- 1State Engineering Technology Institute for Karst Desertfication Control, School of Karst Science, Guizhou Normal University, Guiyang, China
- 2College of Animal Science, Shanxi Agricultural University, Jinzhong, China
The present study investigated the effects of fermented complete feed (FCF) on fecal microbial composition during the grower-finisher period. A total of 20 pigs (Duroc × Landrace × Yorkshire, 48.74± 1.49 kg) were divided randomly into two groups: the CN group (pigs fed with a basal diet) and the FCF group (pigs fed with FCF). After a 60-day trial period, 3 pigs with middle-weight from each treatment were selected for fecal sampling and fecal microbiota analysis. The results showed that the FCF significantly increased operational taxonomic units (OUT) numbers, alpha diversity (Simpson index and Shannon index), and beta diversity, which means that FCF increased the fecal microbiota diversity. At the phylum level, the abundance of Tenericutes, Spirochaetae, Verrucomicrobia, and Cyanobacteria were changed in pigs fed with FCF; and at the genus level, the abundance of Christensenellaceae_R-7_group, Treponema_2, Ruminococcaceae_UCG-005, Prevotellaceae_UCG-003, Phascolarctobacterium, Roseburia, and Prevotella_9 were changed in pigs fed with FCF. The linear discriminant analysis effect size (LEfSe) analysis showed that Roseburia and Prevotella_9 genera were increased, while Tenericutes phyla and Streptococcus, Christensenellaceae_R-7_group, and Lactobacillus genera were decreased in the FCF group compared to the CN group. Phylogenetic Investigation of Communities by Reconstruction of Unobserved States (PICRUSt) results predicted that the relative abundance of infectious diseases: parasitic associated genes, xenobiotics biodegradation, and metabolism-associated genes were significantly reduced in the FCF group when compared with the CN group, and the relative abundance of signal transduction associated genes, amino acid metabolism-related genes, and replication and repair associated genes were significantly higher in the FCF group when compared with the CN group. In addition, the relative abundance of transport and catabolism-associated genes, membrane transport-associated genes, and biosynthesis of other secondary metabolite-associated genes tended to be higher in the FCF group when compared with the CN group; and the relative abundance of immune diseases associated genes tended to be lower in the FCF group when compared with the CN group. In conclusion, the FCF influenced the alpha and beta diversity of the fecal microbiota of pigs.
Introduction
Gut microbiota is thought to be tightly associated with the intestinal barrier and plays a vital role in host health (1, 2). Maintaining the dynamic balance of intestinal microbiota is of prime importance to animal growth and health. However, many factors can cause microbial imbalances in the gastrointestinal tract, which may seriously affect nutrients' utilization and host health (3–5). Gut microbiota diversity and composition in humans as well in animals can be modulated by nutritional strategies, such as changes in dietary composition and the addition of feed additives (3, 6, 7). For example, Yin et al. (8) reported that a lysine-restricted diet affected the abundance of Actinobacteria, Saccharibateria, and Synergistetes in the gut of piglets. Adler et al. (9) showed that a dry feed significantly improved oral bacterial diversity when compared with a wet feed in cats. Wassie et al. (10) highlighted that dietary supplementation with Enteromorpha polysaccharide could modulate the cecal microbiota of broiler chickens. Therefore, nutritional regulation of the gut microbiota of animals may be an effective way to promote animal or host health.
Fermented feeds, which are easily decomposed biologically, contain abundant organic acids, amino acids, and small peptides, and have higher nutritional value than the original ingredients (11–13). For example, we showed in our previous study that, after fermentation with Aspergillus Niger, the contents of hydrolyzed protein and small molecular peptides in cottonseed meal were significantly increased, and the digestibility of amino acid and metabolic energy were also significantly increased in white leghorn roosters (14). The fermented feed has been suggested as one of the effective ways to improve the nutritional status as well as the intestinal morphology and microbiota composition of animals (11, 13). Previous studies reported that fermented feed can promote intestinal health by modulating the gut microbiota (15–17). Xie et al. (18) showed that a fermented soybean meal diet could improve the intestinal function by increasing the relative abundance of Firmicutes phylum and decreasing the relative abundance of Proteobacteria phylum in piglets. Similarly, Wang et al. (19) showed that fermented soybean meal could alleviate diarrhea symptoms in weaned piglets by modulating cecal microbiota composition (Proteobacteria phylum). Firmicutes includes a number of probiotics, such as Lactobacillus and Clostridium, which are beneficial to the intestinal barrier function (20, 21); while Proteobacteria, mainly composed of pathogenic microorganisms, such as Escherichia coli and Campylobacter, is strongly associated with intestinal inflammation (22, 23). So, the fermented feed has been shown to be beneficial in gut microbiota regulation. However, to our knowledge, the effects of fermented complete feed (FCF) on the fecal microbiota of finishing pigs are not known.
While the microbial composition is known to differ between the different intestinal segments, the fecal microbiome is most similar to the colon microbiome (23–25). Moreover, in pig production, fecal wastes are important pollution sources and the main carrier of zoonotic pathogens. Pathogenic bacteria in feces can contaminate the water, soil, and farm environments and can lead to human and animal infections (26, 27). The study of fecal microbiome can reflect the intestinal function as well as environmental pollution risks. Therefore, the aim of the present study was to study the effects of the FCF on fecal microbial diversity and composition during the grower-finisher period.
Materials and Methods
Animals, Diets, and Experimental Design
The basal diet was formulated according to the feeding standard of swine (NY/T 65-2004) to meet the nutritional requirement of the fatting pigs (Supplementary Table 1). The FCF preparation was the same as described in our previous study (28). Briefly, the complete feed was fermented at 24–34°C for 72 h in a ratio of 1:3:3:2 between Lactobacillus plantarum: Candida utilis: Bacillus subtilis: Aspergillus niger. After fermentation, the crude protein content (CP, 16.92%) and the crude fiber content (CF, 3.27%) were determined according to the AOCS (2009) method, and Lactobacillus plantarum (1.5 × 108 Cfu/g), Candida utilis (2.1 × 106 Cfu/g), and Bacillus subtilis (3.0 × 108 Cfu/g) were measured using the plate counting techniques. The animal experimental procedure was the same as our previous study (28). Briefly, a total of 20 pigs (Duroc × Landrace × Yorkshire, 48.74 ± 1.49 kg) were randomly divided into the control group (pigs fed with a basal diet, CN) and the FCF group (pigs fed with an FCF diet), each treatment containing 10 pigs. All pigs were fed 3 times per day and had ad libitum access to water. The experiment lasted 60 days.
Sample Collection
At the end of the feeding trial, three pigs with middle-weight from each treatment were selected for fecal samples collection. When pigs defecated, the fresh fecal was collected directly by hand, installed in cryopreserved tubes, then immediately frozen in liquid nitrogen, and stored at −80°C for microbiota analysis.
Fecal Microflora Analysis
The fecal microbiota was analyzed by 16S high-throughput sequencing following the procedure described in our previous study (28). The main procedure included DNA extraction, PCR amplification of 16s rRNA, amplicon sequencing, and sequence data processing. Total genome DNA from fecal microbiota was extracted using the QIAamp DNA Stool Mini Kit (Qiagen GmbH, Hilden, Germany) following the manufacturer's instructions. The quality and quantity of extracted DNA were measured by reading the whole absorbing spectrum using a NanoDrop 2000 UV–vis spectrophotometer (Thermo Fisher Scientific, Waltham, MA, USA) and were used to calculate the DNA concentration and purity accordingly. DNA integrity was checked using 1% agarose gel electrophoresis. Illumina MiSeq sequencing and bioinformatics pipeline services were provided by a commercial company (Biomarker, Beijing, China). PCR amplified the V3–V4 region of the bacterial 16S rRNA gene to define the bacterial composition and abundance. The PCR amplicon was separated on 2% agarose gels, purified, pooled at equimolar concentrations, and finally subjected to paired-end sequencing on an Illumina MiSeq platform according to the standard methods. High-quality sequences were uploaded to QIIME (version 1.7.0) with effective tags clustered into operational taxonomic units (OTUs) using UCLUST in QIIME (version 1.7.0) at 97% sequence identity. The OTUs were annotated based on Silva (bacteria) taxonomy databases. Then, the microbiome composition of each sample was calculated at each level (phylum, class, order, family, genus, species), and QIIME software was used to generate species abundance tables at different classification levels.
Alpha and beta diversity analyses further determined the composition and abundance of the microflora. The linear discriminant analysis effect size (LEfSe) analysis was conducted by LEfSe software to identify the different abundant taxa between the CN group and FCF group. OTUs were further used for genome prediction of microbial communities by Phylogenetic Investigation of Communities by Reconstruction of Unobserved States (PICRUSt).
Statistical Analysis
Data were presented as the mean ± standard error of the mean (SEM). The data were subjected to the unpaired t-test using SPSS 21.0 programs (SPSS, Inc., Chicago, IL, USA). A p < 0.05 was considered to be statistically significant.
Results
Growth Performance
The effects of FCF on the growth performance of pigs were reported in our previous study (28). Briefly, pigs fed with FCF exhibit a higher (P < 0.05) final body weight (FBW), average daily gain (ADG), and average daily feed intake (ADFI), and a lower (P < 0.05) feed-to-gain ratio (F/G) compared to pigs fed with a basal diet (Supplementary Table 2).
Data Quality Evaluation of Fecal Microflora Sequencing
The data quality was primarily assessed through PE reads, raw tags, clean tags, effective tags, sequence length, and effective (%). The result revealed that an average of 79,998.67 and 79976.00 PE reads were generated from the CN and FCF groups, respectively (Table 1). FCF did not affect the raw tags, clean tags, effective tags, effective (%), and average length.
OTUs Analysis
The effects of FCF on OTUs in fecal samples are illustrated in Figure 1. The results showed that an average of 751 OTUs were identified in the CN group, and an average of 813 OTUs were identified in the FCF group (P < 0.05; Figure 1A). Based on the OTU classification, we calculated the same number of OTUs between the CN group and the FCF group. The Venn diagrams showed that 732 OTUs were shared between the CN group and the FCF group (Figure 1B). Only 19 and 81 OTUs were unique in the CN and FCF groups, respectively.
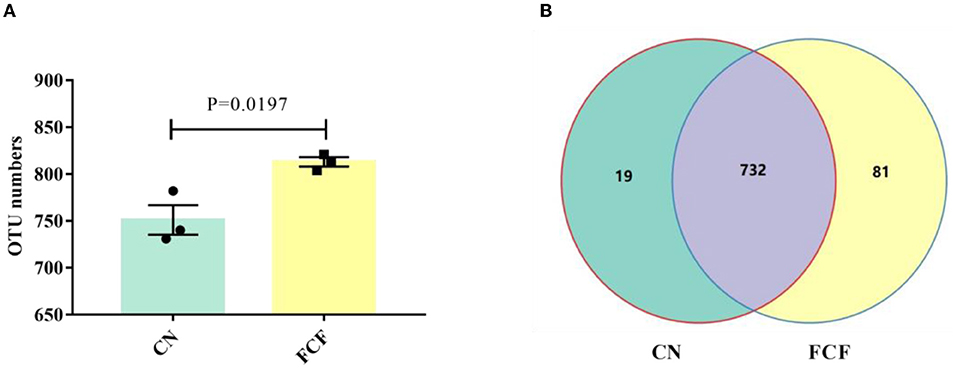
Figure 1. Effects of fermented complete feed on an operational taxonomic unit (OTUs) in fecal samples. Values are expressed as mean ± SEM, n = 3. (A) The number of observed OTUs between CN and FCF groups; (B) Venn diagram of OTUs; Control group; FCF, fermented complete feed group. A p < 0.05 was taken to indicate statistical significance.
Alpha Diversity
Alpha diversity including the ACE index, the Chao1 index, the Shannon index, and the Simpson index are shown in Figure 2. The results showed that FCF significantly increased (P < 0.05) the Simpson index (Figure 2C) and the Shannon index (Figure 2D), and FCF tended to increase (P = 0.0723) the Chao1 index (Figure 2B).
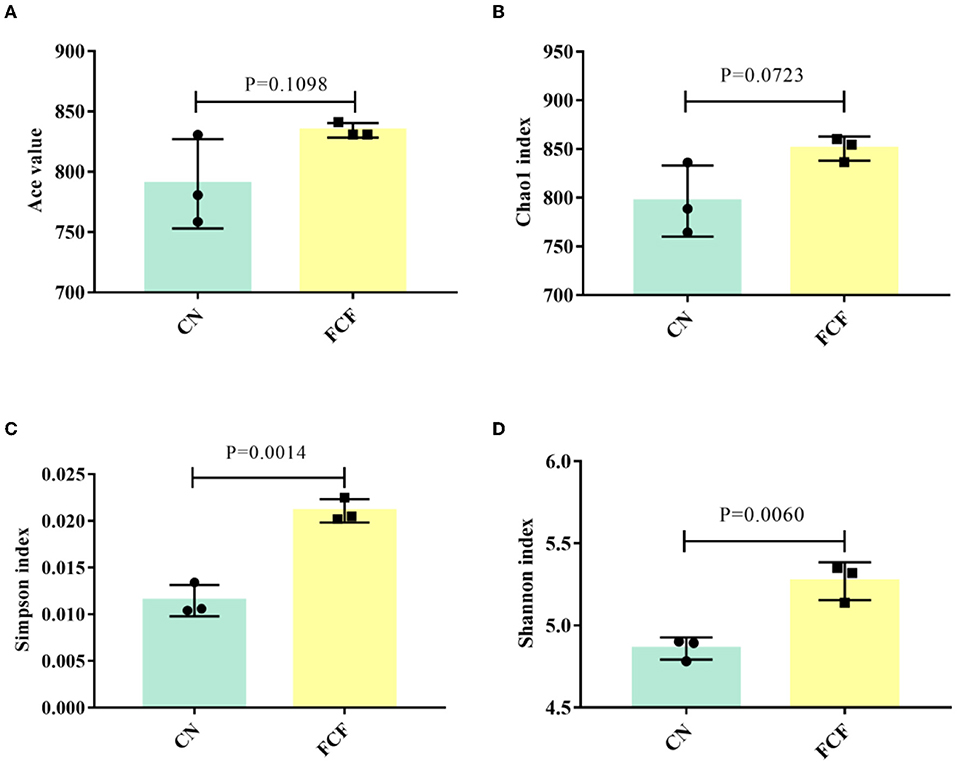
Figure 2. The alpha diversity of the fecal microbial community in pigs fed with a basal diet (CN) or fermented complete feed (FCF). Values are expressed as mean ± SEM, n = 3. (A) ACE index; (B) Chao1 index; (C) Simpson index; (D) Shannon index. A p < 0.05 was taken to indicate statistical significance.
Microbiota Composition at the Phylum Level
The effects of FCF on fecal microbiota composition at the phylum level are presented in Figure 3. Top 10 phyla were identified in the fecal samples, of which Firmicutes, Bacteroidetes, Tenericutes, Proteobacteria, and Spirochaetae comprised the dominant community members (Figure 3A). The abovementioned phyla accounted for 98.86% of the microbial population in the CN group and 99.17% in the FCF group (Figure 3B). Figure 3B shows that pigs fed with FCF have a lower (P < 0.05) abundance of Tenericutes, Spirochaetae, Verrucomicrobia, and Cyanobacteria in fecal samples when compared with pigs fed with a basal diet.
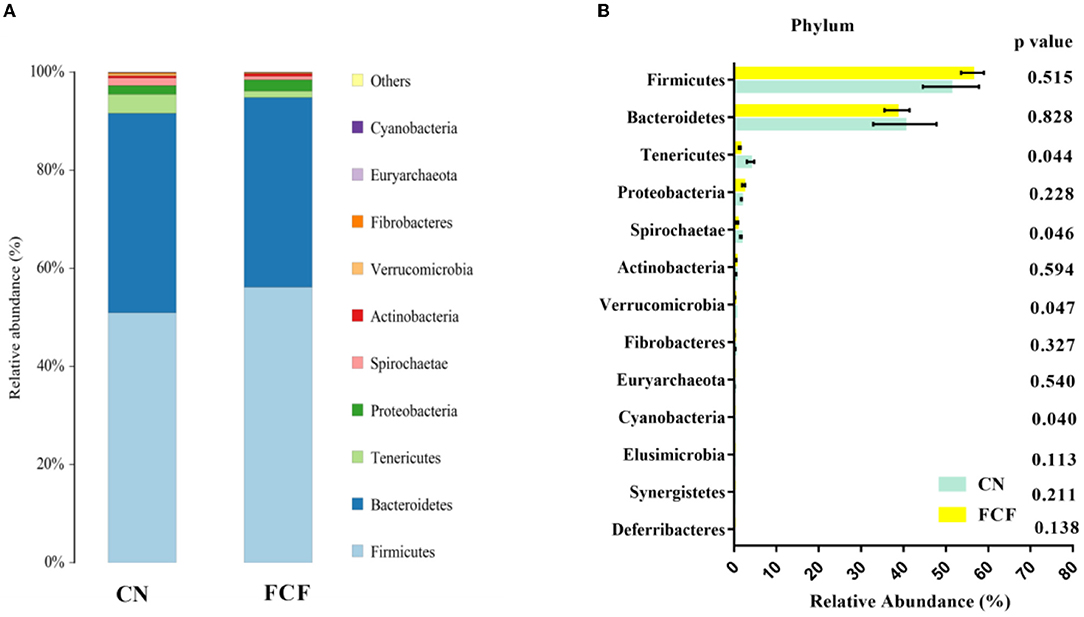
Figure 3. The relative abundance of major phylum in response to pigs fed with a basal diet (CN) or fermented complete feed (FCF). Values are expressed as mean ± SEM, n = 3. (A) Relative abundance of bacterial communities at the phylum level; (B) Unpaired t-test analysis of the difference between CN and FCF group. A p < 0.05 was taken to indicate statistical significance.
Microbiota Composition at the Genus Level
The effects of FCF on the genus-level composition of fecal microbiota are presented in Table 2. Bacterium and Prevotellaceae_NK3B31_group were observed to be the two most abundant genera, accounting for 38.44% and 30% of the microbial population in the CN and FCF groups, respectively. Furthermore, the abundance of Christensenellaceae_R-7_group and Treponema_2 were significantly reduced (P < 0.05) in pigs fed with FCF than in those fed with a basal diet, whereas Ruminococcaceae_UCG-005, Prevotellaceae_UCG-003, Phascolarctobacterium, Roseburia, and Prevotella_9 were found abundantly (P < 0.05) in pigs fed with FCF than in those fed with a basal diet. Upon comparing to the pigs fed with a basal diet, the pigs fed with FCF tended to decrease (P = 0.059) the abundance of Bacterium and tended to increase the abundance of Streptococcus (P = 0.081) and Alloprevotella (P = 0.097).
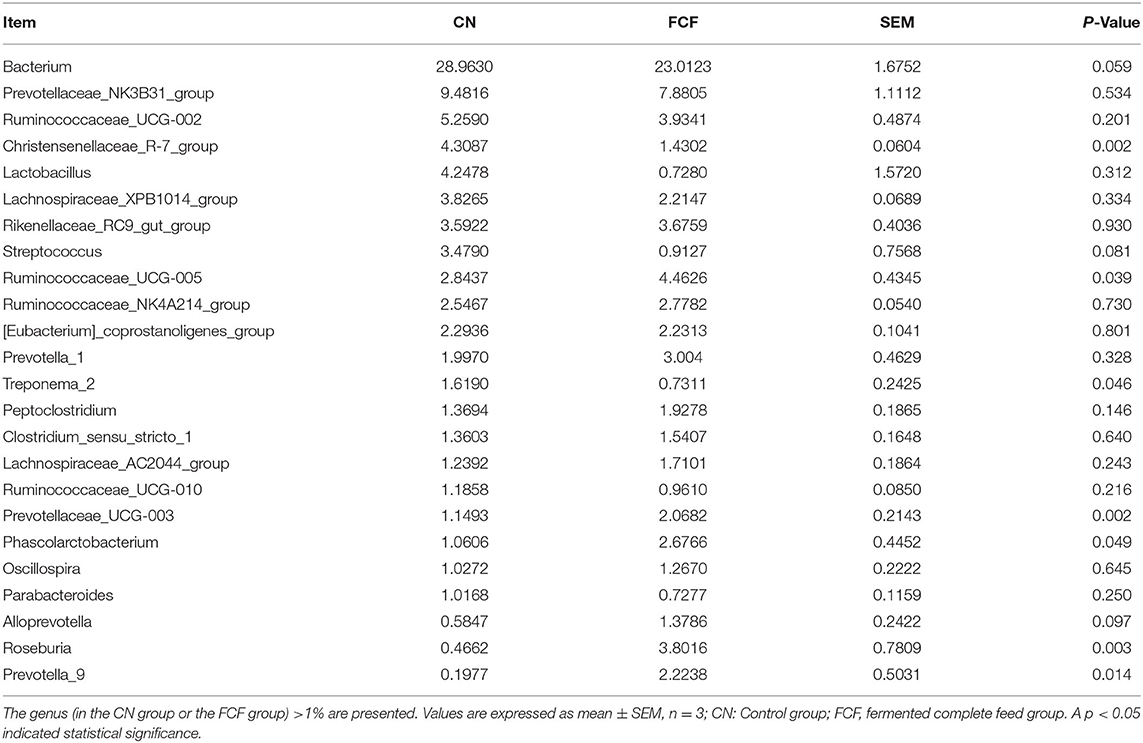
Table 2. The relative abundance of the major genus in response to pigs fed with fermented complete feed.
Beta Diversity
Principal component analysis (PCA) and beta-diversity of distance matrix visualized on a heatmap used Weighted UniFrac distances to estimate the bacterial community's overall differences. The growing microbial community in the FCF group exhibited a distinct clustering. In contrast, the CN group showed a scattered distribution structure in the PCA analysis (Figure 4A). The heatmap chart's color gradient from blue to red represents the distance between samples. The chart showed that the samples in the CN group (F51, F52, F53) are farther apart than that in the FCF group (Figure 4B).
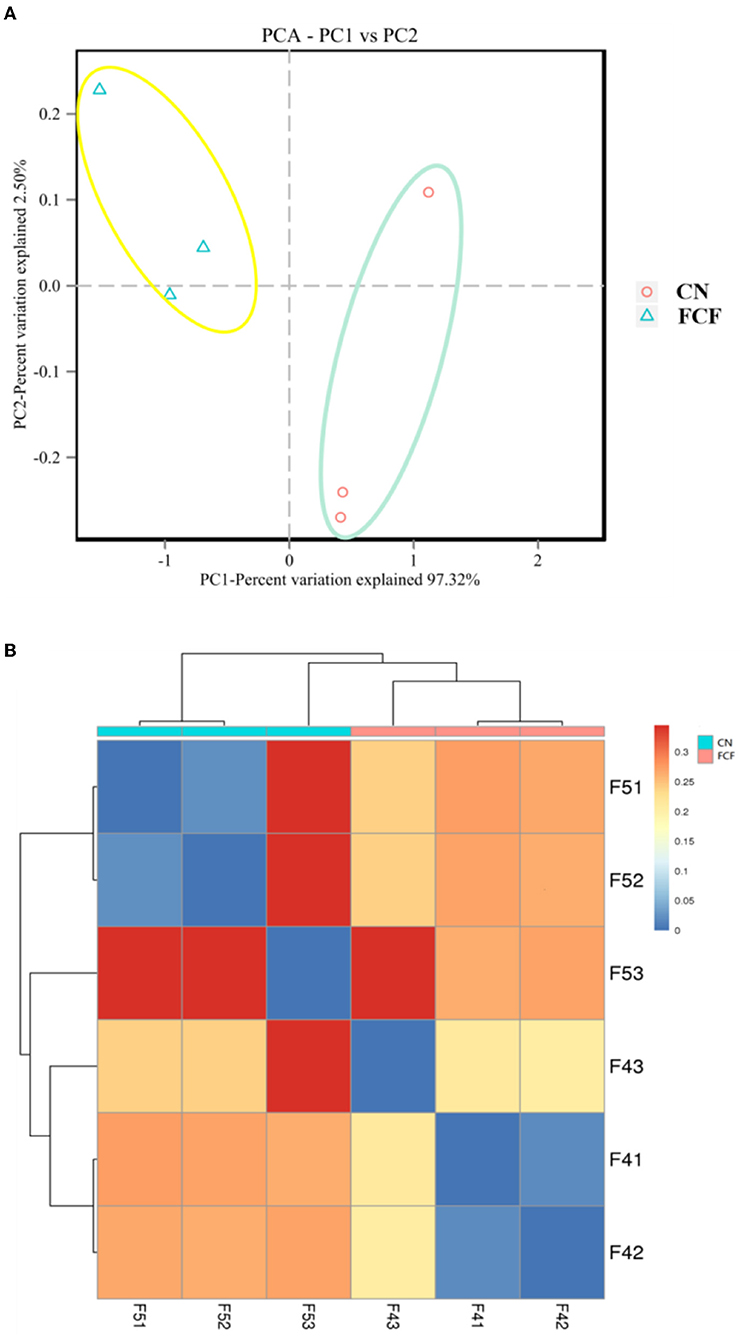
Figure 4. The beta diversity of the fecal microbial community in pigs fed with a basal diet (CN) or fermented complete feed (FCF) (n = 3). (A) Cluster analysis by principal component analysis (PCA); (B) Cluster analysis by a heatmap, the color gradient from blue to red indicates the distance from near to far between samples.
Linear Discriminant Analysis (LDA) Effect Size Analysis
LEfSe package was used to identify the microbial taxa that are differentially abundant across the CN and FCF groups. LEfSe analysis revealed that Tenericutes phylum and Streptococcus, Christensenellaceae_R-7_group, and Lactobacillus genera exhibit lower abundance, which was decreased in the FCF group when compared with the CN group. In contrast, Roseburia and Prevotella_9 genera were abundant in the FCF group (Figure 5).
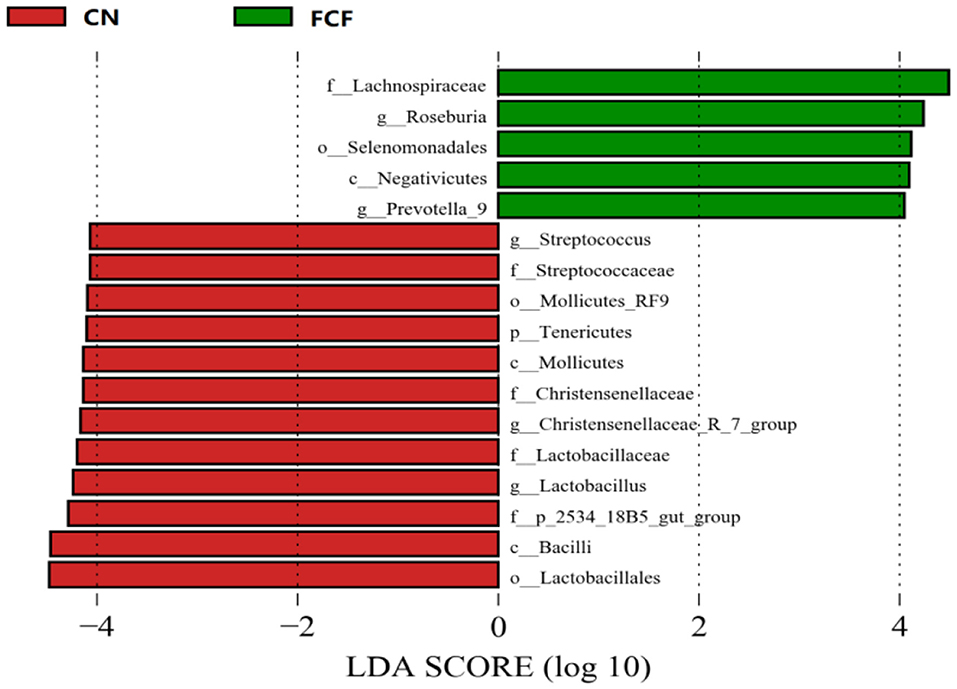
Figure 5. LEfSe analysis filtered out the biomarkers of the microbial community after pigs received basal diet (CN) or fermented complete feed (FCF) (n = 3). Red bars (negative LDA scores) represent bacteria that are more abundant in CN fecal samples than in FCF. Green bars (positive LDA scores) represent bacteria that are more abundant in FCF fecal samples than in CN fecal samples.
Functional Genes Prediction
OTUs were used for generating functional predictions of microbial communities by PICRUSt. The findings revealed the relative abundance of infectious diseases: parasitic genes in bacteria associated with human diseases were significantly reduced in the FCF group when compared with the CN group (P = 0.0423). The relative abundance of immune disorder-related genes is tended to decrease (P = 0.0972) greatly in pigs fed with an FCF diet (Figure 6A). In cellular processes, pigs fed with an FCF diet tended (P = 0.0616) to increase the transport and catabolism-associated gene expression (Figure 6B). Moreover, the relative abundance of signal transduction-associated genes (P = 0.0426) and membrane transport-associated genes (P = 0.0598) were significantly higher in the FCF group than in those of the CN group (Figure 6C). Pertaining to metabolism, the pigs fed with an FCF diet had abundant amino acid metabolism-associated genes (P = 0.0003). On the contrary, a decreasing trend was observed in the relative abundance of xenobiotics biodegradation and metabolism-related genes (P = 0.0360). In addition, a greater tendency (P = 0.0972) was observed for the biosynthesis of other secondary metabolite-associated genes (Figure 6D) compared to the pigs fed with a basal diet. There was no effect on bacterial genes related to organismal systems when pigs were fed with an FCF diet (Figure 6E). For genetic information processing, replication and repair associated genes were significantly increased (P = 0.0266) in pigs fed with FCF (Figure 6F).
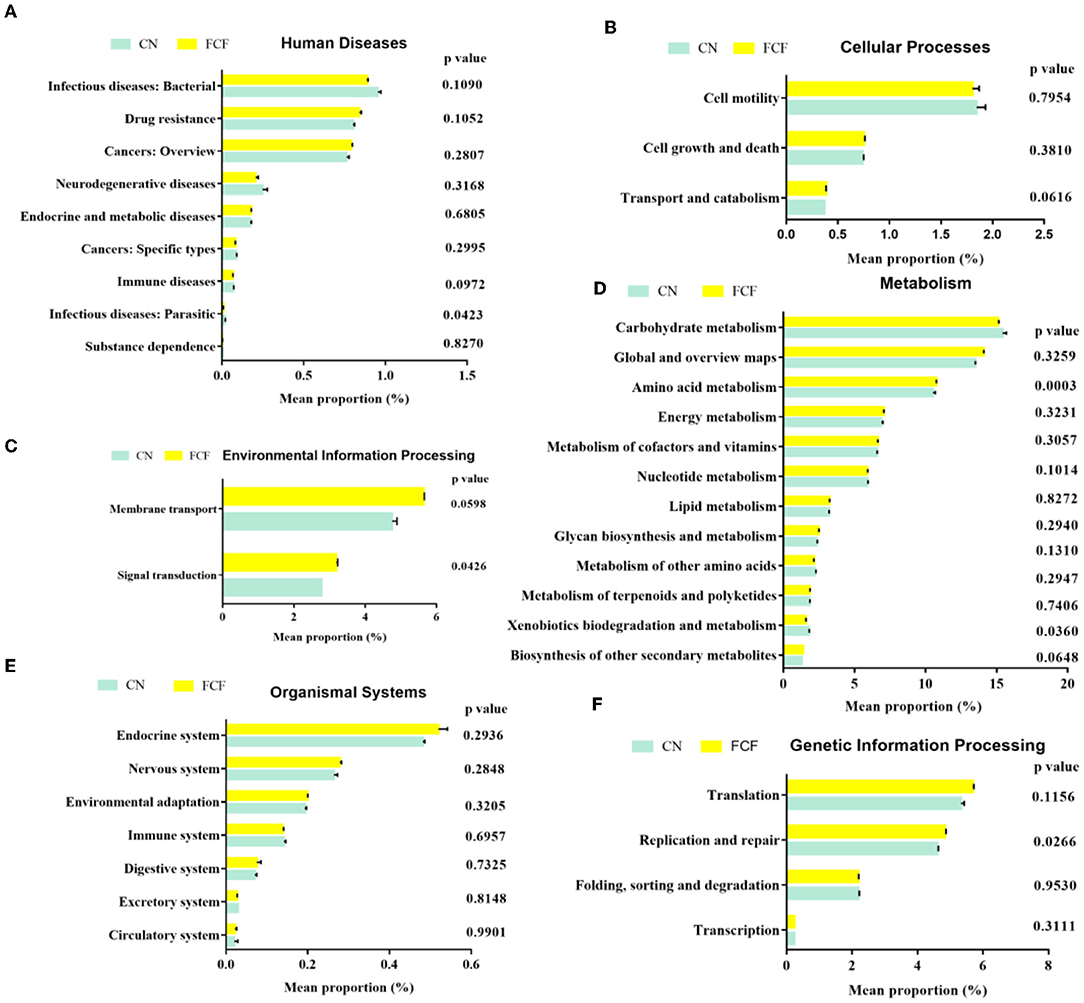
Figure 6. Predictive functional profiling of microbial communities by PICRUSt. Values are expressed as mean ± SEM, n = 3. (A) Human diseases; (B) Cellular processes; (C) Environmental information processing; (D) Metabolism; (E) Organismal systems; (F) Genetic information processing. CN, Control group; FCF, fermented complete feed group. A p < 0.05 was taken to indicate statistical significance.
Discussion
The gut microbiota maintains a symbiotic relationship with the host and regulates several important functions including host metabolism, nutrients digestion and absorption, immunity, and intestinal barrier function (5, 29). So, the dynamic balance of intestinal microbiota is crucial for animal intestinal health, while optimum intestinal health is crucial to animal growth and health (30, 31). Growing shreds of evidence demonstrated that many factors including diets can affect the gut microbiota community and function (6, 7, 29, 32, 33). For instance, Guo et al. (34) illustrated that a high-fat diet exacerbates the pro-inflammatory cytokine-associated gut microbiota in mice. Spring et al. (35) reported that a low–protein diet significantly increased the abundance of Christensenedilaceae, Aligiphilus, and Algoriphagus genera and decreased the relative abundance of Prevotella genus in pigs. The fecal microbiome exhibit a high degree of resemblance with the colon microbiome (23, 24), due to its widespread availability, and fecal microbes are frequently tested to reflect the status of gut microbes. In the present study, we studied the effects of FCF on the fecal microbiota of pigs during the grower-finisher period and observed a change in fecal microbiota in response to FCF diet intake. It is commonly accepted that pigs share similar anatomic, physiologic, and metabolic characteristics with humans (36); however, the findings in the present study can also provide new insight into the influences of fermented food on humans' gut microbiome.
Previous studies showed that fermented feed can alter the gut microbiota structure and composition during different growth stages in pigs (9, 16, 28). In this study, 16S high-throughput sequencing technique was applied to detect the fecal bacteria diversity and compositions of pigs fed with an FCF diet. The results showed that the FCF group had a higher OTU number than the CN group, indicating that FCF elevated the fecal microbial diversity. The richness and diversity of the microbial community can reflect the redundancy of the gut bacterial species (37). Alpha diversity (ACE index, Chao1 index, Shannon index and Simpson index) has been hailed as a comprehensive indicator of species richness and evenness (21, 38). In the present study, the comparative analysis revealed a higher Shannon index and Simpson index for the FCF group than for the CN group, which is concordant with our previous study (28). Pigs fed FCF exhibited higher Shannon index and Simpson index in the jejunum and the cecum and higher ACE index, Chao1 index, Shannon index, and Simpson index in the cecum (28). It was confirmed with an interpretation from our findings that FCF increased the community stability and strengthened the connections between communities of fecal microbes. Beta diversity can reflect the species diversity between communities (21). In the current study, the PCA cluster analysis and heatmap used Weighted UniFrac distances analysis revealed that the samples in the FCF group represent a distinct clustering of microbial community structure. In contrast, the samples in the CN group showed a scattered distribution structure. The heatmap chart analysis revealed that the samples in the CN group are farther apart than those in the FCF group, signifying the FCF-driven alteration of the beta diversity of microorganisms.
The fecal microbial composition was analyzed at the phylum and genus levels. In general, Firmicutes and Bacteroidetes are the most predominant phyla in a healthy gut (39, 40). Our findings are consistent with previous studies and confirmed that Firmicutes and Bacteroidetes phyla are the most dominant bacteria community members both in CN and FCF groups. Firmicutes and Bacteroidetes were considered potentially beneficial autochthonous bacteria in animal intestinal tracts (41), which play an important role in improving intestinal homeostasis, such as reducing intestinal diseases and promoting the production of short-chain fatty acids (42, 43). At the genus level, the dominant bacteria differs among different studies. Quan et al. (44) reported that Streptococcus, Clostridium sensu stricto 1, and Lactobacillus were the three most predominant genera in the feces of pigs. According to Wang et al. (45), Prevotella and Clostridium sensu stricto 1 were the two most abundant genera in the feces of pigs. In the present study, Bacterium and Prevotellaceae_NK3B31_group were the two most abundant genera accounting for 38.44 and 30.89% of the microbial population in the CN and FCF groups, respectively. Altering the diet plan is likely to change the composition of gut microbiota (29, 32, 33). The current study revealed the fact that administration of FCF diets significantly changes the abundance of microbiota, in which the most prominent are Tenericutes, Spirochaetae, Verrucomicrobia, and Cyanobacteria phyla and Christensenellaceae R-7 group, Treponema 2, Ruminococcaceae UCG-005, Prevotellaceae UCG-003, Phascolarctobacterium, Roseburia, and Prevotella_9 genera in the feces of pigs. One of the possible reasons of FCF affecting the composition of the fecal microbiota is the existence of Aspergillus niger, a fungus with strong enzyme (cellulase, protease, and amylase) secreting ability (14), which renders the decomposition of macromolecules into small molecules, thus improving the palatability and nutrient digestibility of feed (28). The increased nutritional intake provides sufficient nutrients for gut microbiota, thereby altering the fecal microbial abundance and composition. Another possible reason is that the FCF diets contain Lactobacillus plantarum, Candida utilis, and Bacillus subtilis (28), which can function as probiotics (46–49), to affect the fecal microbial abundance and composition.
PICRUSt was employed to predict the functional composition of a metagenome using marker genes from 16S rRNA sequencing (50). PICRUSt analysis predicted that FCF could reduce the risk of parasitic infection by reducing the relative abundance of infectious diseases: parasitic-associated genes; FCF could improve signal transduction by increasing the relative abundance of associated genes. Similarly, FCF enhanced amino acid metabolism and reduced xenobiotics biodegradation and metabolism, indicating the beneficial effect of CFC feeding on pig metabolism. Moreover, FCF potentially improved the ability of replication and repair. Previous studies demonstrated that fermented feed can improve not only growth performance but also animal health (12, 28, 51–54). In agreement, the current study demonstrated that FCF could improve the growth potential and health status of pigs. It is possible that FCF alteration of intestinal microbiota affected intestinal bacterial gene expression, such as infectious diseases: parasitic, transport and catabolism, signal transduction, amino acid metabolism, xenobiotics biodegradation, and metabolism, and replication and repair, and ultimately promoted animal health.
Conclusions
The current study showed that FCF significantly increased the fecal bacteria diversity, which is indicated by the increased OUT numbers, alpha diversity (Simpson index and Shannon index), and beta diversity of pigs. At the phylum level, FCF significantly affected the fecal abundance of Tenericutes, Spirochaetae, Verrucomicrobia, and Cyanobacteria; At the genus level, Christensenellaceae_R-7_group, Treponema_2, Ruminococcaceae_UCG-005, Prevotellaceae_UCG-003, Phascolarctobacterium, Roseburia, and Prevotella_9 were changed in response to FCF. Predictive functional profiling of microbial communities by PICRUSt predicted that an altered intestinal microbiome caused by FCF might influence infectious diseases: parasitic, transport and catabolism, signal transduction, amino acid metabolism, xenobiotics biodegradation and metabolism, and replication and repair. In conclusion, FCF modulated the fecal microbial abundance and composition of pigs.
Data Availability Statement
The datasets presented in this study can be found in online repositories. The names of the repository/repositories and accession number(s) can be found at: https://www.ncbi.nlm.nih.gov/, PRJNA787021.
Ethics Statement
The experimental procedures involving animals were reviewed and approved by the animal welfare committee of the Shanxi Agricultural University (Taigu, China) with an ethic approval number SXAU-2018-0093.
Author Contributions
XT and KZ performed the experiments, contributed to experimental concepts and design, provided scientific direction, and finalized the manuscript. XT, KX, and KZ performed the statistical analyses and wrote the manuscript. All authors read and approved the final manuscript.
Funding
This research was funded by grants from the Key Project of Science and Technology Program of Guizhou Province (Grant No. 5411 2017 Qiankehe Pingtai Rencai); the World Top Discipline Program of Guizhou Province (Grant No. 125 2019 Qianjiao Keyan Fa); Guizhou Normal University Academic New Seedling Fund project [Qianshi Xinmiao (2021)B16]; the Key Research and Development Program of Shanxi Province (201603D221026-4); the Central Guide Local Science and Technology Development Special Fund (2017GA630002); and the Natural Science Research Project of Education Department of Guizhou Province [Qianjiaohe KY Zi (2021) 294].
Conflict of Interest
The authors declare that the research was conducted in the absence of any commercial or financial relationships that could be construed as a potential conflict of interest.
Publisher's Note
All claims expressed in this article are solely those of the authors and do not necessarily represent those of their affiliated organizations, or those of the publisher, the editors and the reviewers. Any product that may be evaluated in this article, or claim that may be made by its manufacturer, is not guaranteed or endorsed by the publisher.
Supplementary Material
The Supplementary Material for this article can be found online at: https://www.frontiersin.org/articles/10.3389/fvets.2022.894909/full#supplementary-material
References
1. Allam-Ndoul B, Castonguay-Paradis S, Veilleux A. Gut microbiota and intestinal trans-epithelial permeability. Int J Mol Sci. (2020) 21:6402. doi: 10.3390/ijms21176402
2. Fu Z, Yang H, Xiao Y, Wang X, Yang C, Lu L, et al. Ileal microbiota alters the immunity statues to affect body weight in muscovy ducks. Front Immunol. (2022) 13:844102. doi: 10.3389/fimmu.2022.844102
3. Yang Q, Liang Q, Balakrishnan B, Belobrajdic DP, Feng QJ, Zhang W. Role of dietary nutrients in the modulation of gut microbiota: a narrative review. Nutrients. (2020) 12:381. doi: 10.3390/nu12020381
4. Chen YC, Yu YH. Bacillus licheniformis-fermented products improve growth performance and the fecal microbiota community in broilers. Poult Sci. (2020) 99:1432–1443. doi: 10.1016/j.psj.2019.10.061
5. Tang X, Liu X, Zhong J, Fang R. Potential application of Lonicera japonica extracts in animal production: from the perspective of intestinal health. Front Microbiol. (2021) 12:719877. doi: 10.3389/fmicb.2021.719877
6. Danzeisen JL, Kim HB, Isaacson RE, Tu ZJ, Johnson TJ. Modulations of the chicken cecal microbiome and metagenome in response to anticoccidial and growth promoter treatment. PLoS ONE. (2011) 6:e27949. doi: 10.1371/journal.pone.0027949
7. Dicksved J, Jansson JK, Lindberg JE. Fecal microbiome of growing pigs fed a cereal based diet including chicory (Cichorium intybus L.) or ribwort (Plantago lanceolata L.) forage. J Anim Sci Biotechnol. (2015) 6:53. doi: 10.1186/s40104-015-0054-8
8. Yin J, Han H, Li Y, Liu Z, Zhao Y, Fang R, et al. Lysine restriction affects feed intake and amino acid metabolism via gut microbiome in piglets. Cell Physiol Biochem. (2017) 44:1749–61. doi: 10.1159/000485782
9. Adler CJ, Malik R, Browne GV, Norris JM. Diet may influence the oral microbiome composition in cats. Microbiome. (2016) 4:23. doi: 10.1186/s40168-016-0169-y
10. Wassie T, Lu Z, Duan X, Xie C, Gebeyew K, Yumei Z, et al. Dietary Enteromorpha polysaccharide enhances intestinal immune response, integrity, and caecal microbial activity of broiler chickens. Front Nutr. (2021) 8:783819. doi: 10.3389/fnut.2021.783819
11. Xie Y, Liu J, Wang H, Luo J, Chen T, Xi Q, et al. Effects of fermented feeds and ginseng polysaccharides on the intestinal morphology and microbiota composition of Xuefeng black-bone chicken. PLoS ONE. (2020) 15:e0237357. doi: 10.1371/journal.pone.0237357
12. Missotten JAM, Michiels J Degroote J, De Smet S. Fermented liquid feed technique for the future. J Anim Sci Biotechnol. (2015) 6:4. doi: 10.1186/2049-1891-6-4
13. Zhao J, Zhang Z, Zhang S, Page G, Jaworski NW. The role of lactose in weanling pig nutrition: a literature and meta-analysis review. J Anim Sci Biotechnol. (2021) 12:10. doi: 10.1186/s40104-020-00522-6
14. Tang X, Xiang R, Chen S, Yang S, Liu H, Fang R, et al. Effects of fermented cottonseed meal and enzymatic hydrolyzed cottonseed meal on amino acid digestibility and metabolic energy in white leghorn rooster. Pakistan J Zool. (2018) 50:959–62. doi: 10.17582/journal.pjz/2018.50.3.957.962
15. Yin FG, Farzan A, Wang Q, Yu H, Yin YL, Hou YQ, et al. Reduction of Salmonella enterica Serovar Typhimurium DT104 infection in experimentally challenged weaned pigs fed a Lactobacillus-fermented feed. Foodborne Pathog Dis. (2014) 11:628–34. doi: 10.1089/fpd.2013.1676
16. He YY, Mao CX, Wen H, Chen ZY, Lai T, Li LY, et al. Influence of ad libitum feeding of piglets with Bacillus subtilis fermented liquid feed on gut flora, luminal contents and health. Sci Rep. (2017) 7:44553. doi: 10.1038/srep44553
17. Kiarie E, Bhandari S, Scott M, Krause DO, Nyachoti CM. Growth performance and gastrointestinal microbial ecology responses of piglets receiving Saccharomyces cerevisiae fermentation products after an oral challenge with Escherichia coli (K88). J Anim Sci. (2011) 89:1062–78. doi: 10.2527/jas.2010-3424
18. Xie Z, Hu L, Li Y, Geng S, Cheng S, Fu X, et al. Changes of gut microbiota structure and morphology in weaned piglets treated with fresh fermented soybean meal. World J Microbiol Biotechnol. (2017) 33:213. doi: 10.1007/s11274-017-2374-7
19. Wang W, Wang Y, Hao X, Duan Y, Meng Z, An X, et al. Dietary fermented soybean meal replacement alleviates diarrhea in weaned piglets challenged with enterotoxigenic Escherichia coli K88 by modulating inflammatory cytokine levels and cecal microbiota composition. BMC Vet Res. (2020) 16:245. doi: 10.1186/s12917-020-02466-5
20. Seong CN, Kang JW, Lee JH, Seo SY, Woo JJ, Park C, et al. Taxonomic hierarchy of the phylum firmicutes and novel firmicutes species originated from various environments in Korea. J Microbiol. (2018) 56:1–10. doi: 10.1007/s12275-018-7318-x
21. Che L, Hu L, Zhou Q, Peng X, Liu Y, Luo Y, et al. Microbial insight into dietary protein source affects intestinal function of pigs with intrauterine growth retardation. Eur J Nutr. (2020) 59:327–44. doi: 10.1007/s00394-019-01910-z
22. Pan J, Yin J, Zhang K, Xie P, Kong X. Dietary xylo-oligosaccharide supplementation alters gut microbial composition and activity in pigs according to age and dose. AMB Expr. (2019) 9:134. doi: 10.1186/s13568-019-0858-6
23. Chen L, Xu Y, Chen X, Fang C, Zhao L, Chen F. The maturing development of gut microbiota in commercial piglets during the weaning transition. Front Microbiol. (2017) 8:1688. doi: 10.3389/fmicb.2017.01688
24. Looft T, Allen HK, Cantarel BL, Levine UY, Bayles DO, Alt DP, et al. Bacteria, phages and pigs: the effects of in-feed antibiotics on the microbiome at different gut locations. Isme J. (2015) 8:1576. doi: 10.1038/ismej.2014.12
25. Verschuren LMG, Calus MPL, Jansman AJM, Bergsma R, Knol EF, Gilbert H, et al. Fecal microbial composition associated with variation in feed efficiency in pigs depends on diet and sex. J Anim Sci. (2018) 96:1405–18. doi: 10.1093/jas/sky060
26. Korajkic A, McMinn BR, Harwood VJ. Relationships between microbial indicators and pathogens in recreational water settings. Int J Environ Res Public Health. (2018) 15:2842. doi: 10.3390/ijerph15122842
27. Delahoy MJ, Wodnik B, McAliley L, Penakalapati G, Swarthout J, Freeman MC, et al. Pathogens transmitted in animal feces in low- and middle-income countries. Int J Hyg Environ Health. (2018) 21:661–76. doi: 10.1016/j.ijheh.2018.03.005
28. Tang X, Liu X, Zhang K. Effects of microbial fermented feed on serum biochemical profile, carcass traits, meat amino acid and fatty acid profile, and gut microbiome composition of finishing pigs. Front Vet Sci. (2021) 8:744630. doi: 10.3389/fvets.2021.744630
29. Lobionda S, Sittipo P, Kwon HY, Lee YK. The role of gut microbiota in intestinal inflammation with respect to diet and extrinsic stressors. Microorganisms. (2019) 7:271. doi: 10.3390/microorganisms7080271
30. Tang X, Xiong K, Wassie T Wu X. Curcumin and intestinal oxidative stress of pigs with intrauterine growth retardation: a review. Front Nutr. (2022) 9:847673. doi: 10.3389/fnut.2022.847673
31. Tang X, Xiong K. Intrauterine growth retardation affects intestinal health of suckling piglets via altering intestinal antioxidant capacity, glucose uptake, tight junction, and immune responses. Oxid Med Cell Longev. (2022) 2022:2644205. doi: 10.1155/2022/2644205
32. Gentile CL, Weir TL. The gut microbiota at the intersection of diet and human health. Science. (2018) 362:776. doi: 10.1126/science.aau5812
33. Zhao J, Zhang X, Liu H, Brown MA, Qiao S. Dietary protein and gut microbiota composition and function. Curr Protein Pept Sci. (2019) 20:145–54. doi: 10.2174/1389203719666180514145437
34. Guo X, Li J, Tang R, Zhang G, Zeng H, Wood RJ, et al. High fat diet alters gut microbiota and the expression of paneth cell-antimicrobial peptides preceding changes of circulating inflammatory cytokines. Mediators Inflamm. (2017) 2017:9474896. doi: 10.1155/2017/9474896
35. Spring S, Premathilake H, DeSilva U, Shili C, Carter S, Pezeshki A. Low protein-high carbohydrate diets alter energy balance, gut microbiota composition and blood metabolomics profile in young pigs. Sci Rep. (2020) 10:3318. doi: 10.1038/s41598-020-60150-y
36. Ferenc K, Pietrzak P, Godlewski MM, Piwowarski J, Kiliańczyk R, Guilloteau P, et al. Intrauterine growth retarded piglet as a model for humans–studies on the perinatal development of the gut structure and function. Reprod Biol. (2014) 14:51–60. doi: 10.1016/j.repbio.2014.01.005
37. Alam A, Neish A. Role of gut microbiota in intestinal wound healing and barrier function. Tissue Barriers. (2018) 6:1539595. doi: 10.1080/21688370.2018.1539595
38. He C, Lei J, Yao Y, Qu X, Chen J, Xie K, et al. Black soldier fly (Hermetia illucens) larvae meal modulates intestinal morphology and microbiota in xuefeng black-bone chickens. Front Microbiol. (2021) 12:706424. doi: 10.3389/fmicb.2021.706424
39. Jandhyala SM, Talukdar R, Subramanyam C, Vuyyuru H, Sasikala M, Nageshwar Reddy D. Role of the normal gut microbiota. World J Gastroenterol. (2015) 21:8787–803. doi: 10.3748/wjg.v21.i29.8787
40. Becker C, Neurath MF, Wirtz S. The intestinal microbiota in inflammatory bowel disease. ILAR J. (2015) 56:192–204. doi: 10.1093/ilar/ilv030
41. Borda-Molina D, Mátis G, Mackei M, Neogrády Z, Huber K, Seifert J, et al. Caeca microbial variation in broiler chickens as a result of dietary combinations using two cereal types, supplementation of crude protein and sodium butyrate. Front Microbiol. (2021) 11:617800. doi: 10.3389/fmicb.2020.617800
42. Cui Y, Han C, Li S, Geng Y, Wei Y, Shi W, et al. High-throughput sequencing-based analysis of the intestinal microbiota of broiler chickens fed with compound small peptides of Chinese medicine. Poult Sci. (2021) 100:100897. doi: 10.1016/j.psj.2020.11.066
43. Ratajczak W, Ryl A, Mizerski A, Walczakiewicz K, Sipak O, Laszczynska M. Immunomodulatory potential of gut microbiome-derived shortchain fatty acids (SCFAs). Acta Biochim Pol. (2019) 66:1–12. doi: 10.18388/abp.2018_2648
44. Quan J, Cai G, Yang M, Zeng Z, Ding R, Wang X, et al. Exploring the fecal microbial composition and metagenomic functional capacities associated with feed efficiency in commercial DLY pigs. Front Microbiol. (2019) 10:52. doi: 10.3389/fmicb.2019.00052
45. Wang J, Ji H, Wang S, Liu H, Zhang W, Zhang D, et al. Probiotic Lactobacillus plantarum promotes intestinal barrier function by strengthening the epithelium and mo ulating gut microbiota. Front Microbiol. (2018) 9:1953. doi: 10.3389/fmicb.2018.01953
46. Mizumachi K, Aoki R, Ohmori H, Saeki M, Kawashima T. Effect of fermented liquid diet prepared with Lactobacillus plantarum LQ80 on the immune response in weaning pigs. Animal. (2009) 3:670–6. doi: 10.1017/S1751731109003978
47. Yang KM, Jiang ZY, Zheng CT, Wang L, Yang XF. Effect of Lactobacillus plantarum on diarrhea intestinal barrier function of young piglets challenged with enterotoxigenic Escherichia coli K88. J Anim Sci. (2014) 92:1496–503. doi: 10.2527/jas.2013-6619
48. Tang X, Liu X, Liu H. Effects of dietary probiotic (Bacillus subtilis) supplementation on carcass traits, meat quality, amino acid, and fatty acid profile of broiler chickens. Front Vet Sci. (2021) 8:767802. doi: 10.3389/fvets.2021.767802
49. Li CL, Wang J, Zhang HJ, Wu SG, Hui QR, Yang CB, et al. Intestinal morphologic and microbiota responses to dietary bacillus spp. in a broiler chicken model. Front Physiol. (2019) 9:1968. doi: 10.3389/fphys.2018.01968
50. Langille MGI, Zaneveld J, Caporaso JG, McDonald D, Knights D, Reyes JA, et al. Predictive functional profiling of microbial communities using 16S rRNA marker gene sequences. Nat Biotechnol. (2013) 31:814. doi: 10.1038/nbt.2676
51. Chen W, Zhu XZ, Wang JP, Wang ZX, Huang YQ. Effects of bacillus subtilis var. natto and Saccharomyces cerevisiae fermented liquid feed on growth performance, relative organ weight, intestinal microflora, and organ antioxidant status in landes geese. J Anim Sci. (2013) 91:978–985. doi: 10.2527/jas.2012-5148
52. van Winsen RL, Urlings BAP, Lipman LJA, Snijders JMA, Keuzenkamp D, Verheijden JHM, et al. Effect of fermented feed on the microbial population of the gastrointestinal tracts of pigs. Appl Environ Microbiol. (2001) 67:3071–6. doi: 10.1128/AEM.67.7.3071-3076.2001
53. Canibe N, Jensen BB. Fermented liquid feed -microbial and nutritional aspects and impact on enteric diseases in pigs. Anim Feed Sci Technol. (2012) 173:17–40. doi: 10.1016/j.anifeedsci.2011.12.021
Keywords: fermented complete feed, fecal microbiome, genome prediction, microbial diversity, pigs
Citation: Tang X, Zhang K and Xiong K (2022) Fecal Microbial Changes in Response to Finishing Pigs Directly Fed With Fermented Feed. Front. Vet. Sci. 9:894909. doi: 10.3389/fvets.2022.894909
Received: 12 March 2022; Accepted: 24 June 2022;
Published: 22 July 2022.
Edited by:
Eveline M. Ibeagha-Awemu, Agriculture and Agri-Food Canada (AAFC), CanadaReviewed by:
Yunhe Cao, China Agricultural University, ChinaTariq Jamil, Friedrich Loeffler Institut, Germany
Copyright © 2022 Tang, Zhang and Xiong. This is an open-access article distributed under the terms of the Creative Commons Attribution License (CC BY). The use, distribution or reproduction in other forums is permitted, provided the original author(s) and the copyright owner(s) are credited and that the original publication in this journal is cited, in accordance with accepted academic practice. No use, distribution or reproduction is permitted which does not comply with these terms.
*Correspondence: Kangning Xiong, eGlvbmdrYW5nbmluZzIwMjFAMTI2LmNvbQ==
†These authors have contributed equally to this work