- 1Teagasc, Animal and Bioscience Research Department, Animal and Grassland Research and Innovation Centre, Dunsany, Ireland
- 2UCD School of Agriculture and Food Science, University College Dublin, Dublin, Ireland
Ruminant livestock play a key role in global society through the conversion of lignocellulolytic plant matter into high-quality sources of protein for human consumption. However, as a consequence of the digestive physiology of ruminant species, methane (CH4), which originates as a byproduct of enteric fermentation, is accountable for 40% of global agriculture's carbon footprint and ~6% of global greenhouse gas (GHG) emissions. Therefore, meeting the increasing demand for animal protein associated with a growing global population while reducing the GHG intensity of ruminant production will be a challenge for both the livestock industry and the research community. In recent decades, numerous strategies have been identified as having the potential to reduce the methanogenic output of livestock. Dietary supplementation with antimethanogenic compounds, targeting members of the rumen methanogen community and/or suppressing the availability of methanogenesis substrates (mainly H2 and CO2), may have the potential to reduce the methanogenic output of housed livestock. However, reducing the environmental impact of pasture-based beef cattle may be a challenge, but it can be achieved by enhancing the nutritional quality of grazed forage in an effort to improve animal growth rates and ultimately reduce lifetime emissions. In addition, the genetic selection of low-CH4-emitting and/or faster-growing animals will likely benefit all beef cattle production systems by reducing the methanogenic potential of future generations of livestock. Similarly, the development of other mitigation technologies requiring minimal intervention and labor for their application, such as anti-methanogen vaccines, would likely appeal to livestock producers, with high uptake among farmers if proven effective. Therefore, the objective of this review is to give a detailed overview of the CH4 mitigation solutions, both currently available and under development, for temperate pasture-based beef cattle production systems. A description of ruminal methanogenesis and the technologies used to estimate enteric emissions at pastures are also presented.
Introduction
The global population is expected to exceed nine billion people by the year 2050 (1). To meet the nutritional requirements of the growing population, global agricultural output will be required to increase by an estimated 50% (2). At present, agriculture is accountable for 10–12% of global anthropogenic greenhouse gas (GHG) emissions (3), with emissions from the sector likely to rise in response to increased agricultural output. In light of the urgent need to decrease anthropogenic GHG emissions to limit global surface temperature increase (4), mitigating the environmental burden associated with a rise in global agricultural output will be a challenge.
Global food production has become reliant on ruminant livestock due to the ability of ruminants to transform inaccessible energy stored in plants into high-quality sources of protein and energy for human consumption (5). However, methane (CH4), a potent GHG, estimated to have 28 times greater warming potential than carbon dioxide (CO2) (6), is produced as a metabolic byproduct of the ruminal fermentation of feed by the rumen microbial community (7). Indeed, CH4 originating from enteric fermentation accounts for the majority of global agriculture's 36.5% contribution to anthropogenic CH4 emissions (8). Over the past two decades, numerous CH4 abatement strategies have been developed for ruminant livestock (9–12); however, not all strategies will be easily implemented at the farm level, in particular in pasture-based production systems. The development of anti-methanogenic vaccines would likely appeal to livestock producers; however, the duration of their effectiveness has proven to be an obstacle to their implementation (13). Dietary supplementation may have a role when cattle are housed. However, the supplementation of beef cattle at pasture is likely to be less feasible (14), but may be implemented as part of a winter housing period or as part of a supplementary feeding regime at pasture. Subsequently, due to the low profitability of beef cattle production (15), any CH4 supplementation strategy would at minimum need to be cost-neutral to ensure uptake at the farm level. Mitigation strategies which focus on improving the growth rate of beef cattle at pasture will be advantageous if they reduce the lifetime emissions of growing cattle. However, the suckler cow herd can account for ~80% of the enteric CH4 produced within a cow-calf production system (16). As a result, strategies that can lower the daily CH4 output of an animal will also be required to improve the environmental sustainability of the sector. Equally, animal breeding has been advocated by numerous authors as a mitigation strategy due to its ability to deliver permanent GHG reductions to future generations of livestock (17, 18).
This review aims to give an overview of the relationship of the rumen microbiota with methanogenesis and research focused on developing CH4 mitigation strategies for pasture-based beef cattle production systems. For dietary-focused mitigation strategies, an emphasis is placed on those which are likely to be practically feasible for a temperate pasture-based production system similar to that of the Irish and New Zealand beef cattle production systems. The benefits and limitations on enteric CH4 emissions and animal productivity are discussed for anti-methanogenic vaccinations, pasture management, dietary supplementation, and animal selection-based strategies. Due to potential impacts on animal health and lack of data on seaweeds sourced from temperate geographic regions, the CH4 mitigation potential of supplementing nitrate and seaweeds has not been included but has been reviewed by others (9–12, 19). In addition, while this review will predominantly focus on research conducted in beef cattle, reference to data originating from other ruminants will be utilized where evidence in beef animals is lacking.
Enteric methane
In the rumen, bacteria, protozoa, and fungi hydrolyze plant polysaccharides to monomeric sugars, which are further fermented to produce various products, such as volatile fatty acids (VFA) acetate, propionate, and butyrate along with CO2 and CH4 (20). The Embden–Meyerhof–Parnas (EMP) pathway is the initial pathway in the catabolism of sugars, which results in the formation of pyruvate, a central intermediary metabolite in the rumen (21). During the EMP pathway, carbohydrates are oxidized resulting in the reduction of the electron transporter nicotinamide adenine dinucleotide (NAD+) to NADH, which must be subsequently reoxidized to NAD+ for further fermentation to continue (13). An excessive amount of dihydrogen (H2) in the rumen has the ability to inhibit the activity of hydrogenase enzymes, therefore, limiting the oxidation of sugars when alternative pathways for H2 disposal are absent (13). Methanogenesis contributes to the efficiency of the rumen, preventing increases in the partial pressure of H2 and therefore promoting the function of microbial enzymes involved in electron transfer reactions, such as NADH dehydrogenase (22). The rumen provides a unique environment characterized by a relatively rapid passage rate and a readily available supply of CO2 and H2, resulting in a community of archaea distinct from other anoxic systems (23). During the dominant methanogenesis pathway (hydrogenotrophic methanogenesis) conducted by rumen methanogens, H2 is oxidized to H+, and CO2 is reduced to form CH4 (24). However, CH4 can also be produced via the reduction of methyl compounds and acetate via methylotrophic and acetoclastic methanogenesis, respectively (Table 1). Methanogenesis is considered an energy-inefficient process, which consumes an estimated 2–12% of the animal's gross energy intake (GEI) (26, 27). After its synthesis in the rumen, the bulk of enteric CH4 is expelled from the rumen via eructation (28).
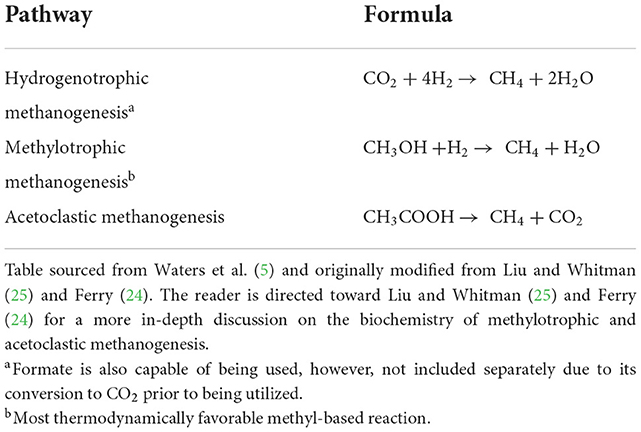
Table 1. The three main methanogenesis pathways in the rumen with estimates of free energies from reactions.
Hydrogenotrophic methanogenesis pathway
In nature, three major substrates are used in the production of CH4: CO2, compounds containing methyl groups, and acetate (25). In the hydrogenotrophic pathway, CH4 is produced from the reduction of CO2 with electrons donated from H2 and formate (25, 29). Compounds containing methyl groups also provide a carbon source for CH4 production (30). In the acetoclastic pathway, the carbonyl group of acetate is cleaved and oxidized to CO2 (24).
Hydrogenotrophic methanogenesis is a seven-step process, which results in the carbon group progressing through the pathway via attachment to various coenzymes and finally getting reduced to CH4 (31). The pathway commences with the binding of CO2 with methanofuran (MF) and the reduction of CO2 to a formyl group with ferredoxin (Fd) acting as the electron donor (25) and formyl-methanofuran dehydrogenase (fmd) as the catalyst for the reaction (24). In the second step of the pathway, the formyl group of the formyl–MF complex is transferred to tetryahydromethanopterin (H4MPT) (32). In step three, the formyl group of formyl–H4MPT is converted to methenyl–H4MPT by the enzyme 5,10-methenyltetrahydromethanpterin cyclohydrolase (31). As part of steps four and five of the pathway, coenzyme F420 (F420 H2) acts as the electron donor on two occasions, firstly converting the methenyl group to a methylene group (step four) followed by a further reduction to a methyl group (step five) resulting in the formation of methyl–H4MPT (25). The oxidation of F420 is catalyzed by F420-reducing hydrogenase (33). In the sixth step, the methyl group is transferred to coenzyme M (HS-CoM), with the formation of methyl-CoM catalyzed by CH3-H4MPT:coenzyme M methyltransferase (mcr) (24). The final reduction step, catalyzed by Methyl-coenzyme M reductase (mcr), reduces methyl-CoM to CH4 (25). Coenzyme B (HS-CoB) provides the electrons for the final step of the pathway and combines with the cleaved HS-CoM forming the heterodisulfide molecule CoM-S-S-CoB as the end product (24) along with CH4.
The hetrosulfide end product, CoM-S-S-CoB, is returned to the reduced molecules HS-CoB and HS-CoM via the cytosol-encompassed hydrogenase–heterodisulfide reductase complex (MvhADG–HdrABC) (34). Electron bifurcation of H2 results in the reduced products HS-CoB and HS-CoM and a reduction of ferredoxin for the methanogenesis cycle (35). A further supply of reduced ferredoxin is created from the energy produced during the translocation of sodium (Na+) ions into the cell via membrane-bound hydrogenases, Eha and Ehb, oxidizing H2 (34, 35).
Expressions of the methanogenic output of ruminant livestock
The methanogenic output of an animal is predominantly reported in four different ways. Enteric CH4 emissions, reported in grams (g/day) or liters (l/day), can be expressed as daily CH4 emissions (DME). However, DME is largely influenced by an animal's voluntary feed intake, as the quantity of feed an animal consumes will influence the availability of substrate in the rumen for fermentation. Ratio expressions, relative to feed intake, had previously been advocated to overcome the influence of dry matter intake (DMI) on DME (36). Enteric emissions can therefore be expressed as a proportion of feed intake, known as CH4 yield (MY; g/kg of DMI). Enteric emissions can also be expressed per unit of animal product produced, which is referred to as CH4 intensity (MI; g/kg of milk yield or carcass weight). In growing animals, MI is also reported per unit of average daily gain (MADG; g/kg of ADG), an indicator of the environmental efficiency of animal growth. More recently, the concept of residual CH4 emissions (RME; g/day) has been proposed by Herd et al. (37) and can be defined as the difference in the animal's actual and expected CH4 output, based on its level of feed intake and body weight (38). The RME index was initially derived from the linear regression of DME on DMI (37); however, more recently, it was calculated as the multiple linear regression of DME on DMI and body weight (38).
Sources of variation in enteric methane emissions
Daily CH4 emissions are a variable trait with coefficients of variation (CV; %) of 12.7–19.2% reported in large populations of beef cattle (36, 38). However, the underlying biological mechanisms contributing to inter-animal divergence in enteric emissions are not yet fully understood, although factors including host genetics, voluntary feed intake, dietary composition, the rumen microbiome, and digestive tract physiology are likely influencers. Furthermore, the methodology used to estimate enteric emissions may also influence our interpretation of the methanogenic output of an animal.
Methane output is a heritable trait indicating that it is under some degree of host control with heritability estimates ranging from 0.19 in Danish Holstein cows [n = 750; (39)] to 0.30 in Australian Angus cattle [n = 1,060; (36)] reported for DME. The heritability of MY, MI, and RME has been estimated as 0.20, 0.25, and 0.19 in beef cattle (40) and ranged from 0.23 to 0.30, 0.33 to 0.42, and 0.18 in dairy cows (40, 41), respectively. In addition, in some studies, breed has been shown to have a significant impact on DME (42, 43). However, when emissions were reported as MY in the previous studies, the effect of the breed was insignificant, indicating differences in DME were likely influenced by inter-animal variation in DMI. Indeed, DME has been shown to be both genetically and phenotypically correlated with DMI (38, 40, 44) with up to 85 and 86% of the variation in DME explained by DMI based on the meta-analysis of published literature by Ramin and Huhtanen (45) and Hristov et al. (46), respectively. Intuitively, the relationship of DME with DMI is to be expected as a greater volume of feed enters the rumen, there will be an increase in ruminal fermentation and production of methanogenesis substrate.
The structure of the rumen microbiome has explained 15–40% of the variation in CH4 output in some studies (39, 47), although, it has not yet been determined if the composition of the rumen microbiota influences the methanogenic output of the animal or vice a versa. As stated, it is assumed that the presence of individual microbes in the rumen is determined by both ruminal conditions supporting their abundance and the ability of certain microbes to adapt their metabolism to the prevailing conditions in the rumen (48). Some members of the rumen methanogen community have been shown to be heritable (39, 49, 50); however, further work is needed to determine the biological principles of host control over the rumen environment.
The composition and digestibility of the diet offered to ruminants have a major impact on methanogenesis by altering H2 concentrations in the rumen, the availability of substrates for fermentation, and the ruminal fermentation profile, which will ultimately influence the availability of substrates for methanogenesis (26, 48). For example, diets high in starch, in comparison to forage, result in elevated production of propionate in the rumen, which results in less CH4 being produced per unit of feed intake (10, 26), likely due to the effects of cereals on ruminal pH and passage rate (48). In addition, the digestive physiology of an animal may also influence its methanogenic output, with low MY sheep shown to have a smaller rumen (51).
The rumen microbiome and methanogenesis
As stated, methanogenesis facilitates ruminal fermentation by reducing the concentration of dissolved H2 through the synthesis and eructation of CH4 from the rumen (13, 22). Therefore, methanogenesis can be viewed as having a homoeostatic role within the rumen by regulating the concentration of H2. However, as the rate of methanogenesis is reduced, prevailing conditions within the rumen favor microbial fermentation pathways, capable of consuming H2, at the expense of H2-yielding pathways, the net effect of which leads to less H2, and ultimately CH4, being produced per unit of feed fermented (48). It is also assumed that the presence of individual microbes in the rumen is determined by both ruminal conditions supporting their abundance and/or the ability of certain microbes to adapt their metabolism to the prevailing conditions in the rumen (48). As a result, the composition of the rumen microbial community, including the methanogen cohort, is a reflection of prevailing conditions within the rumen.
The composition rather than the size of the methanogen community in the rumen is closely associated with the methanogenic output of an animal (30). For example, an increased abundance of members of the Methanosphaera genera was observed in low-CH4-emitting cattle (52–54) and sheep (55, 56). In addition, within the Methanobrevibacter genus, Danielsson et al. (52) reported an increased relative abundance of M. gottcshalkii and M. ruminantium associated with high and low-CH4-emitting dairy cows, respectively. Similarly, an increase in the abundance of M. gottcshalkii was reported in rumen samples obtained from high MY sheep (56), while an increased abundance of the M. RO clade was observed in low RME beef cattle (54).
Martínez-Álvaro et al. (50) proposed that a more diverse methanogen community and an expression of methanogenesis pathways, leading to an increase in competition for methanogenesis substrates among rumen archaea, were associated with a reduced CH4 output. In support of this, an increased abundance of methylotrophic methanogens, such as Methanosphaera, in low-CH4-emitting animals likely is a result of these methanogens having a competitive edge over other groups of methanogens due to their lower H2 requirement for methanogenesis (57, 58). Equally, variation within the Methanobrevibacter genus, between high and low-CH4-emitting animals, has also been considered a product of H2 dynamics within the rumen. The Methanobrevibacter genus can be segmented into two predominant subgroups, the SGMT (M. smithii, M. millerae, M. thaueri, and M. gottschalkii) and the RO clade (M. ruminantium and M. olleyae), with the SGMT clade capable of synthesizing both mcrI and mcrII and the RO subgroup possessing only mcrI (30, 59). The expression of both mcrI and mcrII is regulated by H2 availability in the rumen, with mcrI and mcrII expression occurring in the presence of low and high concentrations of ruminal-dissolved H2, respectively (60), which, as depicted by Danielsson et al. (52), likely gives the SGMT clade a competitive advantage in the presence of a greater availability of H2.
The presence of individual bacteria in the rumen will be dependent on their ability to alter their metabolism to adapt to the prevailing rumen conditions. Rumen bacteria vary greatly in substrate specificity as well as different groups of bacteria that are associated with H2 production and utilization (61). In sheep, Kittelmann et al. (55) identified three different ruminotypes associated with CH4 emissions. Ruminotype Q and S were correlated with low-CH4-emitting sheep, and they harbored higher abundances of bacterial communities associated with producing propionate and a combination of lactate and succinate, respectively. Ruminotype H contained an increased abundance of bacteria belonging to the Firmicutes phylum, including the families Ruminococcaceae, Clostridiales, and Lachnospiraceae, which contains known producers of CO2 and H2 (42, 62). In contrast, an increased abundance of lactate-producing bacteria, such as Sharpea, Kanderila Olsnella, and Intestinibaculum has been reported in low-CH4-emitting sheep (55, 63) and beef cattle (54). Coinciding with the relationships of the ruminotypes described by Kittelmann et al. (55), Wallace et al. (64) reported a four-fold increase in the abundance of members of the succinate-producing bacterial family Succinivibrionaceae in low- compared to high-CH4-emitting beef steers. In addition, high-CH4-emitting cattle have been shown to possess a rumen microbiome characterized by an elevated abundance of the butyrate- and formate-producing bacteria Pseudobutyrivibrio and Butyrivibrio (50, 54).
Metabolic hydrogen (H) is incorporated into the synthesis of propionate in the rumen with succinate and lactate being the known precursors of the VFA (65). In contrast to this, the stoichiometric calculation of rumen fermentation indicates a net production of metabolic H associated with the synthesis of acetate and butyrate (66) with formate estimated to contribute to 18% of CH4 produced in the rumen (67). An increased abundance and/or expression genes involved in the acrylate pathway, including lactate dehydrogenase, lactyl CoA transferase, lactyl CoA dehydrogenase, acyl CoA dehydrogenase, and propionate CoA transferase, has been reported in low MY sheep (63). Greening et al. (68) reported the increased expression of acetyl-CoA synthase and fumarate reductase to be associated with a low-CH4 phenotype in sheep. Thus, the presence of individual rumen bacteria and a varied expression of pathways associated with H2 utilization and production are associated with the quantity of CH4 in animal produce. Figure 1 details the composition of the rumen microbiota of low-CH4-emitting cattle based on the findings of Smith et al. (54).
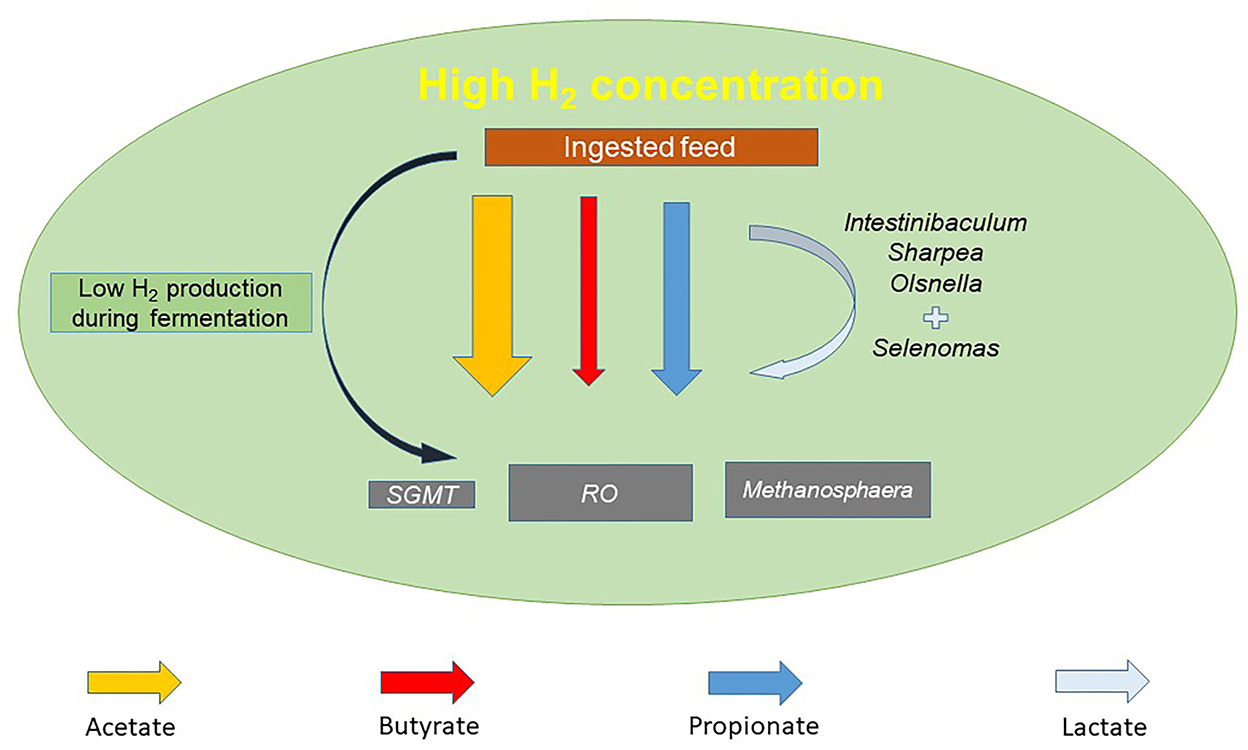
Figure 1. An overview of the rumen microbial community and fermentation profile of a low residual methane emissions (RME) phenotype based on the findings of Smith et al. (54). Differences in the size of the shapes representing individual volatile fatty acids (VFAs) and rumen methanogens are reflective of the dominant VFAs methanogen genera in low RME animals.
Protozoa have a role in predicting the methanogenic output of an animal as they are key H2 producers in the rumen (69). A strong positive linear relationship between log10 protozoal numbers and MY was reported in the meta-analysis of rumen protozoa experiments reported by Guyader et al. (70). Additional meta-analysis studies have estimated defaunation to reduce CH4 emissions by 11% but have shown insignificant decreases in methanogen numbers (71). The contrast in reports could be explained by the discovery of the adhesion-like protein (Mru_1499) in M. ruminantium M1. The Mru_1499 protein which permits the binding of M. ruminantium M1 to the surface of a wide range of rumen protozoa and also to H2-producing Butyrivibrio proteoclasticus suggests that this methanogen may be capable of continued methanogenesis after defaunation (72). However, the reductions in CH4 output associated with rumen defaunation have been reported to be variable, with the practice not being recommended as a mitigation strategy due to its potential negative impact on ruminal feed degradation (9). In addition, the effects of ruminal defaunation on DME have been observed to be short-lived based on data generated from long-term studies (69).
The refaunation work conducted by Belanche et al. (73) showed increased CH4 emissions in sheep as they progressed from being protozoa-free to monofaunated and fully faunated. Belanche et al. (73) also showed a greater diversity and 14.4 times greater total abundance of protozoa in their fully faunated animals; however, they did not report statistical differences between the groups in terms of CH4 emissions or concentration of methanogens. Furthermore, Kittelmann et al. (55) did not report any distinctive clustering of different ciliate protozoal communities in low- and high-CH4-emitting sheep when conducting amplicon sequencing analysis.
Studies in sheep have failed to define clear differences in the clustering of fungal communities of high- and low-emitting animals (55), while studies in dairy cattle have failed to define a definitive relationship between the fungal populations present in rumen samples and CH4 emissions (74). In the study by Cunha et al. (74), 73.19% of the fungal samples were identified as unclassified. Therefore, poor taxonomical identification by reference database could be a contributory factor toward the lack of reported correlations between members of the rumen fungal community and methanogenesis. Equally, anaerobic fungi are more commonly found attached to feed particles and thus may be under-represented in studies investigating the microbial community associated with the fluid fraction of the rumen. Work with anaerobic digesters inoculated with fungi originating from the rumen of fistulated cattle showed a positive correlation between fungal numbers and CH4 generation (75), perhaps indicating the concentration of the fungal population as a whole to be associated with CH4 output.
Enteric methane measurement technologies for ruminant livestock
The eructation and release of CH4 originating from the rumen has led to a variety of techniques being developed to estimate the enteric emissions of livestock. The respiration chamber (RC), the sulfur hexafluoride (SF6) tracer method, and the GreenFeed Emissions Monitoring System (GEM) are the most commonly used techniques for estimating enteric emissions in cattle (76). However, as the RC cannot be used to estimate the emissions of animals at pasture, the focus of this review will be on the SF6 tracer method and GEM. The RC and additional enteric measurement techniques for ruminant livestock, such as the handheld laser CH4 detector and sniffer technique, have been extensively reviewed by others (28). However, as the RC has traditionally been considered the gold standard method for estimating enteric emissions (77), the method, along with the SF6 and GEM, is discussed as part of a comparison between the different technologies utilized to estimate enteric CH4 emissions from cattle.
Sulfur hexafluoride tracer technique
The SF6 tracer technique for the measurement of enteric CH4 emissions was developed by Zimmerman (78), and the use of the method was first published by Johnson et al. (79). An extensive set of guidelines describing the best practice for the use of the SF6 method has been published by the Global Research Alliance (GRA) (80). The fundamental concept of this method relies on the biological inertness of SF6 gas and its comparable dispersion rate from the rumen to that of CH4 (81). Based on such attributes, DME is estimated from the concentration of the mixture of SF6 and CH4 in an animal's breath.
Permeation tubes of SF6 gas, with a known release rate, are administered to the rumen of each animal (82). The release rate of each permeation tube is assessed, prior to administering to each animal, by heating the tubes to 39°C in a water bath and measuring daily mass fluctuation until stabilization (83). After administering the permeation tubes, animals are fitted with a collection apparatus consisting of a harness fitted with pre-evacuated collection cylinders connected via a small diameter sample tubing positioned near the nose and mouth of the animal (28, 78). Gases are typically drawn into collection cylinders under partial vacuum (84). Collection tubes are changed normally every 24 h for the determination of gas concentrations using gas chromatography (GC). Samples of the background air are also collected to determine and discount environmental concentrations of CH4 and SF6 (85).
The below equation is used to determine DME with the differences between the concentration of both gases (SF6 and CH4) in the collection cylinder, attached to individual animals, and the background cylinders accounted for the following:
Equation adapted from Williams et al. (86).
RCH4 = estimated enteric CH4 per animal (g/animal/day); RSF6 = previously determined release rate of SF6 from the permeation tube (mg/d); CH4M = measured concentration of CH4 in collection cylinders fitted to the animal; CH4BG = measured concentration of background CH4 in background collection cylinders; SF6BG = measured concentration of background SF6 in background collection cylinders; SF6M = measured concentration of SF6 in collection cylinders fitted to animal MWCH4 = molecular mass of CH4; MWSF6 = molecular mass of SF6; 1000 = conversion factor to account for differential units for SF6 (ppt) and CH4 (ppm).
GreenFeed emissions monitoring system
The GEM (C-Lock Inc., Rapid City, USA) created by Patrick Zimmerman (87, 88) is a more recently developed technology for the estimation of enteric emissions from ruminants, with the ability to measure CH4, CO2, hydrogen sulfide (H2S), oxygen (O2), and H2 from ruminants. It is a non-invasive web-based system that estimates gaseous emissions from individual animals with the use of short-term breath measurements collected throughout the day (89).
A diagram of both the indoor and outdoor pasture-based GEMs is presented in Figure 2. The GEM consists of a head chamber, feed dish, and automatic diet feeder equipped with radio frequency identification (RFID) sensors to identify individual animals fitted with RFID tags (90). When the animal approaches the feed dish, each animal is identified by the GEM via their unique RFID tag. A small amount of “bait” feed is dispensed to attract and encourage animals to remain in the system for >3 min (89). As the animal consumes the bait feed, an extractor fan, positioned at the top of the unit, draws a mixture of air that is both exhaled and surrounding the animal's head through the unit and out of an exhaust position at the top of the system (81). Airflow is calculated using a hot film anemometer with a subset of air sampled prior to the outlet (28), with gas concentrations measured using non-dispersive infrared (NDIR) sensors embedded beneath the feed dish (87). Emission measurements are normally taken over a period of 3–7 min, spread across several time points throughout the day, and are dependent on the animal's voluntary visitation to the machine (28), with data uploaded to a web-based interface on an hourly basis.
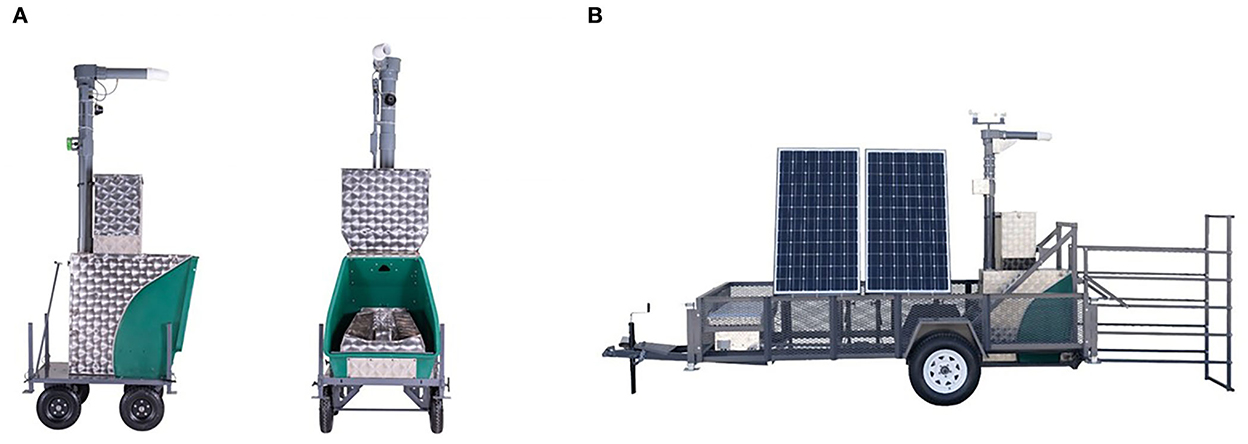
Figure 2. The Greenfeed Emissions Monitoring (GEM) system for (A) indoor and (B) outdoor use. Image sourced from C-Lock Inc.
The concentration of gases emitted by an animal is reported as a gas flux (concentration of an individual gas emitted over a defined period of time; g/day) with the below three equations performed every second (91):
Equation 1: Calculation of airflow adapted from Huhtanen et al. (91).
(i) = calculation at any time; Qair = volumetric airflow rate (l/min) calculated on a dry gas basis at 1 unit of atmospheric (atm) pressure; 60 = time conversion; Vm = velocity of air at the center (m/s) of the pipe which is automatically adjusted by sensors to 1 atm pressure; Vadj = is a correction factor (0.88) to account for the Vm to an average pipe velocity; Ap = cross-sectional area of the pipe (0.00811 m2); 1,000 = unit conversion.
Equation 2: Calculation of gas concentration adapted from Huhtanen et al. (91).
(i) = calculation at any time; Qc = volumetric flow rate of individual gases (l/min); Cp = capture rate of air; Conc = concentration of captured gas; BConc = concentration of background gas; Qair = volumetric airflow from equation 1; 106 = parts per million (ppm) conversion for gas concentration.
Equation 3: Calculation of gas flux, based on ideal gas law, adapted from Huhtanen et al. (91).
(i) = calculation at any time; Qc = volumetric flow rate of individual gases (l/min) from equation 2; 273.15 = temperature equivalent in Kelvin; Tair = air temperature (°C); ρc = density of investigative gas at 273.15 K and 1 atm (0.717 and 1.911 g/L for CH4 and CO2).
A comparison of methodologies for estimating enteric emissions in a research setting
When operated optimally, all gaseous emissions emitted from the animal (exhaled, eructed, and flatulence) can be quantified with the use of the RC (77). However, the RC is limited to only estimating emissions from a small number of animals within an indoor setting. In addition, animal feeding behavior can be altered by the confined conditions of the RC (28, 77), which may not represent a true reflection of an animal's inherent level of voluntary feed intake and DME.
Both the SF6 and GEM method benefit from their ability to be applied in a more natural setting, including at pasture (92, 93), thus having a limited impact on the voluntary feed intake of an animal. Both methods are substantially cheaper when accounting for the greater number of animals that can have their emissions estimated. However, with the SF6 technique, animals require daily handling for the replacement of collection cylinders, which may alter feeding behavior (94). The SF6 method is labor-intensive and very much dependent on the availability of local expertise to manage the equipment (82). In addition, the permeation rate of SF6 from boluses administered to animals can vary (28); however, modifications to the technique, such as the addition of a flow restrictor as proposed by Deighton et al. (84), have improved accuracy. The SF6 method is also noted as being a less robust technique with frequent equipment failure (95).
In contrast to the SF6 tracer method, the GEM is more user-friendly and has the ability to test a greater number of animals simultaneously. However, the GEM is reliant on individual animals voluntarily visiting the unit. Therefore, animals will require an acclimatization period to ensure habituation and regular attendance at the GEM (93). In addition to this, the effects of the bait feed, used to encourage animals to use the GEM, on CH4 output must also be accounted for, particularly when investigations are conducted at pasture (28). Furthermore, emission data are not continually captured from each animal with the method reliant on the collection of measurements over several days. It is reported within the literature that animals will be required to visit the GEM 30–50 times for >3 min or have the emissions evaluated over 2–5 weeks (89, 96–98) for an accurate estimation of DME. With the use of the GEM, it is imperative that the methodology implemented by researchers promotes an even visitation to the unit over a 24h period to reflect the diurnal pattern of enteric emissions (77). Recently, some authors have advocated for the use of daily CO2 emissions (DCE; kg/day) as a proxy for DMI due to the linear relationship observed among both traits (99). As DCE can be measured with the use of the GEM, this technology may have a subsequent benefit in allowing for an approximate estimation of the voluntary feed intake of ruminants at pasture.
Between animal variation for CH4 output (both DME and MY) tends to be lowest with the use of the RC (94, 100); however, some studies have shown the opposite for the GEM (76, 101). A lower DME when RCs are utilized as the reference methodology for quantifying enteric emissions in comparison with both the GEM and SF6 method has been reported in some studies (94, 100, 101), which may be reflective of the impact of the RC on animal feeding behavior. For example, in the meta-analysis of forage-fed cattle conducted by Jonker et al. (94), the mean DMI and DME for cattle measured in the RC (8.2 kg/day; 187 g/day) were observed to be substantially lower in comparison to when it was quantified with the GEM and SF6 method (12.1–13.3 kg/day; 235–292 g/day). However, when a degree of feed restriction is applied during the estimation of emissions with all techniques, an increased DME was observed with the use of the RC in comparison with the GEM and SF6 method (76), which in part, is likely reflective of CH4 released via flatulence being accounted for with the RC.
Velazco et al. (102) reported no statistical difference in CH4 output estimated by the RC or GEM, with a strong and moderate correlation between methods for DME (r = 0.85) and MY (r = 0.58). Similarly, no difference in MY and comparable CV % was detected when measurements were performed on dairy cows using the modified SF6 and RC (84). Estimates of MY between the GEM and RC did not differ for low RFI animals in Alemu et al. (101). A weak and non-significant correlation between the RC and GEM for estimates of MY (r = 0.32) was detected by Doreau et al. (76) however, a strong relationship was determined for the SF6 and GEM (r = 0.72). Garnsworthy et al. (100) reported a correlation of (r = 0.87) and (r = 0.81) for SF6 and GEM estimates of DME with the RC, respectively.
Poor agreement in estimates of MY between the SF6 and GEM has been reported (76, 94) while DME was observed to be moderately correlated (r = 0.40) (100). Differences in estimates of enteric emissions between both methods, could be due to CH4 which is absorbed in the bloodstream and exhaled with it currently unknown if SF6 and CH4 diffuse across the rumen wall at the same rate (76). The CV% for CH4 output has been reported to be greater with the SF6 method in comparison with the GEM (76, 90, 100). However, the GEM will require an increased measurement period and sample size, in comparison with the SF6, to ensure animals attend the unit an appropriate number of times to ensure accurate data collection (28).
Both the SF6 and GEM can accurately estimate enteric emissions from ruminant livestock, with the ultimate choice of methodology reflective of the type of research being conducted (28). However, regardless of the method deployed, rigorous calibrations are warranted to ensure accurate data are collected (28).
Methane mitigation strategies for pastoral-based beef cattle production
Reducing age at slaughter, and thus decreasing the lifetime emissions of an animal, has been advocated as one of the most effective CH4 reduction strategies for beef cattle production (103, 104). A reduction in the lifetime emissions of the animal can be achieved via the implementation of practices that directly hinder the methanogenesis process and thus reduce the quantity of CH4 an animal emits on a daily basis. Strategies that reduce daily CH4 emissions will be of dual benefit in a calf–cow production system as they can likely be utilized to reduce the quantity of CH4 emitted from both the cow and calf. Alternatively, strategies that increase the daily weight gain of growing animals have the potential to reduce the time it takes for cattle to reach slaughter weight, which in turn will decrease the overall quantity of CH4 an animal emits over their lifetime (103). Indeed, in the latter scenario, a slight elevation in DME, associated with the necessary increase in DMI likely required to facilitate a superior growth rate, can be tolerated as long as a reduction in lifetime emissions is achieved.
Both alterations to the diet offered to beef cattle and genetic selection have the potential to reduce the lifetime emissions of beef cattle. Improving the quality of the diet offered to ruminant livestock will likely increase ADG, but depending on the strategy employed, it may also lead to a direct reduction in DME. Equally, through animal genetics, it may be possible to select animals with an inherently lower methanogenic output or enhanced growth rate. In addition, the simultaneous selection for improved animal growth rates and reduced CH4 output may be feasible with the use of a multitrait genetic selection breeding program. Furthermore, an anti-methanogenic vaccine would be mutually beneficial for both breeding and finishing animals. Below, a review of the most effective and practical CH4 abatement strategies for pasture-based beef cattle production within temperate climatic regions is presented.
Dietary-based methane mitigation strategies
The delivery of even some of the most potent antimethanogenic additives to pasture-based livestock will be a challenge for the sector. Some additives may be included as part of a supplementary ration offered during the finishing period, included as part of a winter feeding regime or supplemented to the lactating beef cow. Nonetheless, with forage accounting for 90% of the diet of some pasture-based beef cattle production systems (105), management of the grazing sward, to either reduce enteric CH4 emissions or boost animal growth rates, will likely be the most practical means of reducing the lifetime emissions of beef cattle. A summary of the proposed mode of action for dietary-based CH4 abatement strategies, discussed in this review, is presented in Table 2.
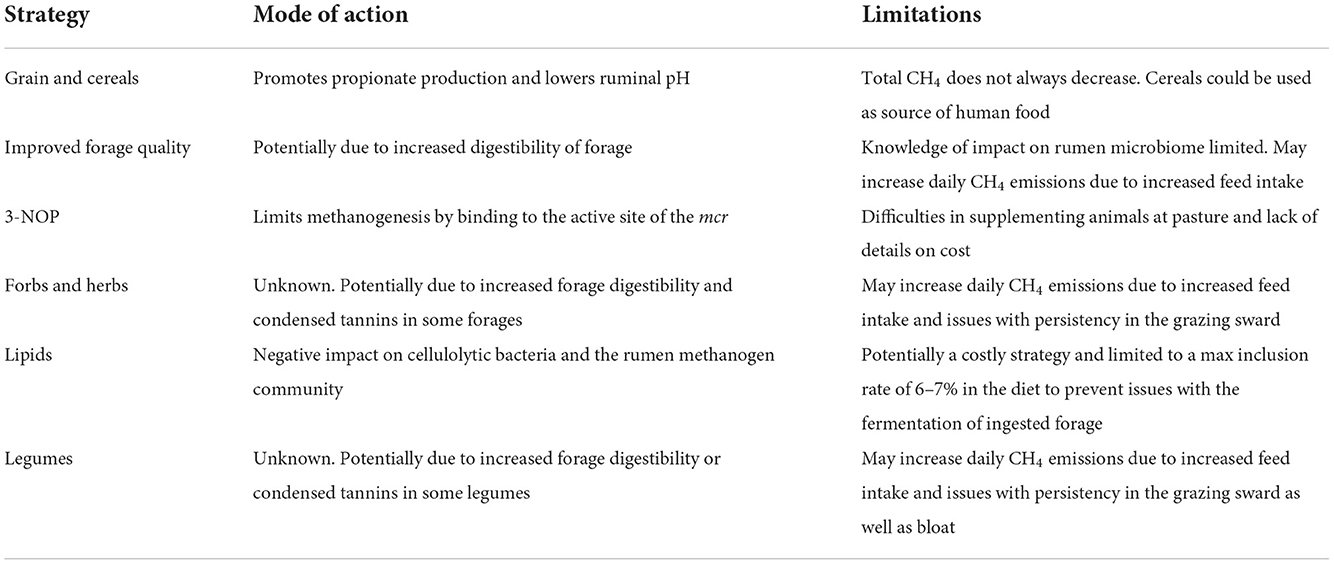
Table 2. Description of the antimethanogenic mode of action of dietary-focused methane abatement strategies.
Management of the grazing sward
In the rumen, the fermentation of the fiber is a complex process, involving a combination of fibrolytic microbes (5). An increase in the concentration of fiber within grazed forage can negatively influence the ruminal fermentation of ingested plant matter due to its slow and sometimes indigestible nature (106). In addition, a reduction in sward digestibility has the potential to increase gut fill, which will likely result in a reduced voluntary feed intake (107) and, potentially, animal productivity. Equally, an increased fermentation of fiber promotes a greater acetate:propionate (A:P) ratio within the rumen, which is known to facilitate a greater rate of methanogenesis per unit of feed intake (108).
Low pre-grazing herbage mass (HM) swards (due to the plant being in a stage of regrowth) have been shown to have lower structural fiber content and greater digestibility (109, 110). As a result of their increased digestibility, low HM swards have been shown to facilitate greater consumption of grazed forage and concurrent positive responses to animal growth rates in some studies. For example, Boland et al. (111) observed an average increase in ADG of 17% when the herbage mass of the grazing sward, offered to Limousin X heifers, reduced from 2,000 to 1,300 kg of DM and 3,200 to 2,800 kg of DM. Although HM had no impact on DME, the environmental efficiency of animal growth improved at lower herbage masses, with a 17 and 22.6% reduction in MADG observed in 1,300 and 2,800 kg of DM swards, respectively. In addition, increased production of VFA was observed on the low HM swards. In contrast to this, however, Doyle et al. (112) recently observed a reduced ADG due to lower consumption of feed, when yearling steers grazed swards with an HM of 1,500 kg of DM in comparison to 2,000 kg of DM. As a result, further analysis of the effects of pre-grazing HM on the lifetime emission profiles of grazing beef cattle is warranted. Equally, the effect of HM on the production of animal protein per unit of land area must also be considered. At a lower HM, the reduced availability of forage will decrease the number of animals that can be produced per unit of land area. However, decreasing the time it takes to slaughter animals can free up land, which can subsequently be utilized to graze additional animals, leading to an increased overall production of animal protein per unit of land and potential improvement in farm profitability.
The nutritional quality of grazed forages can differ between extensive and intensive grassland production systems. Both Fraser et al. (113) and Richmond et al. (114) observed an increased structural carbohydrate content of uphill swards in comparison with well-managed lowland swards. As expected, due to differences in the nutritional quality of both sward types, animals grazing the lowland swards had higher growth rates, which were likely supported by greater consumption of forage. While daily DME was increased in lowland swards due to an elevated feed intake, greater ADGs reduced MADG in both studies by 25–38%.
Grazing platforms with higher stocking rates (i.e., number of animals/livestock unit per ha) have been shown to produce higher quality forage due to pastures being grazed at a lower sward height (115). Equally, rotational grazing is often utilized as a management practice to target grazed grass when it is in a stage of regrowth. In spite of this, there are conflicting reports of the effect of both stocking rate and grazing management on the quantity of CH4 emitted by individual livestock. Pinares-Patiño et al. (116) reported no overall difference in DME or MADG when stocking rates increased from 1.1 to 2.2 LU/ha. Similarly, Chiavegato et al. (117) reported no difference in enteric CH4 output of lactating Angus cows between stocking rates of 1 and 2.5 cows/ha. However, rotational grazing systems have been shown to lower the DME of beef heifers and support higher growth rates (118). Conflicting reports have been observed in sheep, with animals grazing within a continuously stocked system shown to have an increased ADG and reduced CH4 intensity (119), albeit similar results have not been replicated in grazing beef cattle. As a result, for beef cattle, it would appear that grazing management is likely to have a greater influence on the enteric CH4 output of individual animals in comparison with the stocking rate, although more research is required in this area.
Methane mitigation potential of different varieties of perennial ryegrass
Perennial ryegrass (PRG) is one the most common species of grass utilized in pasture-based livestock production due to its superior yield and persistence with the grazing platform (5). With selective breeding, PRG cultivars with an increased water-soluble carbohydrate (WSC) content are now currently available (120). Water-soluble carbohydrates are rapidly fermented in the rumen and have been shown to promote a greater production of propionate at the expense of acetate and lower ruminal pH (121, 122). As a consequence of the aforementioned alterations to the ruminal fermentation profile, increasing the WSC content of a forage-based diet has been shown to reduce enteric CH4 emissions per unit of dry matter digestibility (DMD) by up to 14% in vitro (123). Furthermore, in vivo, offering forages with an increased WSC:NDF to ruminant livestock has been associated with reduced methanogenic output and is shown to account for some 38% of the variation in MY across studies (124). Although data are scarce in beef cattle, elevated growth rates have been reported in sheep (121, 125) offered forages with an elevated WSC content. Reports on the CH4 output of animals grazing swards with an increased WSC have been mixed, with some (125) but not all (126, 127) studies noting a reduction. Based on the literature, reductions in CH4 output have only been observed when the WSC:NDF of PRG varieties was substantially elevated. Nonetheless, if the positive effect of high WSC PRG on the growth rate of lambs can be translated to beef cattle, high-sugar grasses may have the potential to reduce days to slaughter and the lifetime emissions of beef cattle.
As discussed in subsequent sections, increasing the lipid content of the diet offered to ruminants is one of the most established CH4 abatement strategies for ruminant livestock (9, 128). Researchers in New Zealand have made strides to increase the fatty acid content of PRG via gene modification (129). Promising in vitro data suggest that high lipid grass can reduce enteric CH4 production by ~15% without impacting total VFA production (130). Recent data also suggest minimal differences in the establishment between high and low PRG swards (131). Pending results from in vivo trails, the utilization of high-lipid grasses would be an attractive CH4 mitigation solution for grass-based livestock production systems.
Incorporating clover into the grazing sward
Both white (Trifolium repens) and red (T. pratense) clovers are often included in the grazing sward due to their unique ability to fix atmospheric nitrogen (N) into usable means for plants and thereby increase herbage production (132–134). As a result, the incorporation of white clover into the grazing sward is considered a cost-neutral nitrous oxide (N2O) mitigation strategy for temperate grassland production systems (103).
The fermentation of both red and white clover has been associated with a reduced level of CH4 production in comparison with PRG and other grass species in vitro (135, 136). Furthermore, a reduction in the total number of ruminal methanogens (in vitro) and a reduced relative abundance of Methanobrevibacter (in vivo) have been observed with red and white clover, respectively (136, 137). However, data on the antimethanogenic benefits of clover in vivo have to date been limited, with studies conducted in beef cattle lacking. In both grazing (dairy cows) and indoor feeding trials (sheep and dairy cows) investigating the antimethanogenic effects of red and/or white clover, elevated DME has been reported in some (138–140) but not all studies (141). The heightened digestibility of white clover likely results in its rapid passage through the rumen (142), facilitating a greater DMI in comparison to grasses (138, 140, 142). As DME is strongly influenced by feed intake (45, 46, 143), the observed increase in DME in some studies, associated with ruminants grazing white clover/PRG (WCPRG) swards or offered increasing amounts of clover, is likely due to the legumes' ability to support higher feed intakes. To date, reported improvements in the growth rate and milk production have been reported in grazing sheep and dairy cattle (144–147), but not beef cattle (148).
It is evidentially clear from the literature that there is a dearth of published data on the effects of clover on both the performance and methanogenic output of beef cattle. Nonetheless, due to N2O mitigation benefits associated with the legume, clover is likely to contribute to a reduction in total on-farm emissions. Furthermore, work is currently ongoing to increase the tannin content of white clover, which may not only help alleviate the incidence of bloat in grazing ruminants but also reduce enteric CH4 emissions. Tannins are secondary plant compounds that were traditionally investigated due to their ability to reduce rumen degradable protein (149, 150), with condensed tannins (CTs) also shown to have a negative impact on rumen methanogens and reduce the degradation of plant polymers (151, 152). In comparison with other legumes, the CT content of clover plants is low, with the majority of the compound concentrated in the flower of the plant (153). However, transgenic research is currently underway to increase the availability of CTs in the leaf of white clover, which has been shown to produce 19% less CH4 than traditional varieties in vitro (154). Assuming similar results are observed in vivo, high CT white clover could be a potential mitigation solution for pastoral-based production systems, albeit the impact of modified clovers on animal productivity will require assessment.
Alternative legumes and herbs
The combination of herbs and alternative legumes as part of a mixed species grazing platform has been shown to increase N-use efficiency in dairy cows (155), reduce the burden of intestinal parasites in lambs (156), and promote greater milk yields (157) and animal growth rates (156, 158). In addition, mixed species swards have also been shown to have a lower N requirement to PRG swards (147) and reduced N2O emissions (159). As a result of the benefits to animal performance and health, as well as sward digestibility and reduced N2O emissions, interest has increased in the number of studies investigating the antimethanogenic potential of mixed species swards.
Dairy cows grazing swards, containing predominantly the herbs chicory (Cichorium intybus) and plantain (Plantago), have been reported to tentatively emit 15% less CH4 in comparison with cows grazing WCPRG pastures (155). Similarly, offering zero-grazed chicory, in comparison with WCPRG, resulted in a 37% reduction in MY, but a comparable level of DMI, in sheep (160). However, contrasting results have been reported when both herbs have been offered to sheep as part of other trials, with no effect on DME reported in some studies (161, 162). Williams et al. (163) reported increased MY and reduced milk production in dairy cows offered lucerne (Medicago sativa) and chicory in comparison with cows receiving lucerne only. However, reproductive stage chicory was offered in the previous study, which could suggest that similar to other forage species, the methanogenic output of animals offered chicory may fluctuate throughout the grazing season. Any potential antimethanogneic effects of both plantain and chicory are yet to be defined as neither has been reported to have a high CT content, but could potentially arise from a lower fiber content within herbs (164). Importantly, reports of elevated production of CH4 have been limited in the data produced to date when both plantain and chicory are simultaneously combined as part of a mixed species sward. However, similar to investigations of the CH4 abatement potential to white and red clover, there is a dearth of information on the effects of both herbs on the CH4 output of beef cattle.
Both lucerne and birdsfoot trefoil (BT; Lotus corniculatus) are alternative legumes that have been investigated in temperate regions. Lucerne, has to date, shown little promise as forage capable of reducing DME, with similar emissions to that of grasses reported in heifers (165) and goats (166). Nevertheless, in the extensive review published by Popp et al. (167), lucerne was shown to improve the weight gain of cattle across numerous studies. Although a greater number of studies investigating the effects of lucerne on CH4 in beef cattle are warranted, the reported improvements to the growth rate of beef cattle suggest that the legume may have a role in reducing the lifetime emissions of an animal by improving the environmental efficiency of animal growth. In comparison, BT is noted as having a high proportion of CTs (168). Increased milk yield and reductions to MY have been observed when BT silage was offered to dairy cows in comparison with PRG silage (169). In addition, sheep offered BT produced 33% less CH4 compared with those offered PRG, with a minimal difference in DMI (169). In vitro, a simultaneous reduction in CH4 output and decreased abundance Methanobrevibacter was observed for BT in comparison to Orchardgrass (170). However, in the latter study, a reduction in the abundance of cellulolytic bacteria Fibrobacter succinogenes and Ruminococcus flavefaciens was associated with BT. The negative impact of BT on these key cellulolytic bacteria, in particular F. succinogenes, likely originates from a limited degradation of plant polymers as a result of CTs forming indigestible complexes with plant polymers such as cellulose, hemicellulose, and pectin in the rumen (149).
Forage brassicas
Brassicas, primarily consisting of kale (Brassica oleracea L. cv. Kestrel), turnip (B. campestris L. cv. Appin), rape (B. napus L. cv. Titan), and swede (B. napus L. cv. Dominion) are annual forage crops that have traditionally been utilized in temperate geographical regions as feed sources for out-wintered livestock or during periods of herbage deficit due to summer drought (171). In comparison to grasses, forage brassicas are noted as having an increased digestibility, WSC content, and lower proportion of fiber (171, 172). As a result, the ruminal fermentation of some brassica crops has been shown to boost animal productivity (171) and illicit an alteration in the rumen fermentation profile associated with elevated production of propionate and subsequent reduction of ruminal pH (173). Sun et al. (172) assessed the CH4 mitigation potential of different brassicas against PRG in sheep. In their analysis, both forage rape and swedes were shown to have the lowest MY; however, forage rape had the least impact on DMI. As part of numerous additional studies, across all ruminant species, forage rape has been shown to promote a lower MY (174). For example, up to a 40% reduction in MY was observed in beef heifers offered winter forage rape in comparison with pasture (175). In addition in sheep, a linear reduction in both DME and MY was observed as increasing proportions of forage rape replaced PRG (0, 25, 50, 75, and 100%) in the diet. Indeed, when forage rape was offered as the sole forage in the previous study, DME and MY were reduced by 55 and 64%, respectively. The antimethanogenic effects of forage rape have also been shown to persist for over 3 months, with lambs consuming forage rape as the sole component of their diet, on average emitting 22–30% lesser CH4 per unit of feed intake in comparison with lambs offered PRG after 15 weeks (176).
Forage rape likely reduces ruminal methanogenesis due to the rapid fermentation of the crop, having a negative effect on the rumen methanogen community as a result of elevated production of propionate and lower rumen pH (176). The rumen microbial community of lambs consuming forage rape has been shown to have a greater abundance of the rumen bacteria Selenomonas and Sharpea and an increased abundance of the methanogen Methanosphaera (176), similar to the microbial profiles previously associated with low-CH4-emitting beef cattle (54) and sheep (56). In addition, a reduced rumen protozoal abundance was also observed in sheep offered forage rape (176). Some authors have also argued that secondary plant compounds in forage rape, such as glucosinolates, may reduce methanogenesis by increasing the ruminal passage rate (177). As reported by Sun (176), forage rape has also been shown to have a nitrate concentration that is 10 times greater than PRG (176), with the ingestion of nitrate in the rumen known to have a negative impact on ruminal methanogenesis (178, 179). However, nitrate is not believed to contribute to much of the antimethanogenic effects of forage rape (176). The annual persistence of forage rape will likely be its major limitation of the crop being utilized as an antimethanogenic forage source. However, the persistent reductions in daily CH4 production and beneficial responses to animal growth rates observed across numerous studies may see an increased interest in forage rape as an antimethanogenic strategy for some pasture-based beef producers.
Methane mitigation potential of dietary additives for pasture-based beef cattle
Numerous CH4 abatement dietary additives have been identified to date (128). However, supplementing pasture-based beef cattle with antimethanogenic compounds will be a challenge. High energy substrates are routinely supplemented to the diet to increase muscle growth during the finishing period, meet the energy demands of pregnancy/lactation, and overcome deficiencies in nutrient supply in some production systems (180). Therefore, the addition of lipids and other antimethanogenic compounds could be administered at different time points throughout the production cycle via a supplementary concentrate feeding regime. Although it is acknowledged that strategies directly focused on pasture will have the greatest mitigation effect within a grass-based beef system, some mitigation benefits at different stages of the animal's life may arise from the supplementation of concentrates formulate with antimethanogenic compounds. Equally, all dietary supplements can theoretically be administered to ruminant livestock during a winter housing period.
Grain supplementation to forage based diets
An increase in the digestibility of dietary constituents is known to decrease the quantity of CH4 emitted per unit of feed intake (181). This can likely be explained by the model of H2 dynamics proposed by Janssen (48), whereby a reduction of rumen pH and increased passage rate associated with concentrate supplementation is likely to have a negative impact on the rumen methanogen community, ultimately leading to a reduction in methanogenesis. In addition, the feeding of cereals elevates dissolved H2 concentrations in the rumen, which results in a redirection of metabolic H to the synthesis of propionate (48).
As alluded to in previous sections, an increase in the digestibility of the diet offered to ruminants can facilitate an enhanced level of feed intake, with increases in DMI observed in numerous studies when concentrates are offered in the place of forage (42, 182, 183). As a result, some studies have shown that when concentrates are supplemented, DME is either increased or is comparable with high-forage diets (183–185). In contrast, other studies have noted reductions in DME when the forage proportion of the diet is substituted with concentrates (42, 182, 186). However, in the latter studies, with the exception of Rooke et al. (42), although only ~1 kg difference was observed, increasing the proportion of forage in the diet had very little impact on feed intake. Therefore, under scenarios whereby a substantial increase in DMI is associated with concentrate supplementation, a subsequent elevation in DME is likely to occur. However, when supplementation has no effect on DMI, a reduction in DME is expected, with a lower MY generally observed when concentrates account for 35–40% of the diet fed to ruminants (9, 187).
Recent life cycle analysis (LCA) has shown that although MI may be less for finishing systems where concentrates are supplemented at various levels, overall emissions are lower for grass-only finishing systems due to a reduction in emissions arising from the production of animal feed (188, 189). However, in the previous study, all animals were slaughtered at a common age (20 months) with concentrate-supplemented animals producing a heavier carcass in comparison with grass-only animals. Therefore, within a system whereby animals are slaughtered based on a targeted finishing weight, supplementing concentrates to pasture-based animals can reduce the age of slaughter, albeit the impact to on farm profitability will need to be considered.
Increasing the lipid content of the supplemented ration
Traditionally fat supplementation has been utilized as a means of increasing the energy content of ruminant diets. However, supplementing the ruminant diet with fat has been shown to reduce CH4 output in numerous studies (190–194), with meta-analysis conducted by Patra (195) and Beauchemin et al. (196) indicating that for every 1% increase in the lipid content of the diet, DME and MY are predicted to decrease by 3.77 and 5.6%, respectively. The supplementation of lipids, in particular to cattle consuming a high forage diet, has been shown to have a negative impact on fiber digestion and feed intake in beef cattle (190, 191). As a result, fat supplementation is generally recommended not to exceed 6–7% (197) in an effort to negate fiber fermentation and palatability issues.
Medium-chain fatty acids (MCFAs) and polyunsaturated fatty acids (PUFAs) have been observed to have the most potent antimethanogenic capacity (11). However, due to the cost associated with the processing of MCFAs, the use of PUFAs may be a more economical option (196). The supplementation of unsaturated fatty acids was traditionally believed to reduce ruminal available H2 for methanogenesis via biohydrogenation (26). However, the biohydrogenation of unsaturated fatty acid is likely to only to absorb 1–2% of H2 production (9). The antimethanogenic effect of fats is believed to originate from the negative effect of lipids on cellulolytic bacteria leading to a reduction in organic matter digestibility (OMD) and a negative impact on the rumen methanogen community via a diversion of H2 away from methanogenesis (11, 191). Furthermore, rumen microbes possess a limited ability to ferment lipids (198). As a result, the addition of lipids to the diet, at the expense of other carbohydrate sources, likely results in a lower availability of fermentable substrate, leading to less VFA, H2, and ultimately CH4 production per unit of intake and/or digestibility (199). The supplementation of lipids has been identified as a costly CH4 mitigation strategy (103), which may limit its use due to its economic viability (10). However, the processing of crops for the global food, oil, and ethanol industries generates a source of residual byproduct (BP) plant matter, which can be utilized as cheap animal feed (200). Indeed, the nutritional value between BPs is known to vary, with some shown to have a high oil content (201). For example, dried distillers grains plus solubles (DDGS), originating from maize, have been reported to have a fat content of 9–12.7% (202–204). Replacing barley with DDGS, as part of the diet offered to beef cattle, has been shown to reduce enteric CH4 output by >19% by McGinn et al. (203). Increased demand for biodiesel has promoted a more efficient oil extraction process from maize, with the lipid content of refined DDGS reported to be 30% less than traditional forms of DDGS (205). Nonetheless, evidence from the literature still highlights the CH4 abatement potential of refined DDGS, albeit it may be slightly reduced in comparison to unrefined forms. For example, the formulation of concentrates with either DDGS or refined DDGS has been shown to support similar reductions in MY in dairy cows (206). In addition, supplementing concentrates formulated from refined DDGS to dairy cows supported a 6.4% reduction in DME with no impact on DMI or milk yield (207). Furthermore, supplementing rapeseed cake, in place of rapeseed meal, reduced MY by 7% in dairy cows (208). Therefore, increasing the fat content of the diet offered to ruminants may be economically achieved by the supplementation of BPs.
However, the high protein content of some plant-based BPs, such as DDGS, has increased N excretion in some studies (209, 210), which may offset any reductions in enteric emissions and lead to a net increase in farm gate GHGs (211). Subsequently, this issue may be overcome by formulating rations from a combination of BPs, with both Whelan et al. (212) and Condren et al. (213) reporting no differences in N excretion between dairy cows receiving a barely and soybean meal supplemented ration in comparison with a BP ration formulated from DDGS, palm kernel expeller, and soyhulls. Indeed, the same BP formulation was also shown to reduce CH4 output by ~20% (both daily and per unit of OMD) without negatively impacting members of the rumen microbiota when supplemented with a pasture-based diet in vitro (199).
Supplementation of 3-nitrooxypropanol to ruminants
The synthetic compound 3-nitrooxypropanol (3-NOP; DSM Nutritional Products Ltd., Kaiseraugst, Switzerland), Bovaer® as it is commercially known, produced by DSM, has been demonstrated to be a non-toxic antimethanogenic supplement across numerous studies in dairy and beef cattle (214–220). 3-Nitrooxypropanol binds to the active site of the mcr enzyme, rendering it incapable of completing the final methanogenesis step (221). Unsurprisingly, this supplement has been shown to negatively affect members of the methanogen population. At a 2.5-g of 3-NOP/animal/day supplementation rate, 5.6- and 5.5-fold reductions in the total methanogen population and Methanobrevibacter spp. were accompanied by a 30% in DME in the study conducted by Martinez-Fernandez et al. (216). Similarly, Romero-Perez et al. (215) observed a reduction in the total methanogen population when 3-NOP was supplemented daily at 2 g/animal.
Reductions in CH4 production as high as 59% have been reported in some studies when 3-NOP was supplemented in cattle (215). Increasing the supplementation rate of 3-NOP linearly decreases CH4 emissions (222) with an average reduction in DME and MY of 32.5 and 29.3%, respectively, reported in the meta-analysis conducted by Dijkstra et al. (223) based on a mean supplementation rate of 123 mg of 3-NOP/kg of DMI. The meta-analysis by Dijkstra et al. (223) also suggested that 3-NOP is more potent in dairy compared with beef cattle, with on average, a lower dosage (per unit of intake) capable of achieving greater reductions in CH4 output in dairy animals. However, as dairy cows are known to have a higher intake in comparison to beef animals, it may be more appropriate to compare animals based on the total dosage of 3-NOP per day rather than per unit of intake.
Overall, there have been no reports of a negative effect of 3-NOP supplementation on animal productivity in studies conducted on beef cattle. In the recent meta-analysis of some 14 studies, Kim et al. (224) noted a slight reduction in DMI in beef cattle supplemented with 3-NOP. In addition, in the early meta-analysis conducted by Jayanegara et al. (222), a small increase in gain to feed (G:F) was reported in animals receiving 3-NOP. Similarly, in their feedlot study consisting of over 4,000 beef cattle, Alemu et al. (220) reported that animals receiving a 3-NOP dosage of 200 mg/kg DM had a 2.6% reduction and a 2.5% increase in DMI and G:F, respectively. The lack of a substantial benefit to animal productivity with the reduction in enteric CH4-associated 3-NOP's supplementation is somewhat unexpected, but could partially be a result of the fact that 3-NOP does not increase the energy concentration of the diet unlike other anti-methanogenic additives, such as lipids. However, considering methanogenesis is estimated to consume 2–12% of the gross energy content of the diet consumed by ruminants (26), a positive response to ruminant performance would be anticipated as CH4 production decreases. Some authors have noted the slight reductions in DMI associated with 3-NOP could be due to the reported increase in ruminal propionate production (225). Indeed, an increase in the concentration of ruminal propionate production can have a hypophagic effect on ruminants and thus impact both satiety and hunger (226, 227). Therefore, while propionate is a more energy-dense ruminal VFA in comparison to acetate (C2 vs. C3), the lack of a substantial benefit to animal performance may be explained by the negative effect 3-NOP has on DMI and the negligent effect on GEI, which likely counteracts any potential major gains to animal productivity associated with a reduction in energy lost to methanogenesis. However, in the meta-analysis of Kim et al. (224), 3-NOP supplementation only had a negative effect on DMI, but increased propionate, in beef cattle studies. However, as eluded to by Yu et al. (225), the effect on DMI may be due to the higher 3-NOP inclusion rates utilized in beef in comparison to dairy studies, which could indicate issues with palatability at higher dosage rates. Nonetheless, this does not explain the discrepancy in the varied effects of 3-NOP on ruminal propionate and the associated impact on animal performance, as when 3-NOP was supplemented to target an 80% reduction in DME in vitro, Guyader et al. (228) observed no impact on ruminal propionate production. Subsequently, in Guyader et al. (228), an increase in other reduced fatty acids, such as valerate and caproate, along with elevated gaseous H production was observed with 3-NOPs supplementation. Coinciding with this, an increased redirection of ruminal H toward valerate and caproate production, but not propionate was observed. Consequently, when all H production and utilization pathways were assessed, only 54.3% of ruminal H was accounted for, indicating that the excess H associated with 3-NOPs supplementation was not redirected to the main ruminal fermentation pathways and was ultimately expelled as gaseous H (228). Therefore, further work is required to better understand the effects of 3-NOP's supplementation on the main rumen microbial fermentation pathways and the effects this has on ruminal H.
Van Wesemael et al. (219) confirmed the ability of 3-NOP to have a similar mitigation effect when mixed into the forage diet or supplemented in a formulated concentrate. However, the antimethanogenic effects of 3-NOP are short-lived, with enteric emissions shown to increase once supplementation has ceased (214) and the compound is no longer available within the rumen. As a result, even if it was included as part of a supplementary ration, in its current form 3-NOP will likely have minimal impact on reducing the methanogenic output of grazed beef cattle. Slow-releasing forms of 3-NOP are currently under investigation for the use of the compound within a pastoral setting (229), which may deliver some benefit in the future. Currently, Brazil, Chile, and the EU (dairy cows only) are the only countries that have granted regulatory approval for the use of 3-NOP on farms. While 3-NOP is no doubt a very promising CH4 mitigating compound, further investigation is warranted to assess the economic availability of its inclusion in ruminant diets as well as the slow-release versions of the compound that can be utilized within a grass-based system.
Anti-methanogenic vaccinations
The development and utilization of anti-methanogen vaccines, as a CH4 mitigation strategy, is an attractive prospect for the livestock industry. Indeed, the inoculation of ruminants with an antimethanogen vaccine is likely to be among the most popular mitigation strategies among livestock producers, with a high uptake at the farm level, if proven effective (230). The mode of action of an antimethanogenic vaccine is likely to be through the synthesis of antibodies, which enter the rumen via saliva and subsequently target a methanogen cellular surface antigen which inhibits the methanogenesis process (231). Antibody responses following the administration of anti-methanogenic vaccinations have been reported (232); however this has not always led to a reduction in CH4 output (233, 234). Wright et al. (235) observed a 7.7% reduction in MY after sheep were administered a vaccine formulated to target three strains of the Methanobrevibacter genus. With advances in NGS and bioinformatics, reverse vaccinology, whereby potential target antigens are detected by studying the genomes of rumen methanogens (231) may benefit the development of antimethanogen vaccines in the future. However, efforts thus far to develop an effective antimethanogenic vaccine have been limited, primarily due to difficulties in the large diversity of the methanogen community residing in the rumen (236).
Animal breeding strategies for methane mitigation
As previously discussed, CH4 output is a heritable trait presenting the opportunity to breed low-CH4-emitting animals. However, CH4 output is a complex trait under the influence of multiple factors and is both phenotypically and genetically correlated with DMI, ADG, and body weight (37, 38, 44). Therefore, directly selecting animals for a reduced DME phenotype will likely decrease animal performance and therefore be unfavorable among producers due to negative ramifications to on-farm profitability.
The selection of low-CH4-emitting cattle on the basis of ratio traits, such as MY, has traditionally been advocated as MY was perceived to be free from any association with feed intake or body weight but positively correlated with DME, under restricted feeding regimes with the use of RCs (36, 37). Subsequently, in studies offering cattle ad libitum access to both concentrate (38, 44) and forage-based diets (237), DMI and MY have been shown to be negatively correlated. As a result, the selection of animals on the basis of MY has the potential to increase the voluntary feed intake of future generations of livestock. However, the elevated feed intake associated with MY is unlikely to benefit animal performance, as a negative relationship of MY with measures of feed efficiency, such as residual feed intake (RFI; kg/day), has been revealed in studies implementing an ad libitum feeding regime (183, 237, 238). Therefore, a reduction in the DME associated with the selection of cattle for a low MY phenotype will likely occur at the expense of less efficient conversion of feed to animal protein, thus negatively impacting on-farm profitability. Therefore, the selection of animals on the basis of ratio traits should be avoided by virtue of their unpredictable response to other traits of economic importance in cattle production (18). Moreover, the effect of experimental conditions on the relationship between DMI and MY further highlights the complexities of CH4 output as a trait.
By virtue of its calculation, RME has been shown to be phenotypically and genetically independent of DMI and body weight in beef cattle but positively correlated with DME (36, 38). Recently, Smith et al. (143) highlighted beef cattle ranked as low for RME, produced 30% less CH4 (DME, MY, and MI), but had the same level of productivity (DMI, ADG, RFI, and carcass output) as high RME ranked animals. The lack of an apparent relationship of RME with traits of economic importance in cattle production makes it an attractive trait to utilize as part of the CH4 mitigation breeding program (239). Indeed, selection for a low RME phenotype has great potential to reduce the CH4 output of both future generations of breeding and finishing beef animals.
Indirectly selecting animals for enhanced feed efficiency has been advocated as an alternative selection index for reducing the CH4 output of livestock (10). Based on the literature, animals with an enhanced level of feed efficiency have been shown to produce less CH4 in some studies (101, 240–245). However, inconsistencies in the relationship between RFI and enteric emissions have been reported, with no difference in DME observed in animals with superior feed efficiency in some studies (183, 238, 246, 247).
As discussed, reducing days to slaughter is an effective CH4 mitigation strategy for beef cattle. However, slaughtering beef animals at an early age could have negative ramifications on both carcass output and quality. Berry et al. (248) proposed the trait, deviation in age at slaughter (DAGE), as an effective means of selecting animals with the enhanced genetic potential to reach slaughter weight over a shorter time frame, but produce a carcass of sufficient weight and fat cover to meet market specifications. Using records from over 2 million Irish cattle, DAGE was calculated from the regression of age at slaughter on carcass weight and fat score and was shown to have a heritability of 0.23–0.26 and both genetic and phenotypic standard deviation of 14.2–15.1 and 44.2 days, respectively. While the genetic potential exists to breed for faster-growing in-spec cattle, further in-depth analysis is needed to quantify the relationship between DAGE and CH4 production to fully verify the mitigation potential of breeding for a reduced age at slaughter.
Conclusion
With an estimated 37% of enteric CH4 produced from grazing livestock (249), mitigation strategies that can be applied at pasture are urgently needed. Indeed for beef cattle, strategies requiring regular interaction with animals are not feasible during the grazing season, albeit some could have merit when animals are housed for winter. However, due to the low profitability of beef production systems (15), a winter CH4 supplementation strategy would at minimum need to be cost-neutral to ensure uptake at the farm level. Equally, altering the composition of the grazing swards to reduce CH4 emissions could be a challenge for extensive production systems due to the lack of long-term persistency of some alternative forages within the grazing sward (147) and complications with herbicide treatment. Therefore, in the absence of an effective antimethanogenic vaccine, the genetic selection of low-CH4-emitting animals is the only abatement strategy, which is likely effective for extensive pasture-based ruminant production systems. Nonetheless, breeding low-CH4-emitting ruminants will still be a challenge due to the large numbers of animals required to develop a breeding program (250), difficulties in performance testing cattle at pasture, and low uptake of reproductive technologies in non-dairy production systems (251).
Recently, the IPCC identified the urgent need to reduce the atmospheric concentration of CH4 to limit global temperature increases (4), with the EU, United States, and other countries pledging to reduce anthropogenic CH4 emissions by 30% by 2030 as part of the Global CH4 Pledge. However, reducing global enteric CH4 emissions will be a challenge due to the increase in animal production required to meet the projected demand for animal-sourced protein. Due to the linear relationship between DME and DMI, as reported by numerous authors (44, 143, 214), any CH4 mitigation strategies that support a higher level of feed intake have the potential to increase DME. For finishing beef cattle, any increase in DME as a result of higher feed intakes could potentially be offset by improved growth rates due to the relationship between DMI and average daily gain (252), leading to a reduction in the lifetime emissions of an animal. However, for the beef breeding herd, strategies that reduce DME will be required to meet international GHG reduction commitments. To fully understand the overall effects of different CH4 abatement strategies, future LCAs will need to be conducted to evaluate the effects of each CH4 mitigation strategy on total farm gate GHG emissions.
Author contributions
All authors listed have made a substantial, direct, and intellectual contribution to the work and approved it for publication.
Funding
Funding and support from the FACCE ERA-GAS RumenPredict grant (16/RD/ERAGAS/1RUMENPREDICTROI 2017) and Horizon 2020 MASTER grant (818368) is acknowledged. PS was funded by a Teagasc Walsh Scholarship (RMIS 0364).
Conflict of interest
The authors declare that the research was conducted in the absence of any commercial or financial relationships that could be construed as a potential conflict of interest.
Publisher's note
All claims expressed in this article are solely those of the authors and do not necessarily represent those of their affiliated organizations, or those of the publisher, the editors and the reviewers. Any product that may be evaluated in this article, or claim that may be made by its manufacturer, is not guaranteed or endorsed by the publisher.
References
1. UN Department of Economic and Social Affairs, Population Division. World Population Prospects: The 2017 Revision, Key Findings and Advance Tables. Working Paper No. ESA/P/WP/248. UN Department of Economic and Social Affairs, Population Division (2017).
3. Smith P, Clark H, Dong H, Elsiddig EA, Haberl H, Harper R, et al. Agriculture, Forestry and Other Land Use (AFOLU). In: Edenhofer O, Pichs-Madruga R, Sokona Y, Farahani E, Kadner S, Seyboth K, Adler A, Baum I, Brunner S, Eickemeier P, Kriemann B, Savolainen S, Schlömer S, von Stechow C, Zwickel T, Minx JC, editors. Climate Change 2014: Mitigation of Climate Change. Contribution of Working Group III to the Fifth Assessment Report of the IPCC. Cambridge: Cambridge University Press. (2014).
4. IPCC. Climate Change 2021: The Physical Science Basis. Contribution of Working Group I to the Sixth Assessment Report of the Intergovernmental Panel on Climate Change. Masson-Delmotte VP, Zhai Pirani A, Connors SL, Péan C, Berger S, Caud N, Chen Y, Goldfarb L, Gomis MI, Huang M, Leitzell K, Lonnoy E, Matthews JBR, Maycock TK, Waterfield T, Yelekçi O, Yu R, Zhou B, editors. New York, NY: Cambridge University Press (2021).
5. Waters SM, Kenny DA, Smith PE. Role of the rumen microbiome in pasture fed ruminant production systems. In:McSweeney C, Mackie R, editors. Improving Rumen Function. Cambridge: Burleigh Dodds Science Publishing (2020). p. 591–650. doi: 10.19103/AS.2020.0067.21
6. IPCC. Climate Change 2014: Synthesis Report. Contribution of Working Groups I, II and III to the Fifth Assessment Report of the Intergovernmental Panel on Climate Change. Core Writing Team, Pachauri RK, and Meyer LA, editors. Geneva: IPCC (2014).
7. Mizrahi I, Wallace RJ, Moraïs S. The rumen microbiome: balancing food security and environmental impacts. Nat Rev Microbiol. (2021) 19:553–66. doi: 10.1038/s41579-021-00543-6
8. IPCC. Climate Change 2022: Impacts, Adaptation and Vulnerability. Contribution of Working Group II to the Sixth Assessment Report of the Intergovernmental Panel on Climate Change. Pörtner H-O, Roberts DC, Tignor M, Poloczanska ES, Mintenbeck K, Alegría A, Craig M, Langsdorf S, Löschke S, Möller V, Okem A, Rama B, editors. Cambridge; New York, NY: Cambridge University Press (2022).
9. Hristov AN, Oh J, Firkins JL, Dijkstra J, Kebreab E, Waghorn G, et al. Special topics—Mitigation of methane and nitrous oxide emissions from animal operations: I. A review of enteric methane mitigation options. J Anim Sci. (2013) 91:5045–69. doi: 10.2527/jas.2013-6583
10. Beauchemin KA, Ungerfeld EM, Eckard RJ, Wang M. Fifty years of research on rumen methanogenesis: lessons learned and future challenges for mitigation. Animal. (2020) 14:2–16. doi: 10.1017/S1751731119003100
11. Honan M, Feng X, Tricarico JM, Kebreab E. Feed additives as a strategic approach to reduce enteric methane production in cattle: modes of action, effectiveness and safety. Anim Prod Sci. (2021) 62, 1303–17. doi: 10.1071/AN20295
12. Beauchemin KA, Ungerfeld EM, Abdalla AL, Alvarez C, Arndt C, Becquet P, et al. Invited review: current enteric methane mitigation options. J Dairy Sci. (2022) 105:9297–326. doi: 10.3168/jds.2022-22091
13. McAllister TA, Newbold CJ. Redirecting rumen fermentation to reduce methanogenesis. Aust J Exp Agric. (2008) 48:7–13. doi: 10.1071/EA07218
14. Thompson LR, Rowntree JE. Invited review: methane sources, quantification, and mitigation in grazing beef systems. Appl Anim Sci. (2020) 36:556–73. doi: 10.15232/aas.2019-01951
15. Dillon E, Donnellan T, Moran B, Lennon J. Teagasc National Farm Survey 2020 Results. Athenry: Teagasc (2021).
16. Beauchemin KA, Janzen HH, Little SM, McAllister TA, McGinn SM. Life cycle assessment of greenhouse gas emissions from beef production in western Canada: a case study. Agric Syst. (2010) 103:371–9. doi: 10.1016/j.agsy.2010.03.008
17. Wall E, Simm G, Moran D. Developing breeding schemes to assist mitigation of greenhouse gas emissions. Animal. (2010) 4:366–76. doi: 10.1017/S175173110999070X
18. Pickering NK, Oddy VH, Basarab J, Cammack K, Hayes B, Hegarty RS, et al. Animal board invited review: genetic possibilities to reduce enteric methane emissions from ruminants. Animal. (2015) 9:1431–40. doi: 10.1017/S1751731115000968
19. Abbott DW, Aasen IM, Beauchemin KA, Grondahl F, Gruninger R, Hayes M, et al. Seaweed and seaweed bioactives for mitigation of enteric methane: challenges and opportunities. Animals. (2020) 10:2432. doi: 10.3390/ani10122432
20. Hobson PN, Fonty G. Biological models of the rumen function. In:Hobson PN, Stewart CS, editors. The Rumen Microbial Ecosystem. Dordrecht: Springer (1997). p. 661–84.
21. Russell JB, Wallace RJ. Energy-yielding and energy-consuming reactions. In: The Rumen Microbial Ecosystem. Dordrecht: Springer (1997). p. 246–282.
22. Morgavi DP, Forano E, Martin C, Newbold CJ. Microbial ecosystem and methanogenesis in ruminants. Animal. (2010) 4:1024–36. doi: 10.1017/S1751731110000546
23. Patra A, Park T, Kim M, Yu Z. Rumen methanogens and mitigation of methane emission by anti-methanogenic compounds and substances. J Anim Sci Biotechnol. (2017) 8:13. doi: 10.1186/s40104-017-0145-9
24. Ferry JG. Fundamentals of methanogenic pathways that are key to the biomethanation of complex biomass. Curr Opin Biotechnol. (2011) 22:351–7. doi: 10.1016/j.copbio.2011.04.011
25. Liu Y, Whitman WB. Metabolic, phylogenetic, and ecological diversity of the methanogenic archaea. Ann N Y Acad Sci. (2008) 1125:171–89. doi: 10.1196/annals.1419.019
26. Johnson KA, Johnson DE. Methane emissions from cattle. J Anim Sci. (1995) 73:2483–92. doi: 10.2527/1995.7382483x
27. Pacheco D, Waghorn G, Janssen PH. Decreasing methane emissions from ruminants grazing forages: a fit with productive and financial realities? Anim Prod Sci. (2014) 54:1141–54. doi: 10.1071/AN14437
28. Hammond KJ, Crompton LA, Bannink A, Dijkstra J, Yáñez-Ruiz DR, O'Kiely P, et al. Review of current in vivo measurement techniques for quantifying enteric methane emission from ruminants. Anim Feed Sci Technol. (2016) 219:13–30. doi: 10.1016/j.anifeedsci.2016.05.018
29. Janssen PH, Kirs M. Structure of the archaeal community of the rumen. Appl Environ Microbiol. (2008) 74:3619–25. doi: 10.1128/AEM.02812-07
30. Tapio I, Snelling TJ, Strozzi F, Wallace RJ. The ruminal microbiome associated with methane emissions from ruminant livestock. J Anim Sci Biotechnol. (2017) 8:7. doi: 10.1186/s40104-017-0141-0
31. Rouvière E, Wolfe RS. Novel biochemistry of methanogenesis. J Biol Chem. (1988) 263:7913–6. doi: 10.1016/S0021-9258(18)68417-0
32. McAllister TA, Cheng KJ, Okine EK, Mathison GW. Dietary, environmental and microbiological aspects of methane production in ruminants. Can J Anim Sci. (1996) 76:231–43. doi: 10.4141/cjas96-035
33. Deppenmeier U, Müller V. Life close to the thermodynamic limit: how methanogenic archaea conserve energy. In:Schäfer G, Penefsky HS, editors. Bioenergetics. Berlin: Springer (2007). p. 123–52. doi: 10.1007/400_2006_026
34. Evans PN, Boyd JA, Leu AO, Woodcroft BJ, Parks DH, Hugenholtz P, et al. An evolving view of methane metabolism in the archaea. Nat Rev Microbiol. (2019) 17:219–32. doi: 10.1038/s41579-018-0136-7
35. Thauer RK, Kaster AK, Seedorf H, Buckel W, Hedderich R. Methanogenic archaea: ecologically relevant differences in energy conservation. Nat Rev Microbiol. (2008) 6:579–91. doi: 10.1038/nrmicro1931
36. Donoghue KA, Bird-Gardiner T, Arthur PF, Herd RM, Hegarty RF. Genetic and phenotypic variance and covariance components for methane emission and postweaning traits in angus cattle. J Anim Sci. (2016) 94:1438–45. doi: 10.2527/jas.2015-0065
37. Herd RM, Arthur PF, Donoghue KA, Bird SH, Bird-Gardiner T, Hegarty RS. Measures of methane production and their phenotypic relationships with dry matter intake, growth, and body composition traits in beef cattle. J Anim Sci. (2014) 92:5267–74. doi: 10.2527/jas.2014-8273
38. Bird-Gardiner T, Arthur PF, Barchia IM, Donoghue KA, Herd RM. Phenotypic relationships among methane production traits assessed under ad libitum feeding of beef cattle. J Anim Sci. (2017) 95:4391–8. doi: 10.2527/jas2017.1477
39. Difford GF, Plichta DR, Løvendahl P, Lassen J, Noel SJ, Højberg O, et al. Host genetics and the rumen microbiome jointly associate with methane emissions in dairy cows. PLoS Genet. (2018) 14:e1007580. doi: 10.1371/journal.pgen.1007580
40. Manzanilla-Pech CIV, De Haas Y, Hayes BJ, Veerkamp RF, Khansefid M, Donoghue KA, et al. Genomewide association study of methane emissions in angus beef cattle with validation in dairy cattle. J Anim Sci. (2016) 94:4151–66. doi: 10.2527/jas.2016-0431
41. Richardson CM, Nguyen TTT, Abdelsayed M, Moate J, Williams SRO, Chud TCS, et al. Genetic parameters for methane emission traits in Australian dairy cows. J Anim Sci. (2021) 104:539–49. doi: 10.3168/jds.2020-18565
42. Rooke JA, Wallace RJ, Duthie CA, McKain N, de Souza SM, Hyslop JJ, et al. Hydrogen and methane emissions from beef cattle and their rumen microbial community vary with diet, time after feeding and genotype. Br J Nutr. (2014) 112:398–407. doi: 10.1017/S0007114514000932
43. De Mulder T, Peiren N, Vandaele L, Ruttink T, De Campeneere S, Van de Wiele T, et al. Impact of breed on the rumen microbial community composition and methane emission of holstein friesian and belgian blue heifers. Livestock Sci. (2018) 207:38–44. doi: 10.1016/j.livsci.2017.11.009
44. Herd RM, Velazco JI, Arthur PF, Hegarty RF. Associations among methane emission traits measured in the feedlot and in respiration chambers in angus cattle bred to vary in feed efficiency. J Anim Sci. (2016) 94:4882–91. doi: 10.2527/jas.2016-0613
45. Ramin M, Huhtanen P. Development of equations for predicting methane emissions from ruminants. J Anim Sci. (2013) 96:2476–93. doi: 10.3168/jds.2012-6095
46. Hristov AN, Oh J, Lee C, Meinen R, Montes F, Ott T, et al. Mitigation of Greenhouse Gas Emissions in Livestock Production – A Review of Technical Options for Non-CO2 Emissions. Gerber P, Henderson B, Makkar H, editors. FAO Animal Production and Health Paper No. 177. Rome: FAO (2013).
47. Wallace RJ, Sasson G, Garnsworthy PC, Tapio I, Gregson E, Bani P, et al. A heritable subset of the core rumen microbiome dictates dairy cow productivity and emissions. Sci Adv. (2019) 5:eaav8391. doi: 10.1126/sciadv.aav8391
48. Janssen PH. Influence of hydrogen on rumen methane formation and fermentation balances through microbial growth kinetics and fermentation thermodynamics. Anim Feed Sci Technol. (2010) 160:1–22. doi: 10.1016/j.anifeedsci.2010.07.002
49. Li F, Li C, Chen Y, Liu J, Zhang C, Irving B, et al. Host genetics influence the rumen microbiota and heritable rumen microbial features associate with feed efficiency in cattle. Microbiome. (2019) 7:92. doi: 10.1186/s40168-019-0699-1
50. Martínez-Álvaro M, Auffret MD, Duthie CA, Dewhurst RJ, Cleveland MA, Watson M, et al. Bovine host genome acts on rumen microbiome function linked to methane emissions. Commun Biol. (2022) 5:1–16. doi: 10.1038/s42003-022-03293-0
51. Goopy JP, Donaldson A, Hegarty R, Vercoe PE, Haynes F, Barnett M, et al. Low-methane yield sheep have smaller rumens and shorter rumen retention time. Br J Nutr. (2014) 111:578–85. doi: 10.1017/S0007114513002936
52. Danielsson R, Dicksved J, Sun L, Gonda H, Müller B, Schnürer A, et al. Methane production in dairy cows correlates with rumen methanogenic and bacterial community structure. Front Microbiol. (2017) 8:226. doi: 10.3389/fmicb.2017.00226
53. Ramayo-Caldas Y, Zingaretti L, Popova M, Estellé J, Bernard A, Pons N, et al. Identification of rumen microbial biomarkers linked to methane emission in Holstein dairy cows. J Anim Breed Genet. (2020) 137:49–59. doi: 10.1111/jbg.12427
54. Smith P, Kelly A, Kenny D, Waters S. Differences in the composition of the rumen microbiota of finishing beef cattle divergently ranked for residual methane emissions. Front Microbiol. (2022) 13:855565. doi: 10.3389/fmicb.2022.855565
55. Kittelmann S, Pinares-Patino CS, Seedorf H, Kirk MR, Ganesh S, McEwan JC, et al. Two different bacterial community types are linked with the low-methane emission trait in sheep. PLoS ONE. (2014) 9:e0103171. doi: 10.1371/journal.pone.0103171
56. Shi WB, Moon CD, Leahy SC, Kang DW, Froula J, Kittelmann S, et al. Methane yield phenotypes linked to differential gene expression in the sheep rumen microbiome. Genome Res. (2014) 24:1517–25. doi: 10.1101/gr.168245.113
57. Kelly WJ, Leahy SC, Kamke J, Soni P, Koike S, Mackie R, et al. Occurrence and expression of genes encoding methyl-compound production in rumen bacteria. Anim Microbiome. (2019) 1:15. doi: 10.1186/s42523-019-0016-0
58. Feldewert C, Lang K, Brune A. The hydrogen threshold of obligately methyl-reducing methanogens. FEMS Microbiol Lett. (2020) 367:fnaa137. doi: 10.1093/femsle/fnaa137
59. Leahy SC, Kelly WJ, Altermann E, Ronimus RS, Yeoman CJ, Pacheco DM, et al. The genome sequence of the rumen methanogen methanobrevibacter ruminantium reveals new possibilities for controlling ruminant methane emissions. PLoS ONE. (2010) 5:e0008926. doi: 10.1371/journal.pone.0008926
60. Reeve JN, Nölling J, Morgan RM, Smith DR. Methanogenesis: genes, genomes, and who's on first? J Bacterial. (1997) 179:5975. doi: 10.1128/jb.179.19.5975-5986.1997
61. Stewart CS, Flint HJ, Bryant MP. The rumen bacteria. In:Hobson PN, Stewart CS, editors. The Rumen Microbial Ecosystem, (Berlin: Springer), (1997) 10–72.
62. Marounek M, Dušková D. Metabolism of pectin in rumen bacteria butyrivibrio fibrisolvens and prevotella ruminicola. Lett Appl Microbiol. (1999) 29:429–33. doi: 10.1046/j.1472-765X.1999.00671.x
63. Kamke J, Kittelmann S, Soni P, Li Y, Tavendale M, Ganesh S, et al. Rumen metagenome and metatranscriptome analyses of low methane yield sheep reveals a sharpea-enriched microbiome characterised by lactic acid formation and utilisation. Microbiome. (2016) 4:56. doi: 10.1186/s40168-016-0201-2
64. Wallace RJ, Rooke JA, McKain N, Duthie CA, Hyslop JJ, Ross DW, et al. The rumen microbial metagenome associated with high methane production in cattle. BMC Genom. (2015) 16:839. doi: 10.1186/s12864-015-2032-0
65. Wolin MJ, Miller TL, Stewart CS. Microbe-microbe interactions. In:Hobson PN, Stewart CS, editors. The Rumen Microbial Ecosystem. Berlin: Springer (1997). p. 467–91.
66. Marty RJ, Demeyer DI. The effect of inhibitors of methane production of fermentation pattern and stoichiometry in vitro using rumen contents from sheep given molasses. Br J Nutr. (1973) 30:369–76. doi: 10.1079/BJN19730041
67. Hungate RE, Smith W, Bauchop T, Yu I, Rabinowitz JC. Formate as an intermediate in the bovine rumen fermentation. J Bacteriol. (1970) 102:389–97. doi: 10.1128/jb.102.2.389-397.1970
68. Greening C, Geier R, Wang C, Woods LC, Morales SE, McDonald MJ, et al. Diverse hydrogen production and consumption pathways influence methane production in ruminants. ISME. (2019) 13:2617–32. doi: 10.1038/s41396-019-0464-2
69. Morgavi DP, Martin C, Jouany JP, Ranilla MJ. Rumen protozoa and methanogenesis: not a simple cause–effect relationship. Br J Nutr. (2012) 107:388–97. doi: 10.1017/S0007114511002935
70. Guyader J, Eugène M, Noziere P, Morgavi DP, Doreau M, Martin C. Influence of rumen protozoa on methane emission in ruminants: a meta-analysis approach 1. Animal. (2014) 8:1816–25. doi: 10.1017/S1751731114001852
71. Newbold CJ, De La Fuente G, Belanche A, Ramos-Morales E, McEwan NR. The role of ciliate protozoa in the rumen. Front Microbiol. (2015) 6:1313. doi: 10.3389/fmicb.2015.01313
72. Ng F, Kittelmann S, Patchett ML, Attwood GT, Janssen PH, Rakonjac J, et al. An adhesin from hydrogen-utilizing rumen methanogen M ethanobrevibacter ruminantium M 1 binds a broad range of hydrogen-producing microorganisms. Environ Microbiol. (2016) 18:3010–21. doi: 10.1111/1462-2920.13155
73. Belanche A, de la Fuente G, Newbold CJ. Effect of progressive inoculation of fauna-free sheep with holotrich protozoa and total-fauna on rumen fermentation, microbial diversity and methane emissions. FEMS Microbiol Ecol. (2015) 91:fiu026. doi: 10.1093/femsec/fiu026
74. Cunha CS, Veloso CM, Marcondes MI, Mantovani HC, Tomich TR, Pereira LGR, et al. Assessing the impact of rumen microbial communities on methane emissions and production traits in holstein cows in a tropical climate. Syst Appl Microbiol. (2017) 40:492–9. doi: 10.1016/j.syapm.2017.07.008
75. Aydin S, Yildirim E, Ince O, Ince B. Rumen anaerobic fungi create new opportunities for enhanced methane production from microalgae biomass. Algal Res. (2017) 23:150–60. doi: 10.1016/j.algal.2016.12.016
76. Doreau M, Arbre M, Rochette Y, Lascoux C, Eugène M, Martin C. Comparison of 3 methods for estimating enteric methane and carbon dioxide emission in nonlactating cows. J Anim Sci. (2018) 96:1559–69. doi: 10.1093/jas/sky033
77. Hristov AN, Kebreab E, Niu M, Oh J, Bannink A, Bayat AR, et al. Symposium review: uncertainties in enteric methane inventories, measurement techniques, prediction models. J Dairy Sci. (2018) 101:6655–74. doi: 10.3168/jds.2017-13536
78. Zimmerman PR. System for Measuring Metabolic Gas Emissions From Animals. US Patent and Trademark Office, Assignee. US Patent No. 5:265,618 (1993).
79. Johnson K, Huyler M, Westberg H, Lamb B, Zimmerman P. Measurement of methane emissions from ruminant livestock using a sulfur hexafluoride tracer technique. Environ Sci Technol. (1994) 28:359–62. doi: 10.1021/es00051a025
80. Jonker A, Waghorn GC. Guidelines for Use of Sulphur Hexafloride (SF6) Tracer Technique to Measure Enteric Methane Emissions From Ruminants. New Zealand Agricultural Greenhouse Gas Research Centre, New Zealand. Available online at: https://globalresearchalliance.org/publicationlibrary/?doctype=223&research-group=212
81. Lassey KR, Hegarty RS. Chapter 12: SF6 Reporting in Guidelines for Use of Sulphur Hexafluoride (SF 6) Tracer Technique to Measure Enteric Methane Emissions From Ruminants. Lambert MG, editor. New Zealand Agricultural Greenhouse Gas Research Centre, New Zealand (2014).
82. Patra AK. Recent advances in measurement and dietary mitigation of enteric methane emissions in ruminants. Front Vet Sci. (2016) 3:39. doi: 10.3389/fvets.2016.00039
83. Storm IM, Hellwing ALF, Nielsen NI, Madsen J. Methods for measuring and estimating methane emission from ruminants. Animals. (2012) 2:160–83. doi: 10.3390/ani2020160
84. Deighton MH, Williams SRO, Hannah MC, Eckard RJ, Boland TM, Wales WJ, et al. A modified sulphur hexafluoride tracer technique enables accurate determination of enteric methane emissions from ruminants. Anim Feed Sci Technol. (2014) 197:47–63. doi: 10.1016/j.anifeedsci.2014.08.003
85. Williams SRO, Deighton MH, Moate PJ. Chapter 2: OVERVIEW of the SF6 guidelines for use of sulphur hexafluoride (SF6) tracer technique tracer technique and its evolution. In:Lambert MG, editors. Guidelines for Use of Sulphur Hexafluoride (SF 6) Tracer Technique to Measure Enteric Methane Emissions from Ruminants. New Zealand Agricultural Greenhouse Gas Research Centre, New Zealand (2014).
86. Williams CM, Eun JS, MacAdam JW, Young AJ, Fellner V, Min BR. Effects of forage legumes containing condensed tannins on methane and ammonia production in continuous cultures of mixed ruminal microorganisms. Anim. Feed Sci Technol. (2011) 166:364–72. doi: 10.1016/j.anifeedsci.2011.04.025
87. Zimmerman PR. Method and System for Monitoring and Reducing Ruminant Methane Production. US Patents 2009/0288606A1 (2009).
88. Zimmerman PR, Zimmerman RS. Method and System for Monitoring and Reducing Ruminant Methane Production. US Patents 2011/0192213 (2012).
89. Manafiazar G, Zimmerman S, Basarab JA. Repeatability and variability of short-term spot measurement of methane and carbon dioxide emissions from beef cattle using GreenFeed emissions monitoring system. Can J Anim Sci. (2016) 97:118–26. doi: 10.1139/CJAS-2015-0190
90. Hristov AN, Oh J, Giallongo F, Frederick T, Weeks H, Zimmerman PR, et al. The use of an automated system (GreenFeed) to monitor enteric methane carbon dioxide emissions from ruminant animals. JoVE. (2015) 7:52904. doi: 10.3791/52904
91. Huhtanen PE, Cabezas-Garcia H, Utsumi S, Zimmerman S. Comparison of methods to determine methane emissions from dairy cows in farm conditions. J Dairy Sci. (2015) 98:3394–409. doi: 10.3168/jds.2014-9118
92. Morrison SJ, McBride J, Gordon AW, Wylie AR, Yan T. Methane emissions from grazing holstein-friesian heifers at different ages estimated using the sulfur hexafluoride tracer technique. Engineering. (2017) 3:753–9. doi: 10.1016/J.ENG.2017.03.018
93. Gunter SA, Beck MR. Measuring the respiratory gas exchange by grazing cattle using an automated, open-circuit gas quantification system. Translat Anim Sci. (2018) 2:11–8. doi: 10.1093/tas/txx009
94. Jonker A, Green P, Waghorn G, van der Weerden T, Pacheco D, de Klein C. A meta-analysis comparing four measurement methods to determine the relationship between methane emissions and dry-matter intake in New Zealand dairy cattle. Anim Prod Sci. (2018) 60:96–101. doi: 10.1071/AN18573
95. Goopy JP, Chang C, Tomkins N. A comparison of methodologies for measuring methane emissions from ruminants. In: Methods for Measuring Greenhouse Gas Balances and Evaluating Mitigation Options in Smallholder Agriculture. Cham: Springer (2016). p. 97–117. doi: 10.1007/978-3-319-29794-1_5
96. Arbre M, Rochette Y, Guyader J, Lascoux C, Gómez LM, Eugène M, et al. Repeatability of enteric methane determinations from cattle using either the SF6 tracer technique or the GreenFeed system. Anim Prod Sci. (2016) 56:238–43. doi: 10.1071/AN15512
97. Renand G, Maupetit D. Assessing individual differences in enteric methane emission among beef heifers using the GreenFeed emission monitoring system: effect of the length of testing period on precision. Anim Prod Sci. (2016) 56:218–23. doi: 10.1071/AN15429
98. Arthur PF, Barchia IM, Weber C, Bird-Gardiner T, Donoghue KA, Herd RM, et al. Optimizing test procedures for estimating daily methane and carbon dioxide emissions in cattle using short-term breath measures. J Anim Sci. (2017) 95:645–56. doi: 10.2527/jas2016.0700
99. Arthur PF, Bird-Gardiner T, Barchia IM, Donoghue KA, Herd RM. Relationships among carbon dioxide, feed intake, and feed efficiency traits in ad libitum fed beef cattle. J Anim Sci. (2018) 96:4859–67. doi: 10.1093/jas/sky308
100. Garnsworthy PC, Difford GF, Bell MJ, Bayat AR, Huhtanen P, Kuhla B, et al. Comparison of methods to measure methane for use in genetic evaluation of dairy cattle. Animals. (2019) 9:837. doi: 10.3390/ani9100837
101. Alemu AW, Vyas D, Manafiazar G, Basarab JA, Beauchemin KA. Enteric methane emissions from low–and high–residual feed intake beef heifers measured using GreenFeed and respiration chamber techniques. J Anim Sci. (2017) 95:3727–37. doi: 10.2527/jas2017.1501
102. Velazco JI, Herd RM, Cottle DJ, Hegarty RS. Daily methane emissions and emission intensity of grazing beef cattle genetically divergent for residual feed intake. Anim Prod Sci. (2017) 57:627–35. doi: 10.1071/AN15111
103. Lanigan G, Donnellan T, Hanrahan K, Carsten P, Shalloo L, Krol D, et al. An Analysis of Abatement Potential of Greenhouse Gas Emissions in Irish Agriculture 2021–2030. Carlow: Teagasc (2019).
104. Taylor RF, McGee M, Kelly AK, Crosson P. Bioeconomic and greenhouse gas emissions modelling of the factors influencing technical efficiency of temperate grassland-based suckler calf-to-beef production systems. Agric Syst. (2020) 183:102860. doi: 10.1016/j.agsy.2020.102860
105. O'Donovan M, Lewis E, O'Kiely P. Requirements of future grass-based ruminant production systems in Ireland. Irish J Agric Food Res. (2011) 50:1–21.
106. Buxton DR, Redfearn DD. Plant limitations to fiber digestion and utilization. J Nutr. (1997) 127:814S−8S. doi: 10.1093/jn/127.5.814S
107. Ellis WC. Determinants of grazed forage intake and digestibility. J Dairy Sci. (1978) 61:1828–40. doi: 10.3168/jds.S0022-0302(78)83809-0
108. Williams SRO, Hannah M, Jacobs JL, Wales WJ, Moate PJ. Volatile fatty acids in ruminal fluid can be used to predict methane yield of dairy cows. Animals. (2019) 9:1006. doi: 10.3390/ani9121006
109. Holmes CW, Hoogendoorn CJ, Ryan MP, Chu ACP. Some effects of herbage composition, as influenced by previous grazing management, on milk production by cows grazing on ryegrass/white clover pastures. 1. Milk production in early spring: effects of different regrowth intervals during the preceding winter period. Grass Sci. (1992) 47:309–15. doi: 10.1111/j.1365-2494.1992.tb02276.x
110. Wims CM, Deighton MH, Lewis E, O'Loughlin B, Delaby L, Boland TM, et al. Effect of pregrazing herbage mass on methane production, dry matter intake, and milk production of grazing dairy cows during the mid-season period. J Anim Sci. (2010) 93:4976–85. doi: 10.3168/jds.2010-3245
111. Boland TM, Quinlan C, Pierce KM, Lynch MB, Kenny DA, Kelly AK, et al. The effect of pasture pregrazing herbage mass on methane emissions, ruminal fermentation, and average daily gain of grazing beef heifers. J Anim Sci. (2013) 91:3867–74. doi: 10.2527/jas.2013-5900
112. Doyle PR, McGee M, Moloney AP, Kelly AK, O'Riordan EG. Effect of pre-grazing herbage mass on pasture production and performance of suckler-bred steers during the grazing season and subsequent indoor finishing period. Livest Sci. (2022) 256:104814. doi: 10.1016/j.livsci.2021.104814
113. Fraser MD, Fleming HR, Moorby JM. Traditional vs modern: role of breed type in determining enteric methane emissions from cattle grazing as part of contrasting grassland-based systems. PLoS ONE. (2014) 9:107861. doi: 10.1371/journal.pone.0107861
114. Richmond AS, Wylie ARG, Laidlaw AS, Lively FO. Methane emissions from beef cattle grazing on semi-natural upland and improved lowland grasslands. Animal. (2015) 9:130–7. doi: 10.1017/S1751731114002067
115. McCarthy B, Pierce KM, Delaby L, Brennan A, Fleming C, Horan B. The effect of stocking rate and calving date on grass production, utilization and nutritive value of the sward during the grazing season. Grass Forage Sci. (2013) 68:364–77. doi: 10.1111/j.1365-2494.2012.00904.x
116. Pinares-Patiño CS, D'Hour P, Jouany JP, Martin C. Effects of stocking rate on methane and carbon dioxide emissions from grazing cattle. Agric Ecosyst Environ. (2007) 121:30–46. doi: 10.1016/j.agee.2006.03.024
117. Chiavegato MB, Rowntree JE, Carmichael D, Powers WJ. Enteric methane from lactating beef cows managed with high-and low-input grazing systems. J Anim Sci. (2015) 93:1365–75. doi: 10.2527/jas.2014-8128
118. De Ramus HA, Clement TC, Giampola DD, Dickison PC. Methane emissions of beef cattle on forages: efficiency of grazing management systems. J Environ Qual. (2003) 32:269–77. doi: 10.2134/jeq2003.2690
119. Savian JV, Neto AB, de David DB, Bremm C, Schons RMT, Genro TCM, et al. Grazing intensity and stocking methods on animal production and methane emission by grazing sheep: implications for integrated crop–livestock system. Agric Ecosyst Environ. (2014) 190:112–9. doi: 10.1016/j.agee.2014.02.008
120. Edwards G, Parsons A, Rasmussen S, Bryant RH. High Sugar Ryegrasses for Livestock Systems in New Zealand. New Zealand Grassland Association (2007). doi: 10.33584/jnzg.2007.69.2674
121. Lee MR, Jones EL, Moorby JM, Humphreys MO, Theodorou MK, Scollan ND. Production responses from lambs grazed on Lolium perenne selected for an elevated water-soluble carbohydrate concentration. Anim Res. (2001) 50:441–9. doi: 10.1051/animres:2001106
122. Lee MRF, Harris LJ, Moorby JM, humphreys MO, Theodorou MK, MacRae JC, et al. Rumen metabolism and nitrogen flow to the small intestine in steers offered Lolium perenne containing different levels of water-soluble carbohydrate. Anim Sci. (2002) 74:587–96. doi: 10.1017/S1357729800052747
123. Purcell PJ, Boland TM, O'Kiely P. The effect of water-soluble carbohydrate concentration and type on in vitro rumen methane output of perennial ryegrass determined using a 24-hour batch-culture gas production technique. Irish J Agric Food Res. (2014) 53:21–36.
124. Sun X, Cheng L, Jonker A, Munidasa S, Pacheco D. A review: plant carbohydrate types—the potential impact on ruminant methane emissions. Front Vet Sci. (2022) 9:880115. doi: 10.3389/fvets.2022.880115
125. Kim EJ, Newbold CJ, Scollan ND. Effect of water-soluble carbohydrate in fresh forage on growth and methane production by growing lambs. Adv Anim Biosci. (2011) 2:270.
126. Staerfl SM, Amelchanka SL, Kälber T, Soliva CR, Kreuzer M, Zeitz JO. Effect of feeding dried high-sugar ryegrass (‘AberMagic') on methane and urinary nitrogen emissions of primiparous cows. Livest Sci. (2012) 150:293–301. doi: 10.1016/j.livsci.2012.09.019
127. Jonker A, Molano G, Sandoval E, Taylor PS, Antwi C, Olinga S, et al. Methane emissions differ between sheep offered a conventional diploid, a high-sugar diploid or a tetraploid perennial ryegrass cultivar at two allowances at three times of the year. Anim Prod Sci. (2016) 58:1043–8. doi: 10.1071/AN15597
128. Hegarty RS, Passetti RA, Dittmer KM, Wang Y, Shelton S, Emmet-Booth J, et al. An Evaluation of Emerging Feed Additives to Reduce Methane Emissions From Livestock (2021).
129. Eckard RJ, Clark H. Potential solutions to the major greenhouse-gas issues facing Australasian dairy farming. Anim Prod Sci. (2018) 60:10–6. doi: 10.1071/AN18574
130. Winichayakul S, Beechey-Gradwell Z, Muetzel S, Molano G, Crowther T, Lewis S, et al. In vitro gas production and rumen fermentation profile of fresh and ensiled genetically modified high–metabolizable energy ryegrass. J Dairy Sci. (2020) 103:2405–18. doi: 10.3168/jds.2019-16781
131. Beechey-Gradwell Z, Kadam S, Bryan G, Cooney L, Nelson K, Richardson K, et al. Lolium perenne engineered for elevated leaf lipids exhibits greater energy density in field canopies under defoliation. Field Crops Res. (2022) 275:108340. doi: 10.1016/j.fcr.2021.108340
132. Enriquez-Hidalgo D, Gilliland TJ, Egan M, Hennessy D. Production and quality benefits of white clover inclusion into ryegrass swards at different nitrogen fertilizer rates. J Agri Sci. (2018) 156:378–86. doi: 10.1017/S0021859618000370
133. Guy C, Hennessy D, Gilliland TJ, Coughlan F, McClearn B, Dineen M, et al. Comparison of perennial ryegrass, Lolium perenne L., ploidy and white clover, Trifolium repens L., inclusion for herbage production, utilization and nutritive value. Grass For Sci. (2018) 73:865–77. doi: 10.1111/gfs.12366
134. Guy C, Hennessy D, Gilliland TJ, Coughlan F, McClearn B, Dineen M, et al. White clover incorporation at high nitrogen application levels: results from a 3-year study. Anim Prod Sci. (2020) 60:187–91. doi: 10.1071/AN18540
135. Purcell PJ, Grant J, Boland TM, Grogan D, O'Kiely P. The in vitro rumen methane output of perennial grass species and white clover varieties, and associative effects for their binary mixtures, evaluated using a batch-culture technique. Anim Prod Sci. (2012) 52:1077–88. doi: 10.1071/AN12040
136. Belanche A, Lee MRF, Moorby JM, Newbold CJ. Comparison of ryegrass and red clover on the fermentation pattern, microbial community and efficiency of diet utilisation in the rumen simulation technique (Rusitec). Anim Prod Sci. (2013) 53:1052–64. doi: 10.1071/AN12183
137. Smith PE, Enriquez-Hidalgo D, Hennessy D, McCabe MS, Kenny DA, Kelly AK, et al. Sward type alters the relative abundance of members of the rumen microbial ecosystem in dairy cows. Sci Rep. (2020) 10:9317. doi: 10.1038/s41598-020-66028-3
138. Lee JM, Woodward SL, Waghorn GC, Clark DA. Methane emissions by dairy cows fed increasing proportions of white clover (Trifolium repens) in pasture. Proc N Zeal Grassl Assoc. (2004) 66:151–5. doi: 10.33584/jnzg.2004.66.2552
139. Niderkorn V, Martin C, Rochette Y, Julien S, Baumont R. Associative effects between orchardgrass and red clover silages on voluntary intake and digestion in sheep: evidence of a synergy on digestible dry matter intake. J Anim Sci. (2015) 93:4967–76. doi: 10.2527/jas.2015-9178
140. Niderkorn V, Martin C, Le Morvan A, Rochette Y, Awad M, Baumont R. Associative effects between fresh perennial ryegrass and white clover on dynamics of intake and digestion in sheep. Grass Forage Sci. (2017) 72:691–9. doi: 10.1111/gfs.12270
141. Enriquez-Hidalgo D, Gilliland T, Deighton MH, O'Donovan M, Hennessy D. Milk production and enteric methane emissions by dairy cows grazing fertilized perennial ryegrass pasture with or without inclusion of white clover. J Anim Sci. (2014) 97:1400–12. doi: 10.3168/jds.2013-7034
142. Dewhurst RJ, Fisher WJ, Tweed JK, Wilkins RJ. Comparison of grass and legume silages for milk production. 1. Production responses with different levels of concentrate. J Anim Sci. (2003) 86:2598–611. doi: 10.3168/jds.S0022-0302(03)73855-7
143. Smith PE, Waters SM, Kenny DA, Kirwan SF, Conroy S, Kelly AK. Effect of divergence in residual methane emissions on feed intake and efficiency, growth and carcass performance, and indices of rumen fermentation and methane emissions in finishing beef cattle. J Animl Sci. (2021) 99:skab275. doi: 10.1093/jas/skab275
144. Egan M, Lynch MB, Hennessy D. Including white clover in nitrogen fertilized perennial ryegrass swards: effects on dry matter intake and milk production of spring calving dairy cows. J Agri Sci. (2017) 155:657–68. doi: 10.1017/S0021859616000952
145. McClearn B, Gilliland T, Guy C, Dineen M, Coughlan F, McCarthy B. The effect of perennial ryegrass ploidy and white clover inclusion on milk production of dairy cows. Anim Prod Sci. (2020) 60:143–7. doi: 10.1071/AN18539
146. McClearn B, Delaby L, Gilliland TJ, Guy C, Dineen M, Coughlan F, et al. The effect of Lolium perenne ploidy L. and Trifolium repens L. inclusion on dry matter intake and production efficiencies of spring-calving grazing dairy cows. J Anim Sci. (2021) 104:6688–700. doi: 10.3168/jds.2020-19753
147. Grace C, Boland TM, Sheridan H, Lott S, Brennan E, Fritch R, et al. The effect of increasing pasture species on herbage production, chemical composition and utilization under intensive sheep grazing. Grass Forage Sci. (2018) 73:852–64. doi: 10.1111/gfs.12379
148. Moloney AP, O'Riordan EG, Schmidt O, Monahan FJ. The fatty acid profile and stable isotope ratios of C and N of muscle from cattle that grazed grass or grass/clover pastures before slaughter and their discriminatory potential. Irish J Agric Food Res. (2018) 57:84–94. doi: 10.1515/ijafr-2018-0009
149. McSweeney CS, Palmer B, McNeill DM, Krause DO. Microbial interactions with tannins: nutritional consequences for ruminants. Anim Feed Sci Technol. (2001) 91:83–93. doi: 10.1016/S0377-8401(01)00232-2
150. Makkar HPS. Effects and fate of tannins in ruminant animals, adaptation to tannins, and strategies to overcome detrimental effects of feeding tannin-rich feeds. Small Rumin. (2003) 49:241–56. doi: 10.1016/S0921-4488(03)00142-1
151. Patra AK, Saxena J. Exploitation of dietary tannins to improve rumen metabolism and ruminant nutrition. J Sci Food Agric. (2011) 91:24–37. doi: 10.1002/jsfa.4152
152. Jayanegara A, Goel G, Makkar HP, Becker K. Divergence between purified hydrolysable and condensed tannin effects on methane emission, rumen fermentation and microbial population in vitro. Anim Feed Sci Technol. (2015) 209:60–8. doi: 10.1016/j.anifeedsci.2015.08.002
153. Wang Y, Majak W, McAllister TA. Frothy bloat in ruminants: cause, occurrence, and mitigation strategies. Anim Feed Sci Technol. (2012) 172:103–14. doi: 10.1016/j.anifeedsci.2011.12.012
154. Roldan MB, Cousins G, Muetzel S, Zeller WE, Fraser K, Salminen JP, et al. Condensed tannins in white clover (Trifolium repens) foliar tissues expressing the transcription factor TaMYB14-1 bind to forage protein and reduce methane emissions in vitro. Front Plant Sci. (2021) 12:777354. doi: 10.3389/fpls.2021.777354
155. Wilson RL, Bionaz M, MacAdam JW, Beauchemin KA, Naumann HD, Ates S. Milk production, nitrogen utilization, and methane emissions of dairy cows grazing grass, forb, legume-based pastures. J Anim Sci. (2020) 98:skaa220. doi: 10.1093/jas/skaa220
156. Grace C, Lynch MB, Sheridan H, Lott S, Fritch R, Boland TM. Grazing multispecies swards improves ewe and lamb performance. Animal. (2019) 13:1721–9. doi: 10.1017/S1751731118003245
157. Roca-Fernández AI, Peyraud JL, Delaby L, Delagarde R. Pasture intake and milk production of dairy cows rotationally grazing on multi-species swards. Animal. (2016) 10:1448–56. doi: 10.1017/S1751731116000331
158. Hutton PG, Kenyon PR, Bedi MK, Kemp PD, Stafford KJ, West DM, et al. A herb and legume sward mix increased ewe milk production and ewe and lamb live weight gain to weaning compared to a ryegrass dominant sward. Anim Feed Sci Technol. (2011) 164:1–7. doi: 10.1016/j.anifeedsci.2010.11.014
159. Cummins S, Finn JA, Richards KG, Lanigan GJ, Grange G, Brophy C, et al. Beneficial effects of multi-species mixtures on N2O emissions from intensively managed grassland swards. Sci Total Environ. (2021) 792:148163. doi: 10.1016/j.scitotenv.2021.148163
160. Waghorn GC, Tavendale MH, Woodfield DR. January. Methanogenesis from forages fed to sheep. In: Proceedings of the New Zealand Grassland Association. (2002). p. 167–71. doi: 10.33584/jnzg.2002.64.2462
161. Sun XZ, Hoskin SO, Muetzel S, Molano G, Clark H. Effects of forage chicory (Cichorium intybus) and perennial ryegrass (Lolium perenne) on methane emissions in vitro and from sheep. Anim Feed Sci Technol. (2011) 166:391–7. doi: 10.1016/j.anifeedsci.2011.04.027
162. Jonker A, Farrell L, Scobie D, Dynes R, Edwards G, Hague H, et al. Methane and carbon dioxide emissions from lactating dairy cows grazing mature ryegrass/white clover or a diverse pasture comprising ryegrass, legumes and herbs. Anim Prod Sci. (2018) 59:1063–9. doi: 10.1071/AN18019
163. Williams SRO, Moate PJ, Deighton MH, Hannah MC, Wales WJ, Jacobs JL. Milk production and composition, and methane emissions from dairy cows fed lucerne hay with forage brassica or chicory. Anim Prod Sci. (2016) 56:304–11. doi: 10.1071/AN15528
164. McCarthy KM, McAloon CG, Lynch MB, Pierce KM, Mulligan FJ. Herb species inclusion in grazing swards for dairy cows—a systematic review and meta-analysis. J Dairy Sci. (2020) 103:1416–30. doi: 10.3168/jds.2019-17078
165. Chaves AV, Thompson LC, Iwaasa AD, Scott SL, Olson ME, Benchaar C, et al. Effect of pasture type (alfalfa vs. grass) on methane and carbon dioxide production by yearling beef heifers. Can J Anim Sci. (2006) 86:409–18. doi: 10.4141/A05-081
166. Puchala R, Animut G, Patra AK, Detweiler GD, Wells JE, Varel VH, et al. Effects of different fresh-cut forages and their hays on feed intake, digestibility, heat production, and ruminal methane emission by Boer× Spanish goats. J Anim Sci. (2012) 90:2754–62. doi: 10.2527/jas.2011-4879
167. Popp JD, McCaughey WP, Cohen RDH, McAllister TA, Majak W. Enhancing pasture productivity with alfalfa: a review. Can J Plant Sci. (2000) 80:513–9. doi: 10.4141/P99-049
168. Williams SRO, Moate PJ, Hannah MC, Ribaux BE, Wales WJ, Eckard RJ. Background matters with the SF6 tracer method for estimating enteric methane emissions from dairy cows: a critical evaluation of the SF6 procedure. Anim Feed Sci Technol. (2011) 170:265–76. doi: 10.1016/j.anifeedsci.2011.08.013
169. Woodward SL, Waghorn GC, Ulyatt MJ, Lassey KR. Early indications that feeding Lotus will reduce methane emissions from ruminants. In: Proceedings-New Zealand Society of Animal Production. Vol. 61. New Zealand Society of Animal Production (2001). p. 23–6.
170. Christensen RG, Eun JS, Yang SY, Min BR, MacAdam JW. In vitro effects of birdsfoot trefoil (Lotus corniculatus L.) pasture on ruminal fermentation, microbial population, methane production. Anim Sci. (2017) 33:451–60. doi: 10.15232/pas.2016-01558
171. Barry TN. The feeding value of forage brassica plants for grazing ruminant livestock. Anim Feed Sci Technol. (2013) 181:15–25. doi: 10.1016/j.anifeedsci.2013.01.012
172. Sun XZ, Waghorn GC, Hoskin SO, Harrison SJ, Muetzel S, Pacheco D. Methane emissions from sheep fed fresh brassicas (Brassica spp.) compared to perennial ryegrass (Lolium perenne). Anim Feed Sci Technol. (2012) 176:107–16. doi: 10.1016/j.anifeedsci.2012.07.013
173. Sun XZ, Harland R, Pacheco D. Effect of altering ruminal pH by dietary buffer supplementation on methane emissions from sheep fed forage rape. Animal. (2020) 14:952–62. doi: 10.1017/S1751731119002799
174. Sun X, Pacheco D, Luo D. Forage brassica: a feed to mitigate enteric methane emissions? Anim Prod Sci. (2016) 56:451–6. doi: 10.1071/AN15516
175. Sun XZ, Sandoval E, Pacheco D. Brief communication: substitution of perennial ryegrass with forage rape reduces methane emissions from sheep. Proc N Zeal Soc Anim Prod. (2015) 75:64–6.
176. Sun X, Henderson G, Cox F, Molano G, Harrison SJ, Luo D, et al. Lambs fed fresh winter forage rape (Brassica napus L.) emit less methane than those fed perennial ryegrass (Lolium perenne L.), and possible mechanisms behind the difference. PLoS ONE. (2015) 10:e0119697. doi: 10.1371/journal.pone.0119697
177. Sun X. Invited review: glucosinolates might result in low methane emissions from ruminants fed brassica forages. Front Vet Sci. (2020) 7:588051. doi: 10.3389/fvets.2020.588051
178. Zhou Z, Yu Z, Meng Q. Effects of nitrate on methane production, fermentation, and microbial populations in in vitro ruminal cultures. Bioresourc Technol. (2012) 103:173–9. doi: 10.1016/j.biortech.2011.10.013
179. Liu L, Xu X, Cao Y, Cai C, Cui H, Yao J. Nitrate decreases methane production also by increasing methane oxidation through stimulating NC10 population in ruminal culture. Amb Express. (2017) 7:1–7. doi: 10.1186/s13568-017-0377-2
180. McGee M. Recent developments in feeding beef cattle on grass silage-based diets. In: Silage Production and Utilisation: Proceedings of the XIVth International Silage Conference, a Satellite Workshop of the XXth International Grassland Congress, July 2005. Belfast: Wageningen Academic Pub (2005).
181. Blaxter KL, Clapperton JL. Prediction of the amount of methane produced by ruminants. Br J Nutr. (1965) 19:511–22. doi: 10.1079/BJN19650046
182. Duthie CA, Haskell M, Hyslop JJ, Waterhouse A, Wallace RJ, Roehe R, et al. The impact of divergent breed types and diets on methane emissions, rumen characteristics and performance of finishing beef cattle. Animal. (2017) 11:1762–71. doi: 10.1017/S1751731117000301
183. McDonnell RP, Hart KJ, Boland TM, Kelly AK, McGee M, Kenny DA. Effect of divergence in phenotypic residual feed intake on methane emissions, ruminal fermentation, and apparent whole-tract digestibility of beef heifers across three contrasting diets. J Anim Sci. (2016) 94:1179–93. doi: 10.2527/jas.2015-0080
184. Lovett DK, Stack LJ, Lovell S, Callan J, Flynn B, Hawkins M, et al. Manipulating enteric methane emissions and animal performance of late-lactation dairy cows through concentrate supplementation at pasture. J Anim Sci. (2005) 88:2836–42. doi: 10.3168/jds.S0022-0302(05)72964-7
185. Muñoz C, Hube S, Morales JM, Yan T, Ungerfeld EM. Effects of concentrate supplementation on enteric methane emissions and milk production of grazing dairy cows. Livest Sci. (2015) 175:37–46. doi: 10.1016/j.livsci.2015.02.001
186. Aguerre MJ, Wattiaux MA, Powell JM, Broderick GA, Arndt C. Effect of forage-to-concentrate ratio in dairy cow diets on emission of methane, carbon dioxide, and ammonia, lactation performance, manure excretion. J Anim Sci. (2011) 94:3081–93. doi: 10.3168/jds.2010-4011
187. Sauvant D, Giger-Reverdin S. Modélisation des interactions digestives et de la production de méthane chez les ruminants. INRA Product Anim. (2009) 22:375–84. doi: 10.20870/productions-animales.2009.22.5.3362
188. McGee M, Lenehan C, Crosson P, O'Riordan EG, Kelly AK, Moran L, et al. Performance, meat quality, profitability, and greenhouse gas emissions of suckler bulls from pasture-based compared to an indoor high-concentrate weanling-to-beef finishing system. Agric Syst. (2022) 198:103379. doi: 10.1016/j.agsy.2022.103379
189. Herron J, Curran TP, Moloney AP, McGee M, O'Riordan EG, O'Brien D. Life cycle assessment of pasture-based suckler steer weanling-to-beef production systems: effect of breed and slaughter age. Animal. (2021) 15:100247. doi: 10.1016/j.animal.2021.100247
190. McGinn SM, Beauchemin KA, Coates T, Colombatto D. Methane emissions from beef cattle: effects of monensin, sunflower oil, enzymes, yeast, fumaric acid. J Anim Sci. (2004) 82:3346–56. doi: 10.2527/2004.82113346x
191. Beauchemin KA, McGinn SM, Petit HV. Methane abatement strategies for cattle: lipid supplementation of diets. Can J Anim Sci. (2007) 87:431–40. doi: 10.4141/CJAS07011
192. Martin C, Rouel J, Jouany JP, Doreau M, Chilliard Y. Methane output and diet digestibility in response to feeding dairy cows crude linseed, extruded linseed, or linseed oil. J Anim Sci. (2008) 86:2642–50. doi: 10.2527/jas.2007-0774
193. Wang S, Giller K, Kreuzer M, Ulbrich SE, Braun U, Schwarm A. Contribution of ruminal fungi, archaea, protozoa, and bacteria to the methane suppression caused by oilseed supplemented diets. Front Microbiol. (2017) 8:1864. doi: 10.3389/fmicb.2017.01864
194. Bayat AR, Tapio I, Vilkki J, Shingfield KJ, Leskinen H. Plant oil supplements reduce methane emissions and improve milk fatty acid composition in dairy cows fed grass silage-based diets without affecting milk yield. J Anim Sci. (2018) 101:1136–51. doi: 10.3168/jds.2017-13545
195. Patra AK. The effect of dietary fats on methane emissions, and its other effects on digestibility, rumen fermentation and lactation performance in cattle: a meta-analysis. Livest Sci. (2013) 155:244–54. doi: 10.1016/j.livsci.2013.05.023
196. Beauchemin KA, Kreuzer M, O'Mara F, McAllister TA. Nutritional management for enteric methane abatement: a review. Aust J Exp Agric. (2008) 48:21–7. doi: 10.1071/EA07199
198. Nagaraja TG, Newbold CJ, Van Nevel CJ, Demeyer DI. Manipulation of ruminal fermentation. In:Hobson PN, Stewart CS, editors. The Rumen Microbial Ecosystem. Springer (1997). p. 523–632. doi: 10.1007/978-94-009-1453-7_13
199. Smith PE, Waters SM, Kenny DA, Boland TM, Heffernan J, Kelly AK. Replacing barley and soybean meal with by-products, in a pasture based diet, alters daily methane output and the rumen microbial community in vitro using the rumen simulation technique (RUSITEC). Front Microbiol. (2020) 11:1614. doi: 10.3389/fmicb.2020.01614
200. Mirzaei-Aghsaghali A, Maheri-Sis N. Nutritive value of some agro-industrial by-products for ruminants-A review. World J Zool. (2008) 3:40–6.
201. Kim SH, Mamuad LL, Jeong CD, Choi YJ, Lee SS, Ko JY, et al. In vitro evaluation of different feeds for their potential to generate methane and change methanogen diversity. Asian Austral J Anim. (2013) 26:1698–707. doi: 10.5713/ajas.2013.13260
202. O'Mara F. A Net Energy System for Cattle and Sheep. University College Dublin; Department of Animal Science and Production (1996).
203. McGinn SM, Chung YH, Beauchemin KA, Iwaasa AD, Grainger C. Use of corn distillers' dried grains to reduce enteric methane loss from beef cattle. Can J Anim Sci. (2009) 89:409–13. doi: 10.4141/CJAS08133
204. Chrenková M, Cerešnáková Z, Formelová Z, Poláčiková M, Mlyneková Z, Flak P. Chemical and nutritional characteristics of different types of DDGS for ruminants. J Anim Feed Sci. (2012) 21:35. doi: 10.22358/jafs/66108/2012
205. Berger L, Singh V. Changes and evolution of corn coproducts for beef cattle. J Anim Sci. (2010) 88:E143–50. doi: 10.2527/jas.2009-2526
206. Castillo-Lopez E, Jenkins CJR, Aluthge ND, Tom W, Kononoff PJ, Fernando SC. The effect of regular or reduced-fat distillers grains with solubles on rumen methanogenesis and the rumen bacterial community. J Appl Microbiol. (2017) 123:1381–95. doi: 10.1111/jam.13583
207. Foth AJ, Brown-Brandl T, Hanford KJ, Miller PS, Gomez GG, Kononoff PJ. Energy content of reduced-fat dried distillers grains with solubles for lactating dairy cows. J Dairy Sci. (2015) 98:7142–52. doi: 10.3168/jds.2014-9226
208. Bayat AR, Vilkki J, Razzaghi A, Leskinen H, Kettunen H, Khurana R, et al. Evaluating the effects of high-oil rapeseed cake or natural additives on methane emissions and performance of dairy cows. J Dairy Sci. (2022) 105:1211–24. doi: 10.3168/jds.2021-20537
209. Benchaar C, Hassanat F, Gervais R, Chouinard PY, Julien C, Petit HV, et al. Effects of increasing amounts of corn dried distillers grains with solubles in dairy cow diets on methane production, ruminal fermentation, digestion, N balance, milk production. J Dairy Sci. (2013) 96:2413–27. doi: 10.3168/jds.2012-6037
210. Hünerberg M, McGinn SM, Beauchemin KA, Okine EK, Harstad OM, McAllister TA. Effect of dried distillers' grains with solubles on enteric methane emissions and nitrogen excretion from finishing beef cattle. Can J Anim Sci. (2013) 93:373–85. doi: 10.4141/cjas2012-151
211. Hünerberg M, Little SM, Beauchemin KA, McGinn SM, O'Connor D, Okine EK, et al. Feeding high concentrations of corn dried distillers' grains decreases methane, but increases nitrous oxide emissions from beef cattle production. Agric Syst. (2014) 127:19–27. doi: 10.1016/j.agsy.2014.01.005
212. Whelan SJ, Carey W, Boland TM, Lynch MB, Kelly AK, Rajauria G, et al. The effect of by-product inclusion level on milk production, nutrient digestibility and excretion, and rumen fermentation parameters in lactating dairy cows offered a pasture-based diet. J Anim Sci. (2017) 100:1055–62. doi: 10.3168/jds.2016-11600
213. Condren SA, Kelly AK, Lynch MB, Boland TM, Whelan SJ, Grace C, et al. The effect of by-product inclusion and concentrate feeding rate on milk production and composition, pasture dry matter intake, and nitrogen excretion of mid-late lactation spring-calving cows grazing a perennial ryegrass-based pasture. J Dairy Sci. (2019) 102:1247–56. doi: 10.3168/jds.2018-14970
214. Hristov AN, Oh J, Giallongo F, Frederick TW, Harper MT, Weeks HL, et al. An inhibitor persistently decreased enteric methane emission from dairy cows with no negative effect on milk production. Proc Nat Acad Sci USA. 112:10663–8. doi: 10.1073/pnas.1504124112
215. Romero-Perez A, Okine EK, McGinn SM, Guan LL, Oba M, Duval SM, et al. Sustained reduction in methane production from long-term addition of 3-nitrooxypropanol to a beef cattle diet. J Anim Sci. (2015) 93:1780–91. doi: 10.2527/jas.2014-8726
216. Martinez Fernandez G, Duval SM, Kindermann M, Schirra HJ, Denman SE, McSweeney CS. 3-NOP vs Halogenated compound: Methane production, ruminal fermentation and microbial community response in forage fed cattle. Front Microbiol. (2018) 9:1582. doi: 10.3389/fmicb.2018.01582
217. Vyas D, Alemu AW, McGinn SM, Duval SM, Kindermann M, Beauchemin KA. The combined effects of supplementing monensin and 3-nitrooxypropanol on methane emissions, growth rate, and feed conversion efficiency in beef cattle fed high-forage and high-grain diets. J Anim Sci. (2018) 96:2923–38. doi: 10.1093/jas/sky174
218. Kim SH, Lee C, Pechtl HA, Hettick JM, Campler MR, Pairis-Garcia MD, et al. Effects of 3-nitrooxypropanol on enteric methane production, rumen fermentation, and feeding behavior in beef cattle fed a high-forage or high-grain diet. J Anim Sci. (2019) 97:2687–99. doi: 10.1093/jas/skz140
219. Van Wesemael D, Vandaele L, Ampe B, Cattrysse H, Duval S, Kindermann M, et al. Reducing enteric methane emissions from dairy cattle: two ways to supplement 3-nitrooxypropanol. J Anim Sci. (2019) 102:1780–7. doi: 10.3168/jds.2018-14534
220. Alemu AW, Shreck AL, Booker CW, McGinn SM, Pekrul LK, Kindermann M, et al. Use of 3-nitrooxypropanol in a commercial feedlot to decrease enteric methane emissions from cattle fed a corn-based finishing diet. J Anim Sci. (2021) 99:skaa394. doi: 10.1093/jas/skaa394
221. Duin EC, Wagner T, Shima S, Prakash D, Cronin B, Yáñez-Ruiz DR, et al. Mode of action uncovered for the specific reduction of methane emissions from ruminants by the small molecule 3-nitrooxypropanol. PNAS. (2016) 113:6172–7. doi: 10.1073/pnas.1600298113
222. Jayanegara A, Sarwono KA, Kondo M, Matsui H, Ridla M, Laconi EB, et al. Use of 3-nitrooxypropanol as feed additive for mitigating enteric methane emissions from ruminants: a meta-analysis. Italian J Anim Sci. (2018) 17:650–6. doi: 10.1080/1828051X.2017.1404945
223. Dijkstra J, Bannink A, France J, Kebreab E, van Gastelen S. Antimethanogenic effects of 3-nitrooxypropanol depend on supplementation dose, dietary fibre content, cattle type. J Anim Sci. (2018) 101:9041–7. doi: 10.3168/jds.2018-14456
224. Kim H, Lee HG, Baek YC, Lee S, Seo J. The effects of dietary supplementation with 3-nitrooxypropanol on enteric methane emissions, rumen fermentation, and production performance in ruminants: a meta-analysis. J Anim Sci Technol. (2020) 62:31. doi: 10.5187/jast.2020.62.1.31
225. Yu G, Beauchemin KA, Dong R. A review of 3-Nitrooxypropanol for enteric methane mitigation from ruminant livestock. Animals. (2021) 11:3540. doi: 10.3390/ani11123540
226. Allen MS. Effects of diet on short-term regulation of feed intake by lactating dairy cattle. J Dairy Sci. (2000) 83:1598–624. doi: 10.3168/jds.S0022-0302(00)75030-2
227. Oba M, Allen MS. Intraruminal infusion of propionate alters feeding behavior and decreases energy intake of lactating dairy cows. J Nutr. (2003) 133:1099. doi: 10.1093/jn/133.4.1094
228. Guyader J, Ungerfeld EM, Beauchemin KA. Redirection of metabolic hydrogen by inhibiting methanogenesis in the rumen simulation technique (RUSITEC). Front Microbiol. (2017) 8:393. doi: 10.3389/fmicb.2017.00393
229. Muetzel S, Katherine L, Janssen PH, Pacheco D, Bird, Walker N, et al. Towards the application of 3-nitrooxypropanol in pastoral farming systems. In: Proceedings from the 7th GGAA – Greenhouse Gas and Animal Agriculture Conference, Iguassu Falls, Brazil (2019).
230. Buddle BM, Denis M, Attwood GT, Altermann E, Janssen PH, Ronimus RS, et al. Strategies to reduce methane emissions from farmed ruminants grazing on pasture. Vet J. (2011) 188:11–7. doi: 10.1016/j.tvjl.2010.02.019
231. Leahy SC, Kelly WJ, Ronimus RS, Wedlock N, Altermann E, Attwood GT. Genome sequencing of rumen bacteria and archaea and its application to methane mitigation strategies. Animal. (2013) 7:235–43. doi: 10.1017/S1751731113000700
232. Subharat S, Shu D, Zheng T, Buddle BM, Janssen PH, Luo D, et al. Vaccination of cattle with a methanogen protein produces specific antibodies in the saliva which are stable in the rumen. Vet Immunol Immunopathol. (2015) 164:201–7. doi: 10.1016/j.vetimm.2015.02.008
233. Williams YJ, Popovski S, Rea SM, Skillman LC, Toovey AF, Northwood KS, et al. A vaccine against rumen methanogens can alter the composition of archaeal populations. Appl Environ Microbiol. (2009) 75:1860–6. doi: 10.1128/AEM.02453-08
234. Zhang L, Huang X, Xue B, Peng Q, Wang Z, Yan T, et al. Immunization against rumen methanogenesis by vaccination with a new recombinant protein. PLoS ONE. (2015) 10:e0140086. doi: 10.1371/journal.pone.0140086
235. Wright ADG, Kennedy P, O'neill CJ, Toovey AF, Popovski S, Rea SM, et al. Reducing methane emissions in sheep by immunization against rumen methanogens. Vaccine. (2004) 22:3976–85. doi: 10.1016/j.vaccine.2004.03.053
236. Wedlock DN, Janssen PH, Leahy SC, Shu D, Buddle BM. Progress in the development of vaccines against rumen methanogens. Animal. (2013) 7:244–52. doi: 10.1017/S1751731113000682
237. Renand G, Vinet A, Decruyenaere V, Maupetit D, Dozias D. Methane and carbon dioxide emission of beef heifers in relation with growth and feed efficiency. Animals. (2019) 9:1136. doi: 10.3390/ani9121136
238. De Haas Y, Pszczola M, Soyeurt H, Wall E, Lassen J. Invited review: phenotypes to genetically reduce greenhouse gas emissions in dairying. J Dairy Sci. (2017) 100:855–70. doi: 10.3168/jds.2016-11246
239. Flay HE, Kuhn-Sherlock B, Macdonald KA, Camara M, Lopez-Villalobos N, Donaghy DJ, et al. Hot topic: selecting cattle for low residual feed intake did not affect daily methane production but increased methane yield. J Anim Sci. (2019) 102:2708–13. doi: 10.3168/jds.2018-15234
240. Nkrumah JD, Okine EK, Mathison GW, Schmid K, Li C, Basarab JA, et al. Relationships of feedlot feed efficiency, performance, and feeding behavior with metabolic rate, methane production, and energy partitioning in beef cattle. J Anim Sci. (2006) 84:145–53. doi: 10.2527/2006.841145x
241. Hegarty RS, Goopy JP, Herd RM, McCorkell B. Cattle selected for lower residual feed intake have reduced daily methane production. J Anim Sci. (2007) 85:1479–86. doi: 10.2527/jas.2006-236
242. Fitzsimons C, Kenny DA, Deighton MH, Fahey AG, McGee M. Methane emissions, body composition, and rumen fermentation traits of beef heifers differing in residual feed intake. J Anim Sci. (2013) 91:5789–800. doi: 10.2527/jas.2013-6956
243. Mercadante MEZ, Caliman, A.P.D.M., Canesin RC, Bonilha SFM, Berndt A, et al. Relationship between residual feed intake and enteric methane emission in Nellore cattle. Rev Bras Zootec. (2015) 44:255–62. doi: 10.1590/S1806-92902015000700004
244. Sharma VK, Kundu SS, Datt C, Prusty S, Kumar M, Sontakke UB. Buffalo heifers selected for lower residual feed intake have lower feed intake, better dietary nitrogen utilisation and reduced enteric methane production. J Anim Physiol Anim Nutr. (2018) 102:e607–14. doi: 10.1111/jpn.12802
245. Dini Y, Cajarville C, Gere JI, Fernandez S, Fraga M, Pravia MI, et al. Association between residual feed intake and enteric methane emissions in hereford steers. Trans Anim Sci. (2019) 3:239–46. doi: 10.1093/tas/txy111
246. Oliveira LF, Ruggieri AC, Branco RH, Cota OL, Canesin RC, Costa HJU, et al. Feed efficiency and enteric methane production of Nellore cattle in the feedlot and on pasture. Anim Prod Sci. (2018) 58:886–93. doi: 10.1071/AN16303
247. Olijhoek DW, Løvendahl P, Lassen J, Hellwing ALF, Höglund JK, Weisbjerg MR, et al. Methane production, rumen fermentation, and diet digestibility of Holstein and Jersey dairy cows being divergent in residual feed intake and fed at 2 forage-to-concentrate ratios. J Dairy Sci. (2018) 101:9926–40. doi: 10.3168/jds.2017-14278
248. Berry DP, Cromie AR, Judge MM. Rapid communication: large exploitable genetic variability exists to shorten age at slaughter in cattle. J Anim Sci. (2017) 95:4526–32. doi: 10.2527/jas2017.2016
249. FAO GLEAM 2.0—Assessment of Greenhouse Gas Emissions Mitigation Potential. FAO (2017). ASvailable online at: https://www.fao.org/gleam/results/en/ (accessed May, 28 2022).
250. De Haas Y, Veerkamp RF, de Jong G, Aldridge MN. Selective breeding as a mitigation tool for methane emissions from dairy cattle. Animal. (2021) 15:100294. doi: 10.1016/j.animal.2021.100294
251. Howley P, Donoghue CO, Heanue K. Factors affecting farmers' adoption of agricultural innovations: a panel data analysis of the use of artificial insemination among dairy farmers in Ireland. J Agri Sci. (2012) 4:171. doi: 10.5539/jas.v4n6p171
Keywords: methane, beef cattle, rumen microbiome, pasture, nutrition, breeding
Citation: Smith PE, Kelly AK, Kenny DA and Waters SM (2022) Enteric methane research and mitigation strategies for pastoral-based beef cattle production systems. Front. Vet. Sci. 9:958340. doi: 10.3389/fvets.2022.958340
Received: 31 May 2022; Accepted: 09 November 2022;
Published: 23 December 2022.
Edited by:
Tiago Do Prado Paim, Goiano Federal Institute (IFGOIANO), BrazilReviewed by:
Jacobo Arango, International Center for Tropical Agriculture (CIAT), ColombiaPaulo M. T. Lima, Center for Nuclear Energy in Agriculture, University of São Paulo, Brazil
Copyright © 2022 Smith, Kelly, Kenny and Waters. This is an open-access article distributed under the terms of the Creative Commons Attribution License (CC BY). The use, distribution or reproduction in other forums is permitted, provided the original author(s) and the copyright owner(s) are credited and that the original publication in this journal is cited, in accordance with accepted academic practice. No use, distribution or reproduction is permitted which does not comply with these terms.
*Correspondence: Paul E. Smith, UGF1bC5TbWl0aEB0ZWFnYXNjLmll