- 1College of Animal Science, Anhui Science and Technology University, Fengyang, China
- 2College of Animal Science, Guizhou University, Guiyang, China
- 3Biological Applications Department, Nuclear Research Center, Egyptian Atomic Energy Authority, Abu-Zaabal, Egypt
- 4Center of Reproductive Medicine, The First Affiliated Hospital of Bengbu Medical College, Bengbu, China
Lysoforte (LFT) plays a vital role in maintaining broilers' health and intestinal morphology. However, the mechanism behind the effects of LFT improving intestinal morphology and health is still unclear. Therefore, this study was implemented to explore the central genes linked to the regulatory effect of LFT. Seventy-five newly hatched Cobb 500 male broilers were randomly divided into three groups: control, LFT500, and LFT1000 groups, with 25 chicks per group. The control chicks were provided with the basal diet, and the birds in LFT500 and LFT1000 groups were offered the same basal diet with 500 g/ton and 1,000 g/ton LFT, respectively. GSE94622 dataset consisted of the control and two LFT-treated groups (LFT500 and LFT1000). Jejuna samples were obtained from Gene Expression Omnibus (GEO). Totally 106–344 DEGs were obtained by comparing LFT500 and LFT1000 vs. control and LFT1000 vs. LFT500. Gene ontology (GO) enrichment suggested that the DEGs are mainly related to the phosphatidylethanolamine biosynthetic process and neuron projection extension. KEGG analysis suggested the DEGs were enriched in AGE-RAGE, fatty acid elongation, ECM-receptor interaction (ECMRI), glycerophospholipid metabolism, focal adhesion, unsaturated fatty acids biosynthesis, and ABC transporters. Moreover, 29 genes, such as REG4, GJB1, KAT2A, APOA5, SERPINE2, ELOVL1, ABCC2, ANKRD9, CYP4V2, and PISD, might be closely related to promoting jejuna morphology in broilers. Taken together, our observation enhances the understanding of LFT in maintaining intestinal architecture and the general health of broiler chickens.
Introduction
Fats and oils, which are the most important dietary sources of energy, are excellent ways to accumulate the energy requirements for the optimized weight gain of broiler chickens (1, 2). Exogenous emulsifiers played an effective role in improving lipids utilization in broiler chickens, as the latter fail to gain lipids due to their poor emulsification in the gut (3). Lysoforte (LFT), a lysolecithin produced from lecithin that acts as an efficient emulsifier, could improve poultry growth, reproduction, and carcass quality by enhancing nutrient digestion and absorption, as well as reducing their mortality. In broiler chickens, LFT supplementation elevated average daily gain (ADG), final body weight, relative growth rate, dressing percent, carcass quality, net profit, total return, economic efficiency, and reduced energy matrix value, FCR, and mortality rate (4, 5). Previous research has shown that LFT supplementation increased saturated fatty acids absorption. In addition, a synergistic effect has existed between LFT and enzymes in broilers (6). Papadopoulos et al. (7) reported that fat digestibility, digesta viscosity, and apparent metabolizable energy in chicken were improved by LFT supplementation.
LFT may also have a vital role in maintaining intestinal morphology and health in broilers. LFT supplementation decreased the mucosal thickness at 28 day and induced alterations in the duodenum morphology (7). LFT addition significantly increased the average villus length and width (8). LFT addition had the potential to improve the chicken jejunal morphology and health due to the changes inducted by LFT in the intestinal epithelium (8). However, the mechanism by which LFT improves intestinal morphology and health in broilers is unclear. Therefore, we obtained the microarray data of broiler jejuna treated with or without LFT from the GEO dataset (https://www.ncbi.nlm.nih.gov/geo/) and identified differentially expressed genes (DEGs) in birds' jejuna, aiming to explore the mechanism behind the regulation of LFT on the jejuna morphology and health in broilers.
Materials and Methods
Ethics Statement
The present study was approved by the protocol from Anhui Science and Technology University (Bengbu, China) Institutional Animal Care and Use Committee (ECASTU-2015-P08).
Animals, Feed, and Tissue Collection
Seventy-five newly hatched Cobb 500 male broilers were randomly divided into three groups: control, LFT500, and LFT1000 groups, with 25 chicks per group. The control chicks were provided with the basal diet, and the birds in LFT500 and LFT1000 groups were offered the same basal diet with 500 and 1,000 g/ton LFT, respectively (8). The study lasted for 4 weeks. Ingredients and nutrient contents of the basal diet are presented in Supplementary Table 1. All birds were placed in the room with adjoining floor pens and weighed individually per week (8). On test day 10, five chicks per group were randomly chosen and killed via cervical dislocation. Pieces of ~10 cm in length were collected from the middle of the jejuna (8).
RNA Extraction and Microarray Analysis
Given the LFT effects on chicken jejunal morphology (Supplementary Table 2), ~50 mg of jejuna mucosa was homogenized with Tri Reagent (8). Total RNA was extracted using Directzol RNA columns, and the quality, purity, and integrity of RNA were assessed (8). Microarray analysis was performed with the chicken genome 1.0 array (8). The jejuna gene expression data were deposited in GEO (accession number: GSE94622) (8).
Microarray Data
GSE94622 consisted of the control (n = 5; GSM2479490, GSM2479491, GSM2479506, GSM2479507, and GSM2479523), LFT500-treated (n = 5; GSM2479533, GSM2479517, GSM2479516, GSM2479501, and GSM2479500), and LFT1000-treated (n = 5; GSM2479503, GSM2479520, GSM2479521, GSM2479537, and GSM2479536) broiler jejuna samples obtained at the 10th day of the experiment.
Data Processing
To obtain the DEGs between the jejuna samples treated with and without LFT, GEO2R (http://www.ncbi.nlm.nih.gov/geo/geo2r) was used to analyze the data from GSE94622. DEGs were identified as the genes with |log2-fold change (FC)| > 1 and P < 0.05.
Analysis of KEGG and Genetic Ontology for DEGs
KOBAS 3.0 (http://kobas.cbi.pku.edu.cn/kobas3/genelist/) was used to analyze the signaling pathways for DEGs. Regarding the genetic ontology (GO) analysis, DEGs were analyzed with DAVID (https://david.ncifcrf.gov/).
Protein Classification and Reactome Analysis for DEGs
Protein classification and Reactome analysis for DEGs were performed with the PANTHER (http://pantherdb.org/) and KOBAS 3.0.
Protein–Protein Interaction
STRING (https://string-db.org/) was employed to form protein–protein interaction (PPI). Cytoscape (version 3.8.0, http://www.cytoscape.org/) was used for further visualization.
Hub Genes and Their Functions
CytoHubba (http://apps.cytoscape.org/apps/cytohubba) was used to reveal hub genes from the PPI network, then the hub gene functions were summarized using GeneCards (https://www.genecards.org/), previous reports, and NCBI (https://www.ncbi.nlm.nih.gov/).
Results
The Outline of Transcripts and Genes in Broilers Jejuna
A total of 38,535 transcripts and 14,086 genes were observed in the chicken jejuna. Transcripts expression density and UMAP are indicated in Figures 1A,B. Figures 1C–E represents the volcano plots for DEGs in the three comparisons of LFT500 and LFT1000 vs. control and LFT1000 vs. LFT500, respectively. The jejuna diagram for DEGs in the three comparisons mentioned above is shown in Figure 1F. As shown in Supplementary Table 3, a total of 174–547 differentially expressed transcripts (DETs) and 106–344 DEGs were identified by the three comparisons. Compared with the control jejuna, 311 transcripts and 224 genes were upregulated, while 236 transcripts and 120 genes were downregulated in LFT1000-treated jejuna (Additional Files 1, 2); 98 transcripts and 68 genes were upregulated, while 76 transcripts and 38 genes were downregulated in LFT500-treated jejuna (Additional Files 3, 4). The top 20 genes up- and downregulated in the three comparisons (LFT500 and LFT1000 vs. control and LFT1000 vs. LFT500) were revealed in Supplementary Tables 4–9.
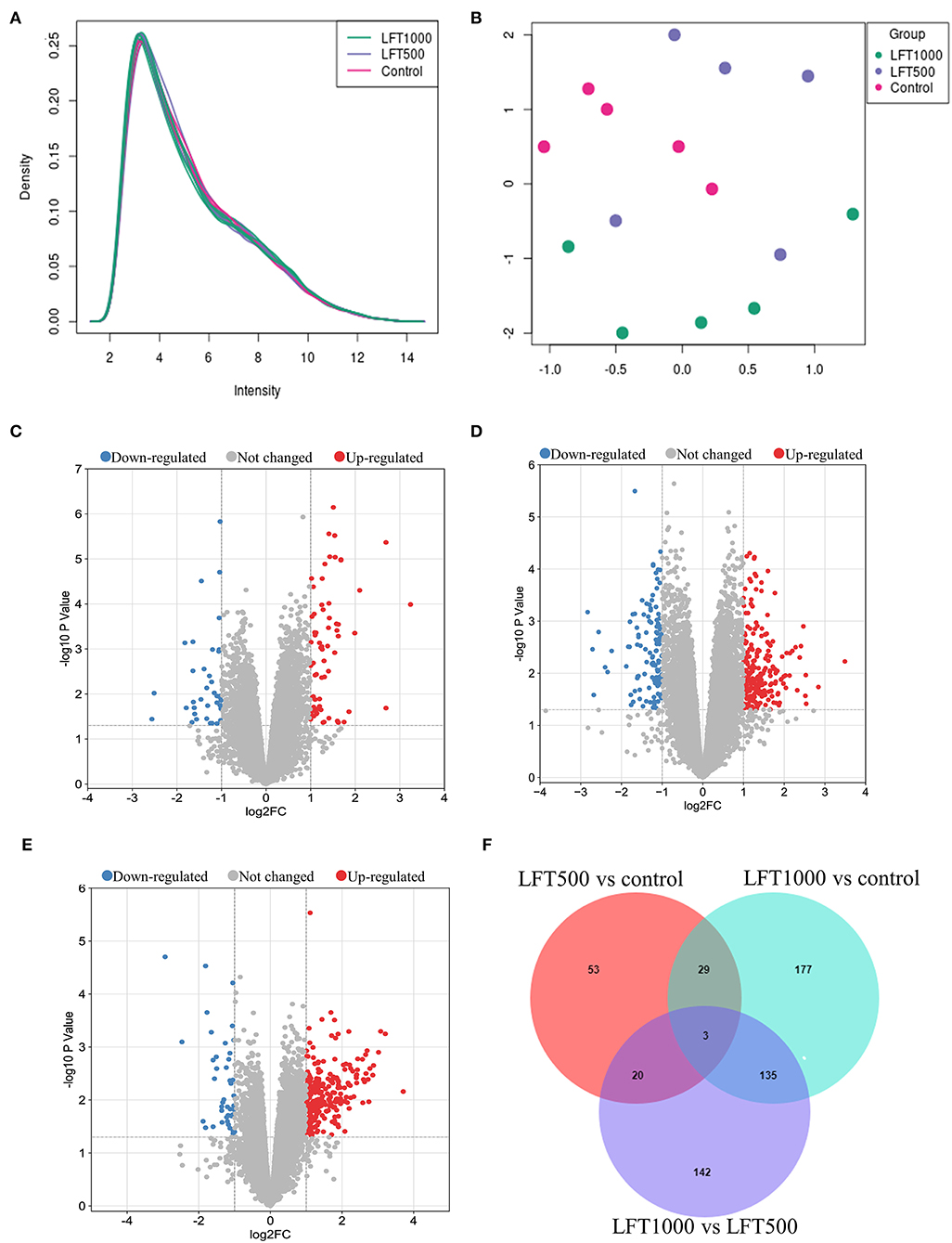
Figure 1. Transcript and Gene Profiles in Broilers Jejuna Treated with and without LFT. (A) The density of transcripts expression; (B) UMAP; (C–E) Volcano plot of DEGs was identified by three comparisons (LFT500 and LFT1000 vs. control and LFT1000 vs. LFT500, respectively). The red, gray, and blue spots represent the upregulated, unchanged, and downregulated genes. (F) Venn diagrams for the DEGs are identified in the three ways of comparisons mentioned above.
Twenty-nine common DEGs from the two comparisons (LFT1000 and LFT500 vs. control) are shown in Supplementary Table 10. These genes (including REG4, GJB1, KAT2A, APOA5, SERPINE2, ELOVL1, ABCC2, ANKRD9, CYP4V2, PISD, PTGR1, and AKAP9) might be closely related to promoting the jejuna morphology and health in broilers.
GO Analysis for DEGs
To reveal the biological processes associated with LFT regulation on broiler jejuna, GO analysis of DEGs in the three comparisons, including LFT1000 and LFT500 vs. control, and LFT1000 vs. LFT500 was illustrated in Figures 2A–C and Additional Files 5–7, respectively. DEGs obtained by comparing LFT1000 vs. control may participate in multiple biological processes, such as angiogenesis, blood coagulation, macrophage chemotaxis, glutathione metabolic process, collagen biosynthetic process, extracellular matrix (ECM) organization, the response to oxidative stress, and cell adhesion, proliferation, and differentiation.
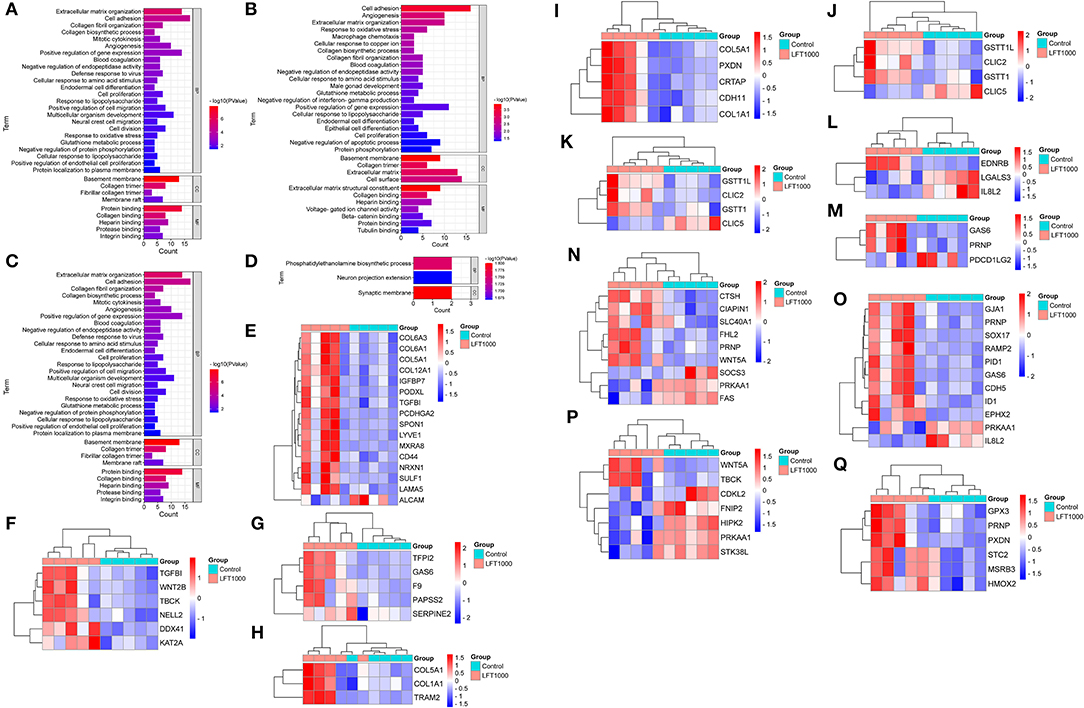
Figure 2. GO Enrichment for DEGs in Broilers Jejuna Treated with and without LFT. (A–C) GO enrichment for DEGs identified in the three comparisons (LFT500 and LFT1000 vs. control and LFT1000 vs. LFT500, respectively). (D) GO enrichment for the common DEGs in the two comparisons (LFT500 and LFT1000 vs. control). (E–Q) The heatmaps for DEGs in cell adhesion, cell proliferation, blood coagulation, collagen biosynthetic process, collagen fibril organization, glutathione metabolic process, macrophage chemotaxis, negative regulation of interferon-gamma production, positive regulation of gene expression, protein phosphorylation, and the response to oxidative stress.
DEGs between LFT1000 and the control may have a vital role in protein phosphorylation; leukocyte activation; adaptive immune response; antibacterial humoral response; inflammatory response; innate immune response; osteoclast differentiation; the negative regulation of viral genome replication and apoptotic process; and the positive regulation of production of chemokine, interferon-beta, interleukin-6, tumor necrosis factor; and the positive regulation of NIK/NF-kB pathway.
Twenty-nine common DEGs of two comparisons (LFT1000 and LFT500 vs. control) in the chicken jejuna were closely associated with phosphatidylethanolamine biosynthetic process and neuron projection extension (Figure 2D). In addition, Figures 2E–Q represents the heatmaps for DEGs in cell adhesion, cell proliferation, blood coagulation, collagen biosynthetic process, collagen fibril organization, glutathione metabolic process, macrophage chemotaxis, the negative regulation of interferon-gamma production, and the positive regulation of gene expression, protein phosphorylation, and the response to oxidative stress, respectively.
KEGG Enrichment for DEGs
To discover the pathways related to the regulation of LFT on broiler intestinal morphology and health, DEGs in the three comparisons, including LFT500 vs. control, LFT1000 vs. control, and LFT1000 vs. LFT500, were implemented in KEGG analysis, and the results were shown in Figures 3A–C, respectively (Additional Files 8–10). As illustrated in Figure 3D, the common DEGs of two comparisons (LFT500 and LFT1000 vs. control) are mainly linked to ABC transporters, AGE-RAGE, fatty acid elongation, focal adhesion, glycerophospholipid metabolism, ECMRI, and unsaturated fatty acids biosynthesis pathways. In addition, Figures 3E–O reveals toll-like receptor (TLR), PPAR, metabolism, adipocytokine, carbon metabolism, cell adhesion molecules, drug metabolism-cytochrome P450, fatty acid degradation, mTOR, fatty acid metabolism, and Wnt signaling pathway, respectively.
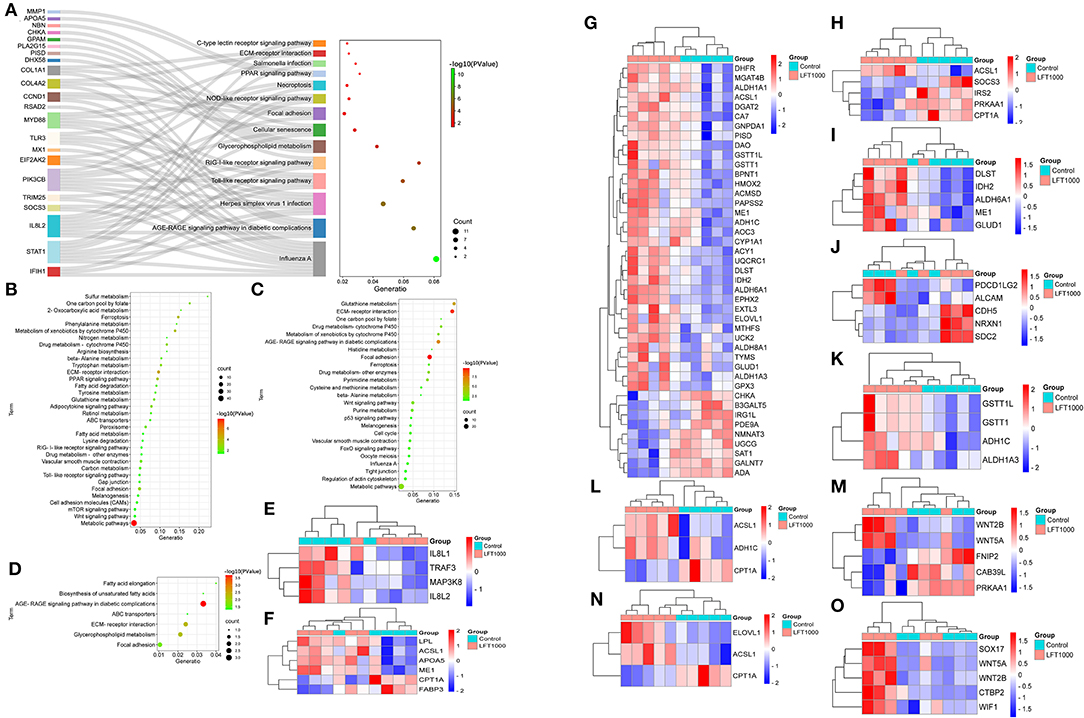
Figure 3. KEGG Enrichment for DEGs in Broilers Jejuna Treated with and without LFT. (A–C) KEGG enrichment for DEGs was identified by three comparisons (LFT500 and LFT1000 vs. control and LFT1000 vs. LFT500, respectively). (D) KEGG enrichment for the common DEGs was identified by two comparisons (LFT500 and LFT1000 vs. control). (E–O) Heatmaps for DEGs in TLR, PPAR, metabolism, adipocytokine, carbon metabolism, CAMs, drug metabolism-cytochrome P450, fatty acid degradation, mTOR, fatty acid metabolism, and Wnt signaling pathway.
Reactome Enrichment and Protein Classification for DEGs
To further reveal the pathways related to LFT regulation on broiler jejuna heath, Reactome enrichment for DEGs in the three comparisons was performed. DEGs identified by comparing LFT1000 vs. the control may link to the metabolism, hemostasis, signal transduction, small molecules transport, carbohydrates metabolism, platelet activation, glycosaminoglycan metabolism, ECM organization, and neuronal system (Figure 4A). DEGs between LFT500 and the control related to hemostasis, signal transduction, cytokine signaling, neutrophil degranulation, TLR cascades, interleukin-2 family signaling, and platelet activation, signaling, and aggregation (Figure 4B).
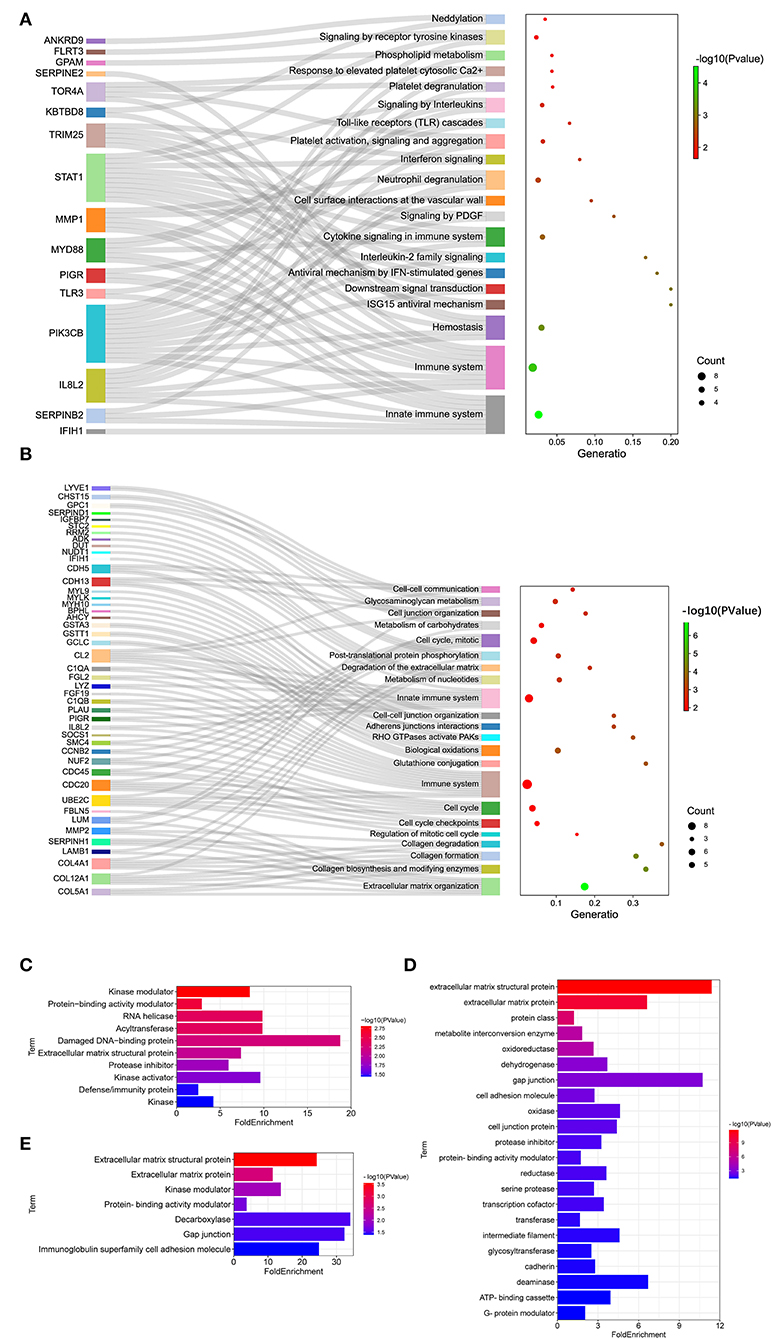
Figure 4. Reactome Analysis and Protein Classification for DEGs. (A,B) Reactome analysis for DEGs was identified in two comparisons (LFT1000 and LFT500 vs. control). (C–E) Protein classification for DEGs was identified in two comparisons (LFT500 and LFT1000 vs. control and LFT1000 vs. LFT500, respectively).
Protein classification for DEGs in the three comparisons was performed. DEGs between LFT500 and the control groups might play an important role in acyltransferase, RNA helicase, kinase modulator, protease inhibitor, kinase activator, damaged DNA-binding protein, protein-binding activity modulator, ECM structural protein, and defense/immunity protein kinase (Figure 4C). DEGs between LFT1000 and the control groups might contribute to reductase, oxidase, cadherin, deaminase, transferase, dehydrogenase, oxidoreductase, glycosyltransferase, gap junction, transcription cofactor, serine protease, intermediate filament, protease inhibitor, cell adhesion molecule, ECM structural protein, ECM protein, metabolite interconversion enzyme, cell junction protein, protein-binding activity modulator, and ATP-binding cassette G-protein modulator (Figure 4D). The common DEGs of two comparisons (LFT500 and LFT1000 vs. control) might link to decarboxylase, kinase modulator, gap junction, ECM protein, ECM structural protein, and protein-binding activity modulator, and immunoglobulin superfamily cell adhesion molecule (Figure 4E).
PPI Network
To further explore key genes, DEGs in the three comparisons, including LFT500 and LFT1000 vs. control and LFT1000 vs. LFT500, were implemented in PPI networks analysis, and the results are shown in Figures 5A–C, respectively.
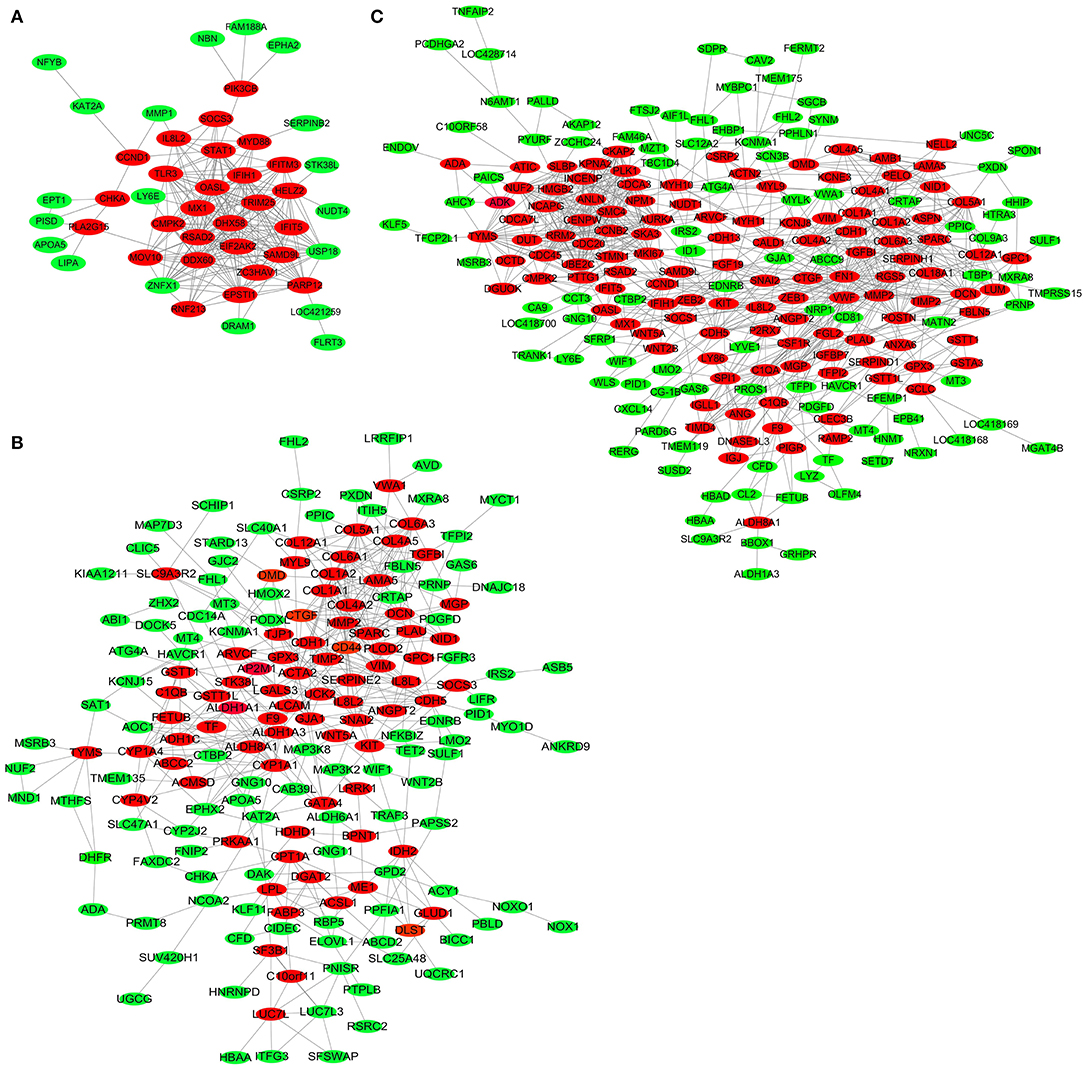
Figure 5. PPI Network for DEGs in broilers jejuna treated with and without LFT. (A–C) PPI network for DEGs was identified by three comparisons (LFT1000 and LFT500 vs. control and LFT1000 vs. LFT500, respectively).
Hub Genes and Their Function
The top 20 hub genes from DEGs between LFT500 and control groups included RSAD2, DHX58, DDX60, OASL, IFIH1, IFIT5, EPSTI1, CMPK2, USP18, and HELZ2 (Figure 6A). The top 28 hub genes from DEGs between LFT1000 and control groups included COL1A1, COL1A2, SPARC, COL6A1, COL5A1, MMP2, DCN, COL4A2, COL6A3, and CTGF (Figure 6B). The top 30 hub genes from DEGs between LFT1000 and LFT500 groups included PLK1, AURKA, CCNB2, CDC20, NCAPG, NUF2, UBE2C, CDCA3, KPNA2, and CKAP2 (Figure 6C).
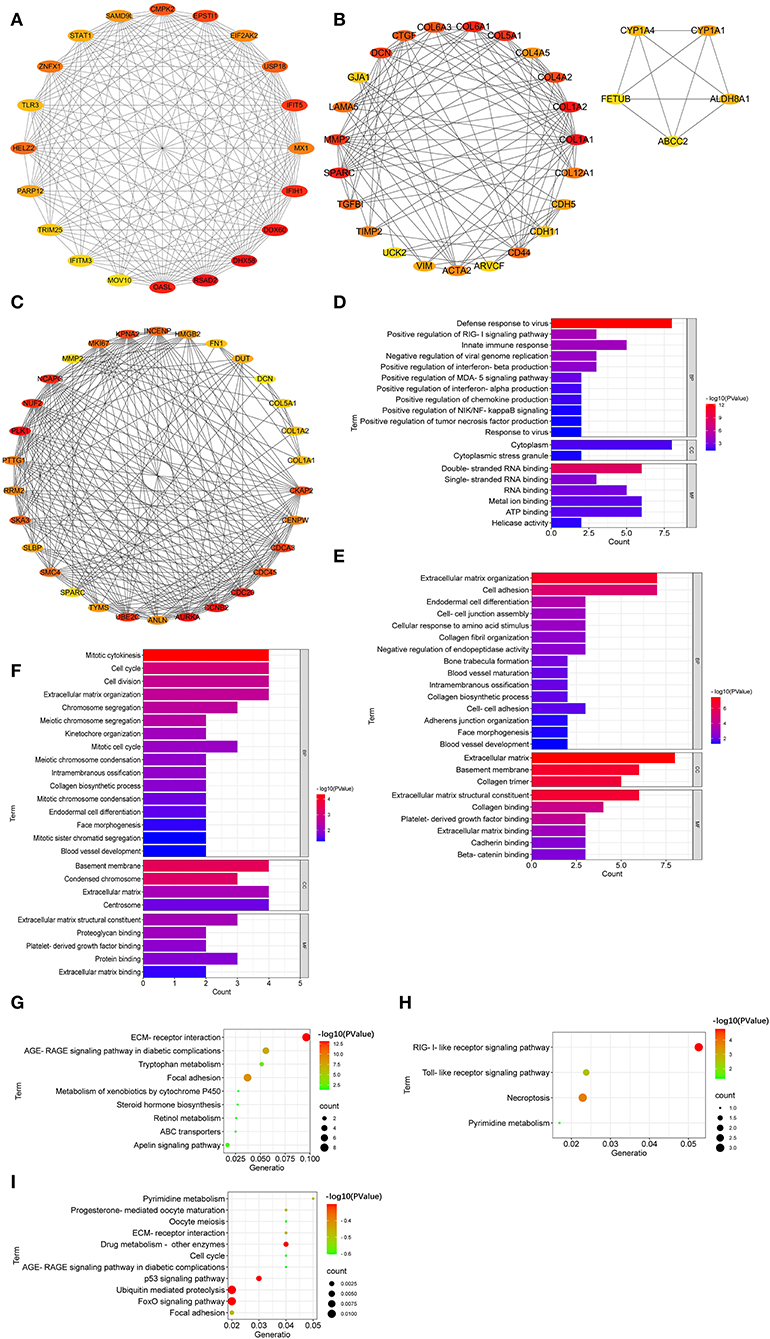
Figure 6. Hub Genes Linked to the Regulation of LFT on the Morphology and Health in Broiler Jejuna. (A–C) Hub genes from DEGs were identified by three comparisons (LFT500 and LFT1000 vs. control and LFT1000 vs. LFT500, respectively). (D–F) GO and (G–I) KEGG enrichment for hub genes mentioned above.
GO enrichment suggested that the top 20 hub genes in the comparison of LFT500 vs. control related to innate immune response; the positive regulation of the production of chemokine, interferon-alpha, interferon-beta, and tumor necrosis factor; and the positive regulation of MDA-5, NIK/NF-kB, and RIG-I signaling pathway (Figure 6D). The top 28 hub genes from DEGs between LFT1000 and control groups participated in cell adhesion, endodermal cell differentiation, cell–cell junction assembly, intramembranous ossification, ECM organization, collagen fibril organization, blood vessel maturation, collagen biosynthetic process, blood vessel development, and the negative regulation of endopeptidase activity (Figure 6E). The top 30 hub genes from DEGs between LFT500 and LFT1000 groups are linked to cell division, cell cycle, mitotic cytokinesis, intramembranous ossification, kinetochore organization, chromosome segregation, blood vessel development, mitotic cell cycle, endodermal cell differentiation, mitotic chromosome condensation, collagen biosynthetic process, meiotic chromosome condensation, ECM, mitotic sister chromatid segregation, etc (Figure 6F).
KEGG enrichment suggested that the top 20 hub genes from DEGs between LFT500 and control involved in RIG-I-like receptor, pyrimidine metabolism, necroptosis, and TLR pathways (Figure 6G). The top 28 hub genes compared to LFT1000 vs. control linked to tryptophan metabolism, focal adhesion, retinol metabolism, ABC transporters, AGE-RAGE, apelin, and xenobiotics metabolism by cytochrome P450 signaling pathways (Figure 6H). The top 30 hub genes from DEGs between LFT1000 and LFT500 groups participated in p53, Fox O, AGE-RAGE, cell cycle, focal adhesion, drug metabolism, pyrimidine metabolism, and ECMRI signaling pathways (Figure 6I).
Discussion
Hub Genes by Which LFT Maintains the Intestinal Morphology and Health in Broilers
In this study, multiple hub genes, such as REG4, KAT2A, APOA5, SERPINE2, ELOVL1, ABCC2, ANKRD9, CYP4V2, and PISD1, might participate in the regulation of LFT on intestinal morphology and health in broilers. For instance, REG4, a member of the small secretory protein family, was reported to participate in inflammatory bowel diseases and intestinal cancers (9–12).
In our study, 500 and 1,000 g/ton LFT treatment increased the REG4 expression in the chicken jejuna (2.36- and 2.60-fold), which is consistent with Qi et al. who demonstrated that REG4 was involved in membrane attack complexes killed inflammatory Escherichia coli (E. coli) to maintain gut health, and REG4 gene knockdown increased the content of E. coli in the intestinal tract (9). REG4 was obviously upregulated in colorectal cancer (CRC) tissue compared to the normal tissue. REG4 expression in CRC tissue was linked to distant and lymph-node metastasis and histologic grade. REG4 expression in CRC patients showed a worse prognosis (10). REG4 was the biomarker to predict concurrent chemoradiotherapy resistance in patients with rectal cancer. Previous research showed that the significant upregulation of the REG4 gene was closely related to the undesirable outcome and the aggressive phenotype in rectal cancer patients (11). In our study, REG4 played an important role in cell regeneration and proliferation. REG4 expression was linked to higher overall survival and favorable clinicopathological parameters in CRC patients (12).
KAT2A, named lysine acetyltransferase 2A, inhibited the proliferation and growth of the intestinal cell, especially in CRC cells. KAT2A, succinylate, and succinyltransferase could decrease the α-KGDH complex entered the nucleus, reduce the gene expression, and inhibit the cell proliferation and growth in intestinal cancers (13). Histone acetyltransferase KAT2A could interact with long noncoding RNA LBX2-AS1 and RNA-binding protein PTBP1 and regulate the cell proliferation and invasion in CRC (14).
In humans and animals, APOA5 was a vital gene for intestine chylomicron production and lipids metabolism. APOA5 decreased the serum triglyceride (TG) by restraining ANGPTL3/8-mediated lipoprotein lipase inhibition (15). Variants in the APOA5 gene affected TG concentrations and the entire lipoprotein subclass distribution and caused hypertriglyceridemia (16). Hypermethylation in exon 3 of the APOA5 gene had a positive correlation with the lipoprotein profile and TG concentration linked to atherogenic dyslipidemia. The highest TG concentrations were observed in carriers with a high methylation percentage in the exon 3 of the APOA5 gene (17). In our study, APOA5 expression was obviously improved 1.65- and 2.05-fold in chicken jejuna by 500 and 1,000 g/ton LFT treatment, which was consistent with the previous finding that APOA5 might control TG synthesis and secretion in the intestine. In the TC-7 cell line, saturated fatty acids stimulation obviously increased the APOA5 gene expression; Similarly, fatty acid butyrate administration improved APOA5 expression by ~4 times; PPARα agonist treatment also enhanced the APOA5 expression by 60% (18). In addition, PPARα has a vital role in lipid metabolism and improves ketogenesis and oxidation of fatty acid. PPARα activation reduced food intake and improved insulin sensitivity. Wy-14643 administration significantly increased the expression of HMG-CoAS2 and CPT1A genes in the jejunum. The induction of HMG-CoAS2 and CPT1A expression in the jejunum was linked to the decreased content of lipid droplet. HMG-CoAS2 and CPT1A were two important enzymes for ketogenesis (19).
SERPINE2 might be a vital gene for intestinal health and disease, such as colorectal cancer. Intestinal epithelial cells (IECs), activation of oncogenic extracellular signal-related kinase (ERK), Ras, or BRAF strongly upregulated the SERPINE2 protein expression and secretion (20). SERPINE2 gene expression was also dramatically increased in CRC cells compared with normal IECs; In the HCT116 cell, SERPINE2 knockdown distinctly decreased the anchorage-independent growth, tumor formation, and cell migration in nude mice; SERPINE2 mRNA level in CRC cell lines was markedly decreased by U0126 (a highly specific MEK1/2inhibitor) administration (21).
ELOVL1, a widely expressed gene in tissues from the ELOVL family, encoded the fatty acid elongase to produce C20–C28 fatty acids. In this study, ELOVL1 was observably upregulated in chicken jejuna from LPC-treated groups, consistent with the report that ELOVL1 regulated the very-long-chain fatty acid and sphingolipids synthesis, and was closely associated with the intestinal barrier function (22, 23). ELOVL1 expression induced by inhibiting mTOR1 decreased fatty acids synthesis (22, 23). A previous study in mice indicated that ELOVL1 knockout induced the defects in the epidermal barrier and the death after birth. In the epidermis of ELOVL1 knockout mice, the content of C24 sphingomyelin was reduced, but the C20 sphingomyelin level was increased (23).
ABCC2, the gene encoding multidrug resistance protein 2 (MRP2), was located on the small intestinal epithelial brush border membrane. ABCC2 had a vital role in regulating the absorption of nutrients and toxins (24–26). In this study, ABCC2 expression was dramatically improved in chicken jejuna from LPC-treated groups. This result agreed with the report that ABCC2 could limit the absorption of toxins, improve the endogenous xenobiotics and substances efflux, and mediate the beneficial effect of Lactobacillus plantarum on poultry intestines (24). The expression pattern of MRP2/ABCC2 in the small intestinal tract was tightly regulated. MRP2/ABCC2 expression in the small intestine was closely associated with ezrin phosphorylation status (25). ABCC2, ABCC3, and ABCG2 were expressed in the intestine and could transport the glucuronidated compounds. ABCC2 knockout significantly decreased the biliary excretion in mice (26). The exposure to thymeleatoxin reduced the amount of ABCC2 protein and the active ezrin. Moreover, cPKC activation weakened the interaction between ABCC2 and ezrin proteins (27).
Signaling Pathways by Which LFT Maintains the Intestinal Morphology and Health in Broilers
Our study found that LFT regulated the intestinal morphology and health in broilers via multifarious signaling pathways, including AGE-RAGE, ECMRI, focal adhesion, and ABC transporters. For example, AGE expression in the jejunal villi crypt as well as RAGE expression in the villi significantly enhanced the jejunal layer thickness, weight per length, and wall area (28). RAGE signaling was closely linked to intestinal permeability and inflammation. AGE-RAGE signaling and RAGE activation in the intestinal epithelium contributed to intestinal permeability and pathogenesis (29).
ECM, a vital component of the intestine, provided the structural framework and conveyed tissue-specific signals to the adjacent enterocytes. Porcine epidemic diarrhea virus (PEDV) infection resulted in extensive ECM remodeling in IECs. SERPINE1 and CD44, two ECM-regulated genes, could enhance or inhibit the PEDV infection (30).
A previous research found that various signaling pathways were involved in intestinal schistosomiasis and trinitro-benzene-sulfonic acid-induced ileitis, such as ABC transporters, cell adhesion, ECMRI, antigen processing and presentation, TLR, and the response to chemical stimulus categories (31). The ABC-transporter mediated the cellular uptake, absorptive permeability, and intestinal absorption. For instance, the ABC-transporter-mediated efflux and the poor permeability were the major reasons for Rh2 poor absorption (32).
Fatty acid metabolism, such as fatty acid biosynthesis and elongation, might play a vital role in intestinal absorption and health. In our study, DEGs in chicken jejuna between LFT-treated groups and the control also enriched in fatty acid elongation and unsaturated fatty acids biosynthesis signaling pathways that were consistent with the report that polyunsaturated fatty acids, including oleic acid, linolenic acid, and conjugated linoleic acid (CLA), had a protective effect in the intestine morphology and health (33). In IECs, long-chain saturated fatty acids stimulated TG synthesis, and stearic acids and palmitic also stimulated phospholipid synthesis (34).
CLA potentially modulates gut microbiota and intestinal permeability. CLA increased intestinal permeability in the normal mice and obviously improved the tight junction proteins in the intestine of leptin-deficient mice (35). CLA increased the abundance of beneficial bacteria (such as Roseburia, Dubosiella, and Anaerostipes) and increased the abundance of pro-inflammatory bacteria (such as Alistipes and Tyzzerella) in eptin-deficient mice. In addition, gut microbiota was associated with intestinal permeability [39]. CLA increased the SIgA mRNA and SIgA protein content in the jejunal mucosa. CLA treatment significantly increased PPARγ expression in jejunum as well as lymphocyte proliferation, and the percent of T lymphocytes (CD8+) in Peyer's node of broilers (36).
CLA addition could obviously enhance the immunity and antioxidant capacity of the intestinal mucosa in broilers. CLA supplementation at the level of 1.50% notably improved the CD8+ T lymphocytes percentage in the duodenal epithelium, reducing the concentration of malondialdehyde and glutathione in the duodenal mucosa of the birds infected by Eimeria acervuline but had no effects on the activities of catalase and superoxide dismutase (37).
Conclusion
Taken together, signaling pathways (such as AGE-RAGE, fatty acid elongation, ECMRI, glycerophospholipid metabolism, focal adhesion, unsaturated fatty acids biosynthesis, and ABC transporters) and 29 genes (including REG4, GJB1, KAT2A, APOA5, SERPINE2, ELOVL1, ABCC2, ANKRD9, CYP4V2, and PISD) might be closely related to promoting jejuna morphology in broilers. Our observation enhances the understanding of LFT in maintaining intestinal architecture and the general health of broiler chickens.
Data Availability Statement
The datasets presented in this study can be found in online repositories. The names of the repository/repositories and accession number(s) can be found in the article/Supplementary Material.
Ethics Statement
The animal study was reviewed and approved by the Protocol from Anhui Science and Technology University (Bengbu, China) Institutional Animal Care and Use Committee (ECASTU-2015-P08).
Author Contributions
BY conceived the study. XL wrote the manuscript and prepared the figures. XS, NM, LL, and ZC prepared the tables and analyzed the results. All authors contributed to the article and approved the submitted version.
Funding
This study was funded by the Talent Introduction Program of Anhui Science and Technology University (No. DKYJ202003) and the National Natural Science Foundation of China (No. 31560642).
Conflict of Interest
The authors declare that the research was conducted in the absence of any commercial or financial relationships that could be construed as a potential conflict of interest.
Publisher's Note
All claims expressed in this article are solely those of the authors and do not necessarily represent those of their affiliated organizations, or those of the publisher, the editors and the reviewers. Any product that may be evaluated in this article, or claim that may be made by its manufacturer, is not guaranteed or endorsed by the publisher.
Supplementary Material
The Supplementary Material for this article can be found online at: https://www.frontiersin.org/articles/10.3389/fvets.2022.946148/full#supplementary-material
References
1. Panda A, Sridhar K, Goodla L, Prakash B, Rao SVR, Raju MVLN. Effect of dietary incorporation of fish oil on performance, carcass characteristics, meat fatty acid profile, and sensory attributes of meat in broiler chickens. Anim Nutr Feed Techno. (2016) 16:417–25. doi: 10.5958/0974-181X.2016.00037.8
2. Saleh AA, Amber KA, Mousa MM, Nada AL, Awad W, Dawood MAO, et al. A Mixture of exogenous emulsifiers increased the acceptance of broilers to low energy diets: growth performance, blood chemistry, and fatty acids traits. Animals. (2020) 10:437. doi: 10.3390/Ani10030437
3. Siyal FA, Babazadeh D, Wang C, Arain MA, Saeed M, Ayasan T, et al. Emulsifiers in the Poultry Industry. World Poult Sci J. (2017) 73:611–20. doi: 10.1017/S0043933917000502
4. Metwally AE, Abdel-Wareth AAA, Saleh AA, Amer SA. Are the energy matrix values of the different feed additives in broiler chicken diets could be summed? BMC Vet Res. (2020) 16:391. doi: 10.1186/S12917-020-02600-3
5. Melegy T, Khaled NF, El-Bana R, Abdellatif H. Dietary fortification of a natural biosurfactant, lysolecithin in broiler. Afr J Agr Res. (2010) 5:2886–92.
6. Schwarzer K, Adams CA. The influence of specific phospholipids as absorption enhancer in animal nutrition. Fett-Lipid. (1996) 98:304–8. doi: 10.1002/Lipi.19960980905
7. Papadopoulos GA, Poutahidis T, Chalvatzi S, Di Benedetto M, Hardas A, Tsiouris V, et al. Effects of Lysolecithin supplementation in low-energy diets on growth performance, nutrient digestibility, viscosity and intestinal morphology of broilers. Brit Poultry Sci. (2018) 59:232–9. doi: 10.1080/00071668.2018.1423676
8. Brautigan DL Li R, Kubicka E, Turner SD, Garcia JS, Weintraut ML, et al. Lysolecithin as feed additive enhances collagen expression and villus length in the jejuna of broiler chickens. Poult Sci. (2017) 968:2889–98. doi: 10.3382/ps/Pex078
9. Qi HB, Wei JM, Gao YH, Yang YZ Li YY, Zhu H, et al. Reg4 and complement factor d prevent the overgrowth of E. Coli in the Mouse gut Commun Biol. (2020) 3:483. doi: 10.1038/S42003-020-01219-2
10. Zhu XW, Han Y, Yuan CW, Tu WW, Qiu GQ, Lu S, et al. Overexpression of Reg4, alone or combined with MMP-7 overexpression, is predictive of poor prognosis in colorectal cancer. Oncol Rep. (2015) 33:320–28. doi: 10.3892/or.2014.3559
11. He HL, Lee YE, Shiue YL, Lee SW, Lin LC, Chen TJ, et al. Overexpression of REG4 confers an independent negative prognosticator in rectal cancers receiving concurrent chemoradiotherapy. J Surg Oncol. (2014) 110:1002–10. doi: 10.1002/jso.23764
12. Kaprio T, Hagstrom J, Mustonen H, Koskensalo S, Andersson LC, Haglund C. REG4 independently predicts better prognosis in non-mucinous colorectal cancer. PLoS ONE. (2014) 9:109600. doi: 10.1371/Journal.Pone.0109600
13. Wang YG, Guo YSR, Liu K, Yin Z, Liu R, Xia Y, et al. KAT2A Coupled With the α-KGDH complex acts as a histone H3 succinyltransferase. Nature. (2017) 552:273–7. doi: 10.1038/Nature25003
14. Zhao AD, Wang Y, Lin FL, Bai KY, Gu C. Long |noncoding RNA LBX2-AS1 promotes colorectal cancer progression via binding with PTBP1 and stabilizing KAT2A expression. J Biochem Mol Toxic. (2022) 36:E23020. doi: 10.1002/jbt.23020
15. Chen YQ, Pottanat G, Zhen EY, Siegel RW, Ehsani M, Qian YW, et al. ApoA5 Lowers triglyceride levels via suppression of ANGPTL3/8-mediated LPL inhibition. J Lipid Res. (2021) 62:100068. doi: 10.1016/j.jlr.2021.100068
16. Guardiola M, Ribalta J. Update on APOA5 Genetics: toward a better understanding of its physiological impact. Curr Atheroscler Rep. (2017) 19:30. doi: 10.1007/S11883-017-0665-Y
17. Oliva I, Guardiola M, Vallvé J, Ibarretxe D, Plana N, Masana L, et al. APOA5 Genetic and epigenetic variability jointly regulate circulating triacylglycerol levels. Clin Sci. (2016) 130:2053–9. doi: 10.1042/CS20160433
18. Guardiola M, Alvaro A, Vallvé JC, Rosales R, Solà R, Girona J, et al. APOA5 Gene expression in the human intestinal tissue and its response to in vitro exposure to fatty acid and fibrate. Nutr Metab Cardiovasc Dis. (2012) 22:756–62. doi: 10.1016/j.Numecd.2010.12.003
19. Azari EK, Leitner C, Jaggi T, Langhans W, Mansouri A. Possible role of intestinal fatty acid oxidation in the eating-inhibitory effect of the PPAR-alpha agonist Wy-14643 in high-fat diet fed rats. PLoS ONE. (2013) 8:E74869. doi: 10.1371/Journal.Pone.0074869
20. Monard D. SERPINE2/Protease Nexin-1 in vivo multiple functions: does the puzzle make sense? Semin Cell Dev Biol. (2017) 62:160–9. doi: 10.1016/j.Semcdb.2016.08.012
21. Bergeron S, Lemieux E, Durand V, Cagnol S, Carrier JC, Lussier JG, et al. The serine protease inhibitor serpine2 is a novel target of ERK signaling involved in human colorectal tumorigenesis. Mol Cancer. (2010) 9:271. doi: 10.1186/1476-4598-9-271
22. Wang WP, He Q, Guo ZX, Yang LM, Bao LL, Bao WL, et al. Inhibition of mammalian target of rapamycin complex 1 (MTORC1) downregulates elovl1 gene expression and fatty acid synthesis in goat fetal fibroblasts. Int J Mol Sci. (2015) 16:16440–53. doi: 10.3390/Ijms160716440
23. Sassa T, Ohno Y, Suzuki S, Nomura T, Nishioka C, Kashiwagi T, et al. Impaired epidermal permeability barrier in mice lacking elovl1, the gene responsible for very-long-chain fatty acid production. Mol Cell Biol. (2013) 33:2787–96. doi: 10.1128/MCB.00192-13
24. Milanova A, Pavlova I, Yordanova V, Danova S. Effect of doxycycline and lactobacillus probiotics on MRNA expression of ABCC2 in small intestines of chickens. Iran J Vet Res. (2016)17:265–7.
25. Nakano T, Sekine S, Ito K, Horie T. Ezrin regulates the expression of Mrp2/Abcc2 and Mdr1/Abcb1 along the rat small intestinal tract. Am J Physiol Gastrointest Liver Physiol. (2013) 305:G807–17. doi: 10.1152/Ajpgi.00187.2013
26. Waart DR, Vlaming MLH, Kunne C, Schinkel AH, Elferink RPJO. Complex pharmacokinetic behavior of ezetimibe depends on ABCC2, ABCC3, and ABCG2. Drug Metab Dispos. (2009) 37:1698–702. doi: 10.1124/dmd.108.026146
27. Nakano T, Sekine S, Ito K, Horie T. Correlation between apical localization of Abcc2/Mrp2 and phosphorylation status of ezrin in rat intestine. Drug Metab Dispos. (2009) 37:1521–27. doi: 10.1124/dmd.108.024836
28. Zhao JB, Chen PM, Gregersen H. Morpho-mechanical intestinal remodeling in type 2 diabetic GK rats-is it related to advanced glycation end product formation? J Biomech. (2013) 46:1128–34. doi: 10.1016/j.Jbiomech.2013.01.010
29. Snelson M, Lucut E, Coughlan MT. The role of AGE-RAGE signalling as a modulator of gut permeability in diabetes. Int J Mol Sci. (2022) 23:1766. doi: 10.3390/Ijms23031766
30. Li YC Li JD, Wang XY, Wu QX, Yang Q. Role of intestinal extracellular matrix-related signaling in porcine epidemic diarrhea virus infection. Virulence. (2021) 12:2352–65. doi: 10.1080/21505594.2021.1972202
31. Avula LR, Knapen D, Buckinx R, Vergauwen L, Adriaensen D, Van Nassauw L, et al. Whole-genome microarray analysis and functional characterization reveal distinct gene expression profiles and patterns in two mouse models of ileal inflammation. BMC Genomics. (2013) 13:377. doi: 10.1186/1471-2164-13-377
32. Gu Y, Wang GJ, Wu XL, Zheng YT, Zhang JW Ai H, et al. Intestinal absorption mechanisms of ginsenoside Rh2: stereoselectivity and involvement of ABC transporters. Xenobiotica. (2010) 40:602–12. doi: 10.3109/00498254.2010.500744
33. Apas AL, Arena ME, Colombo S, Gonzalez SN. Probiotic administration modifies the milk fatty acid profile, intestinal morphology, and intestinal fatty acid profile of goats. J Dairy Sci. (2013) 98:47–54. doi: 10.3168/jds.2013-7805
34. Wang H, Berschneider HM, Du JH, Black DD. Apolipoprotein secretion and lipid synthesis: regulation by fatty acids in newborn swine intestinal epithelial cells. Am J Physiol-Gastr Liver Physiol. (1997) 272:G935–42. doi: 10.1152/Ajpgi.1997.272.5.G935
35. Gao SL, He YY, Zhang LP, Liu LN, Qu CF, Zheng Z, et al. Conjugated linoleic acid ameliorates hepatic steatosis by modulating intestinal permeability and gut microbiota in ob/ob mice. Food Nutr Res. [2022] 66:8226. doi: 10.29219/fnr.v66.8226
36. Liu YX, Yang JP, Tang GP, Jiang DF. Effects of dietary conjugated linoleic acid on the intestinal mucosal immunity of broiler chickens. Ital J Anim Sci. (2017) 16:601–7. doi: 10.1080/1828051X.2017.1305874
Keywords: gene, signaling pathway, Lysoforte, jejuna, broiler
Citation: Li X, Shi X, Mesalam NM, Liu L, Chen Z and Yang B (2022) Mechanism of Lysoforte in Improving Jejuna Morphology and Health in Broiler Chickens. Front. Vet. Sci. 9:946148. doi: 10.3389/fvets.2022.946148
Received: 17 May 2022; Accepted: 21 June 2022;
Published: 19 July 2022.
Edited by:
Vinod Kumar Paswan, Banaras Hindu University, IndiaReviewed by:
Vijay Kumar Singh, Acharya Narendra Deva University of Agriculture & Technology (ANDUAT), IndiaMahmoud M. Alagawany, Zagazig University, Egypt
Qiuning Liu, Yancheng Teachers University, China
Copyright © 2022 Li, Shi, Mesalam, Liu, Chen and Yang. This is an open-access article distributed under the terms of the Creative Commons Attribution License (CC BY). The use, distribution or reproduction in other forums is permitted, provided the original author(s) and the copyright owner(s) are credited and that the original publication in this journal is cited, in accordance with accepted academic practice. No use, distribution or reproduction is permitted which does not comply with these terms.
*Correspondence: Bing Yang, YmluZ3lhbmcxOTg2MDkxOSYjeDAwMDQwOzE2My5jb20=