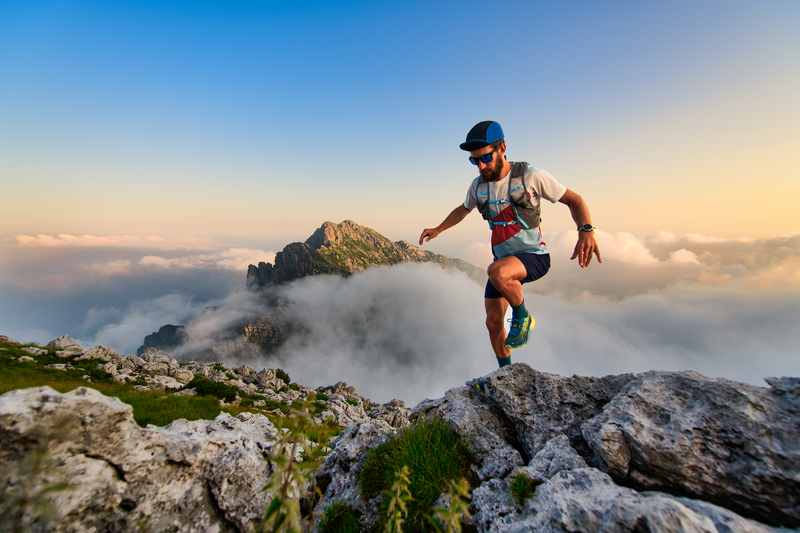
95% of researchers rate our articles as excellent or good
Learn more about the work of our research integrity team to safeguard the quality of each article we publish.
Find out more
REVIEW article
Front. Vet. Sci. , 16 May 2022
Sec. Animal Nutrition and Metabolism
Volume 9 - 2022 | https://doi.org/10.3389/fvets.2022.897302
This article is part of the Research Topic Technological Strategies to Improve Animal Health and Production View all 34 articles
Aflatoxins are the most hazardous fungal-generated secondary metabolites produced by toxigenic Aspergillus species. These toxins are frequently detected in food and feed and impose either acute or chronic effects in humans and animals, causing great public concern. Because of the adverse effects of aflatoxins, many physical, chemical, and biological decontamination approaches have been developed. However, the most commonly used procedure is the addition of adsorbent materials into aflatoxin-contaminated diets to reduce toxin absorption and distribution to blood and target organs. In recent times, sorption technology with agro-waste-based materials has appeared as a promising alternative over conventional binding agents with the benefits of low cost, higher rentability, feasibility, and exceptional efficiencies. This review is mainly focused on discussing the most important agro-waste-based materials able to adsorb aflatoxins such as pomaces, seeds, stems, hulls, peels, leaves, berries, lignins, fibers, weeds, and various horticultural byproducts. Further data of the in vitro, in vivo, and in silico efficacy of these biomaterials to adsorb and then desorb aflatoxins are given. Besides, an overview of the main characterization techniques used to elucidate the most important physical and chemical mechanisms involved in the biosorption is presented. Finally, conclusions and future research necessities are also outlined.
Mycotoxins are a group of low molecular weight substances synthesized during the secondary metabolism of toxigenic fungi. These metabolites vary from simple compounds like moniliformin to very complex chemical structures such as the macrocyclic hexapeptide mycotoxins (1). Up to now, approximately 400 mycotoxins are known (2); however, scientific attention is mainly focused on those of greatest public health and agro-economic importance, such as aflatoxins, ochratoxins, patulin, fumonisins, trichothecenes (nivalenol, deoxynivalenol, T-2 and HT-2 toxins), and zearalenone. These mycotoxins account for millions of dollars in annual losses because these compounds may exert severe adverse health effects in both humans and animals.
Aflatoxins are furanocoumarin derivatives produced by several species of Aspergillus section Flavi (3). Four principal aflatoxins are produced (Figure 1); Aspergillus togoensis synthesize aflatoxin B1 (AFB1) only; A. flavus and A. pseudotamarii synthesize aflatoxin B1 (AFB1) and aflatoxin B2 (AFB2); while A. aflatoxiformans, A. arachidicola, A. austwickii, A. cerealis, A. luteovirescens, A. minisclerotigenes, A. mottae, A. nomius, A. novoparasiticus, A. parasiticus, A. pipericola, A. pseudocaelatus, A. pseudonomius, A. sergii, and A. transmontanensis produce AFB1, AFB2, aflatoxin G1 (AFG1), and aflatoxin G2 (AFG2) (4). AFB1 has a range of biological activities such as acute toxicity, teratogenicity, mutagenicity, and carcinogenicity (5); consequently, aflatoxins, including AFB1, AFB2, AFG1, AFG2, and aflatoxin M1 (AFM1) have been classified as carcinogenic to humans (Group 1) by the International Agency for Research on Cancer (6). As many excellent reviews on aflatoxins already exist in the literature that outline the biosynthesis, ecology, metabolism, chemical structure, biological effects, toxicity, occurrence, detection, control, detoxification, and legislation, only these few lines will be presented herein.
Figure 1. 3D visualization of the chemical structure of aflatoxin B1 (AFB1), aflatoxin B2 (AFB2), aflatoxin G1 (AFG1), and aflatoxin G2 (AFG2). Created with BioRender.com.
The increasing number of reports on the presence of aflatoxins in food and feedstuffs dictates the necessity of safe, practical, and economic decontamination procedures (7). Such procedures can be categorized into physical, chemical, and biological (Figure 2). In general, physical strategies are most effective than the other methods. Among these, the adsorption of aflatoxins onto various types of materials appears to be the most extensively used procedure. In this context, inorganic binders have been reported as the most efficient materials to remove aflatoxins in vitro and in vivo (8); however, facing the relative inefficacy of inorganic binders toward other mycotoxins, biosorption has been also suggested (9). Biosorption is a property of certain biomaterials to bind and concentrate selected ions or molecules from aqueous solutions (10). One of the major advantages of biosorption is its efficacy to remove aflatoxins—totally or up to satisfactory levels—(Figure 3) along with the recycling and/or usage of waste materials and byproducts. Biosorption technology for removing mycotoxins is not new; in 1980, Smith (11) reported that alfalfa and oat fibers significantly reduced the toxic effect of zearalenone on female weanling rats. Ever since that date, an increasing number of publications on the subject have appeared in the scientific literature. Many of these articles have tried to prove the in vitro and in vivo effectiveness of different biomaterials to adsorb mycotoxins such as yeast cell wall, lactic acid bacteria, activated carbon, and polymers. However, few of them have been reported the use of agro-waste-based materials for the adsorption of aflatoxins. In light of the growing interest in this rapidly evolving subject area, we will attempt to provide an update on the most important agro-waste-based materials used to adsorb aflatoxins. Further data of the in vitro, in vivo, and in silico experiments are also given. Moreover, a focus on the main characterization techniques used to elucidate the most important physical and chemical mechanisms involved in the adsorption process is presented. Finally, conclusions and future research necessities are also outlined.
Figure 2. The most important physical, chemical, and biological decontamination technologies for aflatoxin control. Created with BioRender.com.
Figure 3. Biosorbents and their mode of action. Created with BioRender.com.
Grape (Vitis vinifera L.) is the largest fruit crop in the globe. In 2019, the grape production was over 98 million tons per year; about eighty percent was used in the winemaking industry, and the rest was for the preparation of juice, jams, and raisins (12). For this reason, the wine industry produces millions of tons of residues such as grape pomace (about 15 million tons worldwide). This plant-derived byproduct is inexpensive, available in large quantities, and is also known to contain significant amounts of valuable components that remain unexploited. Several processes have been suggested for its utilization; however, few of them have been focused on mycotoxin adsorption. The pomace (pulp and skins) obtained from Primitivo grape has been demonstrated to be an excellent aflatoxin adsorbent in vitro (13). In the research, the adsorption experiments were carried out at 37°C with 1 μg AFB1/mL. In general, the authors found that large particles yielded significantly lower adsorption uptakes; however, adsorption slightly increased by decreasing particle size (<500 μm). The maximum adsorption value recorded for AFB1 was 82% (Table 1). Moreover, the rate of aflatoxin adsorption was accomplished in a short period of time, 50% of adsorption occurred in 3 min, and the maximum was achieved in 15 min. Furthermore, the biomaterial was capable of adsorbing AFB1 to the same proportion in all tested pH values (from 3 to 9), and aflatoxin adsorption was significantly affected by the adsorbent dosage, the percentage of mycotoxins removed from neutral pH increased with increasing dosages of the biosorbent. By using the Langmuir model, the authors theoretically estimated the C50 (the adsorbent dosage to accomplish a 50% reduction of the toxin); thus, the C50 value for AFB1 was 1.2 mg/mL. Five years later, in another study from the same research group, Greco et al. (14) evaluated the ability of 51 agricultural byproducts (including fruit and grape pomaces) to adsorb AFB1. Biomaterials containing high levels of lignin, cellulose, and polyphenols were evaluated at a dosage of 10 mg/mL toward an aflatoxin working solution containing 1 μg/mL (Table 1). Significant AFB1-adsorption uptakes were reported for pomegranate byproducts (seeds and peels), artichoke, plantain peels, almond hulls, and carobs (up to 100% removal). In general, byproducts obtained from grapefruits (seeds and pomaces) adsorbed most aflatoxin. The mycotoxin-binding efficacy of grape pomaces is mainly related to the presence of micronized fibers and phenolic compounds. As the pH influences, the interaction between mycotoxin and biosorbent, Greco et al. (14) also conducted a desorption study to evaluate whether a change of pH can cause toxin release. The setup was as follows: AFB1 was adsorbed at pH 3, and then the aflatoxin-loaded biomaterials were washed with medium at pH 7. In general, grape pomaces and almond hull released <21% of the adsorbed AFB1. Additionally, Fernandes et al. (15) reported the in vitro adsorption of AFB1 by dry micronized olive pomace and grape stems. In the experiment, the biomaterials (20 mg/mL) were tested in buffer solutions containing aflatoxins (0.05, 0.5, 1, 2, 4, 6, and 10 μg/mL). Significant AFB1 adsorption efficiencies were observed (olive pomace 74% and grape stems 96%) in all tested pHs (2, 5, 7, and 8). In general, 30 mg/mL of olive pomace and 10 mg/mL of grape stems were necessary to achieve substantial adsorptions. Regarding desorption studies with buffer at pH 7, olive pomace was the biomaterial with the lowest capacity to retain the mycotoxin since desorption for AFB1 was as high as 40%. Grape stems retained the mycotoxin better; AFB1 was released only in a small amount, <5% (Table 1). Commonly, grape and olive pomaces, as well as grape stems, have good efficiencies in adsorbing mycotoxins at acid pH, and these biomaterials are also capable of retaining the adsorbed toxins when pH increases to 7. Consequently, the efficacy of two of these biomaterials (pomace from white and red grapes and almond hull) toward AFB1 was confirmed in an in vivo trial using urinary biomarkers as indicators of the absorbed mycotoxin in pigs (16). White grape pomace was the most effective biomaterial since it reduced 67% of the urinary biomarker AFM1 (Table 2). Recently, Taranu et al. (17) showed the efficacy of a grape seed waste in counteracting the toxic effects induced by AFB1 (320 ng/g) on productive parameters, plasma levels, liver damage, and intestinal tissues of pigs after weaning. The inclusion of grape seed in the aflatoxin-contaminated diet (8% w/w) enhanced the phase-I antioxidant enzymes activity, restored the pro-inflammatory cytokines and thiobarbituric acid reactive species (TBARS) levels, and improved the growth performance of the AFB1-intoxicated pigs (Table 2). These findings suggest that grape pomace and grape seed wastes are promising biomaterials in counteracting the harmful effects of AFB1 in pigs at higher inclusion levels (without adverse side effects). Unfortunately, the in vivo efficacies of olive pomace and grape stems have not been confirmed yet.
Table 1. In vitro effectiveness of different agro-waste-based materials to adsorb and desorb aflatoxins and the most important characteristic of the material related to the sorption.
Table 2. In vivo efficacy of agro-waste-based materials in counteracting the harmful effects of aflatoxins.
Banana (Musa sapientum L.) is considered the world's fourth most important agricultural crop, with a worldwide production of about 102 million tons per year (12). It is then not surprising that banana peel —which constitutes about 30–40% of the total fruit weight— is a widely available byproduct (over 40 million tons per year worldwide). Several processes have been proposed for banana peel utilization; however, few of them have been focused on mycotoxin adsorption. In this context, Shar et al. (18) showed the effectiveness of banana peel for the in vitro elimination of aflatoxins (AFB1, AFB2, AFG1, and AFG2) at a concentration of 0.5 μg/mL of each toxin. In general, oven-dried banana peel was found to be more effective in removing aflatoxins. The optimum adsorbent dosage was 60 mg/mL, and most of the adsorption occurred in 10 min, while the maximum was reached in 30 min. The sorption capacity of banana peel increased with increasing pH (from 3 to 9). At pH 8, the maximum adsorption uptakes for AFB1, AFB2, AFG1, and AFG2 were 74.9, 63.1, 76.1, and 92.8%, respectively (Table 1). Desorption studies were also conducted, adsorptions were carried out at pH 3 and 8 and desorption at pH 8 and 3, respectively. In both cases, <10% of the adsorbed toxin was desorbed; thus, the adsorption of aflatoxins onto this biomaterial was strong enough to sustain pH changes. Recently, our research group showed—for the first time—the effectiveness of unripe banana peel in removing AFB1 using a laboratory setup simulating the in vivo environment of the poultry gastrointestinal tract (19). The gastrointestinal tract compartments simulated were the crop (pH 5.2), the proventriculus (pH 1.7), and the intestinal section (pH 6.7). A typical maize-soybean meal diet contaminated with 100 μg AFB1/kg was utilized, and the biosorbent was added into the diet at 1.5% w/w (Table 1). In general, when using this multi-compartmental model, banana peel presented a low AFB1-removal capacity (28%). This considerable variation in the efficiency of the biomaterial might be mainly due to the adsorbent dosage, the differences in banana species/cultivar, the maturity stage, the feed matrix effect, the consecutive incubation periods at different pHs, as well as the enzymatic activities utilized in the multi-compartmental model. In general, the efficacy of banana peel in removing aflatoxins is related to the presence of surface functional groups, the heterogeneous microstructure, and pigment content (chlorophylls, carotenoids, and anthocyanins). Further in vitro studies regarding toxin desorption and in vivo trials to evaluate the effectiveness of banana peel, however, need to be conducted.
Formosa firethorn [Pyracantha koidzumii (Hayata) Rehder] is a spiny perennial shrub endemic in Taiwan. In many parts of the world, this one and some related species of the Rosaceae family are cultivated for decorative and walls purposes. Commonly, this plant is used in conventional medicine due to the diuretic, cardiac, and stimulant properties of its berries (27); however, limited evidence exists about other possible applications. In the scientific literature, some studies have been focused on the efficacy of P. coccinea berries for removing synthetic dyes (28–30). To date, there are only three studies —from our research group— regarding the biosorption potential of P. koidzumii against aflatoxins. Ramales-Valderrama et al. (20) reported the in vitro adsorption of AFB1 and AFB2 onto leaves, berries, and the combination of leaves and berries of P. koidzumii. The biosorbents were employed at 0.5% (w/v) in samples contaminated with 100 ng B-aflatoxins/mL. In the experiment, the ratio of AFB1 to AFB2 tested was 7:3, and adsorption was evaluated at 40°C up to 24 h. In general, the highest aflatoxin adsorption values were 86 and 82% using leaves and the combination of leaves and berries, respectively. A modest biosorption uptake (46%) was reported when using berries (Table 1). Unfortunately, most of the adsorption occurred in a long period of time (up to 6 h). Following this line, Zavala-Franco et al. (19), using a laboratory setup simulating the in vivo environment of the poultry gastrointestinal tract, showed the effectiveness of Pyracantha leaves in removing AFB1 (in this work, berries and the mixture of leaves/berries were not evaluated). All conditions used in the adsorption experiments were identical to those described in section Banana Peel. Using this multi-compartmental model, the authors indicated that the efficacy of the biomaterial was moderate; the biosorption uptake achieved was 46% (Table 1). However, neither desorption studies nor the in vivo effectiveness of these biosorbents have been reported yet. Very recently, using a theoretical perspective with density functional theory (DFT), Méndez-Albores et al. (31) showed the interaction of the AFB1 molecule and the chemical functional groups present in the surface of the P. koidzumii adsorbent. Hydroxyl, amino, carboxyl, and carbonyl groups were used as the characteristic functional groups on the biosorbent surface. In silico results showed that the carboxylate ion has the maximum binding energy with the AFB1 molecule (up to −40.2 kcal/mol); thus, the authors suggested that carboxylate ion-enriched adsorbents could be a very good option for AFB1 removal in in vitro and in vivo trials.
Lignin is the main constituent of the xylem of almost all species of terrestrial plants. Several processes have been suggested for lignin application; however, few studies on the adsorption of mycotoxins have been reported. Until today, three in vivo studies demonstrate the effectiveness of dietary lignin in alleviating the adverse effects of deoxynivalenol and zearalenone in broiler chickens (32–34). Additionally, two in vitro studies, one regarding the structure and properties of lignin as an adsorbent for T-2 toxin (35) and the other concerning the adsorption-desorption of AFB1 on lignins (21), have been reported. In the context of this review, Karmanov et al. (21) showed the adsorption potential of lignins isolated from five medicinal plants (Helianthus tuberosus, Atriplex patula, Rhododendron tomentosum, Althaea Officinalis, and Lavatera) and lignins from the wood of spruce Pícea using an in vitro setup simulating the operational conditions of the digestion in the stomach of animals. In the experiment, the sorbents were utilized at 0.1% (w/v) in samples spiked with 1 μg AFB1/mL, which were incubated at 37°C for 30 min at pH 2. In general, lignins from R. tomentosum and A. officinalis exhibited the highest AFB1-adsorption capacities. In these samples, the AFB1-uptakes reached 79.6 and 80.2%, respectively (Table 1). Lignins from Atriplex patula presented the lowest ability to adsorb AFB1 (up to 41%). Furthermore, desorption studies conducted at pH 8 confirmed that lignins from R. tomentosum and A. Officinalis adsorbed irreversible the mycotoxin (<5.3% desorption). The selectivity of certain functional groups (OH) and the capillary-porous structure of lignins were responsible for their strong association with the AFB1 molecule. Although lignins have been proven in vivo to be effective in alleviating the adverse effects of deoxynivalenol, zearalenone, and T-2 toxin, there are no reports of their use in reducing the negative effects of aflatoxins using in vivo models. Furthermore, micronized fibers consisting mainly of cellulose, hemicellulose, and lignin, have also been reported as effective mycotoxin binders. It has been reported that micronized wheat fibers (up to 2% inclusion in diet) decrease the levels of ochratoxin-A in plasma, kidney, and liver of piglets and rats (36, 37). Very recently, Adunphatcharaphon et al. (22) showed the in vitro potential of the acid-treated durian (Durio zibthinus) peel for the adsorption of AFB1. Higher adsorption efficiency toward AFB1 (98.4%) was found for this biomaterial (Table 1). The acid-treated durian peel (mainly composed of 47.2% cellulose, 9.63% hemicellulose, and 9.89% lignin) was considered a promising byproduct for aflatoxin biosorption. In general, the porous structure, larger surface area, and higher surface charge were the principal physical characteristics of the acid-treated durian peel to remove aflatoxins. Nevertheless, other authors have suggested that the efficiency of cellulose products to adsorb AFB1 resulted not significant when proven in an in vitro model simulating the digestion of pigs (23) (Table 1).
Aloe vera (A. barbadensis Miller) is a drought-resistant plant of the Liliaceae family. This plant has been used for medicinal purposes for over 5,000 years. Aloe vera gel is the mucilaginous extract of the leafy pulp, which is usually separated by scratching. It is well-known that the gel contains many phytochemicals (vitamins, minerals, enzymes, polysaccharides, and phenolic compounds) and has been claimed to have several curative and therapeutic properties (38). Although Aloe vera gel has been reported to exhibit several attractive properties (virucidal, bactericidal, and fungicidal) as well as the adsorbent ability against organic and inorganic pollutants, in the literature, there is only one report—from our research group—of its use as a biosorbent against AFB1 (19). In the work, the gel of matured leaves of A. barbadensis Miller was separated, pasteurized, and further concentrated to get a fine powder. The effectiveness of this powder in removing AFB1 was tested in a laboratory setup simulating the in vivo environment of the poultry gastrointestinal tract (all conditions used in the adsorption experiments were identical to those described in section Banana Peel). In general, Aloe vera powder limited the availability of AFB1 in the intestinal segment up to 69% (Table 1). The high negative-charged surface on the biosorbent was linked to the high sorption uptake due to enhancements of attractive forces between AFB1 and the biomaterial. However, further in vivo trials are needed to demonstrate its efficacy in reducing the toxic consequences of aflatoxins. Also, desorption studies will provide valuable information about the potential benefits of Aloe vera powder as a mycotoxin adsorbent biomaterial.
Facing the relative inefficacy of some biosorbents toward mycotoxins, Nava-Ramírez et al. (24) investigated—for the first time—the in vitro potential of lettuce (Lactuca sativa L.) wastes and field horsetail (Equisetum arvense L.) in removing AFB1. The adsorption of AFB1 (190 ng/mL) was explored at two sorbent contents (0.5% and 0.1% w/v) and three pHs (3, 5, and 7). Adsorption was carried out at 40 °C for 2 h (Table 1). In general, at 0.5% (w/v), AFB1 was well-adsorbed by both biomaterials (70 to 100%). However, at 0.1% (w/v), lettuce showed the highest ability against AFB1 removal, the AFB1 biosorption percentage was 95% (at neutral pH). Adsorption was mainly due to the interaction of the AFB1 molecule with the functional groups of the biosorbents as well as to the formation of AFB1-chlorophyll complexes. Thus, the authors concluded that lettuce wastes could have significant potential for the removal of AFB1 in some gastrointestinal tract compartments at low inclusion levels. One year later, in another study by the same research group, Vázquez-Durán et al. (25) evaluated the adsorbent capacity of agricultural residues from kale (Brassica oleracea L.) using a dynamic in vitro model that simulated the conditions of the gastrointestinal tract of poultry. The biosorbent was added to a contaminated poultry feed (100 μg AFB1/kg) at a content of 0.5% (w/w). According to the adsorption results, the maximum adsorption capacity of kale was 93.6% in the intestinal section (Table 1). A biosorbent prepared from lettuce agro-waste was used as a reference, presenting a significantly lower adsorption percentage (83.7%). The researchers pointed out that adsorption of AFB1 may be mediated by simultaneous mechanisms, such as: non-electrostatic interactions (hydrophobic interactions, dipole-dipole interactions, and hydrogen bonds) and electrostatic interactions (ionic attractions). In addition, the authors also concluded that the formation of AFB1-chlorophyll complexes improved the rate of AFB1 adsorption. However, as recommended by Ramales-Valderrama et al. (20), biosorbents with high binding affinity for AFB1 in vitro need to be further tested in vivo to validate their efficacy to reduce the toxic effects of aflatoxins.
Perali et al. (26) investigated the efficiency of the seaweed Lithothamnium calcareum (Pallas) Areschoug to remove AFB1. The study was conducted using two models, one in vitro and the other in vivo; the latter focused on evaluating the capacity of the adsorbent to prevent the toxic effects of AFB1 in broilers. The adsorption of AFB1 (1 μg AFB1/mL) was explored in vitro at four sorbent contents (0.05, 0.1, 0.15 and 0.2%) and two pHs (3 and 6). In general, highest percentages of AFB1 removal were achieved at a content of 0.2% in both evaluated pHs. In these samples, the AFB1-uptakes reached 77.6 and 77.4%, respectively (Table 1). Regarding the in vivo experiment, it was observed that the seaweed (0.2% inclusion) improved productive parameters (live weight, weight gain, and feed conversion ratio), reduced the relative weight of the liver and the macroscopic and microscopic changes caused by the AFB1 intoxication, and improved some biochemical parameters in birds that received a diet contaminated with 1,018 μg AFB1/g feed (Table 2).
FTIR spectroscopy is one of the most used techniques to characterize biosorbents, usually before and after mycotoxin adsorption. This methodology is relatively simple, reproducible, non-destructive, and only small quantities of biomaterials—without any further preparation—are required. Generally, FTIR spectroscopy provides information at the molecular level allowing investigation of functional groups, bonding types, and molecular conformations. In the FTIR spectra, most of the FTIR bands are relatively sharp and can be correlated with single bonds or particular functional groups. The position of a band is expressed into a plot with wavenumber (cm−1) on the x-axis and intensity on the y-axis. Intensity could be measured both in transmittance or absorbance modes. In the literature reviewed, most of the spectra were collected to recognize the main functional groups of the tested biosorbents. However, in the work of Ramales-Valderrama et al. (20), the FTIR spectra were acquired in order to elucidate the possible interaction mechanism between the AFB1 molecule and the biosorbents. In general, the authors indicated that a shift in the frequency (associated to an energy change) or a change in the band intensity confirm the involvement of a specific functional group in the aflatoxin binding. Table 3 summarizes the band assignments of the principal vibrational modes in the biomaterials used to remove aflatoxins. Biosorbents are mainly constituted of proteins, carbohydrates, lipids, and phytochemicals (curcuminoids, flavonoids, alkaloids, steroids, terpenoids, saponins, phenolics, glucosides, and chlorophylls). All of these components have several functional groups (hydroxyl, amino, carboxyl, carboxylate, amide, phosphate, ester, and ketone), which can be partially responsible for the biosorption of aflatoxins (Table 3). For instance, it has been reported that the hydroxyl, amino, carboxyl, and ester groups can efficiently establish hydrogen bonds with the oxygen atoms of the ether, carbonyl, and methoxy groups in the AFB1 molecule (25). Recently, theoretical infrared spectrophotometric studies of the adsorption of B-aflatoxins onto Pyracantha biosorbents showed that the carboxylate ion has the maximum binding energy with the AFB1 molecule. These in silico results imply—but do not yet prove—that an enriched biosorbent with carboxylate groups could increase the AFB1 adsorption (31). Finally, as an important remark, researchers might take into consideration that the main disadvantage of the FTIR technique is that several materials completely absorb IR radiation; therefore, it may be impossible to get reliable results.
Table 3. Overview of the most relevant chemical functional groups responsible for the biosorption of aflatoxins.
The UV-Vis diffuse reflectance spectroscopy (DRS) is a useful technique for the characterization of biomaterials. Diffuse reflectance occurs as UV-Vis-light enters the sample, interacts with its components, and scatters backward. As a consequence, this technique provides information related to structural, physical, and chemical properties of the biomaterials and offers exceptional versatility because of its high sensitivity. Diffuse reflectance measurements make it possible to quickly and non-destructively evaluate—in situ—the content of chlorophylls, carotenoids, and anthocyanins in certain biomaterials. The most representative pigment in biosorbents is chlorophyll, of which the most common and abundant species are chlorophylls a and b. By wet chemical methods, a complex destructive procedure based on extraction and separation with organic solvents and spectrophotometric analysis is necessary for chlorophyll estimation. However, reflectance spectroscopy has been widely used for non-destructive estimation of chlorophylls in plant tissues. Limited information is available in the literature on the use of UV-Vis reflectance spectroscopy for pigment assessment in biosorbents used for aflatoxin removal. To date, there are only two reports using Pyracantha leaves, banana peel, Aloe vera, lettuce, and kale biosorbents (Table 1). Zavala-Franco et al. (19) and Vázquez-Durán et al. (25) found that almost all of the biosorbents presented the characteristic absorption bands at 677 and 650 nm, which correspond to chlorophyll a and chlorophyll b, respectively. Biosorbents also showed absorbance bands from 425 to 485 nm and at 550 nm indicative of the presence of carotenoids and anthocyanins, respectively. Furthermore, many studies have indicated that chlorophylls can form strong non-covalent complexes in vitro with AFB1 independent of temperature or pH. Consequently, the formation of a complex with the aflatoxins (via electrostatic, π-π orbital interactions, and/or hydrogen bonding) may be expected to improve the rate of AFB1 uptake by the biosorbents containing significant amounts of chlorophylls (24, 25).
The adsorption of mycotoxins to a biomaterial surface in aqueous media could be based on a set of chemical and physical mechanisms, including hydrogen bonding, electrostatic attraction, ion exchange, chelation, precipitation, complexation, among others. Apparently, electrostatic interaction is the most important phenomenon during mycotoxin adsorption. Thus, zeta potential is important for the characterization of electrochemical surface properties since the electrokinetic potential at the electrical double layer is associated with the surface charge of colloidal suspensions. Both surface charge and environmental conditions—pH and ions in the medium—influence the zeta potential. Commonly, the zeta potential is determined by the micro-electrophoresis technique. In this procedure, a voltage is applied across a pair of opposite gold-plated electrodes; as a result, charged particles are attracted to the oppositely charged electrode and their velocity measured. The SI unit for electrophoretic mobility is μm cm/V s, since it is a velocity (μm/s) per field strength (V/cm). The electrophoretic mobility is the direct measurement from which zeta potential can be derived using the Helmholtz-Smoluchowski, Debye-Hückel, or Henry functions. Biosorbents with a zeta potential value between −10 and +10 mV are neutral, while those with zeta potentials >+30 mV or <-30 mV are strongly cationic or strongly anionic, respectively. In the literature, various research groups have reported the zeta potential of different biosorbents used for the removal of mycotoxins (19, 20, 22, 39). Table 4 summarizes the zeta potential values of the biomaterials used to adsorb aflatoxins. Considering that the interaction between aflatoxins and the biosorbent would be mainly electrostatic, biosorbents exhibiting higher zeta potential values are most adequate to be used in the adsorption due to the improvement of attractive forces between aflatoxin molecules and the surface of biomaterials. According to the reviewed literature, lettuce and field horsetail were the biosorbents with the high negative-charged surfaces (Table 4). However, up to now, none of the biosorbents shown in Table 4 have been tested in vivo.
The point of zero charge (pHpzc) gives useful information about the surface charge of the biosorbents. It is well-known that pH influences sorption, mainly because pH governs the ionization of functional groups. In consequence, the pH at which the sorbent surface charge become equal to zero is defined as the point of zero charge. In other words, the charge of the positive surface sites is equal to that of the negative ones. It has been suggested that if pH < pHpzc, the surface of the biomaterial will be positively charged, and if pH > pHpzc, the surface will be negatively charged (40). Several methodologies have been reported for the determination of pHpzc, such as the potentiometric mass titration, the mass titration, and the immersion technique. In the literature, all of the biosorbents used for the adsorption of aflatoxins were characterized relative to its pHpzc by using the immersion technique. As an example, Akar et al. (39) reported pHpzc values of 1.9 and 2.7 for natural and modified sugar beet pulp wastes, respectively. The chemically modified biosorbent was used as an efficient material for zearalenone removal. Table 5 summarizes the pHzpc values of the biomaterials used for the in vitro adsorption of aflatoxins. Most of the biomaterials summarized in Table 5 have good efficiencies in adsorbing aflatoxins in vitro, but none of them have confirmed their effectiveness in in vivo trials. Considering the pH in the different compartments of the gastrointestinal tract of poultry, biosorbents with low pHpzc could be the most suitable to be used for the adsorption of aflatoxins when using in vivo models. Consequently, it would be interesting to study the in vivo effectiveness of the modified sugar beet pulp waste to remove aflatoxins since this biomaterial has the lowest pHpzc reported in the literature.
Table 5. Point of zero charge (pHpzc) values of the agro-waste-based materials used for aflatoxins adsorption.
It is well-known that the adsorption properties of biomaterials may also be associated with both the structural and chemical features. In this context, the scanning electron microscopy (SEM) is one of the most versatile techniques available for the examination and analysis of the microstructure and morphology. Commonly, dried biosorbents must be mounted on special holders using a conductive carbon double-sided sticky tape. To increase image contrast and to avoid undesirable charging effects, it is necessary to coat the sample with a thin layer of a high electrical and thermal conductivity material such as gold, platinum, or carbon. Studies reporting the utilization of the SEM analysis for comparing the surface of biosorbents before and after aflatoxin adsorption are still meager (20). In the literature, most of the studies only report the morphological structure of the developed biosorbents previous aflatoxin exposure (18, 22). In general, more pores or cavities on the surface of the biomaterials provide higher capacities for aflatoxin adsorption. On the other hand, energy dispersive X-ray spectroscopy (EDS) is a chemical microanalysis technique typically performed in conjunction with SEM. In this technique, the atoms on the surface are excited by an electron stream, causing X-rays to be emitted. The energy of the X-ray is distinctive of the element from which the X-ray was produced. The chemical composition of certain biomaterials has been reported. Zavala-Franco et al. (19) evaluated the elemental composition of three biosorbents used for the removal of AFB1. The authors found that the main elements of banana peel, Pyracantha leaves, and Aloe powder were C and O, corresponding to 97.3, 99.2, and 85.7% of the total weight, respectively. They also observed other minor elements such as Na, Mg, Al, Si, P, S, Cl, K, and Ca. Adunphatcharaphon et al. (22) showed the elementary composition of the acid-treated durian peel employed as an aflatoxin binder. EDS analysis also revealed that C and O were the main elements that constitute the pristine biomaterial. However, the acidic treatment affected the elemental composition increasing the proportion of C, enhancing its AFB1 binding efficacy. Summarizing, surface characterization (morphology and microstructure) can be accomplished by SEM. When scanning electron microscope is accessorized with EDS, chemical microanalysis can also be conducted with 1–3% accuracy (41).
X-ray diffraction (XRD) is another non-destructive characterization technique suitable to study phase, structure, orientation, and other structural features such as crystallite size, unit cell dimensions, crystallinity, and crystal defects. In this technique, diffraction patterns are formed by constructive interference of a monochromatic beam of X-rays scattered at different angles. In the analyzed samples, amorphous regions generate broad peaks, whereas crystalline regions produce sharp peaks. In general, X-ray diffractograms are collected using CuKα radiation (λ = 0.15406 nm) over the 2θ range (10 to 100 degrees) with a fixed power source. The XRD patterns of biosorbents are rarely presented in the literature due to the fact that most of the biomaterials used for mycotoxin adsorption are essentially carbonaceous. However, the XRD patterns for banana peel and Pyracantha leaves showed a distinctive amorphous structure based on broad diffraction peaks (19). Both biosorbents showed a strong diffraction peak at 20° (2θ) and few small diffraction peaks at around 5.6°, 14.8°, 17.3°, 22.8°, and 24.0°. These diffraction peaks were associated with the structure of semi-crystalline starch. Aloe powder also presented an amorphous phase and crystalline peaks for sylvite (KCl) and halite (NaCl). Moreover, the authors reported the degree of crystallinity of the three tested biosorbents. In general, the degree of crystallinity of banana peel, Pyracantha leaves, and Aloe powder differed significantly, yielding values of 19.1, 10.9, and 44.7%, respectively. In the sorption experiments, Aloe vera powder—the biomaterial with the highest crystallinity index—showed the maximum efficiency against AFB1 removal (68.5%). Although reduction in crystallinity leads to more reactive samples (42), the adsorption of aflatoxins depends on several characteristics of the biosorbents such as the functional group/type (amount), pigment content, surface charge, microstructure, morphology, elemental composition, degree of crystallinity, among others.
Aflatoxins are inevitable contaminants of food and feed. Because of the adverse effects of aflatoxins on human and animal health, effective, practical, and inexpensive decontamination protocols are highly desirable. Recently, biosorption has received extensive attention among scientists for aflatoxin decontamination due to the low cost and the extraordinary efficiency of the biosorbents. Byproducts such as grape and olive (pomaces, seeds, and stems), banana peel, Formosa firethorn (leaves and berries), lignins, micronized fibers, durian peel, seaweeds, Aloe vera powder, lettuce, kale, and field horsetail have received particular attention for the removal of aflatoxins owing their abundance worldwide. As a result, several in vitro, in vivo, and in silico methodologies have been applied to evaluate the potential of these biosorbents in removing or reducing the impact of aflatoxins. A number of factors influencing the adsorption such as physical or chemical modification of the biomaterials, particle size, contact time, pH, temperature, biosorbent dosage, and the aflatoxin concentration, were further reviewed. We found the following:
• Structural changes following physical or chemical modifications of the biosorbents may explain their higher efficiencies in adsorbing aflatoxins.
• Biosorbents with large particles yielded lower adsorption uptakes. However, aflatoxin adsorption significantly increased by decreasing particle size.
• Generally, the rate of aflatoxin adsorption was accomplished in a short period of time (from 3 up to 30 min). This fast kinetic is highly desirable for practical and commercial applications.
• Various kinds of biosorbents have good efficiencies in adsorbing aflatoxins at acid pHs and were also capable of retaining most of the toxins when pH increases to 7, although some exceptions were observed.
• The biosorbents were efficient at temperatures between 37 and 40°C, which is indicative of their ability to adsorb aflatoxins when using in vivo trials.
• Further increments in the amount of biosorbents improve the uptake of aflatoxins due to the existence of more adsorption sites.
• Some biosorbents exhibited higher percentages of aflatoxin removal at lower toxin concentrations and considerable uptake capacities at higher aflatoxin concentrations (Figure 4).
• Generally, when using in vitro digestion procedures simulating the environment in the gastrointestinal tract, the tested biosorbents showed low removal efficiencies toward aflatoxins.
• In in vivo trials, some biosorbents counteracted the harmful effects of AFB1, but these were used at higher inclusion levels (up to 8% w/w).
• Several characterization techniques such as FTIR, UV-Vis DRS, ζ-potential, pHzpc, SEM, EDS, and XRD have been successfully used to explain the possible mechanisms involved in the biosorption of aflatoxins.
Figure 4. The effectiveness of some biosorbents to remove aflatoxins. Adsorption efficiency: red (low), yellow (moderate) and green (high). Created with BioRender.com.
Despite the effectiveness of the biosorbents for the decontamination of aflatoxins, future research should be concentrated on the following topics:
• The effectiveness of biosorbents for removing aflatoxins needs to be extensively studied using dynamic models that simulate the conditions in the gastrointestinal tract on ways of minimizing the use of experimental animals.
• Biosorbents can be tested (in vitro) at low inclusion levels (0.1%, w/v) and challenged with more realistic levels of aflatoxins to make these materials very competitive in the commercial sorbent market.
• Taking into account that multi-exposure to mycotoxins is the most likely scenario, multi-mycotoxin adsorption experiments should be conducted in order to evaluate competitive biosorption.
• Because of the complex nature of the biosorbents, highly selective and sensitive analytical characterization techniques are necessary for a systematic characterization (before and after aflatoxin adsorption).
• Agro-waste-based sorbents such as cereal fibers as well as pulp and peels of some fruits may contain mycotoxins; consequently, these biomaterials need to be further analyzed before using them as mycotoxin binders.
• Novel approaches for the preparation of biosorbents from other agricultural wastes or byproducts are highly encouraged.
• Finally, in screening for new biosorbents, larger amounts of hydroxyl and carboxyl groups, high number of hydrophobic groups, higher amounts of pigments (chlorophylls), higher negative surface charge, lower pHpzc values, porous microstructure, and larger surface area seem to be the most important particularities to predict the ability of agro-waste-based materials to bind aflatoxins.
Considerations on these topics would help advance the search for near-term future commercial applications of unconventional, eco-friendly, and efficient aflatoxin binders of natural origin.
AV-D and AM-A: conceptualization. MN-R: investigation. AV-D: data curation. AM-A: writing—original draft preparation. AV-D and GT-I: writing—review and editing. All authors have read and agreed to the published version of the manuscript.
This research was funded by UNAM-PAPIIT grant number IN207920.
The authors declare that the research was conducted in the absence of any commercial or financial relationships that could be construed as a potential conflict of interest.
All claims expressed in this article are solely those of the authors and do not necessarily represent those of their affiliated organizations, or those of the publisher, the editors and the reviewers. Any product that may be evaluated in this article, or claim that may be made by its manufacturer, is not guaranteed or endorsed by the publisher.
MN-R acknowledges CONACYT for the Ph.D scholarship (866355).
1. Battilani P, Gualla A, Dall'Asta C, Pellacani C, Galaverna G, Giorni P, et al. Phomopsins: An overview of phytopathological and chemical aspects, toxicity, analysis and occurrence. World Mycotoxin J. (2011) 4:345–59. doi: 10.3920/WMJ2011.1302
2. Bhat R, Rai RV, Karim AA. Mycotoxins in food and feed: present status and future concerns. Compr Rev Food Sci F. (2010) 9:57–81. doi: 10.1111/j.1541-4337.2009.00094.x
3. Varga J, Frisvad JC, Samson R. Two new aflatoxin producing species, and an overview of Aspergillus section Flavi. Stud Mycol. (2011) 69:57–80. doi: 10.3114/sim.2011.69.05
4. Frisvad JC, Hubka V, Ezekiel C, Hong SB, Nováková A, Chen A, et al. Taxonomy of Aspergillus section Flavi and their production of aflatoxins, ochratoxins and other mycotoxins. Stud Mycol. (2019) 93:1–63. doi: 10.1016/j.simyco.2018.06.001
5. McLean M, Dutton MF. Cellular interactions and metabolism of aflatoxin: an update. Pharmacol Ther. (1995) 65:163–92. doi: 10.1016/0163-7258(94)00054-7
6. Ostry V, Malir F, Toman J, Grosse Y. Mycotoxins as human carcinogens—the IARC Monographs classification. Mycotoxin Res. (2017) 33:65–73. doi: 10.1007/s12550-016-0265-7
7. Mahato DK, Lee KE, Kamle M, Devi S, Dewangan K, Kumar P, et al. Aflatoxins in food and feed: an overview on prevalence, detection and control strategies. Front Microbiol. (2019) 10:2266. doi: 10.3389/fmicb.2019.02266
8. Phillips TD, Kubena LF, Harvey RB, Taylor DH, Heidelbaugh ND. Hydrated sodium calcium aluminosilicate: a high affinity sorbent for aflatoxin. Poult Sci. (1988) 67:243–7. doi: 10.3382/ps.0670243
9. Jouany JP. Methods for preventing, decontaminating and minimizing the toxicity of mycotoxins in feeds. Animal Feed Sci Tech. (2007) 137:342–62. doi: 10.1016/j.anifeedsci.2007.06.009
11. Smith T. Influence of dietary fiber, protein and zeolite on zearalenone toxicosis in rats and swine. J Anim Sci. (1980) 50:278–85. doi: 10.2527/jas1980.502278x
12. Faostat F. Statistical Databases. Rome: Food and Agriculture Organization of the United Nations (2019).
13. Avantaggiato G, Greco D, Damascelli A, Solfrizzo M, Visconti A. Assessment of multi-mycotoxin adsorption efficacy of grape pomace. J Agr Food Chem. (2014) 62:497–507. doi: 10.1021/jf404179h
14. Greco D, D'Ascanio V, Santovito E, Logrieco AF, Avantaggiato G. Comparative efficacy of agricultural by-products in sequestering mycotoxins. J Sci Food Agr. (2019) 99:1623–34. doi: 10.1002/jsfa.9343
15. Fernandes JM, Calado T, Guimarães A, Rodrigues MAM, Abrunhosa L. In vitro adsorption of aflatoxin B 1, ochratoxin A, and zearalenone by micronized grape stems and olive pomace in buffer solutions. Mycotoxin Res. (2019) 35:243–52. doi: 10.1007/s12550-019-00349-9
16. Gambacorta L, Pinton P, Avantaggiato G, Oswald IP, Solfrizzo M. Grape pomace, an agricultural by-product reducing mycotoxin absorption: in vivo assessment in pig using urinary biomarkers. J Agric Food Chem. (2016) 64:6762–71. doi: 10.1021/acs.jafc.6b02146
17. Taranu I, Marin DE, Palade M, Pistol GC, Chedea VS, Gras MA, et al. Assessment of the efficacy of a grape seed waste in counteracting the changes induced by aflatoxin B1 contaminated diet on performance, plasma, liver and intestinal tissues of pigs after weaning. Toxicon. (2019) 162:24–31. doi: 10.1016/j.toxicon.2019.02.020
18. Shar ZH, Fletcher MT, Sumbal GA, Sherazi STH, Giles C, Bhanger MI, et al. Banana peel: an effective biosorbent for aflatoxins. Food Addict Contam : Part A. (2016) 33:849–60. doi: 10.1080/19440049.2016.1175155
19. Zavala-Franco A, Hernández-Patlán D, Solís-Cruz B, López-Arellano R, Tellez-Isaias G, Vázquez-Durán A, et al. Assessing the aflatoxin B1 adsorption capacity between biosorbents using an in vitro multicompartmental model simulating the dynamic conditions in the gastrointestinal tract of poultry. Toxins. (2018) 10:484. doi: 10.3390/toxins10110484
20. Ramales-Valderrama RA, Vázquez-Durán A, Méndez-Albores A. Biosorption of B-aflatoxins using biomasses obtained from formosa firethorn [Pyracantha koidzumii (Hayata) Rehder]. Toxins. (2016) 8:218. doi: 10.3390/toxins8070218
21. Karmanov AP, Kanarsky AV, Kanarskaya ZA, Kochevar LS, Semenov EI, Bogdanovich NI, et al. In vitro adsorption-desorption of aflatoxin B1 on Pepper's lignins isolated from grassy plants. Int J Biol Macromol. (2020) 144:111–7. doi: 10.1016/j.ijbiomac.2019.12.081
22. Adunphatcharaphon S, Petchkongkaew A, Greco D, D'Ascanio V, Visessanguan W, Avantaggiato G. The effectiveness of durian peel as a multi-mycotoxin adsorbent. Toxins. (2020) 12:108. doi: 10.3390/toxins12020108
23. Kong C, Shin SY, Kim BG. Evaluation of mycotoxin sequestering agents for aflatoxin and deoxynivalenol: an in vitro approach. SpringerPlus. (2014) 3:346. doi: 10.1186/2193-1801-3-346
24. Nava-Ramírez MJ, Salazar AM, Sordo M, López-Coello C, Téllez-Isaías G, Méndez-Albores A, et al. Ability of low contents of biosorbents to bind the food carcinogen aflatoxin B1 in vitro. Food Chem. (2021) 345:128863. doi: 10.1016/j.foodchem.2020.128863
25. Vázquez-Durán A, Nava-Ramírez MJ, Hernández-Patlán D, Solís-Cruz B, Hernández-Gómez V, Téllez-Isaías G, et al. Potential of kale and lettuce residues as natural adsorbents of the carcinogen aflatoxin B1 in a dynamic gastrointestinal tract-simulated model. Toxins. (2021) 13:771. doi: 10.3390/toxins13110771
26. Perali C, Magnoli AP, Aronovich M, Rosa CADR, Cavaglieri LR. Lithothamnium calcareum (Pallas) Areschoug seaweed adsorbs aflatoxin B1 in vitro and improves broiler chicken's performance. Mycotoxin Res. (2020) 36:371–9. doi: 10.1007/s12550-020-00402-y
27. Kowalewski Z, Mrugasiewicz K. Neue flavanonheteroside in Crataegus phenophyrum. Planta Med. (1971) 19:311–7. doi: 10.1055/s-0028-1099647
28. Akar T, Anilan B, Gorgulu A, Akar ST. Assessment of cationic dye biosorption characteristics of untreated and non-conventional biomass: Pyracantha coccinea berries. J Hazard Mater. (2009) 168:1302–9. doi: 10.1016/j.jhazmat.2009.03.011
29. Akar T, Celik S, Akar ST. Biosorption performance of surface-modified biomass obtained from Pyracantha coccinea for the decolorization of dye contaminated solutions. Chem Eng J. (2010) 160:466–72. doi: 10.1016/j.cej.2010.03.047
30. Ari AG, Celik S. Biosorption potential of Orange G dye by modified Pyracantha coccinea: batch and dynamic flow system applications. Chem Eng J. (2013) 226:263–70. doi: 10.1016/j.cej.2013.04.073
31. Méndez-Albores A, Escobedo-González R, Aceves-Hernández JM, García-Casillas P, Nicolás-Vázquez MI, Miranda-Ruvalcaba R, et al. Theoretical study of the adsorption process of B-aflatoxins using Pyracantha koidzumii (Hayata) Rehder biomasses. Toxins. (2020) 12:283. doi: 10.3390/toxins12050283
32. Grešáková L, Borutová R, Faix Š, Plachá I, Cobanová K, Košíková B, et al. Effect of lignin on oxidative stress in chickens fed a diet contaminated with zearalenone. Acta Vet Hung. (2012) 60:103–14. doi: 10.1556/avet.2012.009
33. Klapáčová K, Faixová Z, Faix Š, Miklósová L, Leng L. Effects of feeding wheat naturally contaminated with Fusarium mycotoxins on blood biochemistry and the effectiveness of dietary lignin treatment to alleviate mycotoxin adverse effects in broiler chickens. Acta Vet. (2011) 61:227–37. doi: 10.2298/AVB1103227K
34. Revajová V, Levkut M, Levkutová M, Borutová R, Grešaková L, Košiková B, et al. Effect of lignin supplementation of a diet contaminated with Fusarium mycotoxins on blood and intestinal lymphocyte subpopulations in chickens. Acta Vet Hung. (2013) 61:354–65. doi: 10.1556/avet.2013.023
35. Kanarskaya Z, Kanarskii A, Semenov E, Karmanov A, Kochevar L, Bogdanovich N, et al. Structure and properties of lignin as an adsorbent for mycotoxin T-2. Chem Nat Compd. (2016) 52:1073–7. doi: 10.1007/s10600-016-1864-4
36. Aoudia N, Call P, Grosjean F, Larondelle Y. Effectiveness of mycotoxin sequestration activity of micronized wheat fibres on distribution of ochratoxin A in plasma, liver and kidney of piglets fed a naturally contaminated diet. Food Chem Toxicol. (2009) 47:1485–9. doi: 10.1016/j.fct.2009.03.033
37. Aoudia N, Tangni E, Larondelle Y. Distribution of ochratoxin A in plasma and tissues of rats fed a naturally contaminated diet amended with micronized wheat fibres: effectiveness of mycotoxin sequestering activity. Food Chem Toxicol. (2008) 46:871–8. doi: 10.1016/j.fct.2007.10.029
38. Langmead L, Makins R, Rampton D. Anti-inflammatory effects of aloe vera gel in human colorectal mucosa in vitro. Aliment PharmTher. (2004) 19:521–7. doi: 10.1111/j.1365-2036.2004.01874.x
39. Akar T, Güray T, Yilmazer DT, Tunali Akar S. Biosorptive detoxification of zearalenone biotoxin by surface-modified renewable biomass: process dynamics and application. J Sci Food Agric. (2019) 99:1850–61. doi: 10.1002/jsfa.9379
40. Lim LB, Priyantha N, Tennakoon D, Chieng HI, Dahri MK, Suklueng M. Breadnut peel as a highly effective low-cost biosorbent for methylene blue: equilibrium, thermodynamic and kinetic studies. Arab J Chem. (2017) 10:S3216–28. doi: 10.1016/j.arabjc.2013.12.018
41. Karimi K, Taherzadeh MJ. A critical review of analytical methods in pretreatment of lignocelluloses: composition, imaging, and crystallinity. Bioresour Technol. (2016) 200:1008–18. doi: 10.1016/j.biortech.2015.11.022
42. Lopičić ZR, Stojanović MD, Marković SB, Milojković JV, Mihajlović ML, Radoičić TSK, et al. Effects of different mechanical treatments on structural changes of lignocellulosic waste biomass and subsequent Cu (II) removal kinetics. Arab J Chem. (2019) 12:4091–103. doi: 10.1016/j.arabjc.2016.04.005
Keywords: aflatoxins, agro-waste-based materials, biosorption, characterization techniques, decontamination
Citation: Vázquez-Durán A, Nava-Ramírez MdJ, Téllez-Isaías G and Méndez-Albores A (2022) Removal of Aflatoxins Using Agro-Waste-Based Materials and Current Characterization Techniques Used for Biosorption Assessment. Front. Vet. Sci. 9:897302. doi: 10.3389/fvets.2022.897302
Received: 16 March 2022; Accepted: 25 April 2022;
Published: 16 May 2022.
Edited by:
Tugay Ayasan, Osmaniye Korkut Ata University, TurkeyReviewed by:
Işil Var, Cukurova University, TurkeyCopyright © 2022 Vázquez-Durán, Nava-Ramírez, Téllez-Isaías and Méndez-Albores. This is an open-access article distributed under the terms of the Creative Commons Attribution License (CC BY). The use, distribution or reproduction in other forums is permitted, provided the original author(s) and the copyright owner(s) are credited and that the original publication in this journal is cited, in accordance with accepted academic practice. No use, distribution or reproduction is permitted which does not comply with these terms.
*Correspondence: Abraham Méndez-Albores, YWxib3Jlc0B1bmFtLm14
Disclaimer: All claims expressed in this article are solely those of the authors and do not necessarily represent those of their affiliated organizations, or those of the publisher, the editors and the reviewers. Any product that may be evaluated in this article or claim that may be made by its manufacturer is not guaranteed or endorsed by the publisher.
Research integrity at Frontiers
Learn more about the work of our research integrity team to safeguard the quality of each article we publish.