is expressed in osteosarcoma stem cells (149). Expression has been found in 40% of pediatric and canine osteosarcoma, and associated with higher metastatic rates, reduced response to neoadjuvant chemotherapy, and reduced survival times (150–152). A chimeric human HER2/neu fusion protein (ADXS31-164, also now known as ADXS-HER2 and OST-HER2) was tested in dogs with a histopathological and immunohistochemical diagnosis of HER2/neu OSA, following amputation/limb sparing surgery and treatment with carboplatin (153). Disease-free interval (DFI) following the intervention was 615 days, median survival time (MST) was 956 days, and overall survival rates at 1, 2, and 3 years were 77.8, 67, and 56%, respectively. The authors noted significant outcome improvements compared to matched historical control group rates showing a DFI of 123–257 days, a MST of 207–321 days, and overall survival rates of 35.4% (1 year) and 10–15% (2 years). Additionally, this study showed only mild side-effects of ADXS31-164 when administered to canine patients. This therapy specifically induced HER2-specific immunity, targeting the cells expressing HER2/neu, broke peripheral tolerance to HER2/neu and mediated cytotoxic T-cell–dependent tumor regression (153). In 2016, ADXS-HER2 was granted orphan-drug designation, then rare pediatric disease designation in 2021, from the FDA and EMA, for the treatment of OSA. In 2021 ADVAXIS Immunotherapies, in collaboration with the Children's Oncology Group, reported that the first human OSA patient had received doses in the Phase IIb trial of this drug (154). The outcomes from this trial, including any clinical results and mechanistic studies will be of great interest regarding not only human and canine OSA, but in relation to other cancer types which also express HER2 including mammary carcinoma (126).
The angiotensin-receptor blocker losartan, when used in combination with the kinase inhibitor toceranib, has also shown promising results in canine OSA patients (127). By blocking OSA-elicited monocyte recruitment via the action of losartan inhibiting the CCL2–CCR2 axis, clinical benefits including tumor stabilization and/or regression were observed in half of the dogs. Notably, both human and canine OSA cells secrete CCL2, resulting in monocyte migration. By interrupting the CCR2–CCL2 axis and by blocking monocyte migration, these trials have provided more insights into the tumor microenvironment and indicated a direct mechanism by which these therapeutic agents could work in human OSA patients. Owing to the success of this canine OSA trial published in 2021, a phase I clinical trial (NCT03900793) was initiated in pediatric and young adult OSA patients with lung metastases.
Limitations of canine OSA models
One of the limitations of this area of research is that frequently the research concentrates on either dogs or people with relatively few comparisons of the two using the same analysis and techniques. Although this individual species specific research is required, the number of directly comparative studies is much lower and makes comparative conclusions more complex. This also complicates matters with regards to potential differences observed between breeds and age of onset, as highlighted in this review. For example although the differences between breeds are often presented, in many cases the comparisons between each of the breeds and human OSA are not frequently investigated. Canines are often referred to as a good model for juvenile human OSA but in addition to the published comparisons between general canine OSA and juvenile human OSA, it must be highlighted that many studies do draw any conclusions regarding juvenile or later onset OSA specifically in either species. In some OSA studies, particularly the canine studies, the ages of the patients are not presented or the juvenile/later onset differences, if any, are not specifically referred to or investigated. Additionally, particularly when thinking about juvenile OSA, matters such as whether growth is a risk factor is not as easy to justify in dogs compared to people.
With molecular differences between OSA samples differing between individuals within the same species, it is natural to expect differences between the species and between the ages of the individuals. When comparing canine OSA to human pediatric OSA in particular, mutational burden must be considered. Generally pediatric human cancers present with fewer mutational burdens, compared with geriatric tumors [as reviewed previously (128)], therefore this must be considered when comparing against older canines with pediatric OSA. There are concomitant arguments for using the dog as a model of aging (also presenting with limitations and differences) (131). Although similarities between canine and human DNA repair machinery have been shown, such as in lymphoma, mammary tumors and even OSA (132, 155, 156), not every mechanism may be similar, for example base excision repair and nucleotide excision repair have both been shown to be lower in canines (157). Unfortunately little is known about DNA repair in canine OSA (158) even though it may play significant roles when comparing geriatric with juvenile/pediatric OSA. This further accentuates the need for vigilance when researching OSA in general including both OSA specific molecular mechanisms and pathways, and those related to more general factors such as aging.
Discussion
Canine OSA occurs naturally within the population, reflecting the development of human OSA (7, 159). In contrast animal models of OSA rely on chemical induction, xeno/allografts, and genetically engineered animals which are unlikely to reflect many aspects of naturally occurring disease (159–162). Canine OSA has several features that can accelerate the understanding of the molecular basis of OSA, potentially facilitating more rapid development of novel diagnostic and therapeutic targets relevant to both people and dogs. The advantages of canine OSA parallel patient populations include a shared environment with people, natural disease progression, higher incidence rates, alongside shorter lifespans resulting in a quicker clinical course. Arguments have also been put forward that in addition to the dog being a good parallel patient population for OSA in people, the reverse is also technically true. It has also been indicated that canine OSA may represent a more accelerated biology than human OSA and that novel metastasis-associated tumor targets may be more readily identifiable in canine tissues (25). Whilst this review has concentrated on some of the shared molecular observations and mechanisms, there are many examples presented where canines do not exactly mirror human OSA. Canine OSA parallel patient populations can therefore give valuable insights, advancing knowledge about disease progression and development, cellular and molecular mechanisms, and therapeutic and treatment strategies, in both people and dogs.
Author contributions
CR developed the concept, designed and supervised the manuscript, and created Figure 1. SS and CR drafted the manuscript. CR and AR received funding. All authors revised the manuscript critically, contributed intellectually, and approved the submitted version.
Funding
The authors gratefully acknowledge generous funding from the University of Nottingham BBSRC Doctoral Training Programmes BB/J014508/1 and the School of Veterinary Medicine and Science, University of Nottingham, awarded to CR. AR was supported by the Kazan Federal University Strategic Academic Leadership Program (PRIORITY-2030).
Acknowledgments
The authors would like to thank Aziza Alibhai and James Cockcroft for undertaking some of the immunohistochemistry and microscopy shown in Figure 1, under the supervision of CR.
Conflict of interest
The authors declare that the research was conducted in the absence of any commercial or financial relationships that could be construed as a potential conflict of interest.
Publisher's note
All claims expressed in this article are solely those of the authors and do not necessarily represent those of their affiliated organizations, or those of the publisher, the editors and the reviewers. Any product that may be evaluated in this article, or claim that may be made by its manufacturer, is not guaranteed or endorsed by the publisher.
References
1. Egenvall A, Nødtvedt A, von Euler H. Bone tumors in a population of 400 000 insured Swedish dogs up to 10 y of age: incidence and survival. Can J Vet Res. (2007) 71:292–9.
2. Mirabello L, Troisi RJ, Savage SA. Osteosarcoma incidence and survival rates from 1973 to 2004: data from the Surveillance, epidemiology, and end results program. Cancer. (2009) 115:1531–43. doi: 10.1002/cncr.24121
3. Souhami R, Tobias J. Bone and soft-tissue sarcomas. Cancer Manag. (2005). 12:2609. doi: 10.1002/9780470994887
4. Ragland BD, Bell WC, Lopez RR, Siegal GP. Cytogenetics and molecular biology of osteosarcoma. Lab Invest. (2002) 82:365–73. doi: 10.1038/labinvest.3780431
5. Klein MJ, Siegal GP. Osteosarcoma - anatomic and histologic variants. Am J Clin Pathol. (2006) 125:555–81. doi: 10.1309/UC6KQHLD9LV2KENN
6. Bacci G, Rocca M, Salone M, Balladelli A, Ferrari S, Palmerini E, et al. High grade osteosarcoma of the extremities with lung metastases at presentation: treatment with neoadjuvant chemotherapy and simultaneous resection of primary and metastatic lesions. J Surg Oncol. (2008) 98:415–20. doi: 10.1002/jso.21140
7. Simpson S, Dunning MD, de Brot S, Grau-Roma L, Mongan NP, Rutland CS. Comparative review of human and canine osteosarcoma: morphology, epidemiology, prognosis, treatment and genetics. Acta Vet Scand. (2017) 59:71. doi: 10.1186/s13028-017-0341-9
8. Uluckan O, Segaliny A, Botter S, Santiago JM, Mutsaers AJ. Preclinical mouse models of osteosarcoma. Bonekey Rep. (2015) 4:670. doi: 10.1038/bonekey.2015.37
9. Khanna C, Khan J, Nguyen P, Prehn J, Caylor J, Yeung C, et al. Metastasis-associated differences in gene expression in a murine model of osteosarcoma. Cancer Res. (2001) 61:3750–9.
10. Shimozaki S, Yamamoto N, Domoto T, Nishida H, Hayashi K, Kimura H, et al. Efficacy of glycogen synthase kinase-3beta targeting against osteosarcoma via activation of beta-catenin. Oncotarget. (2016) 7:77038–51. doi: 10.18632/oncotarget.12781
11. Chulpanova DS, Kitaeva KV, Rutland CS, Rizvanov AA, Solovyeva VV. Mouse tumor models for advanced cancer immunotherapy. Int J Mol Sci. (2020) 21:4118. doi: 10.3390/ijms21114118
12. Taneja P, Zhu S, Maglic D, Fry EA, Kendig RD, Inoue K. Transgenic and knockout mice models to reveal the functions of tumor suppressor genes. Clin Med Insights Oncol. (2011) 5:235–57. doi: 10.4137/CMO.S7516
13. Scott MC, Tomiyasu H, Garbe JR, Cornax I, Amaya C, O'Sullivan MG, et al. Heterotypic mouse models of canine osteosarcoma recapitulate tumor heterogeneity and biological behavior. Dis Model Mech. (2016) 9:1435–44. doi: 10.1242/dmm.026849
14. Lee EY, Chang CY, Hu N, Wang YC, Lai CC, Herrup K, et al. Mice deficient for Rb are nonviable and show defects in neurogenesis and haematopoiesis. Nature. (1992) 359:288–94. doi: 10.1038/359288a0
15. Walkley CR, Qudsi R, Sankaran VG, Perry JA, Gostissa M, Roth SI, et al. Conditional mouse osteosarcoma, dependent on p53 loss and potentiated by loss of Rb, mimics the human disease. Genes Dev. (2008) 22:1662–76. doi: 10.1101/gad.1656808
16. Deryugina EI, Quigley JP. Chapter 2. Chick embryo chorioallantoic membrane models to quantify angiogenesis induced by inflammatory and tumor cells or purified effector molecules. Methods Enzymol. (2008) 444:21–41. doi: 10.1016/S0076-6879(08)02802-4
17. Manjunathan R, Ragunathan M. Chicken chorioallantoic membrane as a reliable model to evaluate osteosarcoma-an experimental approach using SaOS2 cell line. Biol Proced Online. (2015) 17:10. doi: 10.1186/s12575-015-0022-x
18. Balke M, Neumann A, Kersting C, Agelopoulos K, Gebert C, Gosheger G, et al. Morphologic characterization of osteosarcoma growth on the chick chorioallantoic membrane. BMC Res Notes. (2010) 3:58. doi: 10.1186/1756-0500-3-58
19. Kapalczynska M, Kolenda T, Przybyla W, Zajaczkowska M, Teresiak A, Filas V, et al. 2D and 3D cell cultures - a comparison of different types of cancer cell cultures. Arch Med Sci. (2018) 14:910–9. doi: 10.5114/aoms.2016.63743
20. Gilazieva Z, Ponomarev A, Rutland C, Rizvanov A, Solovyeva V. Promising applications of tumor spheroids and organoids for personalized medicine. Cancers. (2020) 12:2727. doi: 10.3390/cancers12102727
21. Martins-Neves SR, Lopes AO, do Carmo A, Paiva AA, Simoes PC, Abrunhosa AJ, et al. Therapeutic implications of an enriched cancer stem-like cell population in a human osteosarcoma cell line. BMC Cancer. (2012) 12:139. doi: 10.1186/1471-2407-12-139
22. Chaddad H, Kuchler-Bopp S, Fuhrmann G, Gegout H, Ubeaud-Sequier G, Schwinte P, et al. Combining 2D angiogenesis and 3D osteosarcoma microtissues to improve vascularization. Exp Cell Res. (2017) 360:138–45. doi: 10.1016/j.yexcr.2017.08.035
23. Reddy KIA, Wafa H, Gaston CL, Grimer RJ, Abudu AT, Jeys LM, et al. Does amputation offer any survival benefit over limb salvage in osteosarcoma patients with poor chemonecrosis and close margins? Bone Joint J. (2015) 97-B:115–20. doi: 10.1302/0301-620X.97B1.33924
24. Siegel RL, Miller KD, Jemal A. Cancer statistics, 2015. CA Cancer J Clin. (2015) 65:5–29. doi: 10.3322/caac.21254
25. Paoloni M, Davis S, Lana S, Withrow S, Sangiorgi L, Picci P, et al. Canine tumor cross-species genomics uncovers targets linked to osteosarcoma progression. BMC Genomics. (2009) 10:625. doi: 10.1186/1471-2164-10-625
26. Brown DC, Agnello K, Iadarola MJ. Intrathecal resiniferatoxin in a dog model: efficacy in bone cancer pain. Pain. (2015) 156:1018–24. doi: 10.1097/j.pain.0000000000000115
27. Lee D-F, Su J, Kim Huen S, Chang B, Papatsenko D, Zhao R, et al. Modeling familial cancer with induced pluripotent stem cells. Cell. (2015) 161:240–54. doi: 10.1016/j.cell.2015.02.045
28. Parker HG, Kim LV, Sutter NB, Carlson S, Lorentzen TD, Malek TB, et al. Genetic structure of the purebred domestic dog. Science. (2004) 304:1160–4. doi: 10.1126/science.1097406
29. Lenffer J, Nicholas FW, Castle K, Rao A, Gregory S, Poidinger M, et al. OMIA (online mendelian inheritance in animals): an enhanced platform and integration into the entrez search interface at NCBI. Nucleic Acids Res. (2006) 34:D599–601. doi: 10.1093/nar/gkj152
30. OMIA. Online Mendelian Inheritance in Animals, OMIA. Faculty of Veterinary Science, University of Sydney. (2022). Available online at: http://omia.angis.org.au/ (accessed February 4, 2022).
31. Tsai KL, Clark LA, Murphy KE. Understanding hereditary diseases using the dog and human as companion model systems. Mamm Genome. (2007) 18:444–51. doi: 10.1007/s00335-007-9037-1
32. Mirabello L, Troisi RJ, Savage SA. International osteosarcoma incidence patterns in children and adolescents, middle ages and elderly persons. Int J Cancer. (2009) 125:229–34. doi: 10.1002/ijc.24320
33. Parkin DM, Ferlay J, Curado M-P, Bray F, Edwards B, Shin H-R, et al. Fifty years of cancer incidence: CI5 I-IX. Int J Cancer. (2010) 127:2918–27. doi: 10.1002/ijc.25517
34. Mirabello L, Pfeiffer R, Murphy G, Daw NC, Patiño-Garcia A, Troisi RJ, et al. Height at diagnosis and birth-weight as risk factors for osteosarcoma. Cancer Causes Control. (2011) 22:899–908. doi: 10.1007/s10552-011-9763-2
35. Sadykova LR, Ntekim AI, Muyangwa-Semenova M, Rutland CS, Jeyapalan JN, Blatt N, et al. Epidemiology and risk factors of osteosarcoma. Cancer Invest. (2020) 38:259–69. doi: 10.1080/07357907.2020.1768401
36. Ru G, Terracini B, Glickman LT. Host related risk factors for canine osteosarcoma. Vet J. (1998) 156:31–9. doi: 10.1016/S1090-0233(98)80059-2
37. Forman D, Bray F, Brewster DH, Gombe Mbalawa C, Kohler B, Piñeros MS-F, et al. Cancer Incidence in Five Continents, Vol. X: IARC Scientific Publication No. 164. Lyon: International Agency for Research on Cancer (2014).
38. Troisi R, Masters MN, Joshipura K, Douglass C, Cole BF, Hoover RN. Perinatal factors, growth and development, and osteosarcoma risk. Br J Cancer. (2006) 95:1603–7. doi: 10.1038/sj.bjc.6603474
39. Misdorp W, Hart AAM. Some prognostic and epidemiologic factors in canine osteosarcoma. J Natl Cancer Inst. (1979) 62:537–45. doi: 10.1093/jnci/62.3.537
40. Anfinsen KP, Grotmol T, Bruland OS, Jonasdottir TJ. Breed-specific incidence rates of canine primary bone tumors–a population based survey of dogs in Norway. Can J Vet Res. (2011) 75:209–15.
41. Tuohy JL, Shaevitz MH, Garrett LD, Ruple A, Selmic LE. Demographic characteristics, site and phylogenetic distribution of dogs with appendicular osteosarcoma: 744 dogs (2000-2015). PLoS ONE. (2019) 14:e0223243. doi: 10.1371/journal.pone.0223243
42. Morello E, Martano M, Buracco P. Biology, diagnosis and treatment of canine appendicular osteosarcoma: similarities and differences with human osteosarcoma. Vet J. (2011) 189:268–77. doi: 10.1016/j.tvjl.2010.08.014
43. Edmunds GL, Smalley MJ, Beck S, Errington RJ, Gould S, Winter H, et al. Dog breeds and body conformations with predisposition to osteosarcoma in the UK: a case-control study. Canine Med Genet. (2021) 8:2. doi: 10.1186/s40575-021-00100-7
44. Gardner HL, Sivaprakasam K, Briones N, Zismann V, Perdigones N, Drenner K, et al. Canine osteosarcoma genome sequencing identifies recurrent mutations in DMD and the histone methyltransferase gene SETD2. Commun Biol. (2019) 2:266. doi: 10.1038/s42003-019-0487-2
45. Withrow SJ, Powers BE, Straw RC, Wilkins RM. Comparative aspects of osteosarcoma. Dog versus man. Clin Orthop Relat Res. (1991) (270):159–68. doi: 10.1097/00003086-199109000-00023
46. Mueller F, Fuchs B, Kaser-Hotz B. Comparative biology of human and canine osteosarcoma. Anticancer Res. (2007) 27:155–64.
47. Siegel R, Naishadham D, Jemal A. Cancer statistics, 2013. CA Cancer J Clin. (2013) 63:11–30. doi: 10.3322/caac.21166
48. Dorfman HD, Czerniak B. Bone cancers. Cancer (1995). 75:203–10. doi: 10.1002/1097-0142(19950101)75:1+<203::aid-cncr2820751308>3.0.co;2-v
49. Frimberger AE, Chan CM, Moore AS. Canine osteosarcoma treated by post-amputation sequential accelerated doxorubicin and carboplatin chemotherapy: 38 cases. J Am Anim Hosp Assoc. (2016) 52:149–56. doi: 10.5326/JAAHA-MS-6315
50. Moore AS, Dernell WS, Ogilvie GK, Kristal O, Elmslie R, Kitchell B, et al. Doxorubicin and BAY 12–9566 for the treatment of osteosarcoma in dogs: a randomized, double-blind, placebo-controlled study. J Vet Intern Med. (2007) 21:783–90. doi: 10.1111/j.1939-1676.2007.tb03022.x
51. Straw RC, Withrow SJ, Richter SL, Powers BE, Klein MK, Postorino NC, et al. Amputation and cisplatin for treatment of canine osteosarcoma. J Vet Intern Med. (1991) 5:205–10. doi: 10.1111/j.1939-1676.1991.tb00950.x
52. Spodnick GJ, Berg J, Rand WM, Schelling SH, Couto G, Harvey HJ, et al. Prognosis for dogs with appendicular osteosarcoma treated by amputation alone: 162 cases (1978-1988). J Am Vet Med Assoc. (1992) 200:995–9.
53. Savage SA, Mirabello L, Wang Z, Gastier-Foster JM, Gorlick R, Khanna C, et al. Genome-wide association study identifies two susceptibility loci for osteosarcoma. Nat Genet. (2013) 45:799–803. doi: 10.1038/ng.2645
54. McNeill CJ, Overley B, Shofer FS, Kent MS, Clifford CA, Samluk M, et al. Characterization of the biological behaviour of appendicular osteosarcoma in Rottweilers and a comparison with other breeds: a review of 258 dogs*. Vet Comp Oncol. (2007) 5:90–8. doi: 10.1111/j.1476-5829.2006.00116.x
55. Simpson S, Dunning M, de Brot S, Alibhai A, Bailey C, Woodcock CL, et al. Molecular characterisation of canine osteosarcoma in high risk breeds. Cancers. (2020) 12:2405. doi: 10.3390/cancers12092405
56. Ostrander EA, Galibert F, Patterson DF. Canine genetics comes of age. Trends Genet. (2000) 16:117–24. doi: 10.1016/S0168-9525(99)01958-7
57. Page R, Baneux P, Vail D, Duda L, Olson P, Anestidou L, et al. Conduct, oversight, and ethical considerations of clinical trials in companion animals with cancer: report of a workshop on best practice recommendations. J Vet Intern Med. (2016) 30:527–35. doi: 10.1111/jvim.13916
58. Calcoen D, Elias L, Yu X. What does it take to produce a breakthrough drug? Nat Rev Drug Discov. (2015) 14:161–2. doi: 10.1038/nrd4570
59. Paul SM, Mytelka DS, Dunwiddie CT, Persinger CC, Munos BH, Lindborg SR, et al. How to improve R&D productivity: the pharmaceutical industry's grand challenge. Nat Rev Drug Discov. (2010) 9:203–14. doi: 10.1038/nrd3078
60. Nelson MR, Tipney H, Painter JL, Shen J, Nicoletti P, Shen Y, et al. The support of human genetic evidence for approved drug indications. Nat Genet. (2015) 47:856–60. doi: 10.1038/ng.3314
61. Finan C, Gaulton A, Kruger FA, Lumbers RT, Shah T, Engmann J, et al. The druggable genome and support for target identification and validation in drug development. Sci Transl Med. (2017) 9:eaag1166. doi: 10.1126/scitranslmed.aag1166
62. Floris M, Olla S, Schlessinger D, Cucca F. Genetic-driven druggable target identification and validation. Trends Genet. (2018) 34:558–70. doi: 10.1016/j.tig.2018.04.004
63. Letko A, Minor KM, Norton EM, Marinescu VD, Drogemuller M, Ivansson E, et al. Genome-wide analyses for osteosarcoma in leonberger dogs reveal the CDKN2A/B gene locus as a major risk locus. Genes. (2021) 12:1964. doi: 10.3390/genes12121964
64. Guruharsha KG, Kankel MW, Artavanis-Tsakonas S. The Notch signalling system: recent insights into the complexity of a conserved pathway. Nat Rev Genet. (2012) 13:654–66. doi: 10.1038/nrg3272
65. Le MTN, Shyh-Chang N, Khaw SL, Chin L, Teh C, Tay J, et al. Conserved regulation of p53 network dosage by MicroRNA−125b occurs through evolving miRNA–target gene pairs. PLoS Genet. (2011) 7:e1002242. doi: 10.1371/journal.pgen.1002242
66. Loenarz C, Coleman ML, Boleininger A, Schierwater B, Holland PWH, Ratcliffe PJ, et al. The hypoxia-inducible transcription factor pathway regulates oxygen sensing in the simplest animal, Trichoplax adhaerens. EMBO Rep. (2011) 12:63–70. doi: 10.1038/embor.2010.170
67. Calboli FCF, Sampson J, Fretwell N, Balding DJ. Population structure and inbreeding from pedigree analysis of purebred dogs. Genetics. (2008) 179:593–601. doi: 10.1534/genetics.107.084954
68. Jansson M, Laikre L. Recent breeding history of dog breeds in Sweden: modest rates of inbreeding, extensive loss of genetic diversity and lack of correlation between inbreeding and health. J Anim Breed Genet. (2014) 131:153–62. doi: 10.1111/jbg.12060
69. Marsden CD, Ortega-Del Vecchyo D, O'Brien DP, Taylor JF, Ramirez O, Vilà C, et al. Bottlenecks and selective sweeps during domestication have increased deleterious genetic variation in dogs. Proc Nat Acad Sci. (2016) 113:152–7. doi: 10.1073/pnas.1512501113
70. Ognjanovic S, Olivier M, Bergemann TL, Hainaut P. Sarcomas in TP53 germline mutation carriers. Cancer. (2012) 118:1387–96. doi: 10.1002/cncr.26390
71. Stinco G, Governatori G, Mattighello P, Patrone P. Multiple cutaneous neoplasms in a patient with rothmund–thomson syndrome: case report and published work review. J Dermatol. (2008) 35:154–61. doi: 10.1111/j.1346-8138.2008.00436.x
72. Thomas DM, Ballinger ML. Etiologic, environmental and inherited risk factors in sarcomas. J Surg Oncol. (2015) 111:490–5. doi: 10.1002/jso.23809
73. Chen X, Bahrami A, Pappo A, Easton J, Dalton J, Hedlund E, et al. Recurrent somatic structural variations contribute to tumorigenesis in pediatric osteosarcoma. Cell Rep. (2014) 7:104–12. doi: 10.1016/j.celrep.2014.03.003
74. Srivastava S, Zou Z, Pirollo K, Blattner W, Chang EH. Germ-line transmission of a mutated p53 gene in a cancer-prone family with Li-Fraumeni syndrome. Nature. (1990) 348:747–9. doi: 10.1038/348747a0
75. Zhang J, Walsh MF, Wu G, Edmonson MN, Gruber TA, Easton J, et al. Germline mutations in predisposition genes in pediatric cancer. N Engl J Med. (2015) 373:2336–46. doi: 10.1056/NEJMoa1508054
76. Poos K, Smida J, Nathrath M, Maugg D, Baumhoer D, Neumann A, et al. Structuring osteosarcoma knowledge: an osteosarcoma-gene association database based on literature mining and manual annotation. Database. (2014) 2014:bau042. doi: 10.1093/database/bau042
77. Man T-K, Lu X-Y, Jaeweon K, Perlaky L, Harris CP, Shah S, et al. Genome-wide array comparative genomic hybridization analysis reveals distinct amplifications in osteosarcoma. BMC Cancer. (2004) 4:45. doi: 10.1186/1471-2407-4-45
78. Xiao X, Wang W, Zhang H, Gao P, Fan B, Huang C, et al. Individualized chemotherapy for osteosarcoma and identification of gene mutations in osteosarcoma. Tumour Biol. (2015) 36:2427–35. doi: 10.1007/s13277-014-2853-5
79. Yuan D, Liu B, Liu K, Zhu G, Dai Z, Xie Y. Overexpression of fibroblast activation protein and its clinical implications in patients with osteosarcoma. J Surg Oncol. (2013) 108:157–62. doi: 10.1002/jso.23368
80. de Bruin EC, McGranahan N, Mitter R, Salm M, Wedge DC, Yates L, et al. Spatial and temporal diversity in genomic instability processes defines lung cancer evolution. Science. (2014) 346:251–6. doi: 10.1126/science.1253462
81. Galanos P, Vougas K, Walter D, Polyzos A, Maya-Mendoza A, Haagensen EJ, et al. Chronic p53-independent p21 expression causes genomic instability by deregulating replication licensing. Nat Cell Biol. (2016) 18:777–89. doi: 10.1038/ncb3378
82. Al-Romaih K, Bayani J, Vorobyova J, Karaskova J, Park PC, Zielenska M, et al. Chromosomal instability in osteosarcoma and its association with centrosome abnormalities. Cancer Genet Cytogenet. (2003) 144:91–9. doi: 10.1016/S0165-4608(02)00929-9
83. Carter SL, Eklund AC, Kohane IS, Harris LN, Szallasi Z, A. signature of chromosomal instability inferred from gene expression profiles predicts clinical outcome in multiple human cancers. Nat Genet. (2006) 38:1043–8. doi: 10.1038/ng1861
84. Sakthikumar S, Elvers I, Kim J, Arendt ML, Thomas R, Turner-Maier J, et al. SETD2 is recurrently mutated in whole-exome sequenced canine osteosarcoma. Cancer Res. (2018) 78:3421–31. doi: 10.1158/0008-5472.CAN-17-3558
85. Das S, Idate R, Regan DP, Fowles JS, Lana SE, Thamm DH, et al. Immune pathways and TP53 missense mutations are associated with longer survival in canine osteosarcoma. Commun Biol. (2021) 4:1178doi: 10.1038/s42003-021-02683-0
86. Jackson JG, Pant V, Li Q, Chang LL, Quintas-Cardama A, Garza D, et al. p53-Mediated senescence impairs the apoptotic response to chemotherapy and clinical outcome in breast cancer. Cancer Cell. (2012) 21:793–806. doi: 10.1016/j.ccr.2012.04.027
87. Liu DP, Song H, Xu Y. A common gain of function of p53 cancer mutants in inducing genetic instability. Oncogene. (2010) 29:949–56. doi: 10.1038/onc.2009.376
88. O'Donoghue LE, Ptitsyn AA, Kamstock DA, Siebert J, Thomas RS, Duval DL. Expression profiling in canine osteosarcoma: identification of biomarkers and pathways associated with outcome. BMC Cancer. (2010) 10:506. doi: 10.1186/1471-2407-10-506
89. Karlsson EK, Sigurdsson S, Ivansson E, Thomas R, Elvers I, Wright J, et al. Genome-wide analyses implicate 33 loci in heritable dog osteosarcoma, including regulatory variants near CDKN2A/B. Genome Biol. (2013) 14:R132. doi: 10.1186/gb-2013-14-12-r132
90. Phillips JC, Lembcke L, Chamberlin T. A novel locus for canine osteosarcoma (OSA1) maps to CFA34, the canine orthologue of human 3q26. Genomics. (2010) 96:220–7. doi: 10.1016/j.ygeno.2010.07.002
91. McCleese JK, Bear MD, Kulp SK, Mazcko C, Khanna C, London CA. Met interacts with EGFR and Ron in canine osteosarcoma. Vet Comp Oncol. (2013) 11:124–39. doi: 10.1111/j.1476-5829.2011.00309.x
92. Millanta F, Asproni P, Cancedda S, Vignoli M, Bacci B, Poli A. Immunohistochemical expression of COX-2, mPGES and EP2 receptor in normal and reactive canine bone and in canine osteosarcoma. J Comp Pathol. (2012) 147:153–60. doi: 10.1016/j.jcpa.2012.02.003
93. Pang LY, Argyle SA, Kamida A, Morrison K, Argyle DJ. The long-acting COX-2 inhibitor mavacoxib (Trocoxil™) has anti-proliferative and pro-apoptotic effects on canine cancer cell lines and cancer stem cells. BMC Vet Res. (2014) 10:184. doi: 10.1186/PREACCEPT-9511370941246208
94. Shahi MH, York D, Gandour-Edwards R, Withers SS, Holt R, Rebhun RB. BMI1 is expressed in canine osteosarcoma and contributes to cell growth and chemotherapy resistance. PLoS ONE. (2015) 10:e0131006. doi: 10.1371/journal.pone.0131006
95. Rutland CS, Cockcroft JM, Lothion-Roy J, Harris AE, Jeyapalan JN, Simpson S, et al. Immunohistochemical characterisation of GLUT1, MMP3 and NRF2 in osteosarcoma. Front Vet Sci. (2021) 8:704598. doi: 10.3389/fvets.2021.704598
96. Musser ML, Viall AK, Phillips RL, Hostetter JM, Johannes CM. Analysis of gene expression of prostaglandin EP4 receptor in canine osteosarcoma. Can J Vet Res. (2021) 85:68–71. doi: 10.1186/s12917-020-02431-2
97. Maniscalco L, Iussich S, Morello E, Martano M, Gattino F, Miretti S, et al. Increased expression of insulin-like growth factor-1 receptor is correlated with worse survival in canine appendicular osteosarcoma. Vet J. (2015) 205:272–80. doi: 10.1016/j.tvjl.2014.09.005
98. Selvarajah GT, Kirpensteijn J, van Wolferen ME, Rao NAS, Fieten H, Mol JA. Gene expression profiling of canine osteosarcoma reveals genes associated with short and long survival times. Mol Cancer. (2009) 8:72. doi: 10.1186/1476-4598-8-72
99. Selvarajah GT, Verheije MH, Kik M, Slob A, Rottier PJM, Mol JA, et al. Expression of epidermal growth factor receptor in canine osteosarcoma: association with clinicopathological parameters and prognosis. Vet J. (2012) 193:412–9. doi: 10.1016/j.tvjl.2012.02.009
100. Selvarajah GT, Bonestroo FAS, Kirpensteijn J, Kik MJL, van der Zee R, van Eden W, et al. Heat shock protein expression analysis in canine osteosarcoma reveals HSP60 as a potentially relevant therapeutic target. Cell Stress Chaperones. (2013) 18:607–22. doi: 10.1007/s12192-013-0414-2
101. Kubo T, Shimose S, Fujimori J, Furuta T, Arihiro K, Ochi M. Does expression of glucose transporter protein-1 relate to prognosis and angiogenesis in osteosarcoma? Clin Orthop Relat Res. (2015) 473:305–10. doi: 10.1007/s11999-014-3910-5
102. Klepper J, Florcken A, Fischbarg J, Voit T. Effects of anticonvulsants on GLUT1-mediated glucose transport in GLUT1 deficiency syndrome in vitro. Eur J Pediatr. (2003) 162:84–9. doi: 10.1007/s00431-002-1112-8
103. Louters LL, Stehouwer N, Rekman J, Tidball A, Cok A, Holstege CP. Verapamil inhibits the glucose transport activity of GLUT1. J Med Toxicol. (2010) 6:100–5. doi: 10.1007/s13181-010-0072-z
104. Rufino AT, Rosa SC, Judas F, Mobasheri A, Lopes MC, Mendes AF. Expression and function of K(ATP) channels in normal and osteoarthritic human chondrocytes: possible role in glucose sensing. J Cell Biochem. (2013) 114:1879–89. doi: 10.1002/jcb.24532
105. Shuralyova I, Tajmir P, Bilan PJ, Sweeney G, Coe IR. Inhibition of glucose uptake in murine cardiomyocyte cell line HL-1 by cardioprotective drugs dilazep and dipyridamole. Am J Physiol Heart Circ Physiol. (2004) 286:H627–32. doi: 10.1152/ajpheart.00639.2003
106. Uehara H, Troncoso P, Johnston D, Bucana CD, Dinney C, Dong Z, et al. Expression of interleukin-8 gene in radical prostatectomy specimens is associated with advanced pathologic stage. Prostate. (2005) 64:40–9. doi: 10.1002/pros.20223
107. Yao C, Lin Y, Ye CS Bi J, Zhu YF, Wang SM. Role of interleukin-8 in the progression of estrogen receptor-negative breast cancer. Chin Med J. (2007) 120:1766–72. doi: 10.1097/00029330-200710020-00007
108. Melnikova VO, Bar-Eli M. Bioimmunotherapy for melanoma using fully human antibodies targeting MCAM/MUC18 and IL-8. Pigment Cell Res. (2006) 19:395–405. doi: 10.1111/j.1600-0749.2006.00331.x
109. Du J, He Y, Li P, Wu W, Chen Y, Ruan H. IL-8 regulates the doxorubicin resistance of colorectal cancer cells via modulation of multidrug resistance 1 (MDR1). Cancer Chemother Pharmacol. (2018) 81:1111–9. doi: 10.1007/s00280-018-3584-x
110. Cheng M, Cai W, Huang W, Chen Y, Wu Z, Luo P, et al. Histone deacetylase 6 regulated expression of IL-8 is involved in the doxorubicin (Dox) resistance of osteosarcoma cells via modulating ABCB1 transcription. Eur J Pharmacol. (2018) 840:1–8. doi: 10.1016/j.ejphar.2018.09.032
111. Bielack SS, Kempf-Bielack B, Delling G, Exner GU, Flege S, Helmke K, et al. Prognostic factors in high-grade osteosarcoma of the extremities or trunk: an analysis of 1,702 patients treated on neoadjuvant cooperative osteosarcoma study group protocols. J Clin Oncol. (2002) 20:776–90. doi: 10.1200/JCO.2002.20.3.776
112. Tsai H-C, Su H-L, Huang C-Y, Fong Y-C, Hsu C-J, Tang C-H. CTGF increases matrix metalloproteinases expression and subsequently promotes tumor metastasis in human osteosarcoma through down-regulating miR-519d. Oncotarget. (2014) 5:3800–12. doi: 10.18632/oncotarget.1998
113. Huang JF, Du WX, Chen JJ. Elevated expression of matrix metalloproteinase-3 in human osteosarcoma and its association with tumor metastasis. J BUON. (2016) 21:235–43. Available online at: https://www.jbuon.com/archive/21-1-235.pdf
114. Adiguzel M, Horozoglu C, Kilicoglu O, Ozger H, Acar L, Ergen A. MMP-3 gene polymorphisms and osteosarcoma. Indian J Exp Biol. (2016) 54:175–9.
115. Fray MJ, Dickinson RP, Huggins JP, Occleston NL. A potent, selective inhibitor of matrix metalloproteinase-3 for the topical treatment of chronic dermal ulcers. J Med Chem. (2003) 46:3514–25. doi: 10.1021/jm0308038
116. Bramhall SR, Schulz J, Nemunaitis J, Brown PD, Baillet M, Buckels JAC, et al. double-blind placebo-controlled, randomised study comparing gemcitabine and marimastat with gemcitabine and placebo as first line therapy in patients with advanced pancreatic cancer. Br J Cancer. (2002) 87:161–7. doi: 10.1038/sj.bjc.6600446
117. Baehs S, Herbst A, Thieme SE, Perschl C, Behrens A, Scheel S, et al. Dickkopf-4 is frequently down-regulated and inhibits growth of colorectal cancer cells. Cancer Lett. (2009) 276:152–9. doi: 10.1016/j.canlet.2008.11.003
118. Hall CL, Zhang H, Baile S, Ljungman M, Kuhstoss S, Keller ET. p21(CIP-1/WAF-1) induction is required to inhibit prostate cancer growth elicited by deficient expression of the wnt inhibitor dickkopf-1 (DKK-1). Cancer Res. (2010) 70:9916–26. doi: 10.1158/0008-5472.CAN-10-0440
119. Zhou XL, Qin XR, Zhang XD, Ye LH. Downregulation of Dickkopf-1 is responsible for high proliferation of breast cancer cells via losing control of Wnt/β-catenin signaling. Acta Pharmacol Sin. (2010) 31:202–10. doi: 10.1038/aps.2009.200
120. Dellinger TH, Planutis K, Jandial DD, Eskander RN, Martinez ME Zi X, et al. Expression of the Wnt antagonist Dickkopf-3 is associated with prognostic clinicopathologic characteristics and impairs proliferation and invasion in endometrial cancer. Gynecol Oncol. (2012) 126:259–67. doi: 10.1016/j.ygyno.2012.04.026
121. Hsieh SY, Hsieh PS, Chiu CT, Chen WY. Dickkopf-3/REIC functions as a suppressor gene of tumor growth. Oncogene. (2004) 23:9183–9. doi: 10.1038/sj.onc.1208138
122. Lee E-J, Jo M, Rho SB, Park K, Yoo Y-N, Park J, et al. Dkk3, downregulated in cervical cancer, functions as a negative regulator of β-catenin. Int J Cancer. (2009) 124:287–97. doi: 10.1002/ijc.23913
123. Xiang T, Li L, Yin X, Zhong L, Peng W, Qiu Z, et al. Epigenetic silencing of the WNT antagonist Dickkopf 3 disrupts normal Wnt/β-catenin signalling and apoptosis regulation in breast cancer cells. J Cell Mol Med. (2013) 17:1236–46. doi: 10.1111/jcmm.12099
124. Lin CH, Guo Y, Ghaffar S, McQueen P, Pourmorady J, Christ A, et al. Dkk-3, a Secreted Wnt antagonist, suppresses tumorigenic potential and pulmonary metastasis in osteosarcoma. Sarcoma. (2013) 2013:147541. doi: 10.1155/2013/147541
125. Zhao S, Kurenbekova L, Gao Y, Roos A, Creighton CJ, Rao P, et al. NKD2, a negative regulator of Wnt signaling, suppresses tumor growth and metastasis in osteosarcoma. Oncogene. (2015) 34:5069–79. doi: 10.1038/onc.2014.429
126. Ithimakin S, Day KC, Malik F, Zen Q, Dawsey SJ, Bersano-Begey TF, et al. HER2 drives luminal breast cancer stem cells in the absence of HER2 amplification: implications for efficacy of adjuvant trastuzumab. Cancer Res. (2013) 73:1635–46. doi: 10.1158/0008-5472.CAN-12-3349
127. Regan DP, Chow L, Das S, Haines L, Palmer E, Kurihara JN, et al. Losartan blocks osteosarcoma-elicited monocyte recruitment, and combined with the kinase inhibitor toceranib, exerts significant clinical benefit in canine metastatic osteosarcoma. Clin Cancer Res. (2022) 28:662–76. doi: 10.1158/1078-0432.CCR-21-2105
128. Vogelstein B, Papadopoulos N, Velculescu VE, Zhou S, Diaz LA Jr, Kinzler KW. Cancer genome landscapes. Science. (2013) 339:1546–58. doi: 10.1126/science.1235122
129. Chiappetta C, Mancini M, Lessi F, Aretini P, De Gregorio V, Puggioni C, et al. Whole-exome analysis in osteosarcoma to identify a personalized therapy. Oncotarget. (2017) 8:80416–28. doi: 10.18632/oncotarget.19010
130. Tawa GJ, Braisted J, Gerhold D, Grewal G, Mazcko C, Breen M, et al. Transcriptomic profiling in canines and humans reveals cancer specific gene modules and biological mechanisms common to both species. PLoS Comput Biol. (2021) 17:e1009450. doi: 10.1371/journal.pcbi.1009450
131. Sándor S, Kubinyi E. Genetic pathways of aging and their relevance in the dog as a natural model of human aging. Front Genet. (2019) 10:948. doi: 10.3389/fgene.2019.00948
132. Thamm DH, Grunerud KK, Rose BJ, Vail DM, Bailey SM. DNA repair deficiency as a susceptibility marker for spontaneous lymphoma in golden retriever dogs: a case-control study. PLoS ONE. (2013) 8:e69192. doi: 10.1371/journal.pone.0069192
133. Jiang CY, He C, Wu ZQ, Li FF, Xiao JR. Histone methyltransferase SETD2 regulates osteosarcoma cell growth and chemosensitivity by suppressing Wnt/beta-catenin signaling. Biochem Bioph Res Co. (2018) 503:1178. doi: 10.1016/j.bbrc.2018.06.123
134. Massimini M, Romanucci M, De Maria R, Della Salda L. An update on molecular pathways regulating vasculogenic mimicry in human osteosarcoma and their role in canine oncology. Front Vet Sci. (2021) 8:722432. doi: 10.3389/fvets.2021.722432
135. Leonardi L, Scotlandi K, Pettinari I, Benassi MS, Porcellato I, Pazzaglia L. MiRNAs in canine and human osteosarcoma: a highlight review on comparative biomolecular aspects. Cells. (2021) 10:428. doi: 10.3390/cells10020428
136. Sarver AL, Thayanithy V, Scott MC, Cleton-Jansen AM, Hogendoorn PC, Modiano JF, et al. MicroRNAs at the human 14q32 locus have prognostic significance in osteosarcoma. Orphanet J Rare Dis. (2013) 8:7. doi: 10.1186/1750-1172-8-7
137. Leonardo L, Laura P, Serena BM. miR-1 and miR-133b expression in canine osteosarcoma. Res Vet Sci. (2018) 117:133–7. doi: 10.1016/j.rvsc.2017.12.002
138. Novello C, Pazzaglia L, Cingolani C, Conti A, Quattrini I, Manara MC, et al. miRNA expression profile in human osteosarcoma: role of miR-1 and miR-133b in proliferation and cell cycle control. Int J Oncol. (2013) 42:667–75. doi: 10.3892/ijo.2012.1717
139. Yan K, Gao J, Yang T, Ma Q, Qiu X, Fan Q, et al. MicroRNA-34a inhibits the proliferation and metastasis of osteosarcoma cells both in vitro and in vivo. PLoS ONE. (2012) 7:e33778. doi: 10.1371/journal.pone.0033778
140. Zhao Y, Tu MJ, Wang WP, Qiu JX Yu AX, Yu AM. Genetically engineered pre-microRNA-34a prodrug suppresses orthotopic osteosarcoma xenograft tumor growth via the induction of apoptosis and cell cycle arrest. Sci Rep. (2016) 6:26611. doi: 10.1038/srep26611
141. Lopez CM Yu PY, Zhang X, Yilmaz AS, London CA, Fenger JM. MiR-34a regulates the invasive capacity of canine osteosarcoma cell lines. PLoS ONE. (2018) 13:e0190086. doi: 10.1371/journal.pone.0190086
142. Dailey DD, Hess AM, Bouma GJ, Duval DL. MicroRNA expression changes and integrated pathways associated with poor outcome in canine osteosarcoma. Front Vet Sci. (2021) 8:637622. doi: 10.3389/fvets.2021.637622
143. MacEwen EG, Kurzman ID, Rosenthal RC, Smith BW, Manley PA, Roush JK, et al. Therapy for osteosarcoma in dogs with intravenous injection of liposome-encapsulated muramyl tripeptide. J Natl Cancer Inst. (1989) 81:935–8. doi: 10.1093/jnci/81.12.935
144. Kurzman ID, MacEwen EG, Rosenthal RC, Fox LE, Keller ET, Helfand SC, et al. Adjuvant therapy for osteosarcoma in dogs: results of randomized clinical trials using combined liposome-encapsulated muramyl tripeptide and cisplatin. Clin Cancer Res. (1995) 1:1595–601.
145. Meyers PA, Schwartz CL, Krailo MD, Healey JH, Bernstein ML, Betcher D, et al. Osteosarcoma: the addition of muramyl tripeptide to chemotherapy improves overall survival–a report from the Children's Oncology Group. J Clin Oncol. (2008) 26:633–8. doi: 10.1200/JCO.2008.14.0095
146. Asano T, Kleinerman ES. Liposome-encapsulated MTP-PE: a novel biologic agent for cancer therapy. J Immunother Emphasis Tumor Immunol. (1993) 14:286–92. doi: 10.1097/00002371-199311000-00006
147. Kleinerman ES, Gano JB, Johnston DA, Benjamin RS, Jaffe N. Efficacy of liposomal muramyl tripeptide (CGP 19835A) in the treatment of relapsed osteosarcoma. Am J Clin Oncol. (1995) 18:93–9. doi: 10.1097/00000421-199504000-00001
148. Kleinerman ES, Raymond AK, Bucana CD, Jaffe N, Harris MB, Krakoff IH, et al. Unique histological changes in lung metastases of osteosarcoma patients following therapy with liposomal muramyl tripeptide (CGP 19835A lipid). Cancer Immunol Immunother. (1992) 34:211–20. doi: 10.1007/BF01741788
149. Rainusso N, Brawley VS, Ghazi A, Hicks MJ, Gottschalk S, Rosen JM, et al. Immunotherapy targeting HER2 with genetically modified T cells eliminates tumor-initiating cells in osteosarcoma. Cancer Gene Ther. (2012) 19:212–7. doi: 10.1038/cgt.2011.83
150. Onda M, Matsuda S, Higaki S, Iijima T, Fukushima J, Yokokura A, et al. ErbB-2 expression is correlated with poor prognosis for patients with osteosarcoma. Cancer. (1996). 77:71–8. doi: 10.1002/(SICI)1097-0142(19960101)77:1<71::AID-CNCR13>3.0.CO;2-5
151. Zhou H, Randall RL, Brothman AR, Maxwell T, Coffin CM, Goldsby RE. Her-2/neu expression in osteosarcoma increases risk of lung metastasis and can be associated with gene amplification. J Pediatr Hematol Oncol. (2003) 25:27–32. doi: 10.1097/00043426-200301000-00007
152. Flint AF, U'Ren L, Legare ME, Withrow SJ, Dernell W, Hanneman WH. Overexpression of the erbB-2 proto-oncogene in canine osteosarcoma cell lines and tumors. Vet Pathol. (2004) 41:291–6. doi: 10.1354/vp.41-3-291
153. Mason NJ, Gnanandarajah JS, Engiles JB, Gray F, Laughlin D, Gaurnier-Hausser A, et al. Immunotherapy with a HER2-targeting listeria induces HER2-specific immunity and demonstrates potential therapeutic effects in a phase I trial in canine osteosarcoma. Clin Cancer Res. (2016) 22:4380–90. doi: 10.1158/1078-0432.CCR-16-0088
154. Advaxis. OS Therapies Announces Dosing of First Patient in a Phase IIb Trial of OST-HER2 (Listeria monocytogenes) in Recurred, Resected Osteosarcoma. (2021). Available online at: https://www.advaxis.com/static-files/adc16568-e068-492e-93ea-58892df12a0d (accessed February 9, 2022).
155. Raposo-Ferreira TM, Bueno RC, Terra EM, Avante ML, Tinucci-Costa M, Carvalho M, et al. Downregulation of ATM gene and protein expression in canine mammary tumors. Vet Pathol. (2016) 53:1154–9. doi: 10.1177/0300985816643367
156. Kirpensteijn J, Kik M, Teske E, Rutteman GR. TP53 gene mutations in canine osteosarcoma. Vet Surg. (2008) 37:454–60. doi: 10.1111/j.1532-950X.2008.00407.x
157. Park SH, Kang HJ, Kim HS, Kim MJ, Heo JI, Kim JH, et al. Higher DNA repair activity is related with longer replicative life span in mammalian embryonic fibroblast cells. Biogerontology. (2011) 12:565–79. doi: 10.1007/s10522-011-9355-2
158. Grosse N, van Loon B, Rohrer Bley C. DNA damage response and DNA repair - dog as a model? BMC Cancer. (2014) 14:203. doi: 10.1186/1471-2407-14-203
159. Fenger JM, London CA, Kisseberth WC. Canine osteosarcoma: a naturally occurring disease to inform pediatric oncology. ILAR J. (2014) 55:69–85. doi: 10.1093/ilar/ilu009
160. Berlin Ö, Samid D, Donthineni-Rao R, Akeson W, Amiel D, Woods VL. Development of a novel spontaneous metastasis model of human osteosarcoma transplanted orthotopically into bone of athymic mice. Cancer Res. (1993) 53:4890–5.
161. Jacks T, Remington L, Williams BO, Schmitt EM, Halachmi S, Bronson RT, et al. Tumor spectrum analysis in p53-mutant mice. Current Biology. (1994) 4:1–7. doi: 10.1016/S0960-9822(00)00002-6
Keywords: bone cancer, canine, genes, human, osteosarcoma, protein, treatment
Citation: Simpson S, Rizvanov AA, Jeyapalan JN, de Brot S and Rutland CS (2022) Canine osteosarcoma in comparative oncology: Molecular mechanisms through to treatment discovery. Front. Vet. Sci. 9:965391. doi: 10.3389/fvets.2022.965391
Received: 09 June 2022; Accepted: 23 November 2022;
Published: 08 December 2022.
Edited by:
Raffaella De Maria, University of Turin, ItalyReviewed by:
Kohei Saeki, Okayama University of Science, JapanLeonardo Leonardi, University of Perugia, Italy
Copyright © 2022 Simpson, Rizvanov, Jeyapalan, de Brot and Rutland. This is an open-access article distributed under the terms of the Creative Commons Attribution License (CC BY). The use, distribution or reproduction in other forums is permitted, provided the original author(s) and the copyright owner(s) are credited and that the original publication in this journal is cited, in accordance with accepted academic practice. No use, distribution or reproduction is permitted which does not comply with these terms.
*Correspondence: Catrin S. Rutland, Y2F0cmluLnJ1dGxhbmQmI3gwMDA0MDtub3R0aW5naGFtLmFjLnVr