- 1Laboratory of Molecular Reproduction, Research Institute of Agriculture, Tokai University, Kumamoto, Japan
- 2Department of Obstetrics and Gynecology, Kanazawa University Graduate School of Medical Science, Kanazawa, Japan
The sine qua non of new life is fertilization. However, approximately 50% of fertilized eggs/blastocysts in cattle and up to 75% of those from human assisted reproductive procedures fail during the first 3 to 4 weeks of pregnancy, including peri-implantation periods. In these periods, blastocyst hatching and implantation to the maternal endometrium proceeds, during which physiological events such as epithelial-mesenchymal transition (EMT) and trophoblast cell fusion occur. Quite recently, extracellular vesicles (EVs) with micro RNAs (miRNAs) and long non-coding RNAs (lncRNAs) have been found to play a pivotal role for the establishment of the proper uterine environment required for peri-implantation processes to proceed. New findings of EVs, miRNA, and lncRNAs will be described and discussed to elucidate their connections with conceptus implantation to the maternal endometrium.
Introduction
Approximately 50% of fertilized eggs and/or blastocysts in cattle fail to continue their pregnancy during the first 3 to 4 weeks of pregnancy. During these periods, blastocysts and/or conceptuses (embryo plus extraembryonic membranes) undergo blastocyst hatching, conceptus elongation, conceptus attachment/adhesion to the maternal endometrium and subsequent placentation. It has been generally accepted that the communication between conceptus and endometrium in utero is crucial for the establishment of the proper uterine environment as well as maternal receptivity of the conceptus for pregnancy to proceed.
Communication between two cell types such as trophectoderm and uterine luminal epithelial cells is achieved through secretory molecules such as hormones and cytokines. These findings point to the importance of the analysis of cytokines and their receptor molecules using various methods including RNA-seq and proteomic analyses. In recent years, however, evidence has accumulated that extracellular vesicles [EVs, (1)] produced by the conceptus and endometrium play a role in communication between the two cell types. EVs, covered with a lipid bilayer, contain surface receptors and ligands, and cargo of proteins, lipids, metabolites, DNAs and RNAs from the originating cells. EVs with detergent-resistant membrane domains also contain relatively high concentrations of cholesterol, sphingomyelin, and ceramide, and these vesicles are stable in extracellular spaces (2).
In this review, recent findings of EVs, miRNA, and lncRNA functioning in utero during the early stages of bovine pregnancy will be presented and their roles in pregnancy success will be discussed.
EV Cargoes and Their Effects/Function
It has been found that the cargoes of EVs differ according not only to cellular origin, but also to physiological and/or pathological conditions. In fact, EVs contain a variety of bioactive molecules including proteins, lipids, metabolites, DNAs, and RNAs (mRNA, miRNAs, and other RNA molecules) (3). In addition, there are numerous proteins and receptors, such as epidermal growth factor receptor (EGFR), bound onto the lipid layer of EVs. Through lipidomic analysis, EVs were found to contain cholesterol, sphingomyelin, ceramide, glycerophospholipids, phosphatidylcholine, and phosphatidylserine (1, 4–6). Proteomic analyses have also revealed that EVs contain different types of proteins such as heat shock proteins (HSP70 and HSP90), tetraspanins (CD9, CD63 and others), major histocompatibility complex class I and II (MHC class I and II), endosomal sorting complex proteins (Alix and Tsg101) and chaperons, most of which are often used for protein markers (1, 4, 5). Other cargoes of EVs are membrane trafficking proteins (Annexins, Flotillin and GTPases), cytoskeletal proteins (actin and tubulin), and numerous cytosolic proteins.
Involvement of EVs in Pregnancy Establishment
EVs and Conceptus Hatching and Elongation
It was previously thought that the early pre-implantation mammalian embryos are relatively autonomous, and that they control their own development. During the periods of peri-implantation, fertilized eggs/blastocysts go through numerous physiological changes including blastocyst migration, hatching, conceptus elongation, attachment/adhesion to the uterine epithelium, and placentation. These processes, particularly conceptus implantation and placentation, undoubtedly require not only their own gene functions but also maternal support (7). These two-way communications result in the generation of a proper uterine environment for both conceptus and endometrial developments. It was thought that various cytokines and their receptors play a role in conceptus-maternal communication (8). However, data has recently been accumulated that EVs, derived from both the conceptus trophectoderm and endometrium, play a role in bilateral communication between the conceptus and the endometrium (9–12).
The amount of EVs secreted from in vitro-cultured bovine embryos is correlated with embryo competence (13). In addition, the bovine embryo-derived EVs were found to improve the viability and growth of cloned embryos, as well as improve implantation rates and full-term calving rates (14). The correlation between EV secretion and in vitro embryo viability was also explored, and it was found that EV secretion during blastulation was indicative of viable bovine embryos. Further, EVs isolated from uterine flushing of pregnant and non-pregnancy female sheep (ewes) were tested for their ability to stimulate ovine trophectoderm (oTr1) cell proliferation. It was also found that EVs from pregnant ewes increased trophectoderm cell proliferation as well as the secretion of IFNT (15), the cytokine required for the prevention of corpus luteum demise in ruminants (16).
Non-invasive and elongated conceptuses initiate their attachment process to the uterine epithelium on day 16 in sheep and day 19 in cattle. Recent findings suggest that this is the time when the amounts of EVs in utero from both conceptus and uterine epithelium increase and play a role for conceptus growth, successful implantation and subsequent placentation (17–19).
EVs and Conceptus Cell Fusion
In ruminant trophectodermal cells, the formation of bi- and multi-nucleate cells begins concurrent with initiation of conceptus attachment to the uterine epithelium. The cellular mechanisms associated with the bi- and multi-nucleate cells have not been well-characterized; at present, however, two theories on trophectoderm cell fusion exist: consecutive nuclear divisions without mitotic polyploidy (cytokinesis) (20) or the fusion of mononucleate cells (21). It has been believed for the last several decades that tri- or multi-nucleate cells found in the uterine endometrium of the ruminants result from the cell fusion between the bi- or multi-nucleate trophectoderm and the uterine epithelial cells (22). It was quite recently demonstrated that fused cells in the ovine species comprised all trophectodermal cells (23), agreeing with results recently published elsewhere (24).
It was demonstrated that endogenous retroviruses (ERVs), anciently infected and integrated into the genomes of vertebrates, are involved in the formation of trophoblast bi- and possibly multi-nucleate cells. In the bovine species, ERVs of syncytin-Rum1 (25) and BERV-K1/Fematorin-1 (26) have been found to exhibit cell fusogenic activity. In sheep, endogenous Jaagsiekte retroviruses (enJSRVs), of which the envelop genes are transcribed, are expressed in elongating ovine conceptuses after day 12 of pregnancy (21). Experiments with loss of enJSRVs function demonstrate that this treatment retards trophectoderm outgrowth and inhibits trophoblast bi-nucleate cell formation on day 16. Although these enJSRVs are packaged into endometrium-derived viral particles, another study on EVs provided evidence that between day 12 and 16 of gestation, shortly before the increase in EV exchange, the enJSRVs RNAs are packaged within the EV cargoes (27). These results support the idea that EVs could deliver ERVs, in addition to proteins, miRNA and others, from the endometrium to the conceptus.
EVs and Conceptus Attachment/Adhesion to the Uterine Epithelium
Even in non-invasive placentation, elongated conceptus adhesion to the uterine epithelium is a typical prerequisite for placental formation in ruminant ungulates. It was found that bovine conceptus adhesion is mediated through a cell adhesion mediator, vascular adhesion molecule (VCAM-1) (28). Bovine uterine flushing (UF) containing EVs from the uteri of days 20 and 22 pregnancy has been shown to increase the expression of VCAM-1 in bovine endometrial epithelial cells (EECs) (29). Expression of VCAM-1 receptor, ITGA4, increased in day 22 bovine conceptuses (28). In addition, formation of fibrin at sites of conceptus adhesion has been demonstrated in the ewe (30). These observations strongly suggest that the bovine conceptus does adhere to the EECs on day 22, a couple days following the initiation of conceptus attachment to the uterine epithelium.
Epithelial-mesenchymal transition (EMT) is seen at the trophoblasts in the invasive mode of human and murine placentation. In 2012, however, Yamakoshi et al. found that most EMT-related factors are expressed in non-invasive bovine conceptuses on day 22 (31). The molecular mechanisms leading to conceptus EMT have been identified in the following two experiments. High expression of follistatin in bovine conceptuses on day 20 was down-regulated on day 22 and activin A, an EMT inducer, increased on the same day. Using bovine trophoblast CT-1 cells (32), a transcription factor OVOL2 was high on day 20. As OVOL2 expression decreases on day 22, EMT-related transcription factors, ZEB1 and SNAI2, along with mesenchymal cell markers, N-cadherin (CDH2) and vimentin (VIM), increase. Recently, Calle and coworkers executed an elegant experiment with the establishment of bovine trophoblast primary cells and endometrial mesenchymal cell lines (33). These authors demonstrated that interactions of both cell types through their secretomes (EVs and soluble proteins) lead to EMT in trophectodermal cells necessary for implantation and placentation (33). These investigators also found that after EMT, six proteins associated with the vascular endothelial growth factor (VEGF) pathway are increased (33), agreeing with the previous finding in which a micro-angiogenesis process related to uterine vascularization is necessary for implantation to proceed in the bovine species (34).
Proteomic analysis on human endometrial epithelial-derived EVs revealed that several members of the integrin family are found in these EVs, suggesting that these integrins play a role in docking these EVs to recipient cells and mediating trophoblast adhesion to endometrial cells through the interaction with appropriate ligands (35). In support of these observations, when EVs' miR-30d in the mouse endometrial fluid was transferred to murine embryos, they exhibited the up-regulation of cell adhesion molecules such as Itgb3, Itga7, and Cdh5 (36). These results suggest that regardless of invasive or non-invasive mode of placentation, EVs play a significant role in conceptus attachment and/or adhesion to the endometrium. Together with secretomes, the significance of EVs in these processes is on the rise.
EVs and Regulation of Immune Function
One of the crucial events that mammals must establish is maternal immunologic tolerance to the fetal allograft, which permits conceptus development in utero as well as the continuation of pregnancy. During the conceptus implantation period, EVs carry molecules likely to modulate the local endometrial (37) and possibly systemic immune systems (38). It was recently demonstrated that bovine EVs isolated from day 20 uterine flushing media (UFs) down-regulate the expression of immune-related genes in EECs (39). These investigators identified bta-miR-98 as a likely maternal immune system regulator. In dairy cows, Bta-miR-499, derived from placental exosomes, was found to regulate inflammation locally at the maternal-fetal annex through the inhibition of NK-kB signaling. Inhibition of bta-miR-499 results in deregulation of the inflammatory response at the maternal-fetal interface and fetal growth retardation (40). These results suggest that miRNA in intrauterine EVs play a role in the regulation of the local immune system to facilitate the continuation of pregnancy.
EVs and Their Possible Regulation by Progesterone
The direct action of progesterone (P4), the essential requirement for pregnancy in mammals, on conceptus development has not yet been elucidated, although its action is likely to be mediated through endometrial gene expression (41, 42). It was found numerous times that uterine gene expression in ruminants is regulated by P4 and/or IFNT during the peri-implantation periods (7, 18, 43–46). One novel experiment has demonstrated the biological effect of P4 on the production of EVs: EVs found in endometrial luminal and glandular epithelia increase their numbers over two-fold when ovariectomized sheep are treated with P4 (47). In addition, the results from analyses of ovine endometrial miRNAs and EVs in the uterine lumen revealed that P4 regulates seven miRNAs, of which three miRNAs are down-regulated and four miRNAs are up-regulated (47). A similar experiment in humans found that P4 induces changes in EV production and their protein cargo of EECs, and these EVs could increase the adhesive capacity of human and mouse blastocysts (35). Although P4-regulated EV cargoes have not yet been fully characterized, these results clearly indicate that P4 increases the release of EVs from the endometrial epithelium, which can be detectable in the uterine lumen.
Involvement of miRNA and lncRNA for Pregnancy Establishment
miRNAs and Their Biogenesis
It has been well-documented that miRNAs originate from large primary (pri) and precursor (pre) transcripts that undergo various processing steps till they reach mature and functional forms (48–50). Primary transcripts can be several kilobases in length but can be successfully cleaved by two RNase III enzymes, Drosha and Dicer, to produce approximately 70 nucleotide long precursor miRNA, and finally become 22–24 nucleotide long mature miRNAs (51). When delivered into target cells, the miRNAs are likely to inhibit target mRNAs post-transcriptionally through the formation of RNA induced silencing complex (RISC), leading to changes in gene expression and cellular functions in distant cells (Figure 1).
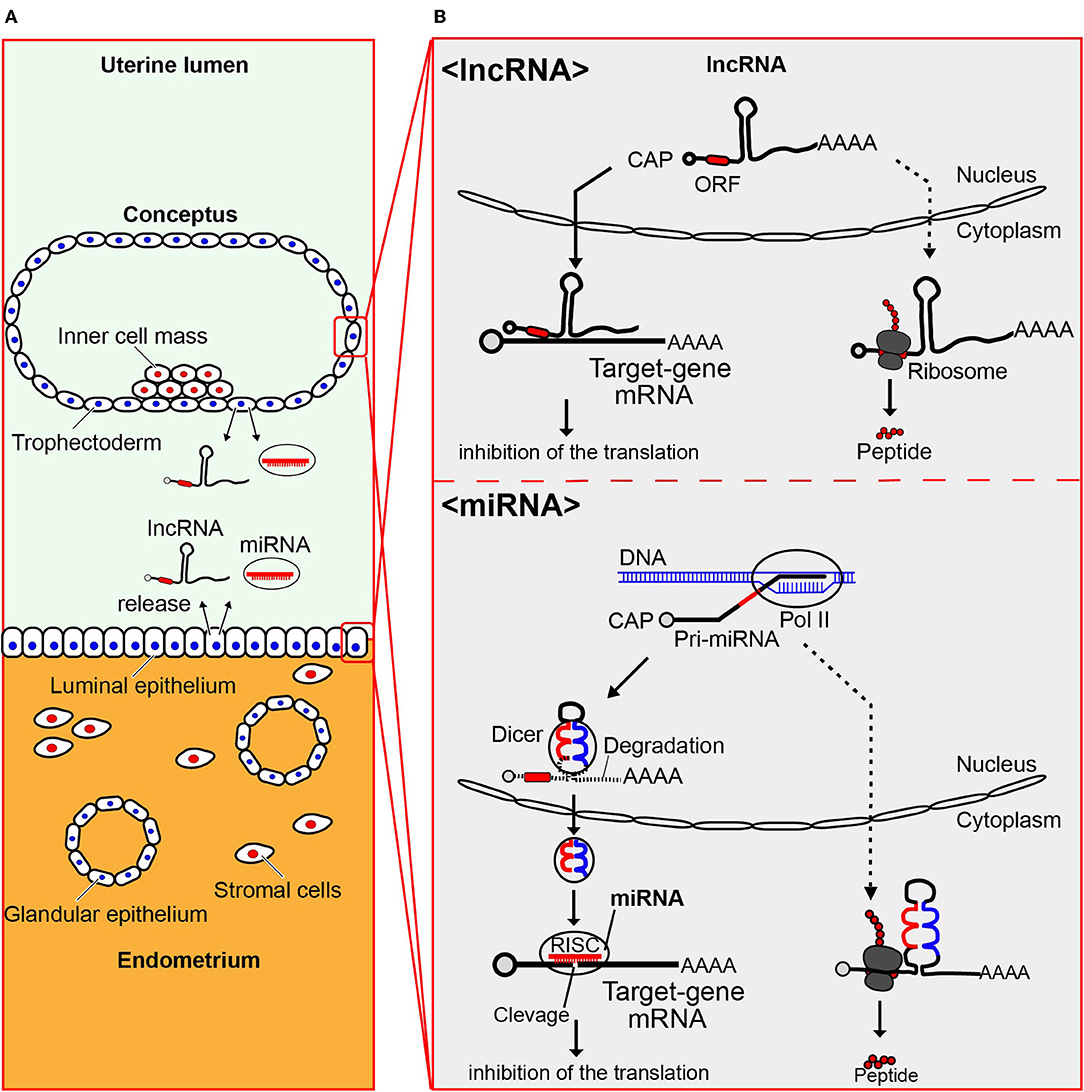
Figure 1. miRNA and lncRNA, produced by both conceptus and endometrium, are present in the uterus. (A) Uterine structure showing both maternal endometrium and conceptus (embryo plus extraembryonic membranes), from which miRNA and lncRNA are produced and miRNA in EVs and lncRNA are released into the uterine lumen. (B) Processing of lncRNA and miRNA is shown. Long non-coding RNAs (lncRNAs) are those with a length exceeding 200 nucleotides, some of which translate proteins/polypeptides (dotted-line arrow)._lncRNA can regulate the expression of target genes at the post-transcriptional level through the inhibition of their translation (solid-line arrow). Primary transcripts of miRNAs can be several kilobases in length, which are successfully cleaved by two RNase III enzymes, Drosha and Dicer, to produce approximately 70 nucleotide long precursor miRNA, and finally become 22–24 nucleotide long mature miRNAs (51) (solid-line arrow). The dotted-line arrow indicates that a part of those possibly produce polypeptides. When delivered into target cells, the miRNAs are likely to inhibit target mRNAs post-transcriptionally through the formation of RNA induced silencing complex (RISC), leading to changes in gene expression and cellular functions in distant cells.
lncRNA, and Functions of miRNAs and lncRNAs
Long non-coding RNAs (lncRNAs), defined as non-coding RNA sequences with a length exceeding 200 nucleotides, have emerged as important regulators in cellular functions such as translation of protein coding genes, signaling pathways, and epigenetic regulation (52, 53). These sequences have limited coding potential due to a lack of an open reading frame in the nucleotide segment. In cancer cells, lncRNAs have been extensively studied because they are potential regulators of many cellular mechanisms associated with cancer progression.
By binding to their target genes, miRNAs or lncRNAs regulate the expression of target genes at the post-transcriptional level, by inhibiting their translation. The miRNAs and lncRNAs should thus be treated with the same importance as those of target genes in the cellular development and differentiation processes. Most research related to miRNAs rests on the assumption that miRNAs exist intrinsically within many cell types. However, the mechanism by which miRNAs are sorted into EVs or retained in cells remains largely unknown.
Emerging Role of miRNA in Conceptus Hatching and Elongation
Hatching from the zona-pellucida is a prerequisite for embryo implantation and is less likely to occur in vitro for reasons not yet characterized. In a recent experiment (54), miR-378a-3p, secreted by individually cultured bovine embryos, is shown to promote blastocyst hatching. The next event required for pregnancy establishment in ruminants is the proper conceptus elongation prior to the initiation of conceptus attachment to the uterine epithelium (55). Several molecules involved in the regulation of conceptus elongation have been identified through loss of function experiments: proline-rich 15 (PRR15), nuclear peroxisome proliferator activator receptors (PPARs), hydroxysteroid (11-beta) dehydrogenase 1 (HSD11B1), and LIN28A/B. Sheep conceptuses treated with the lentivirus expressing shRNA against PRR15 result in embryonic losses on day 15 (56). Sheep conceptuses treated with morpholino antisense oligonucleotide against PPARs or HSD11B1 also result in severely growth-retarded conceptuses or conceptus fragments on day 14 (57, 58). Furthermore, trophectoderm-specific knockdown of LIN28A/B in day 9 ovine blastocysts results in increased let-7 mRNA and reduced conceptus elongation on day 16 (59). It should be noted that while these three molecules play a role during the conceptus elongation in utero, sufficient conceptus elongation in vitro has not been demonstrated.
Emerging Role of lncRNA in Conceptus Implantation
Expression of lncRNA in female reproductive tracts, particularly when expressed in utero during peri-implantation periods, has been characterized in pigs (60–63), goats (64), sheep (65), and mice (66). Quite recently, Matsuno et al. (67) analyzed the lncRNA profiles present in utero on days 15, 17, 19 and 21 of pregnancy in sheep. Among 8,808 lncRNAs identified, 3,423 lncRNAs were novel. Using gene ontology analysis, lncRNA target genes were enriched for cellular processes involved in the respiratory electron transport chain (RETC) (67). The results from these analyses suggest that in addition to secretomes, lncRNA is a potential new regulator in ovine conceptus development during peri-implantation periods.
Recent Finding on miRNA/lncRNA Processing
Quite recently, Garcia-Martin and coworkers (68) have demonstrated sorting sequences of miRNA that determine their secretion in EVs (EXOmotifs) or cellular retention (CELLmotifs). In their observations, insertion or deletion of these CELLmotifs or EXOmotifs in a miRNA increases or decreases retention in the cell of production or secretion into EVs. In addition, two RNA-binding proteins, Alyref and Fus, are involved in the delivery of miRNA with the EXOmotifs, CGGGAG, resulting in increased miRNA export, which leads to enhanced inhibition of target genes in distant cells (68).
Another question is whether non-coding lncRNAs can translate polypeptides. If this were the case, what would be their functions? One such example would be the identification of pri-microRNA encoded polypeptide 133 (miPEP133) (Figure 1). This protein, encoded by the precursor of miR-34, functions as the tumor-suppressor in nasopharyngeal and ovarian cancer cells (69). These findings suggest that polypeptides translated from lncRNA could also be found in bovine uterine and/or conceptus cells.
It has been known that the testis expresses the largest number of tissue-specific lncRNAs (70, 71). A recent publication by Mise et al. (72) has demonstrated that those previously annotated as lncRNAs in the mouse can encode for two small and sperm-specific polypeptides, Kastor and Polluks. These polypeptides are found in the outer mitochondrial membrane and directly interact with the voltage-dependent anion channel (VDAC). Mice without both Kastor and Polluks expression exhibit severely impaired male fertility due to abnormal mitochondrial sheath formation. These authors conclude that cooperative function of these polypeptides in the regulation of VDAC3 may be essential for mitochondrial sheath formation in spermatozoa (72). Moreover, the relationship between lncRNA and spermatogenesis in association with the extracellular matrix and spermatogenesis (73) and in varicocele-induced spermatogenic dysfunction has also been demonstrated (74). These observations suggest that lncRNA, whether non-coding or coding small polypeptides, may become another molecule recognized as essential for vital aspects of the reproductive process.
New Model of Pregnancy Establishment
It is generally accepted that IFNTs are confined in utero, and do not escape from the uterine lumen. Romero et al. (44) identified that the antiviral activity of IFN increases in the uterine vein during early pregnancy in sheep, of which activity on day 15 is blocked by anti-IFNT antibody. Accumulated data recently revealed that Interferon-Stimulated Gene, before (ISG) expression is up-regulated in peripheral blood mononuclear cells (PBMC) when IFNT is the only IFN present during this period (75, 76). Although further experimentation is required, uterine EVs, originating from both conceptuses and the uterine endometrium, could be taken up by uterine and conceptus cells, respectively. It is thus possible that those taken up by the endometrium enter the blood stream, which could up-regulate ISGs in PBMC.
Secretory products and their receptors in utero have been extensively studied for the last 4–5 decades. During the last decade, RNA-seq and iTRAQ analyses have been extensively used to identify key molecules that determine success or failure of early pregnancy. However, molecular, biochemical, and/or cellular mechanisms associated with early embryonic losses during the first 4 weeks of pregnancy have not been characterized. Recent data strongly suggest that in addition to those identified and characterized previously, miRNA in EVs and lncRNA must be evaluated within the context of the uterine environment required for pregnancy establishment and maintenance.
Conclusion
Much research has been conducted to identify and elucidate target genes that encode for transcription factors, cytokines and their receptors. It has become apparent that EVs containing proteins, lipids, metabolites, DNAs, and RNAs (mRNA, miRNAs, and other RNA molecules) play a role in many biological processes, including reproduction. Evidence is mounting that miRNA and lncRNA are deeply involved in the regulation of multiple biological processes required for successful conceptus implantation to the maternal endometrium and placentation. In addition to paracrine actions in utero, it is possible that these molecules encapsulated in EVs could escape from the original cells or tissues and enter circulation. When these EVs reach target cells, they undoubtedly change the paradigm of pregnancy-associated gene regulation as well as phenotypic changes in distant cells or tissues.
Author Contributions
KI had ideas of writing review manuscript on miRNA and lncRNA, which are becoming important molecules in the outcome of implantation in ruminants. YM and HF agreed with the concept and wrote parts of the manuscript. Figure was generated by YM. All authors contributed to the article and approved the submitted version.
Funding
This work was supported by Livestock Promotional Funds of Japan Racing Association (JRA) (KI) and partly by KAKENHI Grants-in-Aid for Scientific Research (16H02584 to KI) from the Japan Society for the Promotion of Science.
Conflict of Interest
The authors declare that the research was conducted in the absence of any commercial or financial relationships that could be construed as a potential conflict of interest.
Publisher's Note
All claims expressed in this article are solely those of the authors and do not necessarily represent those of their affiliated organizations, or those of the publisher, the editors and the reviewers. Any product that may be evaluated in this article, or claim that may be made by its manufacturer, is not guaranteed or endorsed by the publisher.
Acknowledgments
The authors would like to acknowledge their valuable contribution to the program: K Kusama, Tokyo University of Pharmacy and Life Sciences, K Nakamura, The University of Tokyo/Japan International Cooperation Agency (JICA), H Bai, Hokkaido University, R Bai, China Agricultural University (China), and T Sakurai, Ohu University. They would like to thank Robert Moriarty for his editorial assistance.
References
1. Théry C, Witwer KW, Aikawa E, Alcaraz MJ, Anderson JD, et al. Minimal information for studies of extracellular vesicles 2018 (MISEV2018): a position statement of the International Society for Extracellular Vesicles and update of the MISEV2014 guidelines. J Extracell Vesicles. (2018) 7:1535750. doi: 10.1080/20013078.2018.1535750
2. Pollet H, Conrard L, Cloos AS, Tyteca D. Plasma membrane lipid domains as platforms for vesicle biogenesis and shedding? Biomolecules. (2018) 8:94. doi: 10.3390/biom8030094
3. Maas SLN, Breakefield XO, Weaver AM. Extracellular vesicles: unique intercellular delivery vehicles. Trends Cell Biol. (2017) 27:172–88. doi: 10.1016/j.tcb.2016.11.003
4. Haraszti RA, Didiot MC, Sapp E, Leszyk J, Shaffer SA, Rockwell HE., et al. High-resolution proteomic and lipidomic analysis of exosomes and microvesicles from different cell sources. J Extracell Vesicles. (2016) 5:32570. doi: 10.3402/jev.v5.32570
5. Kowal J, Arras G, Colombo M, Jouve M, Morath JP, Primdal-Bengtson B, et al. Proteomic comparison defines novel markers to characterize heterogeneous populations of extracellular vesicle subtypes. Proc Natl Acad Sci USA. (2016) 113:E968–977. doi: 10.1073/pnas.1521230113
6. Record M, Silvente-Poirot S, Poirot M, Wakelam MJO. Extracellular vesicles: lipids as key components of their biogenesis and functions. J Lipid Res. (2018) 59:1316–24. doi: 10.1194/jlr.E086173
7. Imakawa K, Bai R, Fujiwara H, Ideta A, Aoyagi Y, Kusama K. Continuous model of conceptus implantation to the maternal endometrium. J Endocrinol. (2017) 233:R53–R65. doi: 10.1530/JOE-16-0490
8. Forde N, McGettigan PA, Mehta JP, O'Hara L, Mamo S, Bazer FW, et al. Proteomic analysis of uterine fluid during the pre-implantation period of pregnancy in cattle. Reproduction. (2014) 147:575–87. doi: 10.1530/REP-13-0010
9. Bauersachs S, Wolf E. Uterine responses to the preattachment embryo in domestic ungulates: recognition of pregnancy and preparation for implantation. Annu Rev Anim Biosci. (2015) 3:489–511. doi: 10.1146/annurev-animal-022114-110639
10. Liu Q, Rojas-Canales DM, Divito SJ, Shufesky WJ, Stolz DB, Erdos G, et al. Donor dendritic cell-derived exosomes promote allograft-targeting immune response. J Clin Invest. (2016) 126:2805–20. doi: 10.1172/JCI84577
11. Burns GW, Brooks KE, Spencer TE. Extracellular vesicles originate from the conceptus and uterus during early pregnancy in sheep. Biol Reprod. (2016) 94:56. doi: 10.1095/biolreprod.115.134973
12. O'Neil EV, Burns GW, Ferreira CR, Spencer TE. Characterization and regulation of extracellular vesicles in the lumen of the ovine uterus. Biol Reprod. (2020) 102:1020–32. doi: 10.1093/biolre/ioaa019
13. Mellisho EA, Velásquez AE, Nuñez MJ, Cabezas JG, Cueto JA, Fader C, et al. Identification and characteristics of extracellular vesicles from bovine blastocysts produced in vitro. PLoS ONE. (2017) 12:e0178306. doi: 10.1371/journal.pone.0178306
14. Qu P, Qing S, Liu R, Qin H, Wang W, Qiao F, et al. Effects of embryo-derived exosomes on the development of bovine cloned embryos. PLoS ONE. (2017) 12:e0174535. doi: 10.1371/journal.pone.0174535
15. Ruiz-González I, Xu J, Wang X, Burghardt RC, Dunlap KA, Bazer FW, et al. Exosomes, endogenous retroviruses and toll-like receptors: pregnancy recognition in ewes. Reproduction. (2015) 149:281–91. doi: 10.1530/REP-14-0538
16. Roberts RM, Cross JC, Leaman DW. Interferons as hormones of pregnancy. Endocr Rev. (1992) 13:432–52. doi: 10.1210/edrv-13-3-432
17. Stefanski AL, Martinez N, Peterson LK, Callahan TJ, Treacy E, Luck M, et al. Murine trophoblast-derived and pregnancy-associated exosome-enriched extracellular vesicle microRNAs: Implications for placenta driven effects on maternal physiology. PLoS ONE. (2019) 14:e0210675. doi: 10.1371/journal.pone.0210675
18. Nakamura K, Kusama K, Suda Y, Fujiwara H, Hori M, Imakawa K, et al. Emerging role of extracellular vesicles in embryo-maternal communication throughout implantation processes. Int J Mol Sci. (2020) 21:5523. doi: 10.3390/ijms21155523
19. Condrat CE, Varlas VN, Duică F, Antoniadis P, Danila CA, Cretoiu D, et al. Pregnancy-related extracellular vesicles revisited. Int J Mol Sci. (2021) 22:3904. doi: 10.3390/ijms22083904
20. Klisch K, Hecht W, Pfarrer C, Schuler G, Hoffmann B, Leiser R, et al. DNA content and ploidy level of bovine placentomal trophoblast giant cells. Placenta. (1999) 20:451–8. doi: 10.1053/plac.1999.0402
21. Dunlap K. A., Palmarini M., Spencer T. E. (2006). Ovine endogenous betaretroviruses (enJSRVs) and placental morphogenesis. Placenta. 27 (Suppl A):S135–140. doi: 10.1016/j.placenta.2005.12.009
22. Wooding FB. The role of the binucleate cell in ruminant placental structure. J Reprod Fertil Suppl. (1982) 31:31–9.
23. Yamada A, Ohtsuki K, Shiga N, Green JA, Matsuno Y, Imakawa K, et al. Epithelial-mesenchymal transition and bi- and multi-nucleated trophoblast cell formation in ovine conceptuses during the peri-implantation period. J Reprod Dev. (2022) 68:110–7. doi: 10.1262/jrd.2021-088
24. Seo H, Bazer FW, Burghardt RC, Johnson GA. Immunohistochemical examination of trophoblast syncytialization during early placentation in sheep. Int J Mol Sci. (2019) 20:4530. doi: 10.3390/ijms20184530
25. Cornelis G, Heidmann O, Degrelle SA, Vernochet C, Lavialle C, Letzelter C, et al. Captured retroviral envelope syncytin gene associated with the unique placental structure of higher ruminants. Proc Natl Acad Sci USA. (2013) 110:E828–837. doi: 10.1073/pnas.1215787110
26. Nakaya Y, Koshi K, Nakagawa S, Hashizume K, Miyazawa T. Fematrin-1 is involved in fetomaternal cell-to-cell fusion in Bovinae placenta and has contributed to diversity of ruminant placentation. J Virol. (2013) 87:10563–72. doi: 10.1128/JVI.01398-13
27. Burns G, Brooks K, Wildung M, Navakanitworakul R, Christenson LK, Spencer TE, et al. Extracellular vesicles in luminal fluid of the ovine uterus. PLoS ONE. (2014) 9:e90913. doi: 10.1371/journal.pone.0090913
28. Bai R, Bai H, Kuse M, Ideta A, Aoyagi Y, Fujiwara H, et al. Involvement of VCAM1 in the bovine conceptus adhesion to the uterine endometrium. Reproduction. (2014) 148:119–27. doi: 10.1530/REP-13-0655
29. Kusama K, Nakamura K, Bai R, Nagaoka K, Sakurai T, Imakawa K, et al. Intrauterine exosomes are required for bovine conceptus implantation. Biochem Biophys Res Commun. (2018) 495:1370–5. doi: 10.1016/j.bbrc.2017.11.176
30. Matsuno Y, Amin YA, Kusama K, Imakawa K. Formation of fibrin at sites of conceptus adhesion in the ewe. Reproduction. (2021) 161:709–20. doi: 10.1530/REP-20-0531
31. Yamakoshi S, Bai R, Chaen T, Ideta A, Aoyagi Y, Sakurai T, et al. Expression of mesenchymal-related genes by the bovine trophectoderm following conceptus attachment to the endometrial epithelium. Reproduction. (2012) 143:377–87. doi: 10.1530/REP-11-0364
32. Talbot NC, Caperna TJ, Edwards JL, Garrett W, Wells KD, Ealy AD, et al. Bovine blastocyst-derived trophectoderm and endoderm cell cultures: interferon tau and transferrin expression as respective in vitro markers. Biol Reprod. (2000) 62:235–47. doi: 10.1095/biolreprod62.2.235
33. Calle A, Toribio V, Yáñez-Mó M, Ramírez MÁ. Embryonic trophectoderm secretomics reveals chemotactic migration and intercellular communication of endometrial and circulating MSCs in embryonic implantation. Int J Mol Sci. (2021) 22:5638. doi: 10.3390/ijms22115638
34. Hayashi KG, Hosoe M, Fujii S, Kanahara H, Sakumoto R. Temporal expression and localization of vascular endothelial growth factor family members in the bovine uterus during peri-implantation period. Theriogenology. (2019) 133:56–64. doi: 10.1016/j.theriogenology.2019.04.021
35. Greening DW, Nguyen HP, Elgass K, Simpson RJ, Salamonsen LA. Human endometrial exosomes contain hormone-specific cargo modulating trophoblast adhesive capacity: Insights into endometrial-embryo interactions. Biol Reprod. (2016) 94:38. doi: 10.1095/biolreprod.115.134890
36. Vilella F, Moreno-Moya JM, Balaguer N, Grasso A, Herrero M, Martínez S, et al. Hsa-miR-30d, secreted by the human endometrium, is taken up by the pre-implantation embryo and might modify its transcriptome. Development. (2015) 142:3210–21. doi: 10.1242/dev.124289
37. Racicot K, Schmitt A, Ott T. The myxovirus-resistance protein, MX1, is a component of exosomes secreted by uterine epithelial cells. Am J Reprod Immunol. (2012) 67:498–505. doi: 10.1111/j.1600-0897.2012.01109.x
38. Giacomini E, Alleva E, Fornelli G, Quartucci A, Privitera L, Vanni VS, et al. Embryonic extracellular vesicles as informers to the immune cells at the maternal-fetal interface. Clin Exp Immunol. (2019) 198:15–23. doi: 10.1111/cei.13304
39. Nakamura K, Kusama K, Ideta A, Kimura K, Hori M, Imakawa K, et al. Effects of miR-98 in intrauterine extracellular vesicles on maternal immune regulation during the peri-implantation period in cattle. Sci Rep. (2019) 9:20330. doi: 10.1038/s41598-019-56879-w
40. Zhao G, Yang C, Yang J, Liu P, Jiang K, Shaukat A, et al. Placental exosome-mediated Bta-miR-499-Lin28B/let-7 axis regulates inflammatory bias during early pregnancy. Cell Death Dis. (2018) 9:704. doi: 10.1038/s41419-018-0713-8
41. Psychoyos A. Hormonal control of ovoimplantation. Vitam Horm. (1973) 31:201–56. doi: 10.1016/S0083-6729(08)60999-1
42. Spencer TE, Bazer FW. Biology of progesterone action during pregnancy recognition and maintenance of pregnancy. Front Biosci. (2002) 7:d1879–98. doi: 10.2741/A886
43. Ahn HW, Farmer JL, Bazer FW, Spencer TE. Progesterone and interferon tau-regulated genes in the ovine uterine endometrium: identification of periostin as a potential mediator of conceptus elongation. Reproduction. (2009) 138:813–25. doi: 10.1530/REP-09-0208
44. Romero JJ, Antoniazzi AQ, Nett TM, Ashley RL, Webb BT, Smirnova NP, et al. Temporal release, paracrine and endocrine actions of ovine conceptus-derived interferon-tau during early pregnancy. Biol Reprod. (2015) 93:146. doi: 10.1095/biolreprod.115.132860
45. Mathew DJ, Sánchez JM, Passaro C, Charpigny G, Behura SK, Spencer TE, et al. Interferon tau-dependent and independent effects of the bovine conceptus on the endometrial transcriptome. Biol Reprod. (2019) 100:365–80. doi: 10.1093/biolre/ioy199
46. Nakamura K, Kusama K, Ideta A, Imakawa K, Hori M. IFNT-independent effects of intrauterine extracellular vesicles (EVs) in cattle. Reproduction. (2020) 159:503–11. doi: 10.1530/REP-19-0314
47. Burns GW, Brooks KE, O'Neil EV, Hagen DE, Behura SK, Spencer TE, et al. Progesterone effects on extracellular vesicles in the sheep uterus. Biol Reprod. (2018) 98:612–22. doi: 10.1093/biolre/ioy011
48. Allen E, Xie Z, Gustafson AM, Carrington JC. microRNA-directed phasing during trans-acting siRNA biogenesis in plants. Cell. (2005) 121:207–21. doi: 10.1016/j.cell.2005.04.004
49. Büssing I, Yang JS, Lai EC, Grosshans H. The nuclear export receptor XPO-1 supports primary miRNA processing in C. elegans and Drosophila. EMBO J. (2010) 29:1830–9. doi: 10.1038/emboj.2010.82
50. Volk N, Shomron N. Versatility of MicroRNA biogenesis. PLoS ONE. (2011) 6:e19391. doi: 10.1371/journal.pone.0019391
51. Van Wynsberghe PM, Chan SP, Slack FJ, Pasquinelli AE. Analysis of microRNA expression and function. Methods Cell Biol. (2011) 106:219–52. doi: 10.1016/B978-0-12-544172-8.00008-6
52. Wang P, Xue Y, Han Y, Lin L, Wu C, Xu S, et al. The STAT3-binding long non-coding RNA lnc-DC controls human dendritic cell differentiation. Science. (2014) 344:310–3. doi: 10.1126/science.1251456
53. Statello L, Guo CJ, Chen LL, Huarte M. Gene regulation by long non-coding RNAs and its biological functions. Nat Rev Mol Cell Biol. (2021) 22:96–118. doi: 10.1038/s41580-020-00315-9
54. Pavani KC, Meese T, Pascottini OB, Guan X, Lin X, Peelman L, et al. Hatching is modulated by microRNA-378a-3p derived from extracellular vesicles secreted by blastocysts. Proc Natl Acad Sci USA. (2022) 119:e2122708119. doi: 10.1073/pnas.2122708119
55. Dorniak P, Bazer FW, Spencer TE. Prostaglandins regulate conceptus elongation and mediate effects of interferon tau on the ovine uterine endometrium. Biol Reprod. (2011) 84:1119–27. doi: 10.1095/biolreprod.110.089979
56. Purcell SH, Cantlon JD, Wright CD, Henkes LE, Seidel GE Jr, Anthony RV. The involvement of proline-rich 15 in early conceptus development in sheep. Biol Reprod. (2009) 81:1112–21. doi: 10.1095/biolreprod.109.076190
57. Brooks KE, Burns GW, Spencer TE. Peroxisome proliferator activator receptor gamma (PPARG) regulates conceptus elongation in sheep. Biol Reprod. (2015) 92:42. doi: 10.1095/biolreprod.114.123877
58. Brooks K, Burns G, Spencer TE. Biological roles of hydroxysteroid (11-beta) dehydrogenase 1 (HSD11B1), HSD11B2, and glucocorticoid receptor (NR3C1) in sheep conceptus elongation. Biol Reprod. (2015) 93:38. doi: 10.1095/biolreprod.115.130757
59. Ali A, Iqbal MA, Abbas MW, Bouma GJ, Anthony RV, Spencer TE, et al. Trophectoderm transcriptome analysis in LIN28 knockdown ovine conceptuses suggests diverse roles of the LIN28-let-7 axis in placental and fetal development. Cells. (2022) 11:1234. doi: 10.3390/cells11071234
60. Wang Y, Xue S, Liu X, Liu H, Hu T, Qiu X, et al. Analyses of Long Non-Coding RNA and mRNA profiling using RNA sequencing during the pre-implantation phases in pig endometrium. Sci Rep. (2016) 6:20238. doi: 10.1038/srep20238
61. Wang Q, Wang N, Cai R, Zhao F, Xiong Y, Li X, et al. Genome-wide analysis and functional prediction of long non-coding RNAs in mouse uterus during the implantation window. Oncotarget. (2017) 8:84360–72. doi: 10.18632/oncotarget.21031
62. Wang Y, Hua R, Xue S, Li W, Wu L, Kang T, et al. mRNA/lncRNA expression patterns and the function of fibrinogen-like protein 2 in Meishan pig endometrium during the preimplantation phases. Mol Reprod Dev. (2019) 86:354–69. doi: 10.1002/mrd.23109
63. Su T, Yu H, Luo G, Wang M, Zhou C, Zhang L, et al. The interaction of lncRNA XLOC-2222497, AKR1C1, and progesterone in porcine endometrium and pregnancy. Int J Mol Sci. (2020) 21:3232. doi: 10.3390/ijms21093232
64. Hong L, Hu Q, Zang X, Xie Y, Zhou C, Zou X, et al. Analysis and screening of reproductive long non-coding RNAs through genome-wide analyses of goat endometrium during the pre-attachment phase. Front Genet. (2020) 11:568017. doi: 10.3389/fgene.2020.568017
65. La Y, He X, Zhang L, Di R, Wang X, Gan S, et al. Comprehensive analysis of differentially expressed profiles of mRNA, lncRNA, and circRNA in the uterus of seasonal reproduction sheep. Genes. (2020) 11:301. doi: 10.3390/genes11030301
66. Wang Y, Hu T, Wu L, Liu X, Xue S, Lei M, et al. Identification of noncoding and coding RNAs in porcine endometrium. Genomics. (2017) 109:43–50. doi: 10.1016/j.ygeno.2016.11.007
67. Matsuno Y, Kusama K, Imakawa K. Characterization of lncRNA functioning in ovine conceptuses and endometria during the peri-implantation period. Biochem Biophys Res Commun. (2022) 594:22–30. doi: 10.1016/j.bbrc.2022.01.064
68. Garcia-Martin R, Wang G, Brandão BB, Zanotto TM, Shah S, Kumar Patel S, et al. MicroRNA sequence codes for small extracellular vesicle release and cellular retention. Nature. (2022) 601:446–51. doi: 10.1038/s41586-021-04234-3
69. Kang M, Tang B, Li J, Zhou Z, Liu K, Wang R, et al. Identification of miPEP133 as a novel tumor-suppressor microprotein encoded by miR-34a pri-miRNA. Mol Cancer. (2020) 19:143. doi: 10.1186/s12943-020-01248-9
70. Ransohoff JD, Wei Y, Khavari PA. The functions and unique features of long intergenic non-coding RNA. Nat Rev Mol Cell Biol. (2018) 19:143–57. doi: 10.1038/nrm.2017.104
71. Soumillon M, Necsulea A, Weier M, Brawand D, Zhang X, Gu H, et al. H. Cellular source and mechanisms of high transcriptome complexity in the mammalian testis. Cell Rep. (2013) 3:2179–90. doi: 10.1016/j.celrep.2013.05.031
72. Mise S, Matsumoto A, Shimada K, Hosaka T, Takahashi M, Ichihara K, et al. Kastor and Polluks polypeptides encoded by a single gene locus cooperatively regulate VDAC and spermatogenesis. Nat Commun. (2022) 13:1071. doi: 10.1038/s41467-022-28677-y
73. Chen H, Miao X, Xu J, Pu L, Li L, Han Y, et al. Alterations of mRNA and lncRNA profiles associated with the extracellular matrix and spermatogenesis in goats. Anim Biosci. (2022) 35:544–55. doi: 10.5713/ab.21.0259
74. Wang S, Kang J, Song Y, Zhang A, Pan Y, Zhang Z, et al. Long non-coding RNAs regulated spermatogenesis in varicocele-induced spermatogenic dysfunction. Cell Prolif. (2022) 17:e13220. doi: 10.1111/cpr.13220
75. Mauffré V, Grimard B, Eozenou C, Inghels S, Silva L, Giraud-Delville C, et al. Interferon stimulated genes as peripheral diagnostic markers of early pregnancy in sheep: a critical assessment. Animal. (2016) 10:1856–63. doi: 10.1017/S175173111600077X
Keywords: extracellular vesicles (EVs), miRNA – microRNA, lncRNA – long non-coding RNA, implantation, ruminants
Citation: Imakawa K, Matsuno Y and Fujiwara H (2022) New Roles for EVs, miRNA and lncRNA in Bovine Embryo Implantation. Front. Vet. Sci. 9:944370. doi: 10.3389/fvets.2022.944370
Received: 15 May 2022; Accepted: 24 June 2022;
Published: 15 July 2022.
Edited by:
Alejandro Vicente-Carrillo, Investigación y Desarrollo Magapor A.I.E., SpainReviewed by:
Eleanore V. O'Neil, Duke University, United StatesHerve Acloque, Institut National de recherche pour l'agriculture, l'alimentation et l'environnement (INRAE), France
Copyright © 2022 Imakawa, Matsuno and Fujiwara. This is an open-access article distributed under the terms of the Creative Commons Attribution License (CC BY). The use, distribution or reproduction in other forums is permitted, provided the original author(s) and the copyright owner(s) are credited and that the original publication in this journal is cited, in accordance with accepted academic practice. No use, distribution or reproduction is permitted which does not comply with these terms.
*Correspondence: Kazuhiko Imakawa, aWs0NTkxMDJAdHNjLnUtdG9rYWkuYWMuanA=
†Present address: Yuta Matsuno, Albert Einstein College of Medicine, Bronx, NY, United States