- 1National Reference Laboratory of Veterinary Drug Residues, MOA Key Laboratory for Detection of Veterinary Drug Residues, Huazhong Agricultural University, Wuhan, China
- 2MOA Laboratory for Risk Assessment of Quality and Safety of Livestock and Poultry Products, Huazhong Agricultural University, Wuhan, China
Tuberculosis is a chronic consumptive infectious disease, which can cause great damage to human and animal health all over the world. The emergence of multi-drug resistant strains, the unstable protective effect of Bacillus Calmette-Guérin (BCG) vaccine on adults, and the mixed infection with HIV all warn people to exploit new approaches for conquering tuberculosis. At present, there has been significant progress in developing tuberculosis vaccines, such as improved BCG vaccine, subunit vaccine, DNA vaccine, live attenuated vaccine and inactivated vaccine. Among these candidate vaccines, there are some promising vaccines to improve or replace BCG vaccine effect. Meanwhile, the application of adjuvants, prime-boost strategy, immunoinformatic tools and targeting components have been studied concentratedly, and verified as valid means of raising the efficiency of tuberculosis vaccines as well. In this paper, the latest advance in tuberculosis vaccines in recent years is reviewed to provide reliable information for future tuberculosis prevention and treatment.
Highlights
- Although BCG vaccine is the only vaccine approved for tuberculosis prevention, its protective efficacy still needs further improvement.
- Multivalent subunit vaccines and DNA vaccines with good safety and targeting ability have the potential to replace BCG vaccines.
- Live attenuated vaccines are effective against tuberculosis, and the key lies in reliable targeted mutation technology.
Introduction
Tuberculosis is a chronic consumptive infectious disease caused by Mycobacterium tuberculosis (Mtb), which is greatly harmful to humans and animals (1–4). According to World Health Organization Global Tuberculosis Report, 10 million people were infected with tuberculosis and 3.44 million people died from tuberculosis in 2020 (5). Although human tuberculosis is a surmountable disease currently, the course of treatment is a long-term process, which can be influenced by many factors, such as medication continuity, patient compliance and clinical intervention timing. In addition, as the only available vaccine against tuberculosis, BCG's preventive effect is still limited and unstable yet (6, 7). On the other hand, diverse domestic and wild animals are potential hosts of Mycobacterium bovis (Mb), which may threaten the prevention and control of human tuberculosis. Furthermore, besides various wildlife reservoirs (brushtail possums, badgers, ferrets, elks, etc.) of Mb, it is estimated that the cost of national bovine tuberculosis control programme will exceed 1 billion pounds in the UK during 2014–2024 (8). And the cost of bovine tuberculosis reaches 3 billion dollars a year globally (4). Apart from a few countries, which can control bovine tuberculosis by test-and-slaughter strategies, it is hard to reduce the prevalence of bovine tuberculosis for other countries (especially for developing countries). Therefore, it is an urgent priority to seek efficient approaches for the prevention and control of this dangerous and persistent zoonosis (3).
In the in vitro treatment trial of tuberculosis, some classic first-line drugs including pyrazinamide, rifampicin, isoniazid and ethambutol have shown satisfactory curative efficacy. However, in the actual therapies of tuberculosis, the structural characteristics of tubercle bacillus and the generation of drug-resistant strains have brought great obstacles to cure illness. On the one hand, Mtb cell wall is rich in lipids. A large number of mycolic acid molecules surround the peptidoglycan layer, and the outer layer mainly consists of long-chain fatty acids, making Mtb impermeable to most antibiotics (9). The drug resistance mechanism of tuberculosis was discussed in more details in another review (10). On the other hand, some drug-resistant genes of Mtb have been detected by DNA extraction and PCR (11). In 2020, 132,222 of the bacteriologically confirmed tuberculosis cases were multidrug-resistant or rifampicin-resistant tuberculosis. The number of tuberculosis cases resistant to rifampicin and any fluoroquinolone, and resistant to at least one drug including rifampicin, fluoroquinolone, bedaquiline and linezolid reached 25,681 (5). Remarkably, the drug resistance rates are keep rising. To protect against tuberculosis, BCG vaccine serves as the only effective product. Although it has protective effects on extrapulmonary tuberculosis in infants, its prevention efficacy on adults is very unstable (12). Moreover, the tricky part is that the combined infection of tuberculosis with Acquired Immune Deficiency Syndrome (AIDS) causes latent tuberculosis to become active tuberculosis, which has greatly increased the incidence and mortality of tuberculosis (6). Therefore, there is no delay in developing new tuberculosis vaccines which can either enhance BCG vaccine efficacy or stimulate stronger immune effects by means of alternative approaches. According to previous studies, this review summarizes the latest advances of new tuberculosis vaccines in the last decade, including BCG vaccine, evolutionary products that improve existing BCG vaccine performance, novel substitutes such as subunit vaccines, DNA vaccines, and so on.
Immune response to Mtb infection
As an airborne zoonosis, tuberculosis is mainly transmitted by aerosol. If Mtb reach deep alveoli, they will be swallowed by alveolar macrophages and interstitial dendritic cells. The pathogen can lie dormant in macrophages without any replication, which leads to latent infection. And latent infection develops into active disease in asymptomatic or potential infected people and animals after immune system suppression. The factors influence the course of tuberculosis infection including host, bacteria and treatment. Tuberculosis patient-contact, health status, daily habits, vaccination, age and immunity are related inducements for hosts (12–14). The virulence, invasiveness and drug resistance of Mtb also affect the process of infection. Moreover, by producing phosphatase, serine/threonine kinase, Mtb can inhibit the maturation of phagosomes formed after being engulfed by macrophages (15, 16). Meanwhile, Mtb escapes from the body's immune system by expressing anti-stress genes, inhibiting autophagy and inactivating reactive oxygen species, and forms granulomas and causes latent infection (17–19). The influencing factors in treatment include medical conditions, economic status, patient compliance. The cycle of tuberculosis infection and the factors that impact the development of tuberculosis are shown in Figure 1. Additionally, alveolar macrophages are the main invasion targets of Mtb. In infected cells, not only the fusion of phagosomes and lysosomes is blocked, the production of reactive nitrogen intermediates (RNIs) is also hindered (7, 20, 21). This enables bacteria to tolerate the intracellular environment. In this progress, the activation of macrophages is of great importance. Medley et al. (22) discovered potential vaccine and drug targets in the interaction of Mtb and macrophages, such as the enzymes encoded by Cytochrome P450 125 gene and Cytochrome P450 142 gene, which involved in the cholesterol metabolism of Mtb.
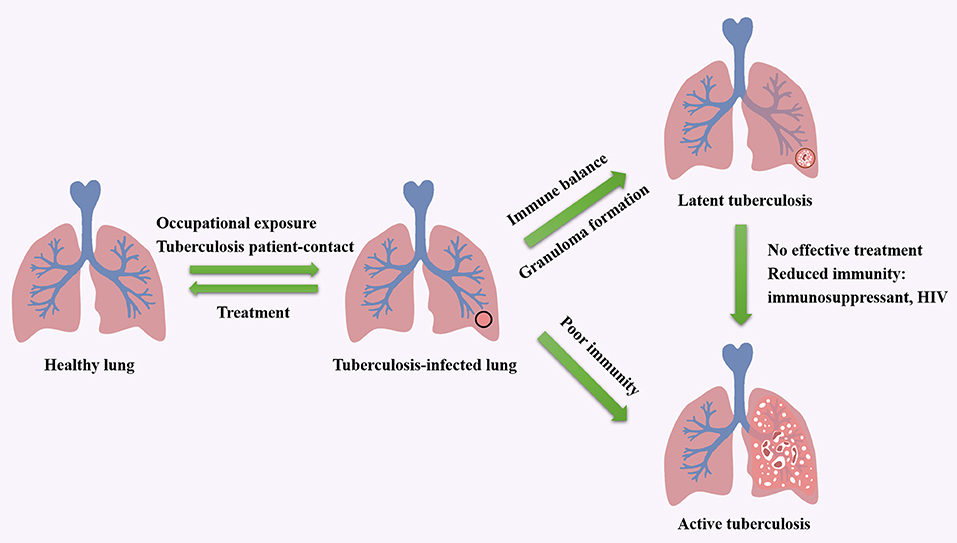
Figure 1. The cycle of tuberculosis infection and the factors that impact the development of tuberculosis.
After infected by Mtb, a series of immunologic cascade reactions are initiated. Mononuclear macrophages release TNF-α to activate T cells. Meanwhile, T cells divide into CD4+ T cells and CD8+ T cells after the stimulation of tuberculosis antigen. CD4+ T cells differentiate into Th2 cells, and IL-4, IL-5, and IL-6 secreted by Th2 cells can assist the activation of B cells to promote the production of antibodies against Mtb. IL-2 and IFN-γ produced by Th1 cells participate in the activation of macrophages together with the aforementioned cytokines (TNF-α, TNF-β), and facilitate the development of Th1-type immune responses. These cytokines induce anti-mycobacterial activities by activating macrophages, forming granulomas, increasing antigen presentation or producing effector molecules, respectively (2). As a biomarker of Th2-type immune response, excessive IL-4 has an antagonistic effect on the recovery of tuberculosis infection (23, 24). Buccheri et al. (25) confirmed that IL-4 deficiency could increase the host's resistance to tuberculosis infection. Monocyte macrophages and Th2 cells also play regulatory roles, which produce inhibitory transforming growth factor-β (TGF-β) and IL-10, respectively. They can prevent the activation of T lymphocytes by inhibiting the production of certain cytokines, e.g., IFN-γ, TNF-α and IL-12. There have been convincing evidences to prove that tuberculosis can induce IL-10 and TGF-β to suppress immune responses (21, 26, 27). As illustrated in Figure 2, cellular immunity and related cytokines play an important role in anti-tuberculosis infection, which is also essential to the protection potency for BCG vaccine. In addition, the roles of various biomarkers involved in the protection against tuberculosis are shown in Figure 3. As a result, the vaccines tend to induce Th1-type immune responses are preferred. However, it does not mean that Th2-type immunity and humoral immunity play an accomplice role of tuberculosis (28, 29). And this implies that it is necessary to seek the balance between cellular immunity and humoral immunity for improving a more effective tuberculosis vaccine.
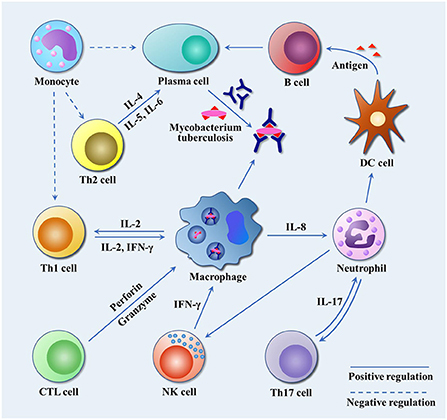
Figure 2. The immune response to Mtb infection. Mtb travels through the respiratory tract to the lungs, where it is engulfed by alveolar macrophages. By inhibiting the fusion of phagosomes and lysosomes, and blocking the production of active nitrogen intermediates, it escapes the body's immune killing and cause latent infection. Infected macrophages release IL-8 to attract neutrophils to the site of infection, and neutrophils in turn release chemokines to attract dendritic cells, NK cells and other immune cells to the site of infection to play an anti-tuberculosis role. IL-17 secreted by Th17 cells promote airway epithelial cells to produce IL-8 and G-CSF to attract more neutrophils, and participate in T cell-mediated IFN-γ and granuloma formation. NK cells release IFN-γ to promote Th1-type immune response. Dendritic cells, as professional antigen presenting cells, present Mtb antigen for B cells. B cells release antigen-specific antibodies to kill extracellular Mtb or Mtb released after macrophage death. Th2 cells release IL-4, IL-5 and IL-6 to assist the activation of B cells and promote the production of specific antibodies. In addition, macrophages release IL-2 to promote the proliferation and differentiation of Th1 cells, and Th1 cells secrete IL-2 and IFN-γ to reactivate infected macrophages. CTL cells differentiated from CD8+ T cells release perforin and granzyme to kill tuberculosis infected cells. Monocytes inhibit Th1 and Th2 by releasing TGF-β, and suppress the production of tuberculosis antibodies.
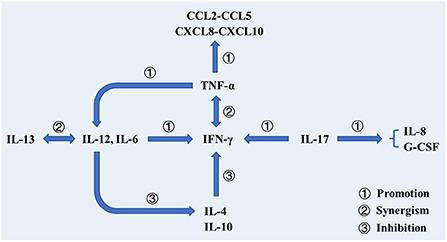
Figure 3. The roles of various biomarkers involved in the protection against tuberculosis. In addition to cooperating with IFN-γ to activate macrophages to phagocytose mycobacterium tuberculosis, TNF-α also activates T cells, prompting them to produce cytokines. Additionally, TNF-α can promote DC differentiation and promote IL-12 production. Il-12 cooperates with IL-13 to promote the production and efficacy of IFN-γ and inhibit the production of IL-4. IL-17 participates in the production of IFN-γ and promotes the production of IL-8 and G-CSF, thereby stimulating neutrophils to the site of infection.
BCG vaccines
BCG vaccine is a live attenuated vaccine made from suspensions of attenuated bovine type tubercle bacilli with enhanced macrophage activity, which potentiates the ability of macrophages to eliminate intracellular parasitic bacteria. However, as mentioned above, the protection efficacy of this classic vaccine is variable in adults, which limits its application. Some researchers put forward that the reason for this change might be the effect of environmental mycobacteria. It masks or blocks the efficacy of BCG vaccine (28). The masking hypothesis supposes that the immunity caused by environmental tuberculosis hides the additional effect of BCG vaccine. According to the blocking hypothesis, the immunity caused by environmental tuberculosis eliminates BCG vaccine and prevents BCG vaccine from working. Apart from environmental mycobacteria, the effector memory T cells stimulated by BCG vaccine will attenuate with time, which is crucial to the protection against tuberculosis (30, 31). The nutritional status of host is crucial to maintain the good reaction capacity of immune system, which accounts for organic resistance to infections, including tuberculosis. And the malnutrition may influence prospective effects of BCG vaccine by inhibiting the production of T cells and some cytokines (32). To improve the efficacy of BCG vaccine, some adjuvants (lipopeptide, fusion protein and lactoferrin) were used and achieved ideal experimental results (33–35). Moreover, researchers proposed that the optimal activation of CD4+ and CD8+ T cells was significant, and the inability of BCG vaccine to effectively activate CD8+ T cells was the reason for its poor performance (29).
On the other hand, alveolar lining fluid (ALF) is an internal factor that may impede the function of BCG vaccine. In accordance with the route of natural infection of Mtb, the first barrier lying in respiratory tract is ALF. ALF is composed of surfactant lipids and associated proteins, as well as of an aqueous hypophysis rich in innate host defense molecules, including hydrolytic enzymes, surfactant proteins A and D, complement proteins and immunoglobulins (36). Under natural conditions, hydrolases remove some lipids, such as dimethyl trehalose at the periphery of the cell wall of Mtb (37). The new antigen motif will be exposed, which is bound to affect the immune responses caused by Mtb in vivo (36). These changes may affect the uptake and phagocytosis of Mtb by immune cells. BCG vaccine is usually injected intracutaneously without ALF treatment, and the in vivo protective effects cannot reverse this blocking influence. Accordingly, what is noteworthy is that the potency of BCG vaccine can be improved by inoculating mice with ALF-treated BCG vaccine (36).
As the first antituberculosis vaccine, BCG has been applied to clinical use over 100 years. During this time, continuing passage of BCG strains have produced various substrains with different genetic mutations, such as BCG Pasteur, BCG Danish, BCG Sweden, and so on (38). The diverse immune efficacy of these substrains within animal models were described by numerous studies and systematically summarized in a previous review (39–42). A random trial carried out in Hong Kong involved 303,092 infants revealed that BCG Pasteur vaccine could reduce the tuberculosis risk by 45% in comparison with that of BCG Glaxo (43). And there was a similar phenomenon observed in a Kazakhstan study of the tuberculosis risk related to neonates, resulting in a reduction of 69%, 22% for BCG Tokyo and BCG Moscow vaccination, respectively (44). However, these seems to be unconvincing evidences owing to distinct study protocols, for instance, inoculation regimen, administration route, challenge schedule and immune evaluation, which failed to attribute the various protection efficacy to BCG strains (39, 45, 46). Moreover, no significant influence was detected among different BCG strains through meta-analysis (45). And some studies suggested the potential reasons might lay in exposure to environmental mycobacteria, prior infection of Mtb and the environment condition of trials (45, 47–49). Therefore, it is hard to determine the relationship between BCG strains and immune efficacy, and a standard scheme including experiment operations and evaluating indicators is an urgent need for assessing vaccination potency of different BCG strains.
In addition, microtubule-associated protein 1A/1B-light chain 3 (LC3) related phagocytosis and autophagy enhance antigen presentation and may contribute to vaccine efficacy, while Mtb can inhibit above effects. And BCG vaccine strain elicits even less LC3-trafficking than Mtb, which may explain its limited efficacy (50). Köster et al. knocked out the CpsA gene which could inhibit LC3 traffic pathways from mc26206 vaccine strain (ΔCpsA) and inoculated mice. They found that vaccination of mice with mc26206, mc26206 ΔcpsA and BCG Danish resulted in similar protective efficacy against Mtb challenge (50). These phenomena suggest that the improvement of BCG vaccine in some aspects may be limited. Some genes missing in BCG vaccine can be supplemented by appropriate means for immune enhancement, but the impact of environmental tuberculosis is difficult to eliminate. However, as the only tuberculosis vaccine currently available, it is an excellent choice to use BCG vaccine as a breakthrough.
Another problem of BCG vaccine is that it brings doubts in the distinction between primary tuberculosis infection and vaccination both in human and animal. The tuberculin skin test is fundamental to diagnose tuberculosis. However, previous BCG vaccine inoculation may interfere with the tuberculin skin test results, which is one of the most important obstructions for animal vaccination against tuberculosis. Recently, Chandran et al. developed a modified BCG vaccine, which could distinguish between guinea pigs inoculated by BCG vaccine and infected with tuberculosis (51). In its preparation of BCG vaccine, some antigen (ESAT-6, CFP-10, etc.) genes were deleted from the genome. The specific antigens between BCG vaccine and environmental strains were used to stimulate the production of IFN-γ in peripheral blood mononuclear cells, which contributed to distinguish vaccinated animals from naturally infected animals. However, this method requires strict experimental conditions. They deleted unnecessary genes for the function of BCG vaccine. These genes, together with ESAT-6 and CFP-10, were used in a skin test for identification (51). The diagnostic and preventive efficacy of this modified BCG vaccine needs to be further validated. Nevertheless, this strategy holds great promise for the control and prevention of tuberculosis as well as other diseases.
Besides, the application of BCG vaccine is lethal for HIV patients with immunodeficiency (52). And TBVAC85, a derivative of mycobacterial shottsii expressing Mtb antigen 85B, is considered a safer alternative to BCG vaccine. Inoculated guinea pigs were subsequently challenged with tuberculosis aerosols and it was found that the protective effect of TBVAC85 was similar to that of BCG vaccine. Moreover, it has a higher safety profile than that of BCG vaccine and deserves further study (53). It is expected to provide a reference regimen for tuberculosis prevention in HIV patients. Moreover, Muttaqin et al. fabricated a combined vaccine of tuberculosis and AIDS, and screened out the dominant epitopes of the two through immune epitope database. In theory, the MHC-I and MHC-II epitope coverage of this vaccine can reach 85 and 99% around the world, respectively. To verify its in vivo effect, further animal experiments need to be conducted (54).
BCG vaccine is injected intradermally nowadays, but its original administration route is oral inoculation. And it is still a challenge to evaluate diverse vaccination routes for the judgement of best delivery approach to stimulate superior protective immune effects. To protect from pulmonary tuberculosis, aerosol might be a better way to delivery BCG vaccine, which can induce the mucosal immunity within trachea (55). Meanwhile, in comparison with transdermal delivery routes, intranasal or inhalation taken BCG vaccine stimulated more effective protective immunity (56–58). And aerosol vaccination is a promising method to maximize the immune effect of BCG vaccine. In addition, intravenous injection of BCG vaccine is proved to be more effective than other routes in animal model. In Rhesus macaques, intravenous inoculation of BCG vaccine exhibited a better protection without pulmonary lesion than that of intradermal inoculation (59, 60). Nevertheless, prior to efficacy, its intravenous application in human needs comprehensive examinations to ensure safety.
Despite above defects, there have been some attempts to explore the protection effect of BCG vaccine against animal tuberculosis recently. To assist the control of bovine tuberculosis for the British cattle, an evaluation was performed by inoculation with BCG Danish in neonatal calves. Two independent groups of cattle were endotracheally challenged by Mb after 12 and 24 months postvaccination, respectively. By comparison with the unvaccinated group, the lung and lymph node pathology scores reduced in both vaccinated groups, while the reduction of the 12 months group was significant statistically. The antigen-specific IFN-γ remained in vaccinated cattle's blood for about 90 weeks. The IFN-γ-secreting central memory T cells of the 12 months group were obviously abundant than those of the unvaccinated group, while the difference between the 24 months group and the unvaccinated group was not notable. These observations proved the protection of BCG vaccine maintaining 12 months in cattle, and revaccination or boost programme might be needed to guarantee a long-term inoculation effect against Mb infection (61). For defensing natural Mb infection within free-living badgers, a field trial of oral vaccination of BCG vaccine was carried out. The median time to seroconversion of the vaccinated badgers was 413 days, which remarkably longer (p = 0.04) than 230 days of the unvaccinated badgers. The Vaccine Efficacy (VE) based on hazard rate ratios rose with the increase of vaccination rate. After the trail, a notable diversity of lesion proportion (9% for the vaccinated group, and 26% for the unvaccinated group) according to the Mb cultures from badgers was determined by post-mortem. And the results provided an alternative tool to control the tuberculosis incidence of wild badgers (62). Moreover, other studies have demonstrated the protection effects of oral BCG vaccine strains Pasteur and Danish are similar in badgers (63). Recent anti-Mb studies concerning domestic animals and wild animals by means of BCG vaccine showed encouraging outcomes, which implied the feasibility of finding a suitable vaccine. Although there is absent of available vaccine for animal tuberculosis, it is still worth to keep investigating the practicability, inoculation strategy and immune reaction on the basis of BCG vaccine for developing new vaccines not only for animal but also for human.
Improved BCG vaccines
Although the protective efficacy of BCG vaccine is unstable, it is the only prophylactic vaccine approved for use. Modification of BCG vaccine to improve its antituberculosis ability represents a relatively easy way to control tuberculosis. Studies in this field have also made promising results, for instance, a boost of immune competence through the addition of adjuvants and prime-boost strategies.
Application of prime-boost strategy
The prime-boost strategy is usually to inoculate BCG vaccine first and then enhance it with homologous or heterologous vaccine. And other vaccines can be used for prime immunization as well (64). To evaluate the putative correlation between protective efficacy and CD4+ T cell subtype phenotype, Choi et al. constructed the HSP90-E6/CIA05 vaccine composed of HSP90 and ESAT-6 proteins accompanying with adjuvant CIA05. By applying a prime-boost strategy in combination with BCG vaccine, the results demonstrated that mice in the HSP90-E6/CIA05 boost group exhibited significantly less lung inflammation and lesion areas compared with those in the BCG group and the ESAT-6/CIA05 enhanced group. Moreover, the bacterial burden in the lung and spleen was significantly lower in HSP90-E6/CIA05-boosted mice than that in ESAT-6/CIA05-boosted mice (65). CIA05 is a toll-like receptor 4 (TLR4) agonist purified from an Escherichia coli strain that expresses lipopolysaccharide (LPS) with short carbohydrate chains and detoxifies by alkaline hydrolysis (66). The ability of CIA05 to promote Th1-type immune response was proved in the development of human papillomavirus vaccine (67). These studies manifested the important role of TLR4 in anti-tuberculosis immunity (68). TLR4 also can activate MyD88-, MAPK- and NF-κB-dependent signaling pathways. The subunit vaccine composed of tuberculosis heat shock protein 90 family Rv2299c and ESAT-6 was developed based on this principle, which significantly improved the effect of BCG vaccine (69). Additionally, several tuberculosis antigens (HspX, PPE44 and EsxV) were encapsulated in liposomes containing dimethyldioctadecylammonium/trehalose-6,6-dibehenate as a boost vaccine, which was used after BCG vaccine. In terms of the generation of related cytokines (IFN-c, IL-12 et al.), IgG and enhancement of immune memory after vaccination, this vaccine exhibited a strong Th1 promoting ability along with a prominent BCG vaccine enhancing effect (70). And more encouraging results have been reported by application of prime-boost strategy (33, 69, 71–73). The combination of primer and booster mainly depends on the BCG potency. The common components of primer or booster other than BCG vaccine in prime-boost strategy include antigen, lipopeptide and live attenuated strain, which promote the cellular immune responses caused by BCG vaccine. And this strategy maybe meaningful to improve the immunogenicity of attenuated BCG strains, and in turn to distinguish critical components in BCG vaccine for keeping its protection potency.
Moreover, the constituent antigens of boost adjuvants are worth to design elaborately. Researchers found that the DNA vaccine expressing tuberculosis antigen Ag85a had a protective effect on mice when used for prime immune, while its protective effect disappeared when used for boost immune (74). A phenomenon called original antigenic sin may be able to explain it well. The original antigenic sin suggests that in vivo immune system makes an immune response and forms immune memory based on the initial antigen immunity. When the same antigen with slight changes in its epitope appears again, immune system will follow existed immune memory instead of adapting to its slight differences of the epitope (75). This phenomenon is attributed to the cross-reaction of T helper cells and antigens (76). Therefore, in order to achieve desired prime-boost strategy, the immune responses triggered by the selected antigen of boost adjuvants should consistent with original T cell responses introduced by the initial immunization of BCG vaccine.
The inoculation interval of prime-boost strategy is also worth investigating. MTBVAC, obtained from live attenuated Mtb strain, was constructed under the genetic background of clinical isolate MT103. Its two genes, PhoP and fadd26, are necessary for the virulence of Mtb (77, 78). The evaluation of MTBVAC showed a better immune potency by applying prime-boost strategy. Two vaccinations with an interval of either 6 or 20 weeks exhibited an enhancement effect in the BCG prime-MTBVAC boost group. For the MTBVAC prime-BCG boost group, the results of two inoculations with an interval of 6 weeks indicated a similar facilitation effect. However, with the extension of vaccination interval, its protective effect gradually decreased to the level of the BCG group (79). The study of GamTBvac vaccine by Vasina et al. was also concerned with inoculation intervals. After a long-term BCG vaccination, GamTBvac could still improve its effect. GamTBvac, a new candidate vaccine as a BCG vaccine booster, contains dextran binding domain modified Ag85A and ESAT6-CFP10-Mtb antigens and CpG ODN adjuvant. It was proved that the semi-dose vaccine group had stable IFN-γ production ability and higher antigen-specific IgG titer compared with the low-dose and high-dose vaccine groups (80). Until now, a universal inoculation interval of prime-boost scheme has not been given by existing researches. Different kinds and sequences of primer and booster often generate various protection effects. This may be attributed to the lack of complete recognition in tuberculosis immunity and related key points of protection. However, it is necessary to build an operation standard including dosage regimen, vaccination route, inoculation interval, animal model and evaluation indicator to provide reference for subsequent studies.
Additionally, some studies have shown that mucosal delivery enhances the generation of tissue-resident memory T cells, and these T cells may inhibit the early invasion of bacterial infection (81). Hence, it is rational to develop modified BCG vaccine administrated through mucosa in virtue of prime-boost strategy. Besides, Lactobacillus plantarum was utilized as a platform to produce tuberculosis antigen protein for BCG vaccine preparation, which had a certain protective effect (82). But it was not as fine as single parenteral inoculation of BCG vaccine (83).
Although it has been absent of approved vaccines for animals so far, constructive efforts including prime-boost strategy have been conducted to make full use of BCG. A long-term evaluation over 2 years tested the protection effect of revaccination with three different boost agents (BCG, ESAT-6 and Antigen 85A complexes, Mb culture filtrate) in BCG-vaccinated cattle. After endotracheally challenged with virulent Mb, cattle were sacrificed and necropsied. The lung and pulmonary lymph node lesion scores were apparently reduced in above three prime-boost groups in contrast to the unvaccinated group, while a single inoculation with BCG vaccine could not protect cattle from Mb challenge (84). In addition, immunization boost by a human type 5 (Ad5)-based Ad5-Ag85A vaccine, Ad5-Ag85A/Rv0251/Rv0287/Rv0288 (Ad5-TBF) vaccine and protein TBF in emulsion adjuvant (Adj-TBF) vaccine in cattle was studied, which resulted in similar strengthen effects of the BCG-priming vaccination (85). These phenomena revealed revaccination could boost the protection of BCG vaccine in cattle. Meanwhile, oral bating is a feasible approach for vaccine administration in the wild, which is convenient for prime-boost strategy. Gortazar et al. (86) found that oral revaccination with BCG vaccine in Eurasian wild boar elicited a potent protection against Mb field strains under experimental conditions. These observations indicated revaccination of BCG vaccine is worth to extend to the future field vaccination trials for verifying its priority of prime-boost strategy.
Introduction of adjuvants
Adjuvants are kinds of non-specific immune enhancers, which can facilitate the immune response to antigens or change the type of immunoreaction when administrated alone in advance or together with antigens. An appropriate adjuvant is an excellent supplementary for vaccines with low immunogenicity (87). The adjuvants summarized in this article are shown in Table 1. Combined with immune mechanism of tuberculosis, the application of adjuvants in autophagy, Th1-type immune response, cytokines and other aspects to improve the effect of BCG vaccine have been discussed subsequently.
Autophagy-facilitating and TLR-2 motivating C5 peptide originated from CFP-10 protein was over-expressed in BCG vaccine with Ag85B to prepare recombinant BCG 85C5 vaccine, in which C5 peptide and Ag85B acted as adjuvants. This vaccine not only promotes the production of Th1-type cytokines, but also inhibits MARCH 1 ubiquitin ligase which degrades MHC-II and enhances the surface expression of MHC-II in macrophages (88). Autophagy is involved in the immune mechanism against tuberculosis. And it is a beneficial attempt to improve autophagy-dependent interaction between antigen-presenting cells (APCs) and T cells in neonates and adults. Additionally, there is a critical review focused on constructing anti-Mtb vaccines with the help of autophagy targeting ability (99).
In addition to autophagy, Th1-type immune response plays an important part in anti-tuberculosis process. Although T-effector memory (TEM) cells, which generate Th1-type immune response cytokines (100), can effectively resist tuberculosis infection, they have low or no proliferation ability. On the other hand, T central memory (TCM) cells can generate TEM cells. Therefore, increasing TCM cells response and providing continuous TEM cells is a strong guarantee for long-term resistance to tuberculosis infection. Kv1.3 is a potassium channel, which is mainly expressed in TEM cells and crucial to the maintenance of related cells and the function of effectors. The knockdown of Kv1.3 led to the differential regulation between TCM cells and TEM cells, which was beneficial to the production of TCM cells (101). 3,4,5,7-tetrahydroxyflavone, also known as luteolin, a plant flavonoid that inhibits Kv1.3, has been studied. The effect of BCG vaccine was improved by giving luteolin to mice at the same time (102). Similarly, IFN-I (IFN-α and IFN-β) is important to the long-term survival of CD8+ T cells by promoting the differentiation/activation of human and mouse dendritic cells (DCs) in response to specific antigens (103–105). After took IFN-α and BCG vaccine in combination in mice, the immune results were superior to those of BCG vaccine, while single IFN-α treatment worsened pulmonary tuberculosis (90).
The dinucleotide SB 9200 (Inarigivir) is a nucleotide-binding oligomeric domain agonist containing protein 2 (NOD2) and retinoic acid-inducible gene I (RIG-I). Both are essential for initiating an effective host immune response against intracellular infection. Khan et al. mixed Inarigivir and BCG vaccine to inoculate mice, resulting in obvious growth of the expression level of IL-12, TNF-α, and IFN-β and relevant cytokines. It was also observed that the ability of macrophages to kill Mtb was improved and the bacterial load in the lungs of mice was reduced more significantly than that of BCG vaccine alone (89). Although the improvement effects of each kind of adjuvant are obvious, there remains many works to illuminate the correlations within immune mechanism of tuberculosis, and to seek most suitable adjuvants for promoting BCG vaccine.
Inactivated vaccines
For preventing animal tuberculosis, inactivated vaccines are of great importance due to no diagnostic cross-reactions and rare virulent strain survival. Therefore, most of the reports focused on heat-inactivated Mb vaccines in recent investigations concerning animal tuberculosis prevention. Thomas et al. gave a challenge with Mb field strains to the red deer after their oral vaccination of heat-inactivated Mb (n = 5/group). In contrast to the unvaccinated control group, the tuberculosis lesion scores of the heat-inactivated Mb group showed a 53% reduction with significant differences. And the vaccination didn't interfere with the IFN-γ assay and antibody detection for TB diagnosis. The level of cytokines (TNF-α, IFN-γ, IL-1β, IL-10 and IL-12) in plasma kept steady after vaccination, while the challenge caused an increase of IL-1β level in all the groups (106). On the contrary, subcutaneous injection of BCG vaccine and intramuscular vaccination of heat-inactivated Mb elicited the response of IFN-γ and some antibodies, which hindered subsequent TB diagnosis (107, 108). As the immune mechanism of TB infection in red deer is still indefinable, the hint provided by the change of above cytokine levels is limited. And further study could make an improvement by increasing the number of animal samples for more representative data to reveal the immunologic profile.
As a primary natural host of Mtb complex in Mediterranean woods, the Eurasian wild boars confer a significant risk for the control of cattle tuberculosis. To evaluate the possibility of wild boar piglet vaccination against tuberculosis, a 4-year field trial was conducted in combination with a mathematical modeling analysis. The heat-inactivated Mb and BCG were filled into selective piglet feeders as vaccine baits in managed sites and natural sites. And the uptake ratio reached 50–74 and 89–92% in natural sites and managed sites, respectively. In comparison with local original tuberculosis morbidity (50–100%), the prevalence of the unvaccinated group increased 6%, while this number notably decreased by 34% in the heat-inactivated Mb group. And the differences in prevalence of other groups were not obvious. With the help of mathematical modeling, the long-term effect of this vaccination trial was assessed, which indicated the prevalence reduction of heat-inactivated Mb vaccinated piglets and the population size increasement of wild boars (109). Although this work had certain regional limitations, the above conclusions preliminarily demonstrated the application prospect of heat-inactivated Mb vaccines as a vital part of tuberculosis prevention for wild boars.
In another study concerning wild boars, the efficacy and safety of heat-inactivated Mb vaccine was investigated in a farm via parenteral inoculation. 3–4 months old piglets were inoculated by longissimus dorsi injection. They were revaccinated 1–2 months later and given yearly revaccination afterwards before their release or productive end. Observed by visual inspection or post-mortem test, there were no adverse reaction after vaccination. The prevalence of tuberculosis compatible lesion was 4.1 and 12.1% for the inoculation group and the control group, respectively. And the mean lesion score exhibited no difference (P > 0.05). The results indicated the safety and protection potency of this heat-inactivated vaccine for wild boar piglets against natural challenge. Moreover, this study implied the possible progress of heat-inactivated Mb in lesion prevalence decrease achieved by scheduled vaccination (110).
As goats are especially vulnerable to tuberculosis infection, a recent report estimates the protection effect of parenteral heat-inactivated Mb vaccine in goats. Mycobacterium caprae was applied to challenge the vaccinated (heat-inactivated Mb and BCG vaccine) and unvaccinated goats. Compared with the unvaccinated group, the relief of extrapulmonary and thoracic lesion volume, and less bacteria within pulmonary lymph nodes were observed in both BCG group and heat-inactivated Mb group with similar levels. Additionally, heat-inactivated Mb vaccine showed no influence on IFN-γ assay (111). These observations are in accordance with previous reports carried out in laboratory and field conditions (112, 113). Hence, to develop a safe and efficient heat-inactivated vaccine against tuberculosis for goats, more factors, such as dose delivery routes, lower challenge bacterial dosage and larger sample size should take into account to improve further assessments. Moreover, Mycobacterium caprae was utilized to build a tuberculosis infection model with gross lesions within the respiratory system in sheep for the first time. And heat-inactivated Mb vaccine and BCG vaccine were assessed in this model. As a result, the reduction of the bacterial load and lesion regions was remarkable in the BCG group, while the heat-inactivated Mb group showed no protection effect as regards to the bacterial load and lesion volumes. These different conclusions may be caused by the delivery route (oral vaccination for the heat-inactivated Mb group, subcutaneous vaccination for the BCG group) and the relatively low dose of the heat-inactivated vaccines, which is worth a further work to promote this heat-inactivated vaccine (114).
European badgers are considered to be a primary tuberculosis reservoir, which may be natural hosts to infect cattle. Based on the successful applications of heat-inactivated vaccines in red deer and wild boars, Balseiro et al. employed heat-inactivated Mb to protect badgers against tuberculosis. The vaccination was conducted by oral administration of heat-inactivated Mb or BCG, respectively. After endobronchial challenge with pathogenic Mb, the microscopic and gross lesions within tuberculosis-infected badgers of both vaccinated groups decreased in the degree and volume. The spread of Mb in vaccinated badgers was inhibited as well. And no adverse clinical signs were observed in the vaccinated groups. In endemic countries, this oral heat-inactivated vaccine was proved to be a potential candidate suit to badgers (115). Furthermore, in order to figure out the protection mechanism of heat-inactivated vaccines in badgers, the immune responses of lung granuloma cells were analyzed according to the lesion immunopathology. By inspection of the lung granulomas from vaccinated (heat-inactivated Mb group and BCG group) and unvaccinated badgers, macrophages existed extensively in the granulomas, while B cells and plasma cells were less in abundance. And T cells were not found in above granulomas. These results illustrated that phagocytic cells were prone to promote non-specific innate responses rather than adaptative humoral immune responses. The plasma cells of the heat-inactivated Mb group were more than those of the BCG group, which indicated the formation of adaptative humoral responses (116).
Additionally, heat-inactivated vaccine was used to vaccinate in zebrafish model for controlling fish mycobacteriosis. In the Mycobacterium marinum challenged zebrafish model, intraperitoneal injection of heat-inactivated Mb could significantly promote the survival rate (30–70%), and reduce the content of mycobacteria in granulomas by detecting the DNA level of Mycobacterium marinum. Through mycobacteria staining and immunolabelling, the qualified granulomas in zebrafish of the heat-inactivated vaccine group were apparently less than those of the unvaccinated group. To identify the influence of vaccination on immune reaction, the mRNA levels of akr2, C3 and IL-1β were evaluated by qRT-PCR. And the results demonstrated a C3 pathway-based innate immune response with the regulation of Ark2 protein, which was consistent with the protective mechanisms in other species (117). Furthermore, mucosal administration of the heat-inactivated Mb by immersion was conducted in zebrafish. And it provided a similar protection effects in the vaccinated group against Mycobacterium marinum, which stimulated IgM antibodies for Mb antigens. The C3 pathway-based innate immune response was found to be the protective mechanisms as well (118).
Due to the complicated relationship behind tuberculosis infection among different livestock and wild animals, the strain sources of heat-inactivated vaccines are expansive. Therefore, the chosen strains are not only related to protection efficacy, but also important to prevent subsequent spreading of antigenicity factor to other animal species, which may interfere with the results of tuberculosis diagnosis. Considering the living habits and immune characteristics of diverse wild animals, it is hard to set a standard study protocol suitable for various hosts. Unlike vaccination studies for human and livestock, the heat-inactivated vaccines can be prepared as baits for once or multiple oral administration, which is much more feasible in practical world. Hence, it provides more possibilities to make further progress in developing reliable inactivated vaccines according to the immune characteristics of wildlife.
Live attenuated vaccines
Different from classic vaccines, the immunogenicity of live attenuated vaccines is acquired from treated pathogens with weakened toxicity, which achieves a balance between immune response and non-pathogenicity. In recent years, the research on live attenuate vaccines against human and animal tuberculosis is quite active.
Mtb mutants
Levillain et al. studied a live attenuated vaccine on the basis of GC1237 mutant which caused the outbreak of tuberculosis on Canary Island. The mutant strain was inactivated both in Rv1503c gene (responsible for surface glycolipid synthesis) and two-component global regulator PhoPR. This vaccine retained obvious virulence in the intravenous infection model of immunodeficient SCID mice. Therefore, researchers attenuated the mutant again and eliminated the two-component system PhoPR which regulated the virulence of numerous Mtb by gene knockout. The latter vaccine had the same protective effect as BCG vaccine in CB6F1 mice and C3H/HeNRj mice. Additionally, considering that the vaccine originated from the Beijing strain, C57BL/6 mice were challenged with Beijing strain HN878 and European and American strain M2 respectively. And it was found that the vaccine had stronger resistance to HN878 than M2 as expected (119). The results indicated that live attenuated vaccines were prone to be influenced by genetic background, which might generate BCG vaccines with diverse protective power around the world. It also suggested that local vaccines could be prepared from strains prevalent in corresponding regions.
ST28 is a mutant of Mtb, which lacks the gene encoding the extracellular sigma factor SigE. It is strongly attenuated in comparison with BCG vaccine. But ST28 can induce more effective protection of mice from Mtb infection. After inoculation of guinea pigs, the CFU formation and lung lesion area challenged by the highly virulent tuberculosis strain H37Rv were reduced, and the protective effect was similar to that of BCG vaccine. However, its protection mechanism is still not clear (120).
Rogelio-Hernandez-Pando et al. constructed and identified a label-free double mutant of Mtb sigE fadD26, which was also weaker than BCG vaccine, and more effective than single sigE mutant. Its protective effect was similar to that of BCG vaccine in guinea pig model. The results indicated that sigE fadD26 mutant might be used as an effective candidate to replace or supplement BCG vaccine (121). MTBVAC vaccine was also a promising alternative to BCG vaccine, which was based on the modified form of human Mtb gene. The vaccine was relatively safe with immunogenicity to tuberculosis antigens of adults and newborns. By inducing glycolysis, glutamine decomposition and accumulating histone methylation markers at the promoter of pro-inflammatory genes, MTBVAC vaccine could produce trained immunity as well. Trained immunity is related to the long-term epigenetic and metabolic reprogramming of innate immune system cells. Therefore, if it has a better protective effect on tuberculosis in the third stage efficacy test, this vaccine may be considered as a substitute for BCG vaccine available at present (122). Although it has been a long time since the invention of BCG vaccine, which represents the most successful Mtb mutant, there is no breakthrough in exploring novel alternatives. The targeted genes are involved in virulence but do not participate in immune protection. Nevertheless, the limited knowledge about the specific mechanism of host-Mtb interactions and related genes accounting for immune response hinders the development of ideal mutant candidates. Until now, researchers are still seeking genomic loci eliminating virulence from Mtb genome without damaging immunogenicity of candidate vaccine strains. This dilemma may continue until figuring out the relationship between Mtb genome and immune effect. And the situation of subsequent mentioned Mb mutant is similar.
Mb mutants
The knockout of causative genes from wild type mycobacterium pathogens is an appealing approach for circumventing BCG's insufficient protection. Accordingly, in order to seek potential candidate vaccines for bovine tuberculosis, Federico Carlos Blanco et al. generated an attenuated mutant strain (Δmce2) of Mb by deleting mce2A and mce2B genes, and detected its immunological properties in a cattle model of bovine tuberculosis. The cattle administrated with Δmce2 exhibited an equivalent activation of CD4+ T cells to that of cattle infected with the original Mb strain. And the amount of mRNA Th1 cytokines was higher in the peripheral blood of the Δmce2 group after in vitro stimulation. Moreover, in comparison with the BCG group, the histopathological lesion scores of the Δmce2 group were notably lower. However, the inoculation of Δmce2 in cattle resulted in a positive response of tuberculin skin test, which might be further modified by locating and eliminating related immunogenic genes. And this Δmce2 was considered to be a promising vaccine for cattle against Mb (123, 124).
As revealed in a previous review, phoP gene involves in the adjustment of Mb lipid metabolism, which is associated with down-regulated expression of diacyltrehaloses, polyacyltrehaloses and sulfolipids. Additionally, phoP gene affects Mtb complex virulence by influencing ESX-1 secretion system related genes as well. And the expression of ESAT6 and CFP10 in Mtb H37Rv ΔphoP and Mtb H37Ra is obviously decreased (125). Hence, Elizabeth and collaborators knocked out phoP gene (Rv0757) in Δmce2 to obtain a novel Mb mutant strain (Δmce2-phoP). It was observed that the virulence of Δmce2-phoP was significantly attenuated than that of wild type strain in mice. By compared with the BCG group, Δmce2-phoP inoculation stimulated Th1 cytokines after 30 days in mice without statistical differences. And the protection of Δmce2-phoP was proved to be a potential candidate as Mb vaccine in cattle (126).
Together with p55, which encodes an efflux pump or transporter, the gene that encodes P27/LprG forms a virulence operon. On this basis, a study constructed an Mb mutant Δp27-p55, which exhibited a distinct attenuation than its original strain, but more infectious than BCG Pasteur in nude mice. Mb or Mtb strains challenged mice and guinea pigs were used to evaluate the protection of Δp27-p55. The results of pathology and bacterial loads in spleen were similar between Δp27-p55 and BCG vaccine, while the effect of Δp27-p55 were inferior to that of BCG vaccine in lung. However, the lower amount of IFN-γ and IL-2 negative expression in cattle of the Δp27-p55 group represented an inadequate Th1 response, which indicating P27's immunogenic potency. And further research need to be conducted to improve Δp27-p55 as a TB vaccine (51, 127).
Although the tests of Differentiating Infected from Vaccinated Animals (DIVA) for tuberculosis have been invented, their cost is high, accompanying with an insufficient sensitivity. Hence, vaccines without disturbance to animal tuberculosis detection are significant for clinical practice. This dilemma may be solved by means of searching potential DIVA antigens from Mb or locating the antigens irrelevant to the persistence and defense in BCG vaccine. According to this clue, a diagnostic-compatible triple knock-out ΔBCG TK strain was reported by deleting unnecessary genes in BCG Danish, which showed a similar protection effect to wild-type BCG in Mb-infected guinea pigs (51). In contrast to other potential tuberculosis vaccines other than BCG vaccine, this study provides a possibility of generating modified BCG vaccines not only keep protection potency against Mb infection, but also satisfy the DIVA skin test for controlling animal tuberculosis in future.
Subunit vaccines
The deficiency of viral nucleic acid ensures the safety of subunit vaccines, which contain typical proteins isolated from pathogens. And the effect of this vaccine depends largely on the selected antigen proteins (128). Tuberculosis-related antigens are summarized in Table 2. For tuberculosis, the antigen Acr1 and TB10.4 of incubation period and active period were chosen respectively to construct the multi-stage double epitope vaccine L4.8 (129). By reducing the bacterial load, and increasing the number of CD4+ T cells and CD8+ T cells in mice, L4.8 exhibited superior protective effect than that of BCG vaccine. In addition, Kim et al. studied the InsB antigen, an ESAT-6-like antigen which belonged to the Mtb9.9 subfamily of the Esx family. The results showed that InsB might be an excellent vaccine antigen component, which was conducive to develop a multi-antigen Mtb subunit vaccine by producing Th1 biased memory T cells with multifunction ability. And its anti-tuberculosis protection was similar to that of ESAT-6. More importantly, the vaccine might have long-lasting protection against high virulence Mtb K (130).
Improvement of targeting performance
Accurate positioning is vital to prevent and control kinds of diseases including tuberculosis, which not only reduces medication dosage and side effect, but also enhances therapeutic effect and patient compliance. For a better prevention efficiency of subunit vaccines, bioactive ligands including Fc domain and APC surface molecule have been utilized to target tuberculosis immune system. Baghani et al. developed a subunit vaccine consisting of Mtb CFP-10 and the Fc domain of mouse IgG2a which could target APC. Further evaluation in mice showed that this vaccine induced a better Th1-type immune response than the control groups (BCG vaccine and PBS). It implies that subunit vaccines based on the fusion immunogens containing distinct functional domains are potential candidates with ability to target vital immune cells (131). In addition to antibodies, some APC surface molecules can also be utilized to realize targeting. Anneliese S. Tyne et al. used Mtb antigen cutinase-like protein (Culp) 1–6 and MPT83 directly combining with a new adjuvant Lipokel (Lipotek Pty Ltd.) to construct a tuberculosis subunit vaccine. Lipokel is a ligand for TLR2, which allows the vaccine to target TLR2-expressing immune cells (monocytes, macrophages, dendritic cells, and so on). Seven days after the mice were inoculated, the Culp1-6-Lipokel group had significantly increased lung neutrophils, myeloid DCs, recruited monocytes and alveolar macrophages compared with the simple Lipokel group. Both the Culp1-6-Lipokel group and the MPT83-Lipokel group showed an increase in the number of activated DCs, but only the MPT83-Lipokel group lasted until 28 days. It was encouraging that CD4+ T cells in the MPT83-Lipokel group increased significantly after 28 days of vaccination (133). Besides immunological activity, the size and connection pattern are noteworthy features of target components, which may affect the original immunogenicity of subunit vaccine. Moreover, there is rarely reported that common target molecules used for drug delivery are applied to improve the targeting performance of targeting performances. Considering various types of these target molecules, it may be a potential way to create more ideal subunit vaccines with superior anti-tuberculosis effects.
Assistance of adjuvants
Due to the relatively low immunogenicity of subunit vaccines, it is necessary to employ adjuvants for enhancing immune efficiency. And it has been proved that the immune potency was improved obviously by introducing appropriate adjuvants (87). At present, most of these adjuvants are involved in Th1-type reaction derived from CD4+ T cells, which plays an important role in anti-tuberculosis immunity.
The Ag85B and CysD antigen protein fusion mucosal vaccine used deltamethrin Advax as an adjuvant, and its performance by intratracheal administration was better than that by parenteral administration. The effect of Advax mainly depends on the CD4+ T cells residing in lungs which produce IL-17 and retinoid-related orphan nuclear receptor γt (RORγt) (91). Among them, RORγt is involved in the development of Th17 cells, which further support Th1 response and accelerate the clearance of pathogens (134, 135). In another study, Advax adjuvant was applied to develop an anthrax subunit vaccine with recombinant protective antigen. After the inoculation and challenge experiments within mice, the vaccine exhibited good protective efficacy against Bacillus anthracis 7702 strain with a relatively high safety (136).
Retinoic acid (RA), a metabolite of vitamin A, can regulate the differentiation of CD4+ T cells, and subsequently affect the homeostasis of dendritic cells and the homing of T cells and B cells (137–139). Mice immunized by subcutaneous injection of tuberculosis subunit vaccine (CAF01 + H56) in the presence of RA showed intensive mucosal H56 specific IgA response and ameliorative Ag-specific CD4+ T lymphocyte homing to lung (92). Hence, RA can be considered in the development of tuberculosis mucosal vaccine. Furthermore, Pro-Glu/Pro-Pro-Glu (PE/PEE) family proteins are significant in the pathogenesis of tuberculosis. Started with CD4+ T cells in the same way as RA, researchers fused ESAT-6 with the common CD4+ T cell epitope of PE/PEE family. After inoculation of mice, CD4+ T cells secreting IL-2 and IFN-γ were induced, and the protective effect on mice was strengthened (132). It manifests that the optimization of essential epitopes with satisfactory immunogenicity is a promising strategy for exploring alternatives of complicated antigens.
Except the adaptive immune response associated with CD4+ T cells, the innate immune response also contributes to protecting against tuberculosis infection. α-galactosylceramide not only stimulates T cells to produce antigen-specific IFN-γ-mediated Th1 immunity fighting against tuberculosis, but also activates natural killer cells in innate immunity (140, 141). After combined with α-galactosylceramide, the immune effect of Mtb antigens (Ag85B and ESAT-6) was raised by more than 50 times (93). Meanwhile, the efficiency of the Ag85B-ESAT6 fusion subunit vaccine was also increased through utilizing DC-activated antigen, Rv2299c, as an adjuvant (69, 142).
Nanoemulsion (NE) is a kind of oil in water emulsion, which can be prepared with antigens. It has a nice assistant effect when combined with influenza vaccine (143). What's more, NE together with Mtb specific antigen could elicit an obvious mucosal IL-17 T cell response (94). Additionally, CAF01 is a compound based on dimethyl octadecyl ammonium bromide and trehalose-6,60-dibenzoate, which promote antigen presentation to dendritic cells and activate dendritic cells (144). CAF01 can be made into powder by freeze-drying method, removing the cold-chain transportation restriction of vaccines. And the freeze-dried H56 tuberculosis subunit vaccine was equivalent to the unfreeze-dried vaccine in anti-tuberculosis, which induced antigen-specific Th1, Th17 and humoral immune responses as well (95). These freeze-dried vaccines reduce transportation cost and guarantee stability, which will be of great benefit to tuberculosis prevention and treatment in economically undeveloped area.
Utilization of immunoinformatic tools
As the derivative of big data and artificial intelligence (AI) technology, bioinformatics tools can also be utilized to screen effective antigen epitopes for fabricating potential vaccine candidates for tuberculosis (145). Owing to the important function of DNA binding proteins in the process of DNA replication, transcription, regulation and repair, Sunita et al. applied DNABIND tool to identify 1453 DNA binding proteins from the genes of Mtb for screening potential tuberculosis vaccines. Through ABCpred server, 18 DNA binding proteins were chosen to analyze the B-cell epitopes. And antigenic and non-allergenic B-cell epitopes were decided to predict the T-cell epitopes by ProPredI and ProPred server. Then, the evaluated T-cell epitopes (Rv1088, Rv3923c, Rv3235, Rv2871, Rv2731 and Rv0707) and DNA binding proteins were used for structural modeling to determine the location within the corresponding proteins. And the interaction of these epitope and human leukocyte antigens were finally detected to prove their effects, which implied their potential as vaccine candidates against tuberculosis (146). Similarly, enhanced intracellular survival proteins from Mtb were exploited to construct a chimeric vaccine by immunoinformatics approaches. The combined results of toxicity, allergenicity, antigenicity, MHC allele binding and IFN epitopes predicted 8 cytotoxic T lymphocyte epitopes, 6 helper T lymphocyte epitopes and a B cell epitope as suitable construction components. Further structure modification and 3D structure analysis of the chimeric vaccine were utilized to validate docking studies with Toll-like receptor 4. And their stable interaction was proved by molecular dynamic simulation, which provided a chimeric vaccine candidate for subsequent in vitro and in vivo test (147). The frequently-used bioinformatic tools and corresponding applications are listed in Table 3. The application of immunoinformatic tools, which provides numerous reliable combinations of different epitopes, facilitates the construction of tuberculosis vaccines. Meanwhile, it simplifies the preliminary screening process of the obtained outcomes without practical operation (7, 20, 21). Moreover, the completion of genome sequencing of Mtb, the development of computer technology, and the excellent safety of subunit vaccine may be the reasons why tuberculosis subunit vaccines have attracted more attention in recent years (148, 149). At the same time, further development and intersection of bioinformatics, genomics, proteomics and system biology has provided more opportunities for the evolution of subunit vaccines (150).
DNA vaccines
DNA vaccines are recombinant eukaryotic expression vectors encoding antigens. After administration, the exogenous gene is expressed in vivo. The resulting antigens activate the body's immune system, thus inducing specific humoral immunity and cellular immune response. According to this principle, the safety of DNA vaccine is excellent, which is similar to subunit vaccines. The key to both vaccines lies in the selection of antigen, but DNA vaccine focuses on antigen gene. For example, Mce1A gene may be involved in the invasion and survival of Mtb in human macrophages (151, 152). It is suggested that the selection of antigen genes should start from the pathogenesis of tuberculosis and block the pathogenic pathway of Mtb. Some genes employed by DNA vaccines against tuberculosis are shown in Table 4.
Considering that BCG vaccine could not eliminate latent Mtb infection (157), some DNA vaccines were designed complying with prime-boost strategy. C6 vaccine contains DNA sequence of six immunodominant CD4+ and CD8+ T cell epitopes of Mtb expressed in latent, acute and chronic infection stages, respectively. After inoculation of mice, the release of IFN-γ and TNF-α grew, and the activation of dendritic cells and macrophages increased. The bacterial load in lung and spleen of the C6 + BCG vaccine group mice was significantly reduced (153). Moreover, prime-boost strategy shows greater advantages compared with the DNA vaccine alone. Teimourpour et al. constructed the DNA plasmid vaccine encoding Mtb32C-HBHA fusion protein, which was verified by RT-PCR and Western blot in vitro. When the mice were inoculated with the immunization strategy promoted by DNA vaccine and primary immunization with BCG vaccine, the yield of IFN-γ was superior to that of the BCG group and the DNA vaccine group alone. In addition, a large amount of IL-12 and TGF-β were also induced successfully, which was sufficient to effectively stimulate the organism immune system (154).
Other than above prime-boost strategy, the application of immunoinformatics tools to exploit DNA vaccines can also be used as supplementary. Moradi et al. designed a candidate DNA vaccine consisting of three tuberculosis latency antigens Rv2029c, Rv2031c, and Rv2627c, along with microtubule-associated protein light chain 3 (LC3). It is expected that LC3 can increase the reaction of CD4+ T cells through autophagy. It has been reported that autophagy promotes the recognition and processing of MHC-2 molecules to antigens (158, 159). Moreover, there are 8 methods for predicting MHC class I-binding peptides: Artificial neural network (ANN); average relative binding (ARB); stabilized matrix method (SMM); stabilized matrix method with a peptide: MHC binding energy covariance matrix (SMMPMBEC); scoring matrices derived from combinatorial peptide libraries (Comblib-Sidney2008); Consensus; NetMHCpan and IEDB. After preliminary computational analysis, the vaccines can be used as candidate vaccines against tuberculosis, but it still needs animal experiments to prove its ability (155).
Although the construction technology of DNA vaccines is relatively mature and convenient, its immune potency needs further optimization. Peeridogaheh et al. constructed Ag85a-CFP10 fusion DNA vaccine, and the RT-PCR results illuminated the introduction of target mRNA in eukaryotic cells. Compared with the BCG group, vaccinated mice produced more IL-4, IFN-γ and TGF-β, while the level of IL-12 did not rise, which indicated that the vaccine cannot potentially induce cellular immunity (2).
Enhancement of immunogenicity
As a newly developed product, DNA vaccines possess some distinct advantages, such as no need to express and purify antigens in vitro, easy preparation and low cost. However, its immunogenicity and physiologic stability are slightly poor (160).
On one hand, some special gene sequences encoding substances that promote anti-tuberculosis immunity can be utilized as adjuvants of DNA vaccines by constructing them into a plasmid together with various antigen genes. For example, FMS-like tyrosine kinase 3 plays an important part in the proliferation and differentiation of myeloid and lymphoid progenitor cells and dendritic cells (DCs) (161). As a promising adjuvant, FMS-like tyrosine kinase 3 can enhance the recruitment and amplification of DC, and facilitate antigen uptake and cross-presentation (162, 163). Ganzhu Feng et al. developed a recombinant DNA vaccine based on nanoparticles, which contained three T cell epitopes of ESAT-6 and FMS-like tyrosine kinase 3 gene, and was encapsulated by chitosan (CS) nanoparticles. The results showed that the vaccine significantly enhanced T cell immunity and protected against Mtb H37Rv attack (22, 164).
On the other hand, some non-viral vector materials are used as adjuvants, which can protect plasmids from degradation, improve transfection efficiency and enhance the immunogenicity of DNA vaccines. Moreover, these materials (liposomes, polylactic acid-glycolic acid copolymer, polyethylene glycol, etc.) can be further modified to target specific immune cells (165, 166). Rosada et al. developed cationic liposome-based HSP65 DNA vaccines with construction either by encapsulation or adsorption, and compared the difference between intramuscular injection and intranasal administration. The study found both liposome preparations induced a typical Th1-type immune response pattern, but the intramuscular delivery pathway did not reduce the number of bacteria. However, a single intranasal immunization with HSP65 complexes carried plasmid DNA as low as 25 μg resulted in a significant quantity reduction of bacterium in lungs. These effects were accompanied by increasing IFN-γ level and preserving the lung parenchyma, which were similar to those found in mice given four intramuscular injections of naked DNA-HSP65 (167). Furthermore, the application of nanotechnology can improve the effectiveness of vaccines (168). Maytal Bivas-Benita et al. coated a DNA plasmid encoding eight HLA-A*0201 restricted T cell epitopes of Mtb with chitosan. By comparing with common intramuscular immune route, pulmonary administration of the DNA plasmid incorporating chitosan nanoparticles raised the secretion level of IFN-γ. And the immunogenicity was improved by the chitosan nanoparticles as well (97). It reveals the potential influence of vaccination route on vaccine efficacy. Considering the characteristics of different non-viral vectors, novel inoculation routes such as pulmonary, transdermal and nasal administration, are encouraged to take attempts in future vaccine design and clinical trials.
Application of viral vectors
Apart from non-viral vectors, viral vectors have also been studied for DNA vaccines. Okada et al. developed a DNA vaccine expressing tuberculosis antigen HSP65 and cytokine IL-12, which was encapsulated by Japanese hemagglutination virus (HVJ). The study found that the ability to induce IFN-γ and IL-12 is stronger when using 100 μg DNA, which was better than the PDD control group (156). Mangalakumari Jeyanathan et al. developed an adenovirus-based tuberculosis vaccine for respiratory inoculation, which provided a valuable reference of aerosol vaccine strategies. And it was also expected to be transformed into a vaccine against Corona Virus Disease 2019 (COVID-19) (169). However, viral vectors have some inherent disadvantages, for example, small encapsulation size and security threat caused by integration into host genome (170). In consideration of safety, non-viral vector candidates with modified immunity will be promising DNA vaccine adjuvants for large-scale clinical application in future.
Conclusions and perspectives
Tuberculosis is still one of the most important infectious diseases in human history because of its unique characteristics. As prevention can solve tuberculosis fundamentally, the development of vaccines is a very important job. Until now, there have been numerous candidate vaccines for anti-tuberculosis, but few of them have successfully applied to clinical practice. This situation warns people of the urgency to develop novel tuberculosis vaccines. Otherwise, the goal of reducing the incidence and mortality of tuberculosis by 90 and 95% respectively before 2035 may become hard to achieve (171).
Fortunately, there have been many studies concentrated on subunit vaccines against tuberculosis in recent years. As mentioned above, targeting and immunogenicity improvement have been achieved under laboratory conditions. Subunit vaccines have also appeared more frequently than other types of tuberculosis vaccines in clinical trials in the past decade, which indicates their potential for practical therapeutic application. The clinical trials of tuberculosis vaccines in recent years are shown in Table 5. Subunit vaccine and DNA vaccine are both new generation vaccines with several similarities, such as high safety, multiple antigen mechanism and relatively low immunogenicity. Introducing targeting and adjuvants for subunit vaccines are also applicable to DNA vaccines. Nevertheless, the implemented strategy is different between protein and plasmid. For example, Ali Asghar Baghani et al. fabricated the fusion protein of tuberculosis antigen CFP-10 and Fc domain of mouse IgG2a to achieve targeting. While the DNA vaccine can package CFP-10 encoding plasmid with a carrier, and then connect the Fc domain of mouse IgG2a with the carrier through covalent bond or electrostatic interaction to achieve targeting. What is noteworthy is that tuberculosis is a poverty-related disease, which reminds us to pay more attention to controlling the cost (172). From this point of view, DNA vaccine is more economical than subunit vaccine, which does not need protein purification, but directly immunizes with plasmids.
In addition, the evaluation results of some live attenuated vaccines seem to be superior to those of subunit vaccines and DNA vaccines. But the preparation of live attenuated vaccines mostly depends on the mutant strains of Mtb. Gene mutation has the characteristics of randomness, hazardous risk, and non-orientation. Although various gene editing techniques such as CRISPR/Cas9, zinc finger nucleases (ZFNs), transcription activator-like effector nucleases (TALENs), and homing endonucleases have been established (173), we cannot guarantee feasible site-directed mutations for vaccines. Thus, exploring reliable mutation methods is crucial to screen potential live attenuated vaccines.
Consequently, the summary of current research progress of different tuberculosis vaccines has provided valuable experiences and suggestions, through which tuberculosis will finally be conquered in the near future.
Author contributions
WQ: ideas and formulation or evolution of overarching research goals and aims, and revise the first draft. YG: preparation, creation and/or presentation of the published work, and specifically writing the initial draft (including substantive translation). YX: preparation, creation and/or presentation of the published work, and specifically revising the manuscript. JZ: preparation, creation and/or presentation of the published work, and specifically visualization/data presentation. ZW: oversight and leadership responsibility for the research activity planning and execution, including mentorship external to the core team. CD: performed the statistical analysis. YP: acquisition of the financial support for the project leading to this publication. All authors contributed to the article and approved the submitted version.
Funding
This work was supported by National Key Research and Development Program of China (2017YFD0501400).
Conflict of interest
The authors declare that the research was conducted in the absence of any commercial or financial relationships that could be construed as a potential conflict of interest.
Publisher's note
All claims expressed in this article are solely those of the authors and do not necessarily represent those of their affiliated organizations, or those of the publisher, the editors and the reviewers. Any product that may be evaluated in this article, or claim that may be made by its manufacturer, is not guaranteed or endorsed by the publisher.
References
1. Saqib M, Khatri R, Singh B, Gupta A, Kumar A, Bhaskar A, et al. Mycobacterium indicus pranii as a booster vaccine enhances BCG induced immunity and confers higher protection in animal models of tuberculosis. Tuberculosis (Edinb). (2016) 101:164–73. doi: 10.1016/j.tube.2016.10.002
2. Peerdogaheh H, Teimourpour R, Moradi B, Yousefipour M, Gholoobi A, Baghani A, et al. Evaluation of immune responses to a DNA vaccine encoding Ag85a-Cfp10 antigen of Mycobacterium tuberculosis in an animal model. Jundishapur J Microbiol. (2019) 12:e65689. doi: 10.5812/jjm.65689
3. Buddle BM, Parlane NA, Wedlock DN, Heiser A. Overview of vaccination trials for control of tuberculosis in cattle, wildlife and humans. Transbound Emerg Dis. (2013) 60:136–46. doi: 10.1111/tbed.12092
4. Vordermeier HM, Jones GJ, Buddle BM, Hewinson RG, Villarreal-Ramos B. Bovine tuberculosis in cattle: vaccines, DIVA tests, and host biomarker discovery. Annu Rev Anim Biosci. (2016) 4:87–109. doi: 10.1146/annurev-animal-021815-111311
5. World Health Organization. (2021). Available online at: https://www.who.int/publications/i/item/9789240037021 (accessed September 27, 2022).
6. Correa-Macedo W, Fava VM, Orlova M, Cassart P, Olivenstein R, Sanz J, et al. Alveolar macrophages from persons living with HIV show impaired epigenetic response to Mycobacterium tuberculosis. J Clin Invest. (2021) 22:e148013. doi: 10.1172/JCI148013
7. Schluger NW, Rom WN. The host immune response to tuberculosis. Am J Respir Crit Care Med. (1998) 3 Pt 1:679–91. doi: 10.1164/ajrccm.157.3.9708002
8. Olea-Popelka F, Muwonge A, Perera A, Dean AS, Mumford E, Erlacher-Vindel E, et al. Zoonotic tuberculosis in human beings caused by Mycobacterium bovis—a call for action. Lancet Infect Dis. (2017) 1:e21–5. doi: 10.1016/S1473-3099(16)30139-6
9. Grover N, Paskaleva EE, Mehta KK, Dordick JS, Kane RS. Growth inhibition of Mycobacterium smegmatis by mycobacteriophage-derived enzymes. Enzyme Microb Technol. (2014) 63:1–6. doi: 10.1016/j.enzmictec.2014.04.018
10. Swain SS, Sharma D, Hussain T, Pati S. Molecular mechanisms of underlying genetic factors and associated mutations for drug resistance in Mycobacterium tuberculosis. Emerg Microbes Infect. (2020) 1:1651–63. doi: 10.1080/22221751.2020.1785334
11. Salmanzadeh S, Karamian M, Alavi SM, Nashibi R. Evaluation of the frequency of resistance to 2 drugs (Isoniazid and Rifampin) by molecular investigation and it's risk factors in new cases of smear positive pulmonary tuberculosis in health centers under the cover of Jundishapur University of Medical Sciences in 2017. J Family Med Prim Care. (2020) 4:1958–62. doi: 10.4103/jfmpc.jfmpc_983_19
12. Dye C. Making wider use of the world's most widely used vaccine: Bacille Calmette-Guerin revaccination reconsidered. J R Soc Interface. (2013) 87:20130365. doi: 10.1098/rsif.2013.0365
13. Nathan Back IRC, Abel NS, Lajtha JD. The new paradigm of immunity to tuberculosis. Adv Exp Med Biol. (2013) 66:294. doi: 10.1007/978-1-4614-6111-1
14. Bates M, Marais BJ, Zumla A. Tuberculosis comorbidity with communicable and noncommunicable diseases. Cold Spring Harb Perspect Med. (2015) 11:a017889. doi: 10.1101/cshperspect.a017889
15. Philips JA, Ernst JD. Tuberculosis pathogenesis and immunity. Annu Rev Pathol. (2012) 7:353–84. doi: 10.1146/annurev-pathol-011811-132458
16. Ehrt S, Schnappinger D. Mycobacterial survival strategies in the phagosome: defence against host stresses. Cell Microbiol. (2009) 8:1170–8. doi: 10.1111/j.1462-5822.2009.01335.x
17. Schnappinger D, Ehrt S, Voskuil MI, Liu Y, Mangan JA, Monahan IM, et al. Transcriptional adaptation of Mycobacterium tuberculosis within macrophages: insights into the phagosomal environment. J Exp Med. (2003) 5:693–704. doi: 10.1084/jem.20030846
18. Ouimet M, Koster S, Sakowski E, Ramkhelawon B, van Solingen C, Oldebeken S, et al. Mycobacterium tuberculosis induces the miR-33 locus to reprogram autophagy and host lipid metabolism. Nat Immunol. (2016) 6:677–86. doi: 10.1038/ni.3434
19. Li Z, Kelley C, Collins F, Rouse D, Morris S. Expression of katG in Mycobacterium tuberculosis is associated with its growth and persistence in mice and guinea pigs. J Infect Dis. (1998) 4:1030–5. doi: 10.1086/515254
20. Cooper AM. Cell-mediated immune responses in tuberculosis. Annu Rev Immunol. (2009) 27:393–422. doi: 10.1146/annurev.immunol.021908.132703
21. Sia JK, Georgieva M, Rengarajan J. Innate immune defenses in human tuberculosis: an overview of the interactions between Mycobacterium tuberculosis and innate immune cells. J Immunol Res. (2015) 2015:747543. doi: 10.1155/2015/747543
22. Medley J, Goff A, Bettencourt PJG, Dare M, Cole L, Cantillon D, et al. Dissecting the Mycobacterium bovis BCG response to macrophage infection to help prioritize targets for anti-tuberculosis drug and vaccine discovery. Vaccines (Basel). (2022) 1. doi: 10.3390/vaccines10010113
23. Cooper AM, Solache A, Khader SA. Interleukin-12 and tuberculosis: an old story revisited. Curr Opin Immunol. (2007) 4:441–7. doi: 10.1016/j.coi.2007.07.004
24. Vignali DA, Kuchroo VK. IL-12 family cytokines: immunological playmakers. Nat Immunol. (2012) 8:722–8. doi: 10.1038/ni.2366
25. Buccheri S, Reljic R, Caccamo N, Ivanyi J, Singh M, Salerno A, et al. IL-4 depletion enhances host resistance and passive IgA protection against tuberculosis infection in BALB/c mice. Eur J Immunol. (2007) 3:729–37. doi: 10.1002/eji.200636764
26. Redford PS, Murray PJ, O'Garra A. The role of IL-10 in immune regulation during M. tuberculosis infection. Mucosal Immunol. (2011) 3:261–70. doi: 10.1038/mi.2011.7
27. Abdalla AE, Lambert N, Duan X, Xie J. Interleukin-10 family and tuberculosis: an old story renewed. Int J Biol Sci. (2016) 6:710–7. doi: 10.7150/ijbs.13881
28. Kuan R, Muskat K, Peters B, Lindestam Arlehamn CS. Is mapping the BCG vaccine-induced immune responses the key to improving the efficacy against tuberculosis? J Intern Med. (2020). 6:651–60. doi: 10.1111/joim.13191
29. Sud D, Bigbee C, Flynn JL, Kirschner DE. Contribution of CD8+ T cells to control of Mycobacterium tuberculosis infection. J Immunol. (2006) 7:4296–314. doi: 10.4049/jimmunol.176.7.4296
30. Orme IM. The Achilles heel of BCG. Tuberculosis (Edinb). (2010) 6:329–32. doi: 10.1016/j.tube.2010.06.002
31. Sterne JA, Rodrigues LC, Guedes IN. Does the efficacy of BCG decline with time since vaccination? Int J Tuberc Lung Dis. (1998). 2:200–7.
32. Dai G, Phalen S, McMurray DN. Nutritional modulation of host responses to mycobacteria. Front Biosci. (1998) 3:e110–122. doi: 10.2741/A371
33. Blanco FC, Garcia EA, Aagaard C. Subunit vaccine H65 + CAF01 increased the BCG-protection against Mycobacterium bovis infection in a mouse model of bovine tuberculosis. Res Vet Sci. (2021) 136:595–7. doi: 10.1016/j.rvsc.2021.04.014
34. Hwang SA, Wilk KM, Budnicka M, Olsen M, Bangale YA, Hunter RL, et al. Lactoferrin enhanced efficacy of the BCG vaccine to generate host protective responses against challenge with virulent Mycobacterium tuberculosis. Vaccine. (2007) 37–38:6730–43. doi: 10.1016/j.vaccine.2007.07.005
35. Wedlock DN, Denis M, Painter GF, Ainge GD, Vordermeier HM, Hewinson RG, et al. Enhanced protection against bovine tuberculosis after coadministration of Mycobacterium bovis BCG with a Mycobacterial protein vaccine-adjuvant combination but not after coadministration of adjuvant alone. Clin Vaccine Immunol. (2008) 5:765–72. doi: 10.1128/CVI.00034-08
36. Moliva JI, Hossfeld AP, Canan CH, Dwivedi V, Wewers MD, Beamer G, et al. Exposure to human alveolar lining fluid enhances Mycobacterium bovis BCG vaccine efficacy against Mycobacterium tuberculosis infection in a CD8(+) T-cell-dependent manner. Mucosal Immunol. (2018) 3:968–78. doi: 10.1038/mi.2017.80
37. Arcos J, Sasindran SJ, Fujiwara N, Turner J, Schlesinger LS, Torrelles JB. Human lung hydrolases delineate Mycobacterium tuberculosis-macrophage interactions and the capacity to control infection. J Immunol. (2011) 1:372–81. doi: 10.4049/jimmunol.1100823
38. Behr MA. BCG—different strains, different vaccines? Lancet Infect Dis. (2002) 2:86–92. doi: 10.1016/S1473-3099(02)00182-2
39. Ritz N, Hanekom WA, Robins-Browne R, Britton WJ, Curtis N. Influence of BCG vaccine strain on the immune response and protection against tuberculosis. FEMS Microbiol Rev. (2008) 5:821–41. doi: 10.1111/j.1574-6976.2008.00118.x
40. Castillo-Rodal AI, Castanon-Arreola M, Hernandez-Pando R, Calva JJ, Sada-Diaz E, Lopez-Vidal Y. Mycobacterium bovis BCG substrains confer different levels of protection against Mycobacterium tuberculosis infection in a BALB/c model of progressive pulmonary tuberculosis. Infect Immun. (2006) 3:1718–24. doi: 10.1128/IAI.74.3.1718-1724.2006
41. Chege GK, Williamson AL, Passmore JS, Bourn W, Ryffel B, Shephard EG. The immune response of the Chacma baboon to Bacille Calmette Guerin: development of a primate model for BCG-based vaccine research. Vaccine. (2005) 50:5783–91. doi: 10.1016/j.vaccine.2005.07.106
42. Lagranderie MR, Balazuc AM, Deriaud E, Leclerc CD, Gheorghiu M. Comparison of immune responses of mice immunized with five different Mycobacterium bovis BCG vaccine strains. Infect Immun. (1996) 1:1–9. doi: 10.1128/iai.64.1.1-9.1996
43. Comstock GW. Simple, practical ways to assess the protective efficacy of a new tuberculosis vaccine. Clin Infect Dis. (2000) 30:S250–253. doi: 10.1086/313870
44. Favorov M, Ali M, Tursunbayeva A, Aitmagambetova I, Kilgore P, Ismailov S, et al. Comparative tuberculosis (TB) prevention effectiveness in children of Bacillus Calmette-Guerin (BCG) vaccines from different sources, Kazakhstan. PLoS ONE. (2012) 3:e32567. doi: 10.1371/journal.pone.0032567
45. Mangtani P, Abubakar I, Ariti C, Beynon R, Pimpin L, Fine PE, et al. Protection by BCG vaccine against tuberculosis: a systematic review of randomized controlled trials. Clin Infect Dis. (2014) 4:470–80. doi: 10.1093/cid/cit790
46. da Costa AC, Nogueira SV, Kipnis A, Junqueira-Kipnis AP. Recombinant BCG: innovations on an old vaccine scope of BCG strains and strategies to improve long-lasting memory. Front Immunol. (2014) 5:152. doi: 10.3389/fimmu.2014.00152
47. Mangtani P, Nguipdop-Djomo P, Keogh RH, Trinder L, Smith PG, Fine PE, et al. Observational study to estimate the changes in the effectiveness of bacillus Calmette-Guerin (BCG) vaccination with time since vaccination for preventing tuberculosis in the UK. Health Technol Assess. (2017) 39:1–54. doi: 10.3310/hta21390
48. Rodrigues LC, Diwan VK, Wheeler JG. Protective effect of BCG against tuberculous meningitis and miliary tuberculosis: a meta-analysis. Int J Epidemiol. (1993) 6:1154–8. doi: 10.1093/ije/22.6.1154
49. Moliva JI, Turner J, Torrelles JB. Immune responses to Bacillus Calmette-Guerin vaccination: why do they fail to protect against Mycobacterium tuberculosis? Front Immunol. (2017) 8:407. doi: 10.3389/fimmu.2017.00407
50. Koster S, Klevorn T, Papavinasasundaram K, Sassetti CM, Portal-Celhay C, Philips JA. Consequence of enhanced LC3-trafficking for a live, attenuated M. tuberculosis vaccine. Vaccine. (2018) 7:939–44. doi: 10.1016/j.vaccine.2018.01.012
51. Chandran A, Williams K, Mendum T, Stewart G, Clark S, Zadi S, et al. Development of a diagnostic compatible BCG vaccine against Bovine tuberculosis. Sci Rep. (2019) 1:17791. doi: 10.1038/s41598-019-54108-y
52. Klevno NI, Aksenova VA. BCG tuberculosis vaccine: immunological and clinical efficacy in children born to HIV-infected women. BIOpreparations Prev Diagn Treat. (2018) 18:114–20. doi: 10.30895/2221-996X-2018-18-2-114-120
53. Gupta T, LaGatta M, Helms S, Pavlicek RL, Owino SO, Sakamoto K, et al. Evaluation of a temperature-restricted, mucosal tuberculosis vaccine in guinea pigs. Tuberculosis (Edinb). (2018) 113:179–88. doi: 10.1016/j.tube.2018.10.006
54. Ihsan Muttaqin M, Stephanie F, Saragih M, Friend Tambunan US. Epitope-based vaccine design for tuberculosis HIV infection through in silico approach. Pak J Biol Sci. (2021) 7:765–72. doi: 10.3923/pjbs.2021.765.772
55. Perdomo C, Zedler U, Kuhl AA, Lozza L, Saikali P, Sander LE, et al. Mucosal BCG vaccination induces protective lung-resident memory T cell populations against tuberculosis. MBio. (2016) 7:e01686–16. doi: 10.1128/mBio.01686-16
56. Garcia-Contreras L, Wong YL, Muttil P, Padilla D, Sadoff J, Derousse J, et al. Immunization by a bacterial aerosol. Proc Natl Acad Sci USA. (2008) 12:4656–60. doi: 10.1073/pnas.0800043105
57. Giri PK, Verma I, Khuller GK. Protective efficacy of intranasal vaccination with Mycobacterium bovis BCG against airway Mycobacterium tuberculosis challenge in mice. J Infect. (2006) 5:350–6. doi: 10.1016/j.jinf.2005.12.017
58. Aguilo N, Toledo AM, Lopez-Roman EM, Perez-Herran E, Gormley E, Rullas-Trincado J, et al. Pulmonary Mycobacterium bovis BCG vaccination confers dose-dependent superior protection compared to that of subcutaneous vaccination. Clin Vaccine Immunol. (2014) 4:594–7. doi: 10.1128/CVI.00700-13
59. Darrah PA, Zeppa JJ, Maiello P, Hackney JA, Wadsworth MH, Hughes TK, et al. Prevention of tuberculosis in macaques after intravenous BCG immunization. Nature. 7788:95–102. doi: 10.1038/s41586-019-1817-8
60. Sharpe S, White A, Sarfas C, Sibley L, Gleeson F, McIntyre A, et al. Alternative BCG delivery strategies improve protection against Mycobacterium tuberculosis in non-human primates: protection associated with mycobacterial antigen-specific CD4 effector memory T-cell populations. Tuberculosis (Edinb). (2016) 174–90. doi: 10.1016/j.tube.2016.09.004
61. Thom ML, McAulay M, Vordermeier HM, Clifford D, Hewinson RG, Villarreal-Ramos B, et al. Duration of immunity against Mycobacterium bovis following neonatal vaccination with bacillus Calmette-Guerin Danish: significant protection against infection at 12, but not 24, months. Clin Vaccine Immunol. (2012) 8:1254–60. doi: 10.1128/CVI.00301-12
62. Gormley E, Bhuachalla DNi, O'Keeffe J, Murphy D, Aldwell FE, Fitzsimons T, et al. Oral vaccination of free-living badgers (Meles meles) with Bacille Calmette Guerin (BCG) vaccine confers protection against tuberculosis. PLoS ONE. (2017) 1:e0168851. doi: 10.1371/journal.pone.0168851
63. Murphy D, Costello E, Aldwell FE, Lesellier S, Chambers MA, Fitzsimons T, et al. Oral vaccination of badgers (Meles meles) against tuberculosis: comparison of the protection generated by BCG vaccine strains Pasteur and Danish. Vet J. (2014) 3:362–7. doi: 10.1016/j.tvjl.2014.02.031
64. Vierboom MPM, Chenine AL, Darrah PA, Vervenne RAW, Boot C, Hofman SO, et al. Evaluation of heterologous prime-boost vaccination strategies using chimpanzee adenovirus and modified vaccinia virus for TB subunit vaccination in rhesus macaques. NPJ Vaccines. (2020) 1:39. doi: 10.1038/s41541-020-0189-2
65. Choi HG, Kwon KW, Choi S, Back YW, Park HS, Kang SM, et al. Antigen-specific IFN-gamma/IL-17-Co-producing CD4(+) T-cells are the determinants for protective efficacy of tuberculosis subunit vaccine. Vaccines (Basel). (2020) 8:300. doi: 10.3390/vaccines8020300
66. Wui SR, Kim HK, Han JE, Kim JM, Kim YH, Chun JH, et al. A combination of the TLR4 agonist CIA05 and alum promotes the immune responses to Bacillus anthracis protective antigen in mice. Int Immunopharmacol. (2011) 9:1195–204. doi: 10.1016/j.intimp.2011.03.020
67. Han JE, Kim HK, Park SA, Lee SJ, Kim HJ, Son GH, et al. A nontoxic derivative of lipopolysaccharide increases immune responses to Gardasil HPV vaccine in mice. Int Immunopharmacol. (2010) 2:169–76. doi: 10.1016/j.intimp.2009.10.012
68. Thada S, Horvath GL, Müller MM, Dittrich N, Conrad ML, Sur S, et al. Interaction of TLR4 and TLR8 in the innate immune response against Mycobacterium tuberculosis. Int J Mol Sci. (2021) 22:1560. doi: 10.3390/ijms22041560
69. Choi HG, Choi S, Back YW, Paik S, Park HS, Kim WS, et al. Rv2299c: a novel dendritic cell-activating antigen of Mycobacterium tuberculosis, fused-ESAT-6 subunit vaccine confers improved and durable protection against the hypervirulent strain HN878 in mice. Oncotarget. (2017) 12:19947–67. doi: 10.18632/oncotarget.15256
70. Mansury D, Ghazvini K, Amel Jamehdar S, Badiee A, Tafaghodi M, Nikpoor AR, et al. Enhancement of the effect of BCG vaccine against tuberculosis using DDA/TDB liposomes containing a fusion protein of HspX, PPE44, and EsxV. Artif Cells Nanomed Biotechnol. (2019) 1:370–7. doi: 10.1080/21691401.2018.1557674
71. Choi S, Choi HG, Back YW, Park HS, Lee KI, Gurmessa SK, et al. A dendritic cell-activating Rv1876 protein elicits Mycobacterium bovis BCG-prime effect via Th1-immune response. Biomolecules. (2021) 11:1306 doi: 10.3390/biom11091306
72. Kumar S, Bhaskar A, Patnaik G, Sharma C, Singh DK, Kaushik SR, et al. Intranasal immunization with peptide-based immunogenic complex enhances BCG vaccine efficacy in a murine model of tuberculosis. JCI Insight. (2021) 6:e145228. doi: 10.1172/jci.insight.145228
73. Komine-Aizawa S, Mizuno S, Matsuo K, Namiki T, Hayakawa S, Honda M. Recombinant BCG-prime and DNA-boost immunization confers mice with enhanced protection against Mycobacterium kansasii. Vaccines (Basel). (2021). 9:1260. doi: 10.3390/vaccines9111260
74. Romano M, D'Souza S, Adnet PY, Laali R, Jurion F, Palfliet K, et al. Priming but not boosting with plasmid DNA encoding mycolyl-transferase Ag85A from Mycobacterium tuberculosis increases the survival time of Mycobacterium bovis BCG vaccinated mice against low dose intravenous challenge with M. tuberculosis H37Rv. Vaccine. (2006) 24:3353–64. doi: 10.1016/j.vaccine.2005.12.066
75. Vatti A, Monsalve DM, Pacheco Y, Chang C, Anaya JM, Gershwin ME. Original antigenic sin: a comprehensive review. J Autoimmun. (2017) 83:12–21. doi: 10.1016/j.jaut.2017.04.008
76. Ivanyi J. Recall of antibody synthesis to the primary antigen following successive immunization with heterologous albumins. A two-cell theory of the original antigenic sin. Eur J Immunol. (1972) 2:354–9. doi: 10.1002/eji.1830020411
77. Singh PR, Vijjamarri AK, Sarkar D. Metabolic switching of Mycobacterium tuberculosis during hypoxia is controlled by the virulence regulator PhoP. J Bacteriol. (2020) 202:e00705-1. doi: 10.1128/JB.00705-19
78. Camacho LR, Ensergueix D, Perez E, Gicquel B, Guilhot C. Identification of a virulence gene cluster of Mycobacterium tuberculosis by signature-tagged transposon mutagenesis. Mol Microbiol. (1999) 2:257–67. doi: 10.1046/j.1365-2958.1999.01593.x
79. Clark S, Lanni F, Marinova D, Rayner E, Martin C, Williams A. Revaccination of guinea pigs with the live attenuated Mycobacterium tuberculosis vaccine MTBVAC improves BCG's protection against tuberculosis. J Infect Dis. (2017) 216:525–33. doi: 10.1093/infdis/jix030
80. Vasina DV, Kleymenov DA, Manuylov VA, Mazunina EP, Koptev EY, Tukhovskaya EA, et al. First-in-human trials of GamTBvac, a recombinant subunit tuberculosis vaccine candidate: safety and immunogenicity assessment. Vaccines (Basel). (2019) 7:166. doi: 10.3390/vaccines7040166
81. Bull NC, Stylianou E, Kaveh DA, Pinpathomrat N, Pasricha J, Harrington-Kandt R, et al. Enhanced protection conferred by mucosal BCG vaccination associates with presence of antigen-specific lung tissue-resident PD-1(+) KLRG1(-) CD4(+) T cells. Mucosal Immunol. (2019) 12:555–64. doi: 10.1038/s41385-018-0109-1
82. Kuczkowska K, Kleiveland CR, Minic R, Moen LF, Overland L, Tjaland R, et al. Immunogenic properties of Lactobacillus plantarum producing surface-displayed Mycobacterium tuberculosis antigens. Appl Environ Microbiol. (2017) 83:e02782-16. doi: 10.1128/AEM.02782-16
83. Kuczkowska K, Copland A, Øverland L, Mathiesen G, Tran AC, Paul MJ, et al. Inactivated Lactobacillus plantarum carrying a surface-displayed Ag85B-ESAT-6 fusion antigen as a booster vaccine against Mycobacterium tuberculosis infection. Front Immunol. (2019) 10:1588. doi: 10.3389/fimmu.2019.01588
84. Parlane NA, Shu D, Subharat S, Wedlock DN, Rehm BH, de Lisle GW, et al. Revaccination of cattle with bacille Calmette-Guerin two years after first vaccination when immunity has waned, boosted protection against challenge with Mycobacterium bovis. PLoS ONE. (2014) 9:e106519. doi: 10.1371/journal.pone.0106519
85. Dean G, Whelan A, Clifford D, Salguero FJ, Xing Z, Gilbert S, et al. Comparison of the immunogenicity and protection against bovine tuberculosis following immunization by BCG-priming and boosting with adenovirus or protein based vaccines. Vaccine. (2014) 32:1304–10. doi: 10.1016/j.vaccine.2013.11.045
86. Gortazar C, Beltran-Beck B, Garrido JM, Aranaz A, Sevilla IA, Boadella M, et al. Oral re-vaccination of Eurasian wild boar with Mycobacterium bovis BCG yields a strong protective response against challenge with a field strain. BMC Vet Res. (2014) 10:96. doi: 10.1186/1746-6148-10-96
87. Ashhurst AS, Parumasivam T, Chan JGY, Lin LCW, Florido M, West NP, et al. PLGA particulate subunit tuberculosis vaccines promote humoral and Th17 responses but do not enhance control of Mycobacterium tuberculosis infection. PLoS ONE. (2018) 13:e0194620. doi: 10.1371/journal.pone.0194620
88. Khan A, Bakhru P, Saikolappan S, Das K, Soudani E, Singh CR, et al. An autophagy-inducing and TLR-2 activating BCG vaccine induces a robust protection against tuberculosis in mice. NPJ Vaccines. (2019) 4:34. doi: 10.1038/s41541-019-0122-8
89. Khan A, Singh VK, Mishra A, Soudani E, Bakhru P, Singh CR, et al. NOD2/RIG-I activating inarigivir adjuvant enhances the efficacy of BCG vaccine against tuberculosis in mice. Front Immunol. (2020) 11:592333. doi: 10.3389/fimmu.2020.592333
90. Telesca C, Angelico M, Piccolo P, Nosotti L, Morrone A, Longhi C, et al. Interferon-alpha treatment of hepatitis D induces tuberculosis exacerbation in an immigrant. J Infect. (2007) 54:e223–226. doi: 10.1016/j.jinf.2006.12.009
91. Counoupas C, Ferrell KC, Ashhurst A, Bhattacharyya ND, Nagalingam G, Stewart EL, et al. Mucosal delivery of a multistage subunit vaccine promotes development of lung-resident memory T cells and affords interleukin-17-dependent protection against pulmonary tuberculosis. NPJ Vaccines. (2020) 5:105. doi: 10.1038/s41541-020-00255-7
92. Riccomi A, Piccaro G, Christensen D, Palma C, Andersen P, Vendetti S, et al. Parenteral vaccination with a tuberculosis subunit vaccine in presence of retinoic acid provides early but transient protection to M. tuberculosis. Infection Front Immunol. (2019) 10:934. doi: 10.3389/fimmu.2019.00934
93. Khan A, Singh S, Galvan G, Jagannath C, Sastry KJ. Prophylactic Sublingual immunization with Mycobacterium tuberculosis subunit vaccine incorporating the natural killer T cell agonist alpha-galactosylceramide enhances protective immunity to limit pulmonary and extra-pulmonary bacterial burden in mice. Vaccines (Basel). (2017) 5:47. doi: 10.3390/vaccines5040047
94. Ahmed M, Smith DM, Hamouda T, Rangel-Moreno J, Fattom A, Khader SA. A novel nanoemulsion vaccine induces mucosal interleukin-17 responses and confers protection upon Mycobacterium tuberculosis challenge in mice. Vaccine. (2017) 37:4983–9. doi: 10.1016/j.vaccine.2017.07.073
95. Thakur A, Ingvarsson PT, Schmidt ST, Rose F, Andersen P, Christensen D, et al. Immunological and physical evaluation of the multistage tuberculosis subunit vaccine candidate H56/CAF01 formulated as a spray-dried powder. Vaccine. (2018) 23:3331–9. doi: 10.1016/j.vaccine.2018.04.055
96. Feng GZ, Jiang QT, Xia M, Lu YL, Qiu W, Zhao D, et al. Enhanced immune response and protective effects of nano-chitosan-based DNA vaccine encoding T cell epitopes of Esat-6 and FL against Mycobacterium tuberculosis infection. PLoS One. (2013) 8:e61135. doi: 10.1371/journal.pone.0061135
97. Bivas-Benita M, van Meijgaarden KE, Franken KL, Junginger HE, Borchard G, Ottenhoff TH, et al. Pulmonary delivery of chitosan-DNA nanoparticles enhances the immunogenicity of a DNA vaccine encoding HLA-A*0201-restricted T-cell epitopes of Mycobacterium tuberculosis. Vaccine. (2004) 13–14:1609–15. doi: 10.1016/j.vaccine.2003.09.044
98. Kita Y, Hashimoto S, Nakajima T, Nakatani H, Nishimatsu S, Nishida Y, et al. Novel therapeutic vaccines [(HSP65+IL-12) DNA-, granulysin- and Ksp37-vaccine] against tuberculosis and synergistic effects in the combination with chemotherapy. Hum Vaccin Immunother. (2013) 9:526–33. doi: 10.4161/hv.23230
99. Strong EJ, Lee S. Targeting autophagy as a strategy for developing new vaccines and host-directed therapeutics against mycobacteria. Front Microbiol. (2020) 11:614313. doi: 10.3389/fmicb.2020.614313
100. Whelan AO, Villarreal-Ramos B, Vordermeier HM, Hogarth PJ. Development of an antibody to bovine IL-2 reveals multifunctional CD4 T(EM) cells in cattle naturally infected with bovine tuberculosis. PLoS ONE. (2011) 12:e29194. doi: 10.1371/journal.pone.0029194
101. Hu L, Gocke AR, Knapp E, Rosenzweig JM, Grishkan IV, Baxi EG, et al. Functional blockade of the voltage-gated potassium channel Kv1.3 mediates reversion of T effector to central memory lymphocytes through SMAD3/p21cip1 signaling. J Biol Chem. (2012) 287:1261–8. doi: 10.1074/jbc.M111.296798
102. Lewinsohn DM, Singh DK, Dwivedi VP, Singh SP, Kumari A, Sharma SK, et al. Luteolin-mediated Kv13 K+ channel inhibition augments BCG vaccine efficacy against tuberculosis by promoting central memory T cell responses in mice. PLoS Pathog. (2020) 16:e1008887. doi: 10.1371/journal.ppat.1008887
103. Vilcek J. Fifty years of interferon research: aiming at a moving target. Immunity. (2006) 25:343–8. doi: 10.1016/j.immuni.2006.08.008
104. Paquette RL, Hsu NC, Kiertscher SM, Park AN, Tran L, Roth MD, et al. Interferon-alpha and granulocyte-macrophage colony-stimulating factor differentiate peripheral blood monocytes into potent antigen-presenting cells. J Leukoc Biol. (1998) 64:358–67. doi: 10.1002/jlb.64.3.358
105. Hervas-Stubbs S, Perez-Gracia JL, Rouzaut A, Sanmamed MF, Le Bon A, et al. Direct effects of type I interferons on cells of the immune system. Clin Cancer Res. (2011) 17:2619–27. doi: 10.1158/1078-0432.CCR-10-1114
106. Thomas J, Risalde MA, Serrano M, Sevilla I, Geijo M, Ortiz JA, et al. The response of red deer to oral administration of heat-inactivated Mycobacterium bovis and challenge with a field strain. Vet Microbiol. (2017) 208:195–202. doi: 10.1016/j.vetmic.2017.08.007
107. Garrido JM, Sevilla IA, Beltran-Beck B, Minguijon E, Ballesteros C, Galindo RC, et al. Protection against tuberculosis in Eurasian wild boar vaccinated with heat-inactivated Mycobacterium bovis. PLoS ONE. (2011) 9:e24905. doi: 10.1371/journal.pone.0024905
108. Nol P, Palmer MV, Waters WR, Aldwell FE, Buddle BM, Triantis JM, et al. Efficacy of oral and parenteral routes of Mycobacterium bovis bacille Calmette-Guerin vaccination against experimental bovine tuberculosis in white-tailed deer (Odocoileus virginianus): a feasibility study. J Wildl Dis. (2008) 44:247–59. doi: 10.7589/0090-3558-44.2.247
109. Diez-Delgado I, Sevilla IA, Romero B, Tanner E, Barasona JA, White AR, et al. Impact of piglet oral vaccination against tuberculosis in endemic free-ranging wild boar populations. Prev Vet Med. (2018) 155:11–20. doi: 10.1016/j.prevetmed.2018.04.002
110. Diez-Delgado I, Rodriguez O, Boadella M, Garrido JM, Sevilla IA, Bezos J, et al. Parenteral vaccination with heat-inactivated Mycobacterium bovis reduces the prevalence of tuberculosis-compatible lesions in farmed wild boar. Transbound Emerg Dis. (2017) 155:e18–21. doi: 10.1111/tbed.12526
111. Arrieta-Villegas C, Peralvarez T, Vidal E, Puighibet Z, Moll X, Canturri A, et al. Efficacy of parenteral vaccination against tuberculosis with heat-inactivated Mycobacterium bovis in experimentally challenged goats. PLoS ONE. (2018) 13:e0196948. doi: 10.1371/journal.pone.0196948
112. Pérez de Val B, Villarreal-Ramos B, Nofrarías M, López-Soria S, Romera N, Singh M, et al. Goats primed with Mycobacterium bovis BCG and boosted with a recombinant adenovirus expressing Ag85A show enhanced protection against tuberculosis. Clin Vaccine Immunol. (2012) 19:1339–47. doi: 10.1128/CVI.00275-12
113. Vidal E, Arrieta-Villegas C, Grasa M, Mercader I, Domingo M, Pérez de Val B. Field evaluation of the efficacy of Mycobacterium bovis BCG vaccine against tuberculosis in goats. BMC Vet Res. (2017) 13:252. doi: 10.1186/s12917-017-1182-5
114. Balseiro A, Altuzarra R, Vidal E, Moll X, Espada Y, Sevilla IA, et al. Assessment of BCG and inactivated Mycobacterium bovis vaccines in an experimental tuberculosis infection model in sheep. PLoS ONE. (2017) 12:e0180546. doi: 10.1371/journal.pone.0180546
115. Balseiro A, Prieto JM, Alvarez V, Lesellier S, Dave D, Salguero FJ, et al. Protective effect of oral BCG and inactivated Mycobacterium bovis vaccines in European badgers (Meles meles) experimentally infected with M. bovis. Front Vet Sci. (2020) 7:41. doi: 10.3389/fvets.2020.00041
116. Blanco Vazquez C, Prieto M, Barral M, Juste RA, Lesellier S, Salguero FJ, et al. Local lung immune response to Mycobacterium bovis challenge after BCG and M. bovis heat-inactivated vaccination in European badger (Meles meles). Pathogens. (2020) 9:456. doi: 10.3390/pathogens9060456
117. Lopez V, Risalde MA, Contreras M, Mateos-Hernandez L, Vicente J, Gortazar C, et al. Heat-inactivated Mycobacterium bovis protects zebrafish against mycobacteriosis. J Fish Dis. (2018) 41:1515–28. doi: 10.1111/jfd.12847
118. Risalde MA, López V, Contreras M, Mateos-Hernández L, Gortázar C, de la Fuente J. Control of mycobacteriosis in zebrafish (Danio rerio) mucosally vaccinated with heat-inactivated Mycobacterium bovis. Vaccine (2018). 30:4447–53. doi: 10.1016/j.vaccine.2018.06.042
119. Levillain F, Kim H, Woong Kwon K, Clark S, Cia F, Malaga W, et al. Preclinical assessment of a new live attenuated Mycobacterium tuberculosis Beijing-based vaccine for tuberculosis. Vaccine. (2020) 38:1416–23. doi: 10.1016/j.vaccine.2019.11.085
120. Troudt J, Creissen E, Izzo L, Bielefeldt-Ohmann H, Casonato S, Manganelli R, et al. Mycobacterium tuberculosis sigE mutant ST28 used as a vaccine induces protective immunity in the guinea pig model. Tuberculosis. (2017) 38:1416–23. doi: 10.1016/j.tube.2017.07.009
121. Hernandez-Pando R, Shin SJ, Clark S, Casonato S, Becerril-Zambrano M, Kim H, et al. Construction and characterization of the Mycobacterium tuberculosis sigE fadD26 unmarked double mutant as a vaccine candidate. Infect Immun. (2019) 88:e00496-19. doi: 10.1128/IAI.00496-19
122. Tarancon R, Dominguez-Andres J, Uranga S, Ferreira AV, Groh LA, Domenech M, et al. New live attenuated tuberculosis vaccine MTBVAC induces trained immunity and confers protection against experimental lethal pneumonia. PLoS Pathog. (2020) 16:e1008404. doi: 10.1371/journal.ppat.1008404
123. Blanco FC, Soria M, Gravisaco MJ, Bianco MV, Meikle V, Garbaccio S, et al. Assessment of the immune responses induced in cattle after inoculation of a Mycobacterium bovis strain deleted in two mce2 genes. J Biomed Biotechnol. (2012) 2012:258353. doi: 10.1155/2012/258353
124. Blanco FC, Bianco MV, Garbaccio S, Meikle V, Gravisaco MJ, Montenegro V, et al. Mycobacterium bovis Deltamce2 double deletion mutant protects cattle against challenge with virulent M. bovis. Tuberculosis (Edinb). (2013) 93:363–72. doi: 10.1016/j.tube.2013.02.004
125. Forrellad MA, Klepp LI, Gioffré A, Sabio y Garcia J, Morbidoni HR, Santangelo MD, et al. Virulence factors of the Mycobacterium tuberculosis complex. Virulence. (2014) 4:3–66. doi: 10.4161/viru.22329
126. García E, Bianco MV, Gravisaco MJ, Rocha RV, Blanco FC, Bigi F. Evaluation of Mycobacterium bovis double knockout mce2-phoP as candidate vaccine against bovine tuberculosis. Tuberculosis (Edinb). (2015) 95:186–9. doi: 10.1016/j.tube.2015.01.001
127. Bianco MV, Clark S, Blanco FC, Garbaccio S, Garcia E, Cataldi AA, et al. Assessment of Mycobacterium bovis deleted in p27-p55 virulence operon as candidate vaccine against tuberculosis in animal models. Biomed Res Int. (2014) 2014:951978. doi: 10.1155/2014/951978
128. Stylianou E, Harrington-Kandt R, Beglov J, Bull N, Pinpathomrat N, Swarbrick GM, et al. Identification and evaluation of novel protective antigens for the development of a candidate tuberculosis subunit vaccine. Infect Immun. (2018) 86:e00014-18. doi: 10.1128/IAI.00014-18
129. Rai PK, Chodisetti SB, Maurya SK, Nadeem S, Zeng W, Janmeja AK, et al. A lipidated bi-epitope vaccine comprising of MHC-I and MHC-II binder peptides elicits protective CD4 T cell and CD8 T cell immunity against Mycobacterium tuberculosis. J Transl Med. (2018) 16:279. doi: 10.1186/s12967-018-1653-x
130. Kim WS, Kim H, Kwon KW, Cho SN, Shin SJ. Immunogenicity and vaccine potential of InsB, an ESAT-6-like antigen identified in the highly virulent Mycobacterium tuberculosis Beijing K Strain. Front Microbiol. (2019) 10:220. doi: 10.3389/fmicb.2019.00220
131. Baghani AA, Soleimanpour S, Farsiani H, Mosavat A, Yousefi M, Meshkat Z, et al. CFP10: mFcgamma2 as a novel tuberculosis vaccine candidate increases immune response in mouse. Iran J Basic Med Sci. (2017) 20:122–30. doi: 10.22038/ijbms.2017.8231
132. Choi SY, Kwon KW, Kim H, Choi HH, Shin SJ. Vaccine potential of ESAT-6 protein fused with consensus CD4(+) T-cell epitopes of PE/PPE proteins against highly pathogenic Mycobacterium tuberculosis strain HN878. Biochem Biophys Res Commun. (2018) 503:2195–201. doi: 10.1016/j.bbrc.2018.06.017
133. Tyne AS, Chan JG, Shanahan ER, Atmosukarto I, Chan HK, Britton WJ, et al. TLR2-targeted secreted proteins from Mycobacterium tuberculosis are protective as powdered pulmonary vaccines. Vaccine. (2013) 40:4322–9. doi: 10.1016/j.vaccine.2013.07.022
134. Mickael ME, Bhaumik SR. Retinoid-related orphan receptor RORgammat in CD4(+) T-cell-mediated intestinal homeostasis and inflammation. Am J Pathol. (2020) 190:1984–99. doi: 10.1016/j.ajpath.2020.07.010
135. Bystrom J, Taher TE, Muhyaddin MS, Clanchy FI, Mangat P, Jawad AS, et al. Harnessing the therapeutic potential of Th17 Cells. Mediators Inflamm. (2015) 2015:205156. doi: 10.1155/2015/205156
136. Feinen B, Petrovsky N, Verma A, Merkel TJ. Advax-adjuvanted recombinant protective antigen provides protection against inhalational anthrax that is further enhanced by addition of murabutide adjuvant. Clin Vaccine Immunol. (2014) 21:580–6. doi: 10.1128/CVI.00019-14
137. Gajardo T, Pérez F, Terraza C, Campos-Mora M, Noelle RJ, Pino-Lagos K. IL-33 enhances retinoic acid signaling on CD4+ T cells. Cytokine. (2016) 85:120–2. doi: 10.1016/j.cyto.2016.06.016
138. Roe MM, Hashimi M, Swain S, Woo KM, Bimczok D. p38 MAPK signaling mediates retinoic acid-induced CD103 expression in human dendritic cells. Immunology. (2020) 161:230–44. doi: 10.1111/imm.13246
139. Hammerschmidt SI, Friedrichsen M, Boelter J, Lyszkiewicz M, Kremmer E, Pabst O, et al. Retinoic acid induces homing of protective T and B cells to the gut after subcutaneous immunization in mice. J Clin Invest. (2011) 121:3051–61. doi: 10.1172/JCI44262
140. Sada-Ovalle I, Skold M, Tian T, Besra GS, Behar SM. Alpha-galactosylceramide as a therapeutic agent for pulmonary Mycobacterium tuberculosis infection. Am J Respir Crit Care Med. (2010) 182:841–7. doi: 10.1164/rccm.200912-1921OC
141. Chamoto K, Takeshima T, Kosaka A, Tsuji T, Matsuzaki J, Togashi Y, et al. NKT cells act as regulatory cells rather than killer cells during activation of NK cell-mediated cytotoxicity by alpha-galactosylceramide in vivo. Immunol Lett. (2004) 95:5–11. doi: 10.1016/j.imlet.2004.04.012
142. Back YW, Bae HS, Choi HG, Binh DT, Son YJ, Choi S, et al. Fusion of dendritic cells activating Rv2299c protein enhances the protective immunity of Ag85B-ESAT6 vaccine candidate against tuberculosis. Pathogens. (2020) 9:865. doi: 10.3390/pathogens9110865
143. Stanberry LR, Simon JK, Johnson C, Robinson PL, Morry J, Flack MR, et al. Safety and immunogenicity of a novel nanoemulsion mucosal adjuvant W805EC combined with approved seasonal influenza antigens. Vaccine. (2012) 30:307–16. doi: 10.1016/j.vaccine.2011.10.094
144. Desel C, Werninghaus K, Ritter M, Jozefowski K, Wenzel J, Russkamp N, et al. The Mincle-activating adjuvant TDB induces MyD88-dependent Th1 and Th17 responses through IL-1R signaling. PLoS ONE. (2013) 8:e53531. doi: 10.1371/journal.pone.0053531
145. Ortega-Tirado D, Arvizu-Flores AA, Velazquez C, Garibay-Escobar A. The role of immunoinformatics in the development of T-cell peptide-based vaccines against Mycobacterium tuberculosis. Expert Rev Vaccines. (2020) 19:831–41. doi: 10.1080/14760584.2020.1825950
146. Singhvi N, Singh Y, Shukla P. Computational approaches in epitope design using DNA binding proteins as vaccine candidate in Mycobacterium tuberculosis. Infect Genet Evol. (2020) 83:104357. doi: 10.1016/j.meegid.2020.104357
147. Logesh R, Lavanya V, Jamal S, Ahmed N. Designing of a Chimeric vaccine using EIS (Rv2416c) protein against Mycobacterium tuberculosis H37Rv: an immunoinformatics approach. Appl Biochem Biotechnol. (2021) 194:187–214. doi: 10.1007/s12010-021-03760-0
148. Shah P, Mistry J, Reche PA, Gatherer D, Flower DR. In silico design of Mycobacterium tuberculosis epitope ensemble vaccines. Mol Immunol. (2018) 97:56–62. doi: 10.1016/j.molimm.2018.03.007
149. Andersen P, Doherty TM. TB subunit vaccines—putting the pieces together. Microbes Infect. (2005) 5–6:911–21. doi: 10.1016/j.micinf.2005.03.013
150. Sette A, Rappuoli R. Reverse vaccinology: developing vaccines in the era of genomics. Immunity. (2010) 33:530–41. doi: 10.1016/j.immuni.2010.09.017
151. Indriarini D, Rukmana A, Yasmon A. Cloning and expression of Mce1a gene from Mycobacterium tuberculosis Beijing and H37rv strain for vaccine candidate development. Afr J Infect Dis. (2018) 12(1 Suppl):127–132. doi: 10.21010/ajid.v12i1S.19
152. Srivastava V, Rouanet C, Srivastava R, Ramalingam B, Locht C, Srivastava BS, et al. Macrophage-specific Mycobacterium tuberculosis genes: identification by green fluorescent protein and kanamycin resistance selection. Microbiology (Reading). (2007) 153:659–66. doi: 10.1099/mic.0.2006/000547-0
153. Maurya SK, Aqdas M, Das DK, Singh S, Nadeem S, Kaur G, et al. A multiple T cell epitope comprising DNA vaccine boosts the protective efficacy of Bacillus Calmette-Guerin (BCG) against Mycobacterium tuberculosis. BMC Infect Dis. (2020) 20:677. doi: 10.1186/s12879-020-05372-1
154. Teimourpour R, Teimourpour A, Arzanlou M, Meshkat Z. A study on the immune response induced by a DNA vaccine encoding Mtb32C-HBHA antigen of Mycobacterium tuberculosis. Iran J Basic Med Sci. (2017) 20:1119–24. doi: 10.22038/ijbms.2017.9445
155. Moradi J, Tabrizi M, Izad M, Mosavari N, Feizabadi MM. Designing a novel multi-epitope DNA-based vaccine against tuberculosis: in silico approach. Jundishapur J Microbiol. (2017) 10:e43950. doi: 10.5812/jjm.43950
156. Okada M, Kita Y, Hashimoto S, Nakatani H, Nishimastu S, Kioka Y, et al. Preclinical study and clinical trial of a novel therapeutic vaccine against multi-drug resistant tuberculosis. Hum Vaccin Immunother. (2017) 13:298–305. doi: 10.1080/21645515.2017.1264781
157. Lin PL, Dietrich J, Tan E, Abalos RM, Burgos J, Bigbee C, et al. The multistage vaccine H56 boosts the effects of BCG to protect cynomolgus macaques against active tuberculosis and reactivation of latent Mycobacterium tuberculosis infection. J Clin Invest. (2012) 122:303–14. doi: 10.1172/JCI46252
158. Gannage M, Munz C. Autophagy in MHC class II presentation of endogenous antigens. Curr Top Microbiol Immunol. (2009) 335:123–40. doi: 10.1007/978-3-642-00302-8_6
159. Munz C. Antigen processing via autophagy—not only for MHC class II presentation anymore? Curr Opin Immunol. (2010) 22:89–93. doi: 10.1016/j.coi.2010.01.016
160. Eusebio D, Neves AR, Costa D, Biswas S, Alves G, Cui Z, et al. Methods to improve the immunogenicity of plasmid DNA vaccines. Drug Discov Today. (2021) 11:2575–92. doi: 10.1016/j.drudis.2021.06.008
161. Toliver-Kinsky TE, Lin CY, Herndon DN, Sherwood ER. Stimulation of hematopoiesis by the Fms-like tyrosine kinase 3 ligand restores bacterial induction of Th1 cytokines in thermally injured mice. Infect Immun. (2003) 71:3058–67. doi: 10.1128/IAI.71.6.3058-3067.2003
162. Zhou Q, Wang F, Yang F, Wang Y, Zhang X, Sun S, et al. Augmented humoral and cellular immune response of hepatitis B virus DNA vaccine by micro-needle vaccination using Flt3L as an adjuvant. Vaccine. (2010) 28:1357–62. doi: 10.1016/j.vaccine.2009.11.006
163. Xu J, Xu W, Chen X, Zhao D, Wang Y. Recombinant DNA vaccine of the early secreted antigen ESAT-6 by Mycobacterium tuberculosis and Flt3 ligand enhanced the cell-mediated immunity in mice. Vaccine. (2008) 26:4519–25. doi: 10.1016/j.vaccine.2008.06.044
164. Nagpal PS, Kesarwani A, Sahu P, Upadhyay P. Aerosol immunization by alginate coated mycobacterium (BCG/MIP) particles provide enhanced immune response and protective efficacy than aerosol of plain mycobacterium against M. tb H37Rv infection in mice. BMC Infect Dis. (2019) 19:568. doi: 10.1186/s12879-019-4157-2
165. Lima KM, Santos SA, Lima VM, Coelho-Castelo AA, Rodrigues JM Jr, Silva CL, et al. Single dose of a vaccine based on DNA encoding mycobacterial hsp65 protein plus TDM-loaded PLGA microspheres protects mice against a virulent strain of Mycobacterium tuberculosis. Gene Ther. (2003) 10:678–85. doi: 10.1038/sj.gt.3301908
166. Park SH, Lee SR, Hyun BH, Kim BM, Sung YC. Codelivery of PEG-IFN-alpha inhibits HCV DNA vaccine-induced T cell responses but not humoral responses in African green monkeys. Vaccine. (2008) 32:3978–83. doi: 10.1016/j.vaccine.2008.05.017
167. Rosada RS, Torre LG, Frantz FG, Trombone AP, Zárate-Bladés CR, Fonseca DM, et al. Protection against tuberculosis by a single intranasal administration of DNA-hsp65 vaccine complexed with cationic liposomes. BMC Immunol. (2008) 9:1–13. doi: 10.1186/1471-2172-9-38
168. Jazayeri SD, Lim HX, Shameli K, Yeap SK, Poh CL. Nano and microparticles as potential oral vaccine carriers and adjuvants against infectious diseases. Front Pharmacol. (2021) 2021:682286. doi: 10.3389/fphar.2021.682286
169. Jeyanathan M, Fritz DK, Afkhami S, Aguirre E, Howie KJ, Zganiacz A, et al. Aerosol delivery, but not intramuscular injection, of adenovirus-vectored tuberculosis vaccine induces respiratory-mucosal immunity in humans. JCI Insight. (2022) 2022:155655. doi: 10.1172/jci.insight.155655
170. Chen YH, Keiser MS, Davidson BL. Viral vectors for gene transfer. Curr Protoc Mouse Biol. (2018) 8:e58. doi: 10.1002/cpmo.58
171. Fatima S, Kumari A, Das G, Dwivedi VP. Tuberculosis vaccine: a journey from BCG to present. Life Sci. (2020) 2020:117594. doi: 10.1016/j.lfs.2020.117594
172. Kaufmann SH, Weiner J, von Reyn CF. Novel approaches to tuberculosis vaccine development. Int J Infect Dis. (2017) 56:263–7. doi: 10.1016/j.ijid.2016.10.018
Keywords: tuberculosis, immunization, vaccine, construction strategy, bioinformatics tools
Citation: Qu W, Guo Y, Xu Y, Zhang J, Wang Z, Ding C and Pan Y (2022) Advance in strategies to build efficient vaccines against tuberculosis. Front. Vet. Sci. 9:955204. doi: 10.3389/fvets.2022.955204
Received: 28 May 2022; Accepted: 04 November 2022;
Published: 24 November 2022.
Edited by:
Arturo Anadón, Complutense University of Madrid, SpainReviewed by:
Guiyan Yang, University of California, Davis, United StatesFábio Muniz de Oliveira, Indiana University School of Medicine, United States
Angelo Izzo, Royal Prince Alfred Hospital, Australia
Ved Prakash Dwivedi, International Centre for Genetic Engineering and Biotechnology, India
Copyright © 2022 Qu, Guo, Xu, Zhang, Wang, Ding and Pan. This is an open-access article distributed under the terms of the Creative Commons Attribution License (CC BY). The use, distribution or reproduction in other forums is permitted, provided the original author(s) and the copyright owner(s) are credited and that the original publication in this journal is cited, in accordance with accepted academic practice. No use, distribution or reproduction is permitted which does not comply with these terms.
*Correspondence: Yuanhu Pan, cGFueXVhbmh1JiN4MDAwNDA7bWFpbC5oemF1LmVkdS5jbg==
†These authors have contributed equally to this work