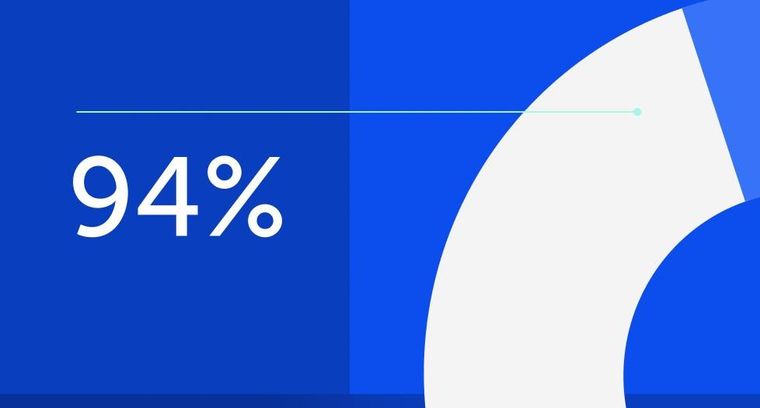
94% of researchers rate our articles as excellent or good
Learn more about the work of our research integrity team to safeguard the quality of each article we publish.
Find out more
REVIEW article
Front. Vet. Sci., 16 June 2022
Sec. Veterinary Neurology and Neurosurgery
Volume 9 - 2022 | https://doi.org/10.3389/fvets.2022.889561
This article is part of the Research TopicEpilepsy in Veterinary ScienceView all 23 articles
Modulation of neuronal activity for seizure control using various methods of neurostimulation is a rapidly developing field in epileptology, especially in treatment of refractory epilepsy. Promising results in human clinical practice, such as diminished seizure burden, reduced incidence of sudden unexplained death in epilepsy, and improved quality of life has brought neurostimulation into the focus of veterinary medicine as a therapeutic option. This article provides a comprehensive review of available neurostimulation methods for seizure management in drug-resistant epilepsy in canine patients. Recent progress in non-invasive modalities, such as repetitive transcranial magnetic stimulation and transcutaneous vagus nerve stimulation is highlighted. We further discuss potential future advances and their plausible application as means for preventing epileptogenesis in dogs.
Epilepsy is the most common neurological brain disorder affecting both humans and non-human animals, with a prevalence in the human population of 0.64% in the active form or 0.76% with cases in remission (lifetime prevalence) (1), and in dogs 0.6–0.75% of the general dog population (2). However, the mere presence of genetically very homogenous purebred populations favors a more frequent occurrence of epilepsy in some canine breeds. Here, the prevalence can range from 3% up to 18% (2) or even 33% as described in a family of Belgian shepherd dogs (3). The high prevalence rates underscore the relevance of this condition for veterinary practice.
Epilepsy poses a significant challenge for veterinary and human medicine, in part because of the high rates of resistance to first and second line anti-seizure medications. The occurrence of drug-resistant epilepsy (DRE) has been reported in 13.7% of the community out-patient and 36.3% of the clinic-based human population (4), and similar numbers are assumed to apply in dogs (5). Many hypotheses exist regarding the pathophysiology of DRE, including alterations in blood brain barrier's multidrug transporter expression, pharmacokinetics, pharmacodynamics, genetic variability, functional changes of neural networks and intrinsic severity of the disease, as well as involvement of inflammatory processes (6, 7). Most third line therapeutic approaches aim to circumvent some of those challenges. Common treatment approaches in human medicine include dietary approaches, brain surgery and neurostimulation (6, 8). While the first two approaches are relatively easy to implement in veterinary practice (9, 10), surgery and neurostimulation remain problematic because of their cost, time, and high level of skills required. However, the growing body of evidence for efficacy of neurostimulation techniques in human patients is raising awareness and interest in this therapeutic approach among veterinary practitioners. Therefore, it is of interest to know, which techniques have already been applied in canine patients with DRE, to understand their advantages and disadvantages, and to develop a road-map for their further development and assessment in canine patients.
First mentions of neurostimulation as a therapeutic method date back to the first century CE. At that time, electric fish attachment to skin was used to relief pain in patients (11). Advances in understanding of physics of electricity in the late nineteenth and early twentieth century revived interest in neurostimulation, which became a popular topic in the 1950s and 1960s when various devices, including those for epilepsy management, were developed (12–17). However, although significant technological improvements have been made in recent decades, our understanding of the mechanism of action of neurostimulation in the context of many diseases remains vague.
Neurostimulation can be performed both in the peripheral and in the central nervous system. While first one is e.g., performed in cases of neuropathic pain (18, 19), for nerve regeneration after injury (20) and to re-establish sensation in people with prostheses (21), central stimulation serves alleviation of symptoms of e.g., tremor diseases (22–24), neuropsychiatric disorders (25–27), pain (28, 29) and epilepsy (30–32). Vagus nerve stimulation (VNS), deep brain stimulation (DBS), and transcranial magnetic stimulation (TMS) are the current methods applied and described in veterinary medicine. Therefore, the review article has focused on these three therapy options.
Neurostimulation exerts effects on nervous tissue at cellular, molecular and structural levels. Mathematical modeling of high frequency stimulation in neural networks revealed its stabilizing influence on cells (33). Neural circuits showed reduced susceptibility to sudden transitions into oscillations usually marking the onset of a seizure. Moreover, inhibitory cells were recruited more strongly than excitatory cells, putting the system in an “anti-seizure state” (33). This mechanism may be the basis for acute seizure termination after application of high frequency stimulation. Brain stimulation also led to changes in connectivity of the brain inside and outside of epileptic foci and different protocols led to promotion or suppression of circuit synchronicity (34, 35).
Stimulation of neural tissue alters not only its electrical properties but also its chemical microenvironment. Several studies describe its modulatory influence on release and production of neurotransmitters, extracellular vesicles, brain-derived neurotrophic factor (BDNF) and on receptor function (36–40). Similarly, neurostimulation promotes glial cell activation, astrocytic signaling and proliferation of neuronal progenitor cells (41, 42). This might serve as a double-edged sword in the process of epileptogenesis, starting regenerative processes in the brain on one hand, which on the other hand might lead to creation of hyperexcitable networks, when they turn abnormal, as observed in rodent models of epilepsy and epileptogenesis (43, 44). However, early concerns about therapeutic electrical brain stimulation kindling human brain has not been seen in the class-I evidence trials of responsive neural stimulation (RNS) (45) and deep brain stimulation of anterior nucleus of thalamus (DBS) (46) in long-term human trials.
As a matter of course, the main goal of electrical stimulation of the epileptic brain is better seizure control. As can be seen from the neuronal network studies, this can be achieved either by stopping a developing seizure or by preventing its occurrence in the first place. The goal can be achieved either by targeted stimulation ideally before a seizure manifests (using sophisticated prediction algorithms), or by providing a cumulative long-term anti-seizure effect of regular continuous stimulations. Since long-term complete freedom from seizures is rarely achieved with electrical stimulation, therapeutic success can be difficult to define and quantify. Moreover, it often depends on patient's age, sex, and individual variability (47). Particularly important in human epilepsy is the impact of epilepsy on mood, memory, and quality of life. While rarely achieving complete seizure freedom the class-I evidence trials in humans demonstrate improved quality of life.
The need for individualized decisions is also evident when it comes to selection of the optimal method of neurostimulation: not every patient will be eligible for surgery or anesthesia, so electrode implantations might be contraindicated in these cases. Understanding the advantages and disadvantages of the most commonly used neurostimulation methods will certainly be beneficial to many veterinary neurologists. Learning from veterinary researchers conducting pilot studies in canine patients and from experienced human neurologists applying neurostimulation approaches in their clinics will be useful for applying neurostimulation in their veterinary research and practice.
Vagus nerve stimulation (VNS) as treatment of human epilepsy was first introduced in 1988 (48); however, initial trials of external stimulation of the vagus nerve date more than 100 years earlier (49). Even before the first implantation in humans, Zabara managed to attenuate seizures evoked by injections of strychnine or pentylenetetrazole (PTZ) in dogs (50), which paved the way for further clinical trials. VNS got approval for management of epilepsy in Europe in 1994 and in the USA in 1997 (49) and currently, it is being used by more than 100,000 patients worldwide (51).
The exact mechanism of action of VNS in epilepsy has not been fully elucidated yet, but anatomy and physiology of the vagus nerve gives insight into possible processes involved. Vagus nerve, the longest cranial nerve, arises in the nucleus ambiguous of the medulla, exits the cranium via the jugular foramen and extends into the neck, thorax and abdomen, where it supplies muscles and inner organs. Over 80% of vagal fibers carry sensory information from the viscera toward the brain (afferent fibers), while only around 20% of fibers are responsible for motor signaling (efferent fibers) (49, 52). Afferents terminate in the nucleus of solitary tract, which projects to multiple brain regions, among which the most crucial for the anti-seizure effect seem to be locus coeruleus and raphe nuclei (52). These regions are strongly activated by VNS and they are heavily engaged in production of neurotransmitters, such as noradrenaline and serotonin, which further stimulate interneurons to release gamma-amino butyric acid (GABA), increasing seizure threshold of neurons. Other potential mechanisms of anti-seizure action of VNS include changes in blood flow in regions correlating with seizure reduction (52–54), up-regulation of neurotrophin production (55) and anti-inflammatory effects (56, 57).
Histologically, the vagus nerve is composed of A-, B-, and C-fibers, the first two types being myelinated. Myelination and diameter of fibers (the largest in A-fibers, the smallest in C-fibers) directly translates in their various stimulation thresholds. Recordings from de-sheathed vagus nerves in healthy dogs placed amplitude thresholds to evoke action potentials at 0.4 mA for A-fibers, for fast B-fibers: 1.6 mA, for slow B-fibers: 3.8 mA and for C-fibers: 17 mA (58). Since current amplitude used for invasive VNS in dogs ranges in literature from 0.25 to 1.5 mA (Table 1), it can be assumed the effects of stimulation are mostly associated with activation of A- and fast B-fibers, consisting of motor and sensory afferent fibers (58).
In human neurology, VNS is indicated, as a third line treatment of epilepsy, when candidates meet following criteria: medically refractory seizures; adequate trials of at least 2 anti-seizure drugs; exclusion of non-epileptic events; and ineligibility for epileptogenic focus resection surgery (66). Usually, it is applied in cases of intractable focal and secondarily generalized tonic-clonic epilepsy, in epilepsy of generalized onset (including atonic seizures) and in epileptic syndromes (54). The implantable device consists of a helical electrode placed around the cervical part of the vagus nerve, a connective lead and a pulse generator, usually localized in a subclavicular region (57). Usually in epilepsy treatment, VNS is applied to the left vagus nerve due to its innervation of the atrioventricular node of the heart. The right vagus nerve innervates the sinoatrial node, the stimulation of which could lead to severe cardiac adverse effects (67). Additionally, care is taken to place the VNS electrodes distal to the superior and inferior cervical cardiac branches of the vagus nerve.
In dogs, it is impossible to spare the cardiac branches from stimulation, because they leave the nerve more distally in the thoracic cavity. Therefore, the electrodes are wrapped around the left vagosympathetic trunk, as both nerves are fused in the cervical region in this species (68) (Figure 1). Consequently, additional sympathetic stimulation and influence on the heart cannot be excluded. During the surgery, the cathode is placed rostrally, the anode in the middle and anchor tether on the caudal portion (68). This configuration (proximal cathode/distal anode) stimulates predominantly afferent vagal fibers, while proximal anode/distal cathode leads mostly to the stimulation of efferents (69). Simultaneously, it does not influence vagal fibers' threshold to evoke action potentials, which only remain sensitive to the amplitude of current used for stimulation (58, 69). Pulse generator can be located dorsally on the left cervical region (68) or on thorax (61), underneath muscular fascia or muscle. Subcutaneous placing is discouraged to avoid migration and seroma formation at the surgery site (68).
Figure 1. A demonstrative illustration of assembly of invasive VNS (A) and DBS (B) in a dog. VNS electrodes are mostly wrapped around the cervical portion of left vagus nerve, whereas DBS electrodes are usually placed in thalamic nuclei. Wires and a controlling device are usually located in a dorsal cervical region. Created with BioRender.com.
In people, the vagus nerve is mostly stimulated in an open-loop fashion: duty cycle (ON and OFF periods) with additional extra stimulation delivered by an external magnet swipe delivered by the patient or caregiver for acute seizures (57). Available closed-loop stimulators utilize sophisticated algorithms to detect seizure events based on ictal cardiac activity associated with seizures (70, 71). Comparison between open- and closed-loop approaches in one cohort study of pediatric patients suggests a better response to VNS after 2 years of treatment, especially among children with generalized epilepsy (71). In dogs, closed-loop VNS has not been studied yet, the evidence from humans suggests however, it could prove beneficial, especially in long term. Additionally, it could decrease the burden of caretakers and veterinary staff, since they would not have to apply additional stimulation with external magnet swipe at the seizure onset.
Human patients with epilepsy undergoing VNS experience a decrease of seizure intensity, seizure duration and a shortening of the post-ictal period (54, 57, 72, 73). The main outcome crucial for the success of anti-seizure therapy, namely reduction of seizure frequency by ≥50%, is reported in ~60% of patients (73) and this effect increases with time (49), often requiring more than half a year for maximal effect (74). Long-term studies demonstrated an improvement of seizure frequency reduction after 1 year of treatment as compared to 3 months stimulation (75, 76) and it reached its peak after 2 years of VNS (77). Secondary effects associated with VNS include improvement of mood, cognition and memory (52, 78–80) as well as lowering of anxiety (78, 81). More recently VNS has been shown to reduce the incidence of sudden unexplained death in epilepsy (SUDEP) (82). Evidence of VNS effects in canine epilepsy is much less abundant than of those gathered from human patients, nevertheless this mode of stimulation has already proved beneficial for dogs with DRE. In the first clinical study published in 2002 by Muñana et al. 10 dogs with DRE demonstrated a decrease in mean seizure frequency by 34.4% in the last 4 weeks of 13-week long therapy (59). Four of nine dogs showed a reduction of seizure frequency by ≥50% (so-called good responders) in this period, while two of them responded in that way during the whole study period (59). This study has shown VNS to reduce seizure frequency in a subpopulation of dogs with DRE, but it is unknown if the seizure suppressing effect increases further, like in people, in the first 6 to 8 months or if VNS remains effective long term. Hirashima et al. recently published a case study with a longer follow-up period (62). A 5-year old Shetland sheepdog had focal seizures and generalized seizures with focal onset for 4 years before implantation of the VNS system. The study followed the patient from 3 months before the implantation up to 1 year after the beginning of the stimulation and described in detail protocol adjustments and their outcomes. After a 1-year follow-up the authors noticed 87% reduction in generalized seizures with focal onset, 89% reduction of focal-to-generalized cluster seizures and 76% decrease of days in which focal-to-generalized seizures appeared (62). Moreover, focal seizures did not progress into generalization, when the owner activated the VNS system externally with a magnet at their onset (62). The cognitive effect of VNS has not been described in canine epileptic patients to date. However, the treatment improved their overall quality of life (62), even in cases when the seizure frequency was not reduced (59).
The most common adverse effects of invasive VNS in humans include postoperative infection (3–6% of cases), vocal cord paresis and lower facial nerve palsy (54, 57, 74). Cardiac side effects such as bradycardia or asystole usually happen during the intraoperative device testing and cease after protocol modification (54). In dogs, side effects associated with VNS include seroma at the site of implantation (53, 59, 68), coughing (62) and muscle twitching during the treatment (60). Cardiac adverse effects such as bradycardia, asystole, and apnea were observed only during intraoperative device testing (59). A prospective cohort study by Harcourt-Brown et al. examined in detail short-term adverse effect in dogs suffering from DRE, reporting cough as the most common one, having developed in 11 out of 14 dogs (61). To eliminate severe coughs (mild and moderate coughing few to several times a day was considered tolerable) the authors introduced protocol modifications based on guidelines published for humans (83). Briefly, when intolerable (harsh or accompanied by retching) coughing was encountered, the authors first changed duty cycle (ON-time: 30 s to 7 s; OFF-time; 5 min to 1.8 min), in the case of no effect they reduced frequency (25 to 20 Hz), and as the last step they reduced current to the highest tolerable level (61). A similar approach was used by Hirashima et al. and proved beneficial for the examined patient (62). Recently, a prospective, double-blind clinical trial aiming to develop new titration protocols has been conducted in human DRE-patients (84). It could lead to better optimization of stimulation parameters and perhaps offer better adjustment strategy for veterinary patients as well.
In recent years, popularity in human epileptology was gained by transcutaneous non-invasive VNS (nVNS), which can be applied either on skin of pinna (auricular branch of the vagus) or along the nerve trajectory on the neck (57). Transcutaneous approaches require higher current intensity, while other stimulation parameters (pulse width, frequency and duty cycles) remain usually similar to invasive VNS (85). Although extensive clinical evidence regarding nVNS is still lacking, data from preliminary human trials showed that this method engages the same neural pathways as invasive VNS (86) and yields seizure reduction in patients with DRE (87–89). nVNS requires less frequent stimulation schedules, which leads to overall less adverse effects (88). Most frequently reported adverse effects of nVNS are headache, ear/facial pain and skin irritation at the stimulation site (57). nVNS constitutes an attractive alternative approach for veterinary medicine, especially for patients not eligible for surgery. In a study published in 2020 by Robinson et al., 14 dog patients with refractory idiopathic epilepsy underwent 8- or 16-week long VNS treatment with a non-invasive stimulator along the cervical portion of the left vagus nerve (63). Nine dogs showed reduction in seizure frequency compared to baseline, among which four were considered good responders (reduction of seizure frequency by ≥50%) (63). Authors also mention that one patient did not show any change and four experienced an increase in seizure frequency (63). More studies would be welcome to elucidate long-term applicability and safety of nVNS in canine epilepsy. Additionally, auricular stimulation could prove beneficial, especially in patients who do not accept manipulations around their neck. However, diverse anatomy of canine ears could negatively influence standardization of such study.
VNS application extends beyond neurological diseases: a growing body of clinical evidence from human patients indicates its suitability for treatment of chronic heart failure (90, 91) or inflammatory diseases such as Crohn's disease (92, 93) or rheumatoid arthritis (94, 95). Recently, nVNS has been proposed and applied to patients with respiratory symptoms of COVID-19 to modulate their inflammatory response (96–98). VNS improved cardiovascular parameters and decreased plasma and heart tissue biomarkers associated with heart failure in a canine model of heart failure (99) and lead to weight loss in dogs (100) and minipigs (101). VNS is undoubtedly a powerful tool, which, if understood and applied properly, could bring a new value to human and veterinary medicine and lead to bidirectional translation of methodology and applications.
Intracranial deep brain stimulation (DBS) in human patients with epilepsy has been investigated for many targets including: cerebellum, subthalamic nucleus, centromedium thalamus and hippocampus (102). DBS is an approved therapy for human focal epilepsy in Europe, USA, Canada, South America and Australia targeting the anterior nuclei of the thalamus (ANT). Responsive neurostimulation (RNS) of the epileptogenic focus and network is approved in the USA (45, 103). The latter approach is further discussed below detailing the closed-loop approach interfering with ongoing ictal activity.
One major question addressed in experimental studies and clinical pilot studies related to the choice of the optimal anatomical target (104). Several potential target regions have been assessed in experimental and clinical pilot studies. Among these the ANT has been selected for a large double-blind randomized multicenter trial. In this initial trial (the SANTE trial) a gradual increase in efficacy was observed in the group of patients with a high frequency 145 Hz bilateral stimulation (46). In this group, the reduction in seizure frequency at 3 month amounted to 40.4% as compared to 14.5% in the control group without stimulation. However, group differences did not reach significance, when considering the entire 3-month stimulation phase. Trial data resulted in approval of ANT for treatment of drug-resistant epilepsy in patients with focal-onset seizures in Europe, Australia and South America, but was delayed in the US until 2018. Long-term follow-up studies provided evidence that efficacy may further increase with prolonged stimulation (105).
Adverse effects described in the initial clinical trial and subsequent studies comprised surgery-related risks including infection, hemorrhage and pain, and stimulation-related effects including headache, sleep disturbance, increased anxiety, and depression (16).
Despite the growing amount of human clinical data and the increasing interest in ANT deep brain stimulation for management of DRE, there a still various open questions concerning the mechanisms, patient selection, electrode placement techniques, and optimal programming (106, 107). In line with the role of the ANT as a network hub in limbic circuits, evidence exists that patients with temporal lobe epilepsy show a favorable response as compared to patients with frontal lobe epilepsy and epilepsies with other locations. Further clinical factors in patient selection include patient preference, operability, history of psychogenic seizure and of psychiatric disorders (106). According to an expert consensus contraindications for ANT deep brain stimulation comprise progressive etiology, psychiatric disorders, MRI contraindications (e.g., older generations electric implants such as cardiac pacemakers, insulin pumps as well as metal foreign bodies), and incomplete seizure diaries (106).
Considering the impact of high frequency stimulation on ictogenesis different mechanisms are discussed. These comprise preferential activation of inhibitory GABAergic neurons, alterations in extracellular potassium concentrations, desynchronization of neuronal activities, and reduction of the recruitment of neurons to epileptic rhythmic activity (108). Recently, attempts with continuous stimulation paradigms (109–111) and multiple thalamic targets using 4-lead devices (112) have been undertaken.
Recently a first case study has been published reporting deep brain stimulation in a canine patient with a progressive increase in seizure severity with frequent cluster seizures and repeated escalation of seizure activity into status epilepticus (64). Considering evidence that the centromedian nucleus of the thalamus (CMNT) can play a role during the early or late phase of an epileptic seizure the stimulation electrode was placed in this thalamic nucleus (113). Case reports in human patients with super-refractory status epilepticus have already suggested DBS of the CMNT or ANT as a rescue therapy for super-refractory status epilepticus (114–119).
Building on this clinical experience, Zamora et al. (64) have applied a multi-scale, rhythm entrained stimulation of the CMNT in a 4-year old, mixed breed dog suffering from idiopathic drug-refractory epilepsy with seizure occurrence associated with awake/sleep phases (Figure 1). The individualized approach considered circardian and infradian rhythmicity and the modulation of biological rhythms by pathophysiological disease-associated mechanisms. The development of respective approaches is of particular interest considering the detrimental impact of DBS on sleep patterns and quality, and the frequent link between ictogenesis and selected sleep or awakening phases in many patients. Thus, an individualized approach which takes biological rhythms into account can on one hand limit adverse effects of DBS and on the other hand better prevent or stop breakthrough seizures by adjusting stimulation to the situation and vigilance states. The adjusted stimulation algorithm applied in the case study comprised three levels with increasing stimulation intensity: (1) circadian basal stimulation during awakefulness and active phases with a day- and a night-time mode (13 Hz, 0.5 or 0.7 mA, respectively), (2) elevated stimulation during the patient's more seizure-prone sleep phases to protect from sleep-associated breakthrough seizures, controlled by activity/inactivity-assessing accelerometry (13 Hz, 1.3 mA), and (3) high-amplitude, high-frequency stimulation aiming to terminate seizures activity in case of breakthrough seizures (burst of 130 Hz, 1.5 mA) (64). The latter mode can be activated by the carer by a tap on the device on the forehead (detected via accelerometry) or by a tablet computer. Implantation and application of the described stimulation algorithm in the canine patient successfully prevented status epilepticus and reduced coherent cluster seizures during the follow-up phase of 7 months (64). Another closed-loop investigational device, sensing and stimulating both hippocampi and anterior nuclei of the thalamus, was implanted in two dogs with idiopathic epilepsy (120, 121). The authors reported that the device tracked successfully seizure activity, but did not report about how successful the device was in suppressing epileptic seizures. Lessons learned from these case studies in canines have now informed human trials. However, further randomized trials are also needed in veterinary medicine to explore if DBS should be developed as a clinical therapeutic tool despites its significant costs and the need of advanced neurosurgical expertise [a summary of the equipment needed and surgical approach can be found in the Supplementary Material of (64)]. In summary, these case studies provided proof-of-concept for adaptive devices combining physiological sensing of activity and vigilance states with a chronotherapy approach. The findings suggest that it is worthwhile to further explore the therapeutic potential and tolerability of multi-scale rhythmic brain stimulation approaches and highlights the dog's role as a translational model.
Transcranial magnetic stimulation (TMS) uses alternating magnetic fields to create a secondary electric field allowing for a non-invasive brain stimulation. Over the past decades repetitive TMS (rTMS) have increased clinical use with low frequency stimulation (<1 Hz) to induce reduced excitability or high frequency stimulation (>1 Hz) to achieve increased excitability (122). The principle behind this is mostly attributed to changes in synaptic plasticity in the form of long-term potentiation or depression (123). In epilepsy, rTMS focuses either on a precise epileptogenic zone or diffuse epileptogenic networks (124, 125). Cortical areas are mainly affected by the rTMS as its effect declines with the square of the distance from the coil; this is in contrast to other neurostimulation techniques such as DBS which can directly affect subcortical areas. Hence, epileptogenic networks located deeper than the cortex (e.g., cerebral or in particular thalamic nuclei) are less likely to be stimulated, unless the coil output is strong and/or the tissues between the coil and the brain (i.e., skull, muscles) are thin enough to allow penetration of the focused magnetic field up to these areas (126). However, studies have shown that rTMS can also have an impact on these subcortical areas through altering the function and connectivity of various neural networks (127–129).
Low frequency rTMS targeting a predetermined cortical area has been considered as a supportive therapy for suppression of seizures in refractory status epilepticus unresponsive to the conventional treatment options (130). Ictal rTMS in human patients provided promising results to abort ongoing prolonged seizures (ranging from few to 40–50 seizures per day) of human patients in inpatient or intensive care units. In a case report (131), one patient was treated with rTMS for 8 days (0.5 Hz, 60 min), which resulted in a marked clinical improvement successfully allowing the patient to be weaned off the respirator and sent to a rehabilitation clinic after discharge. In another study (132) similar improvement was achieved with only a single train of stimulation in one of the two patients (1 Hz, 20 min), whilst another patient (1 Hz, 30 min) responded with increased seizure frequency at 72 h post rTMS after a temporary improvement at 48 h. In another patient rTMS resulted in seizure freedom on lower doses anti-seizure medications after 11 days of stimulation (1 Hz, 10 min) (133). It should be noted that the improvements reported show quite heterogeneous periods ranging from hours to months.
Interictal rTMS, on the other hand, is applied at predetermined intervals and in structured sessions. The first pivotal study of interictal rTMS reported a transient improvement of about 38% reduced incidence of seizures per week in 9 patients during the 4 weeks post-treatment (0.33 Hz, 500 pulses of 2 trains per day, 5 consecutive days) (134). A later study reported improvements only in patients with single epileptic focus (2/4 patients) after a treatment that spanned 4 weeks (0.5 Hz, 100 pulses, applied biweekly), but not in patients with multiple foci (135). Whilst such a beneficial effect was not possible to be reproduced in another study with either single or multiple epileptic foci (136), the heterogeneous results were attributed to the differences in coil type, coil positioning, number and location of the epileptic foci (137, 138). A relatively recent controlled clinical trial (139) reported absence of any improvement after rTMS (0.5 Hz, 1,500 pulses/day, 10 weekdays) in patients with well-defined focal epilepsy during 10 weeks of follow-up period, regardless of the coil type used (8-shaped, circular or sham).
Differences in stimulation frequency (ranging 0.3 to 1 Hz), coil type (8-shaped, cone-shaped or round coils), output (>70% vs. <70%) and positioning (over epileptic focus, vertex or cerebellum) as well as stimulation period (days to weeks) and pattern (consecutive days or intermittent) in addition to the patient heterogeneity and small cohort sizes in clinical studies altogether hinder a direct systematic comparison and deduction of a standardized treatment protocol.
The first report on the use of rTMS in dogs with epilepsy was presented as an abstract during the 60th Annual Meeting of the American-Epilepsy-Society in 2006 (140). Although this study was a non-randomized uncontrolled trial and included only a very small number of subjects (n = 3), its preliminary results showed an increased seizure interval after stimulation compared to the baseline; however, further details on the outcome were not reported. Recently, a single-blinded randomized sham-controlled clinical trial was published by Charalambous et al. (65), which involved 12 dogs with drug-resistant idiopathic epilepsy. A round coil was used over the vertex to globally stimulate the cortex (1 Hz, 90 pulses, 18 trains/day, 5 consecutive days). Significant reductions in the monthly seizure frequency and monthly seizure day frequency were observed in the actively stimulated patients (7/12), but not in the sham treated patients (5/12). In a second trial, the sham group received active stimulation using the same parameters, which also resulted in a significant improvement. The positive effects lasted for 4 months, and no treatment-related side effects were reported. These results are quite encouraging compared to the discrepant reports in human studies. Due to practical reasons, canine patients, unlike human patients, invariably require sedation, which attenuates the extent of cortical excitation achieved by TMS (141). Although anesthetic drugs can suppress the neuronal activity (142–144), neuronal effects of rTMS have been shown in anesthetized rats (145). In an experimental study in dogs, an increase in the cerebral blood flow at the stimulation site was detected under both anesthesia and sedation, with higher but shorter increases in dogs under sedation (146). The study showed that, despite the effect of anesthesia and sedation on the neural networks, comparable and clinically relevant increases on the cerebral blood flow can be achieved in dogs when stimulated with rTMS.
A fundamental gap in epileptology is the lack of accurate seizure diaries. In fact, all pharmacologic and neurostimulation device studies to date have relied on patient diaries despite their unreliability (147, 148). While NeuroPace RNS and Medtronic Percept have recording capabilities, they do not reliably provide accurate seizure diaries (149, 150).
Recent device advances including continuous intracranial electroencephalography (iEEG) streaming, embedded and off-the-body detection algorithms and increasing on device data storage are poised to overcome this important engineering gap (151–153).
The potential importance of seizure forecasting is widely recognized (154). Evidence from RNS Neuropace Inc. investigations support that seizures are difficult to stop once they are detected on clinical iEEG macroelectrodes. In clinical practice this generally leads to using a highly sensitive detector resulting in >100 responsive electrical stimulations a day for optimal efficacy. Forecasting seizures with relatively good sensitivity has been demonstrated in canines (147, 155) and humans (148, 156) using continuously recorded iEEG. This has opened a potential new therapeutic window where neurostimulation or pharmacological treatments could be adjusted according to the probability of seizure occurrence (157).
The advances in device technology have yielded important insights into the generation of seizures. In particular, it is now well-established that seizures and seizure risk show multidien rhythms (158) in humans (159, 160) and canines (161). This important observation, that was first reported nearly 100 years ago (162), should prove useful for seizure forecasting and intelligent chronotherapy (64, 120).
Neurostimulation (VNS, DBS, and RNS) are established therapies in human DRE. Transcutaneous VNS and TMS appear well-tolerated, but there are currently insufficient data to support the efficacy of any of these modalities for drug-resistant epilepsy (163). Although each of the described approaches possesses its specific advantages and challenges (Figure 2), they all proved to reduce seizure frequency and disease burden in both human and veterinary medicine. These methods are mostly associated with mild, often local side effects, therefore should be considered as alternative long-term treatment option of DRE in canine patients. However, the application of brain stimulation is currently rather limited to halting seizures on their onset, either in an open-loop or in a closed-loop manner. A reasonable next step in the research of neurostimulation in epileptology would be exploration of its anti-epileptogenic potential and possibility of disease modification (Figure 3).
Figure 2. Advantages and challenges related to each of the neurostimulation methods used in veterinary medicine to treat drug-resistant epilepsy in dogs. VNS, vagus nerve stimulation; DBS, deep brain stimulation; TMS, transcranial magnetic stimulation.
Figure 3. Future perspectives for neurostimulation in drug-resistant epilepsy in dogs. Long-term stimulation might lead to disease modifying effects through alterations in neuronal networks (upper part) or anti-inflammatory effects (middle part), which might be utilized to curb epileptogenic processes. Use of repetitive transcranial magnetic stimulation (rTMS) or transcranial direct current stimulation (tDCS) might both be considered non-invasive strategies for long-term stimulation in patients not eligible for surgery.
Empirical evidence of the influence of electrical stimulation on epileptogenic process is already available from animal models. DBS performed in irregular intervals during interictal phases slowed progression of kindling-induced epileptogenesis and decreased generalized seizure duration in rats (164). High frequency DBS applied during 3 months in a macaque model of mesial temporal lobe epilepsy decreased levels of mRNA of genes involved in focal-adhesion and extracellular matrix-receptor interaction pathway (165), known to be up-regulated in epileptogenesis (166). Low frequency stimulation improved cognitive functions and memory during epileptogenesis in a kindling rat model (167), suggesting its influence on vast neuronal networks. In case of confirmation of these processes taking place in a canine brain, this might be of future interest for dogs following epileptogenic insults such as traumatic brain injury or virus encephalitis.
It is difficult to pinpoint, which exact mechanisms are involved in long-term outcomes of neurostimulation in epilepsy. Hypothetically, they could arise due to modifications in epileptic networks, their anti-inflammatory effects or due to other, more elusive processes, such as involvement of gut microbiota or anti-oxidative processes.
The effect on neuronal networks can be explained in the context of prolonged stimulation. The number of applied treatments may exceed the number of actual seizures and occur predominantly in the interictal period. Long-term iEEG recordings in patients with focal epilepsy undergoing chronic responsive neurostimulation system (RNS) therapy revealed reorganization of their brain networks: connectivity was lower between epileptic foci than in brain regions outside the foci (34). This effect was more prominent in patients with a better outcome in seizure reduction, which may suggest that neurostimulation helps disrupt pathological epileptogenic networks. However, epileptic networks could later re-adapt to the stimulation pattern, which might be responsible for the emergence of a “honeymoon phase” after the stimulation—this effect has been observed in patients with Parkinson's disease treated with DBS (168, 169). Therefore, if this change is permanent or why in some patients an alternate epileptogenic network re-organizes does require further research. In this context it needs to be considered that the outcome is also influenced by the parameters of the stimulation: e.g., in patients with Parkinson's disease, DBS performed with low frequency signals promoted circuit synchronization, whereas high frequency DBS suppressed synchronous activity (35). Long-term VNS resulted in changes in neural networks as well. Chronic VNS performed in naïve rats led to long-lasting increases of doublecortin-positive cells in the hippocampus as well as their dendritic complexity and expression of brain-derived neurotrophic factor (170), all of which are hallmarks of neuroplasticity. The data from patients additionally supports the evidence that VNS modulates neuronal networks into a less epilepsy-prone state (108). Moreover, unlike DBS, VNS does not induce a “honeymoon phase”—on the contrary, its effect seems to improve with time, which could indicate a beneficial influence of this stimulation mode on epileptic networks.
Inflammation is a process inseparably connected to epilepsy. Seizures can provoke production of pro-inflammatory cytokines, prostaglandins and chemokines by glia and neurons, by which they recruit immune cells from peripheral blood and lead to brain inflammation (171). Inversely, activation on innate immunity receptors causes rapid changes in ionic fluxes in neurons, which results in hyperexcitability and leads to onset or progression of a seizure (172). Brain inflammation also modifies expression of genes involved in production of neurotransmitter receptors, in neurogenesis and cell death and survivability (171, 172). This leads to network reorganization and changes in neuronal excitability, which can result in precipitation of the epileptogenic process.
The vagus nerve, as a part of the autonomic nervous system, is heavily involved in modulation of immune response (173). Stimulation of both vagal efferents and afferents has shown anti-inflammatory effects, attributed to cholinergic signaling (174). Experimental data supports positive effect of VNS on neuroinflammation in various animal disease models (175–178). Importantly, chronic VNS decreased levels of pro-inflammatory cytokines in hippocampus of rats with spontaneous recurrent seizures (178). Moreover, in a traumatic brain injury (TBI) rat model, VNS significantly suppressed expression of nuclear factor-kappa B (176), which is critically important for both inflammation and epileptogenesis (171). These findings could prove vital for prevention of disease development after epileptogenic insults.
Anti-inflammatory effects of DBS have also been established in animal models of epilepsy. DBS of ANT reduced blood-brain barrier disruption and albumin extravasation (179) as well as inflammation and apoptosis in rats with chemically induced status epilepticus (179, 180). It might suggest positive influence of stimulation on anti-inflammatory state of the brain is more pronounced than local inflammation caused by electrode insertion.
There might be other processes influencing to lesser extent the onset and progression of epileptogenesis, which might be targeted by brain stimulation. Recently, considerable insight has been gained into the role gastrointestinal microbiota plays in epilepsy (181). Even though short VNS did not alter gut microbiota composition in mice (182), repeated TMS of prefrontal cortex influenced rectal function of human volunteers, supposedly also affecting their microbiota (183). Another important epileptogenic factor is oxidative stress, leading to mitochondrial dysfunction and ionic dysbalance in neurons (184). Anti-oxidative effects have been reported in TMS in humans (185) and described in ischemic myocardiac injury in dogs (186), so it is plausible to assume they might also play a role in epileptic brains.
Canine patients with epilepsy have been included in clinical research involving three brain stimulation methods: VNS, DBS, and TMS. One of non-invasive stimulation methods used as treatment in humans with epilepsy and not researched in dogs as to date is transcranial direct current stimulation (tDCS). It utilizes weak (1–2 mA), constant, unidirectional flow of electrical charge applied to the scalp via electrodes mounted on a skin using an electrolytic contact medium (e.g. conductive gel) (187). The current modulates membrane potentials, leading to alteration of neuronal excitability. The effect of the stimulation depends on the direction and intensity of the applied current—anodal (positive) tDCS generally leads to increase of cortical excitability, while cathodal (negative) tDCS results in inhibition (187). Several studies in humans showed promising results including suppression of epileptiform discharges and decrease of seizure frequency following tDCS treatment (32, 187). Among adverse effects, minor skin itching and irritation at the stimulation site were reported (187). Considering lack of invasiveness, positive stimulation results, relatively short stimulation sessions (usually 20 min a day) and lack of serious side effects described, tDCS poses an excellent opportunity for canines with epilepsy (Figure 3).
To introduce new methodology into veterinary medicine and further establish existing ones, more clinical research in canines is needed. This would allow development of reliable protocols to improve the anti-seizure effect and avoid undesirable side effects, so that the neurostimulation becomes more effective and more safe for the patients. Equally important is further elucidation of the mechanisms governing respective stimulation approaches. So far, thanks to the basic research on dogs, it was possible to identify parameters for VNS in dogs (58) and describe its effect on seizure threshold and monoamine concentration (60). Nevertheless, further research is vital to better understand methods applied to the patients and ascertain the best possible management of refractory epilepsy.
MN, HV, HP, GW, TD, and MÜ: outline of the review. MN, HV, HP, GW, MÜ, MC, SB, and SM: writing of the manuscript. TD and HV: supervision. All authors contributed to the article and approved the submitted version.
MN and MÜ are financed from ZK 17 Zukunftskolleg provided by the Austrian Science Fund (FWF – Der Wissenschaftsfonds). GW has received funding from National Institutes of Health (U01-NS073557, R01-NS92882, and UH2/3-NS95495) and the Epilepsy Foundation Epilepsy Innovation Institute My Seizure Gauge. This open access publication was funded by the Deutsche Forschungsgemeinschaft (DFG, German Research Foundation) - 491094227 Open Access Publication Costs and the University of Veterinary Medicine Hannover, Foundation.
GW has rights to receive future royalties from the licensing of technology to Cadence Neuroscience Inc, and has received research support from Medtronic, LivaNova, and was previously on the scientific advisory board of NeuroPace Inc. HV served as paid consultant in the field of epilepsy for Boehringer Ingelheim, CEVA animal health, Nestle Purina and served as contract researcher for: Nestle Purina, Desitin Pharma and Boehringer Ingelheim. HP received funding for consulting, talks and research collaborations from Eisai, Zogenix, Elanco, Roche, Exeed Epidarex, Arvelle and MSD.
The remaining authors declare that the research was conducted in the absence of any commercial or financial relationships that could be construed as a potential conflict of interest.
All claims expressed in this article are solely those of the authors and do not necessarily represent those of their affiliated organizations, or those of the publisher, the editors and the reviewers. Any product that may be evaluated in this article, or claim that may be made by its manufacturer, is not guaranteed or endorsed by the publisher.
Clipart icons by Servier https://smart.servier.com/ are licensed under CC-BY 3.0 Unported https://creativecommons.org/licenses/by/3.0/ and were imported from Bioicons.
1. Beghi E. The epidemiology of epilepsy. Neuroepidemiology. (2020) 54:185–91. doi: 10.1159/000503831
2. Hulsmeyer VI, Fischer A, Mandigers PJ, DeRisio L, Berendt M, Rusbridge C, et al. International Veterinary Epilepsy Task Force's current understanding of idiopathic epilepsy of genetic or suspected genetic origin in purebred dogs. BMC Vet Res. (2015) 11:175. doi: 10.1186/s12917-015-0463-0
3. Berendt M, Gullov CH, Fredholm M. Focal epilepsy in the Belgian shepherd: evidence for simple Mendelian inheritance. J Small Anim Pract. (2009) 50:655–61. doi: 10.1111/j.1748-5827.2009.00849.x
4. Sultana B, Panzini MA, Veilleux Carpentier A, Comtois J, Rioux B, Gore G, et al. Incidence and prevalence of drug-resistant epilepsy: a systematic review and meta-analysis. Neurology. (2021) 96:805–17. doi: 10.1212/WNL.0000000000011839
5. Thomas WB. Idiopathic epilepsy in dogs and cats. Vet Clin N Am Small Anim Pract. (2010) 40:161–79. doi: 10.1016/j.cvsm.2009.09.004
6. Loscher W, Potschka H, Sisodiya SM, Vezzani A. Drug resistance in epilepsy: clinical impact, potential mechanisms, and new innovative treatment options. Pharmacol Rev. (2020) 72:606–38. doi: 10.1124/pr.120.019539
7. Tang F, Hartz AMS, Bauer B. Drug-resistant epilepsy: multiple hypotheses, few answers. Front Neurol. (2017) 8:301. doi: 10.3389/fneur.2017.00301
8. Fattorusso A, Matricardi S, Mencaroni E, Dell'Isola GB, Di Cara G, Striano P, et al. The pharmacoresistant epilepsy: an overview on existant and new emerging therapies. Front Neurol. (2021) 12:674483. doi: 10.3389/fneur.2021.674483
9. Han FY, Conboy-Schmidt L, Rybachuk G, Volk HA, Zanghi B, Pan Y, et al. Dietary medium chain triglycerides for management of epilepsy: new data from human, dog, and rodent studies. Epilepsia. (2021) 62:1790–806. doi: 10.1111/epi.16972
10. McGrath S, Bartner LR, Rao S, Packer RA, Gustafson DL. Randomized blinded controlled clinical trial to assess the effect of oral cannabidiol administration in addition to conventional antiepileptic treatment on seizure frequency in dogs with intractable idiopathic epilepsy. J Am Vet Med Assoc. (2019) 254:1301–8. doi: 10.2460/javma.254.11.1301
11. Ottestad E, Orlovich DS. History of peripheral nerve stimulation-update for the 21st century. Pain Med. (2020) 21(Suppl. 1):S3–5. doi: 10.1093/pm/pnaa165
12. Peterson JTB, Deer TR. A history of neurostimulation. In: Deer TR, Leong MS, Buvanendran A, Gordin V, Kim PS, Panchal SJ,. editors. Comprehensive Treatment of Chronic Pain by Medical, Interventional, and Integrative Approaches: The AMERICAN ACADEMY OF PAIN MEDICINE Textbook on Patient Management. New York, NY: Springer New York (2013). p. 583–6.
13. Lin Y, Wang Y. Neurostimulation as a promising epilepsy therapy. Epilepsia Open. (2017) 2:371–87. doi: 10.1002/epi4.12070
14. Hariz MI, Blomstedt P, Zrinzo L. Deep brain stimulation between 1947 and 1987: the untold story. Neurosurg Focus. (2010) 29:E1. doi: 10.3171/2010.4.FOCUS10106
15. Ponce GV, Klaus J, Schutter DJLG. A brief history of cerebellar neurostimulation. Cerebellum. (2021) doi: 10.1007/s12311-021-01310-2. [Epub ahead of print].
16. Fisher RS, Velasco AL. Electrical brain stimulation for epilepsy. Nat Rev Neurol. (2014) 10:261–70. doi: 10.1038/nrneurol.2014.59
17. Worrell GA. Electrical brain stimulation for epilepsy and emerging applications. J Clin Neurophysiol. (2021) 38:471–7. doi: 10.1097/WNP.0000000000000819
18. Kaye AD, Ridgell S, Alpaugh ES, Mouhaffel A, Kaye AJ, Cornett EM, et al. Peripheral nerve stimulation: a review of techniques and clinical efficacy. Pain Ther. (2021) 10:961–72. doi: 10.1007/s40122-021-00298-1
19. Ni YC, Yang LQ, Han R, Guo GW, Huang ST, Weng LL, et al. Implantable peripheral nerve stimulation for trigeminal neuropathic pain: a systematic review and meta-analysis. Neuromodulation. (2021) 24:983–91. doi: 10.1111/ner.13421
20. Lanier ST, Hill JR, Dy CJ, Brogan DM. Evolving techniques in peripheral nerve regeneration. J Hand Surg Am Vol. (2021) 46:695–702. doi: 10.1016/j.jhsa.2021.04.019
21. Kim K. A review of haptic feedback through peripheral nerve stimulation for upper extremity prosthetics. Curr Opin Biomed Eng. (2022) 14:9–17. doi: 10.1016/j.cobme.2022.100368
22. Khan S, Khan F, Sikander QU, Alam MM, Su'Ud MM. Intelligent deep brain stimulation systems: a general review. IEEE Access. (2021) 9:136929–43. doi: 10.1109/ACCESS.2021.3105457
23. Kogan M, McGuire M, Riley J. Deep brain stimulation for Parkinson disease. Neurosurg Clin N Am. (2019) 30:137–+. doi: 10.1016/j.nec.2019.01.001
24. Lyons MK. Deep brain stimulation: current and future clinical applications. Mayo Clin Proc. (2011) 86:662–72. doi: 10.4065/mcp.2011.0045
25. Sonmez AI, Camsari DD, Nandakumar AL, Vande Voort JL, Kung S, Lewis CP, et al. Accelerated TMS for Depression: a systematic review and meta-analysis. Psychiatry Res. (2019) 273:770–81. doi: 10.1016/j.psychres.2018.12.041
26. Ward HE, Hwynn N, Okun MS. Update on deep brain stimulation for neuropsychiatric disorders. Neurobiol Dis. (2010) 38:346–53. doi: 10.1016/j.nbd.2010.01.011
27. Allawala A, Bijanki KR, Goodman W, Cohn JF, Viswanathan A, Yoshor D, et al. A novel framework for network-targeted neuropsychiatric deep brain stimulation. Neurosurgery. (2021) 89:E116–21. doi: 10.1093/neuros/nyab112
28. Owen SLF, Green AL, Nandi D, Bittar RG, Wang S, Aziz TZ. Deep brain stimulation for neuropathic pain. Neuromodulation. (2006) 9:100–6. doi: 10.1111/j.1525-1403.2006.00049.x
29. Wolter T. Spinal cord stimulation for neuropathic pain: current perspectives. J Pain Res. (2014) 7:651–63. doi: 10.2147/JPR.S37589
30. Lockman J, Fisher RS. Therapeutic brain stimulation for epilepsy. Neurol Clin. (2009) 27:1031–40. doi: 10.1016/j.ncl.2009.06.005
31. Nagel SJ, Najm IM. Deep brain stimulation for epilepsy. Neuromodulation. (2009) 12:270–80. doi: 10.1111/j.1525-1403.2009.00239.x
32. VanHaerents S, Chang BS, Rotenberg A, Pascual-Leone A, Shafi MM. Noninvasive brain stimulation in epilepsy. J Clin Neurophysiol. (2020) 37:118–30. doi: 10.1097/WNP.0000000000000573
33. Rich S, Hutt A, Skinner FK, Valiante TA, Lefebvre J. Neurostimulation stabilizes spiking neural networks by disrupting seizure-like oscillatory transitions. Sci Rep. (2020) 10:15408. doi: 10.1038/s41598-020-72335-6
34. Khambati AN, Shafi A, Rao VR, Chang EF. Long-term brain network reorganization predicts responsive neurostimulation outcomes for focal epilepsy. Sci Transl Med. (2021) 13:eabf6588. doi: 10.1126/scitranslmed.abf6588
35. Brown P, Mazzone P, Oliviero A, Altibrandi MG, Pilato F, Tonali PA, et al. Effects of stimulation of the subthalamic area on oscillatory pallidal activity in Parkinson's disease. Exp Neurol. (2004) 188:480–90. doi: 10.1016/j.expneurol.2004.05.009
36. Jakobs M, Fomenko A, Lozano AM, Kiening KL. Cellular, molecular, and clinical mechanisms of action of deep brain stimulation-a systematic review on established indications and outlook on future developments. EMBO Mol Med. (2019) 11:e9575. doi: 10.15252/emmm.201809575
37. Xu KY, Liu ZY, Wang LK, Wu GF, Liu T. Influence of hippocampal low-frequency stimulation on GABA(A) R alpha 1, ICER and BNDF expression level in brain tissues of amygdala-kindled drug-resistant temporal lobe epileptic rats. Brain Res. (2018) 1698:195–203. doi: 10.1016/j.brainres.2018.08.013
38. Smirnova EY, Chizhov AV, Zaitsev AV. Presynaptic GABA(B) receptors underlie the antiepileptic effect of low-frequency electrical stimulation in the 4-aminopyridine model of epilepsy in brain slices of young rats. Brain Stimul. (2020) 13:1387–95. doi: 10.1016/j.brs.2020.07.013
39. Wang Y, Melvin R, Bemis LT, Worrell GA, Wang H-L. Programmable modulation for extracellular vesicles. bioRxiv [Preprint]. (2019) 566448. doi: 10.1101/566448
40. Wang Y, Burghardt TP, Worrell GA, Wang H-L. The frequency-dependent effect of electrical fields on the mobility of intracellular vesicles in astrocytes. Biochem Biophys Res Commun. (2021) 534:429–35. doi: 10.1016/j.bbrc.2020.11.064
41. Gellner AK, Reis J, Fritsch B. Glia: a neglected player in non-invasive direct current brain stimulation. Front Cell Neurosci. (2016) 10:188. doi: 10.3389/fncel.2016.00188
42. Vedam-Mai V, van Battum EY, Kamphuis W, Feenstra MG, Denys D, Reynolds BA, et al. Deep brain stimulation and the role of astrocytes. Mol Psychiatry. (2012) 17:124–31:15. doi: 10.1038/mp.2011.61
43. McNamara JO, Constant Byrne M, Dasheiff RM, Gregory Fitz J. The kindling model of epilepsy: a review. Prog Neurobiol. (1980) 15:139–59. doi: 10.1016/0301-0082(80)90006-4
44. Brandt C, Glien M, Potschka H, Volk H, Löscher W. Epileptogenesis and neuropathology after different types of status epilepticus induced by prolonged electrical stimulation of the basolateral amygdala in rats. Epilepsy Res. (2003) 55:83–103. doi: 10.1016/S0920-1211(03)00114-1
45. Morrell MJ. Responsive cortical stimulation for the treatment of medically intractable partial epilepsy. Neurology. (2011) 77:1295–304. doi: 10.1212/WNL.0b013e3182302056
46. Fisher R, Salanova V, Witt T, Worth R, Henry T, Gross R, et al. Electrical stimulation of the anterior nucleus of thalamus for treatment of refractory epilepsy. Epilepsia. (2010) 51:899–908. doi: 10.1111/j.1528-1167.2010.02536.x
47. Brodie MJ, Leach JP. Success or failure with antiepileptic drug therapy: beyond empiricism? Neurology. (2003) 60:162–3. doi: 10.1212/01.WNL.0000049681.91195.B0
48. Penry JK, Dean JC. Prevention of intractable partial seizures by intermittent vagal-stimulation in humans - preliminary-results. Epilepsia. (1990) 31:S40–3. doi: 10.1111/j.1528-1157.1990.tb05848.x
49. Groves DA, Brown VJ. Vagal nerve stimulation: a review of its applications and potential mechanisms that mediate its clinical effects. Neurosci Biobehav Rev. (2005) 29:493–500. doi: 10.1016/j.neubiorev.2005.01.004
50. Zabara J. Inhibition of experimental seizures in canines by repetitive vagal-stimulation. Epilepsia. (1992) 33:1005–12. doi: 10.1111/j.1528-1157.1992.tb01751.x
51. Fisher B, DesMarteau JA, Koontz EH, Wilks SJ, Melamed SE. Responsive vagus nerve stimulation for drug resistant epilepsy: a review of new features and practical guidance for advanced practice providers. Front Neurol. (2021) 11:610379. doi: 10.3389/fneur.2020.610379
52. Attenello F, Amar AP, Liu C, Apuzzo MLJ. Theoretical basis of vagus nerve stimulation. Prog Neurol Surg. (2015) 29:20–8. doi: 10.1159/000434652
53. Martlé V, Peremans K, Raedt R, Vermeire S, Vonck K, Boon P, et al. Regional brain perfusion changes during standard and microburst vagus nerve stimulation in dogs. Epilepsy Res. (2014) 108:616–22. doi: 10.1016/j.eplepsyres.2014.02.004
54. Yang J, Phi JH. The present and future of vagus nerve stimulation. J Korean Neurosurg Soc. (2019) 62:344–52. doi: 10.3340/jkns.2019.0037
55. Rosso P, Iannitelli A, Pacitti F, Quartini A, Fico E, Fiore M, et al. Vagus nerve stimulation and Neurotrophins: a biological psychiatric perspective. Neurosci Biobehav Rev. (2020) 113:338–53. doi: 10.1016/j.neubiorev.2020.03.034
56. Ulloa L, Quiroz-Gonzalez S, Torres-Rosas R. Nerve stimulation: immunomodulation and control of inflammation. Trends Mol Med. (2017) 23:1103–20. doi: 10.1016/j.molmed.2017.10.006
57. Mertens A, Raedt R, Gadeyne S, Carrette E, Boon P, Vonck K. Recent advances in devices for vagus nerve stimulation. Expert Rev Med Devices. (2018) 15:527–39. doi: 10.1080/17434440.2018.1507732
58. Yoo PB, Lubock NB, Hincapie JG, Ruble SB, Hamann JJ, Grill WM. High-resolution measurement of electrically-evoked vagus nerve activity in the anesthetized dog. J Neural Eng. (2013) 10:026003. doi: 10.1088/1741-2560/10/2/026003
59. Muñana KR, Vitek SM, Tarver WB, Saito M, Skeen TM, Sharp NJ, et al. Use of vagal nerve stimulation as a treatment for refractory epilepsy in dogs. J Am Vet Med Assoc. (2002) 221:977–83. doi: 10.2460/javma.2002.221.977
60. Martlé V, Raedt R, Waelbers T, Smolders I, Vonck K, Boon P, et al. The effect of vagus nerve stimulation on CSF monoamines and the PTZ seizure threshold in dogs. Brain Stimul. (2015) 8:1–6. doi: 10.1016/j.brs.2014.07.032
61. Harcourt-Brown TR, Carter M. Implantable vagus nerve stimulator settings and short-term adverse effects in epileptic dogs. J Vet Intern Med. (2021) 35:2350–8. doi: 10.1111/jvim.16226
62. Hirashima J, Saito M, Igarashi H, Takagi S, Hasegawa D. Case report: 1-year follow-up of vagus nerve stimulation in a dog with drug-resistant epilepsy. Front Vet Sci. (2021) 8:708407. doi: 10.3389/fvets.2021.708407
63. Robinson K, Platt S, Stewart G, Reno L, Barber R, Boozer L. Feasibility of non-invasive vagus nerve stimulation (gammaCORE VET™) for the treatment of refractory seizure activity in dogs. Front Vet Sci. (2020) 7:569739. doi: 10.3389/fvets.2020.569739
64. Zamora M, Meller S, Kajin F, Sermon JJ, Toth R, Benjaber M, et al. Case report: embedding “digital chronotherapy” into medical devices-a canine validation for controlling status epilepticus through multi-scale rhythmic brain stimulation. Front Neurosci. (2021) 15:10. doi: 10.3389/fnins.2021.734265
65. Charalambous M, Van Ham L, Broeckx BJG, Roggeman T, Carrette S, Vonck K, et al. Repetitive transcranial magnetic stimulation in drug-resistant idiopathic epilepsy of dogs: a noninvasive neurostimulation technique. J Vet Intern Med. (2020) 34:2555–61. doi: 10.1111/jvim.15919
66. Wheless JW, Gienapp AJ, Ryvlin P. Vagus nerve stimulation (VNS) therapy update. Epilepsy Behav. (2018) 88:2–10. doi: 10.1016/j.yebeh.2018.06.032
67. Schachter SC, Saper CB. Vagus nerve stimulation. Epilepsia. (1998) 39:677–86. doi: 10.1111/j.1528-1157.1998.tb01151.x
68. Martlé V, Van Ham LML, Boon P, Caemaert J, Tshamala M, Vonck K, et al. Vagus nerve stimulator placement in dogs: surgical implantation technique, complications, long-term follow-up, and practical considerations. Vet Surg. (2016) 45:71–8. doi: 10.1111/vsu.12427
69. Castoro MA, Yoo PB, Hincapie JG, Hamann JJ, Ruble SB, Wolf PD, et al. Excitation properties of the right cervical vagus nerve in adult dogs. Exp Neurol. (2011) 227:62–8. doi: 10.1016/j.expneurol.2010.09.011
70. Romero-Ugalde HM, Le Rolle V, Bonnet JL, Henry C, Mabo P, Carrault G, et al. Closed-loop vagus nerve stimulation based on state transition models. IEEE Trans Biomed Eng. (2018) 65:1630–8. doi: 10.1109/TBME.2017.2759667
71. Muthiah N, Akwayena E, Vodovotz L, Sharma N, Jeong JH, White GE, et al. Comparison of traditional and closed loop vagus nerve stimulation for treatment of pediatric drug-resistant epilepsy: a propensity-matched retrospective cohort study. Seizure Eur J Epilepsy. (2022) 94:74–81. doi: 10.1016/j.seizure.2021.11.016
72. Vonck K, Raedt R, Boon P. Vagus nerve stimulation and the postictal state. Epilepsy Behav. (2010) 19:182–5. doi: 10.1016/j.yebeh.2010.06.020
73. Panebianco M, Zavanone C, Dupont S, Restivo DA, Pavone A. Vagus nerve stimulation therapy in partial epilepsy: a review. Acta Neurol Belg. (2016) 116:241–8. doi: 10.1007/s13760-016-0616-3
74. Toffa DH, Touma L, El Meskine T, Bouthillier A, Nguyen DK. Learnings from 30 years of reported efficacy and safety of vagus nerve stimulation (VNS) for epilepsy treatment: a critical review. Seizure. (2020) 83:104–23. doi: 10.1016/j.seizure.2020.09.027
75. Salinsky MC, Uthman BM, Ristanovic RK, Wernicke JF, Tarver WB. Vagus nerve stimulation for the treatment of medically intractable seizures. Results of a 1-year open-extension trial. Vagus Nerve Stimulation Study Group. Arch Neurol. (1996) 53:1176–80. doi: 10.1001/archneur.1996.00550110128021
76. DeGiorgio CM, Schachter SC, Handforth A, Salinsky M, Thompson J, Uthman B, et al. Prospective long-term study of vagus nerve stimulation for the treatment of refractory seizures. Epilepsia. (2000) 41:1195–200. doi: 10.1111/j.1528-1157.2000.tb00325.x
77. Morris GL 3rd, Mueller WM. Long-term treatment with vagus nerve stimulation in patients with refractory epilepsy. The Vagus Nerve Stimulation Study Group E01-E05. Neurology. (1999) 53:1731–5. doi: 10.1212/WNL.53.8.1731
78. Freire RC, Cabrera-Abreu C, Milev R. Neurostimulation in anxiety disorders, post-traumatic stress disorder, and obsessive-compulsive disorder. In: Kim YK,. editor. Anxiety Disorders: Rethinking and Understanding Recent Discoveries. Advances in Experimental Medicine and Biology. Singapore: Springer-Verlag Singapore Pte Ltd (2020). p. 331–46.
79. Weymar M, Zaehle T. Editorial: new frontiers in noninvasive brain stimulation: cognitive, affective and neurobiological effects of transcutaneous vagus nerve stimulation. Front Psychol. (2021) 12:694723. doi: 10.3389/fpsyg.2021.694723
80. Schachter SC. Vagus nerve stimulation: mood and cognitive effects. Epilepsy Behav. (2004) 5:S56–9. doi: 10.1016/j.yebeh.2003.11.007
81. Burger AM, Van der Does W, Thayer JF, Brosschot JF, Verkuil B. Transcutaneous vagus nerve stimulation reduces spontaneous but not induced negative thought intrusions in high worriers. Biol Psychol. (2019) 142:80–9. doi: 10.1016/j.biopsycho.2019.01.014
82. Ryvlin P, So EL, Gordon CM, Hesdorffer DC, Sperling MR, Devinsky O, et al. Long-term surveillance of SUDEP in drug-resistant epilepsy patients treated with VNS therapy. Epilepsia. (2018) 59:562–72. doi: 10.1111/epi.14002
83. Heck C, Helmers SL, DeGiorgio CM. Vagus nerve stimulation therapy, epilepsy, and device parameters - scientific basis and recommendations for use. Neurology. (2002) 59:S31–7. doi: 10.1212/WNL.59.6_suppl_4.S31
84. Vagus Nerve Stimulation Titration Protocol to Improve Tolerance and Accelerate Adaptation. Available online at: https://ClinicalTrials.gov/show/NCT02385526 (accessed February 22, 2022).
85. Thompson SL, O'Leary GH, Austelle CW, Gruber E, Kahn AT, Manett AJ, et al. A review of parameter settings for invasive and non-invasive Vagus Nerve Stimulation (VNS) Applied in neurological and psychiatric disorders. Front Neurosci. (2021) 15:709436. doi: 10.3389/fnins.2021.709436
86. Assenza G, Campana C, Colicchio G, Tombini M, Assenza F, Di Pino G, et al. Transcutaneous and invasive vagal nerve stimulations engage the same neural pathways: in-vivo human evidence. Brain Stimul. (2017) 10:853–4. doi: 10.1016/j.brs.2017.03.005
87. Ben-Menachem E, Rydenhag B, Silander H. Preliminary experience with a new system for vagus nerve stimulation for the treatment of refractory focal onset seizures. Epilepsy Behav. (2013) 29:416–9. doi: 10.1016/j.yebeh.2013.08.014
88. Ben-Menachem E, Revesz D, Simon BJ, Silberstein S. Surgically implanted and non-invasive vagus nerve stimulation: areview of efficacy, safety and tolerability. Eur J Neurol. (2015) 22:1260–8. doi: 10.1111/ene.12629
89. Hamer HM, Bauer S. Lessons learned from transcutaneous vagus nerve stimulation (tVNS). Epilepsy Res. (2019) 153:83–4. doi: 10.1016/j.eplepsyres.2019.02.015
90. Akdemir B, Benditt DG. Vagus nerve stimulation: an evolving adjunctive treatment for cardiac disease. Anatolian J Cardiol. (2016) 16:804–10. doi: 10.14744/AnatolJCardiol.2016.7129
91. Li MH, Zheng C, Kawada T, Inagaki M, Uemura K, Sugimachi M. Chronic vagal nerve stimulation exerts additional beneficial effects on the beta-blocker-treated failing heart. J Physiol Sci. (2019) 69:295–303. doi: 10.1007/s12576-018-0646-0
92. Bonaz B, Pellissier S, Mathieu N, Hoffmann D, Trocme C, Baudrant-Boga M, et al. Vagus nerve stimulation in Crohn's disease. J Crohns Colitis. (2014) 8:S188–9. doi: 10.1016/S1873-9946(14)60420-7
93. Benjamin S, Kristine P, Kevin T, James M. Non-invasive vagal nerve stimulation to treat Crohn disease and ulcerative colitis in children and young adults: a proof-of-concept clinical trial. Am J Gastroenterol. (2021) 116:S19–S. doi: 10.14309/01.ajg.0000798888.27546.b9
94. Courties A, Berenbaum F, Sellam J. Vagus nerve stimulation in musculoskeletal diseases. Joint Bone Spine. (2021) 88:105149. doi: 10.1016/j.jbspin.2021.105149
95. Koopman FA, Chavan SS, Miljko S, Grazio S, Sokolovic S, Schuurman PR, et al. Vagus nerve stimulation inhibits cytokine production and attenuates disease severity in rheumatoid arthritis. Proc Natl Acad Sci USA. (2016) 113:8284–9. doi: 10.1073/pnas.1605635113
96. Rangon CM, Barruet R, Mazouni A, Le Cossec C, Thevenin S, Guillaume J, et al. Auricular neuromodulation for mass vagus nerve stimulation: insights from SOS COVID-19 a multicentric, randomized, controlled, double-blind french pilot study. Front Physiol. (2021) 12:704599. doi: 10.3389/fphys.2021.704599
97. Staats P, Giannakopoulos G, Blake J, Liebler E, Levy RM. The use of non-invasive vagus nerve stimulation to treat respiratory symptoms associated with COVID-19: a theoretical hypothesis and early clinical experience. Neuromodulation. (2020) 23:784–8. doi: 10.1111/ner.13172
98. Boezaart AP, Botha DA. Treatment of stage 3 COVID-19 with transcutaneous auricular vagus nerve stimulation drastically reduces interleukin-6 blood levels: a report on two cases. Neuromodulation. (2021) 24:166–7. doi: 10.1111/ner.13293
99. Hamann JJ, Ruble SB, Stolen C, Wang M, Gupta RC, Rastogi S, et al. Vagus nerve stimulation improves left ventricular function in a canine model of chronic heart failure. Eur J Heart Fail. (2013) 15:1319–26. doi: 10.1093/eurjhf/hft118
100. Reddy R, Horovitz J, Roslin M. Chronic bilateral vagal nerve stimulation (VNS) changes eating behavior resulting in weight loss in a canine model. J Am Coll Surg. (2000) 191:S27–8. doi: 10.1016/S1072-7515(00)00452-X
101. Val-Laillet D, Biraben A, Randuineau G, Malbert CH. Chronic vagus nerve stimulation decreased weight gain, food consumption and sweet craving in adult obese minipigs. Appetite. (2010) 55:245–52. doi: 10.1016/j.appet.2010.06.008
102. Li MCH, Cook MJ. Deep brain stimulation for drug-resistant epilepsy. Epilepsia. (2018) 59:273–90. doi: 10.1111/epi.13964
103. Nair DR, Laxer KD, Weber PB, Murro AM, Park YD, Barkley GL, et al. Nine-year prospective efficacy and safety of brain-responsive neurostimulation for focal epilepsy. Neurology. (2020) 95:e1244–56. doi: 10.1212/WNL.0000000000010154
104. Schulze-Bonhage A. Deep brain stimulation: a new approach to the treatment of epilepsy. Deutsches Arzteblatt Int. (2009) 106:407–12. doi: 10.3238/arztebl.2009.0407
105. Salanova V, Witt T, Worth R, Henry TR, Gross RE, Nazzaro JM, et al. Long-term efficacy and safety of thalamic stimulation for drug-resistant partial epilepsy. Neurology. (2015) 84:1017–25. doi: 10.1212/WNL.0000000000001334
106. Kaufmann E, Bartolomei F, Boon P, Chabardes S, Colon AJ, Eross L, et al. European Expert Opinion on ANT-DBS therapy for patients with drug-resistant epilepsy (a Delphi consensus). Seizure Eur J Epilepsy. (2020) 81:201–9. doi: 10.1016/j.seizure.2020.08.015
107. Ryvlin P, Jehi LE. Neuromodulation for refractory epilepsy. Epilepsy Curr. (2021) 22:11–17. doi: 10.1177/15357597211065587
108. Schulze-Bonhage A. Brain stimulation as a neuromodulatory epilepsy therapy. Seizure Eur J Epilepsy. (2017) 44:169–75. doi: 10.1016/j.seizure.2016.10.026
109. Lundstrom B, Gompel J, Khadjevand F, Worrell G, Stead M. Chronic subthreshold cortical stimulation and stimulation-related EEG biomarkers for focal epilepsy. Brain Commun. (2019) 1:1–8. doi: 10.1093/braincomms/fcz010
110. Lundstrom BN, Van Gompel J, Britton J, Nickels K, Wetjen N, Worrell G, et al. Chronic subthreshold cortical stimulation to treat focal epilepsy. JAMA Neurol. (2016) 73:1370–2. doi: 10.1001/jamaneurol.2016.2857
111. Cukiert A, Cukiert CM, Burattini JA, Mariani PP, Bezerra DF. Seizure outcome after hippocampal deep brain stimulation in patients with refractory temporal lobe epilepsy: a prospective, controlled, randomized, double-blind study. Epilepsia. (2017) 58:1728–33. doi: 10.1111/epi.13860
112. Alcala-Zermeno JL, Gregg NM, Wirrell EC, Stead M, Worrell GA, Van Gompel JJ, et al. Centromedian thalamic nucleus with or without anterior thalamic nucleus deep brain stimulation for epilepsy in children and adults: a retrospective case series. Seizure. (2021) 84:101–7. doi: 10.1016/j.seizure.2020.11.012
113. Martin-Lopez D, Jimenez-Jimenez D, Cabanes-Martinez L, Selway RP, Valentin A, Alarcon G. The role of thalamus versus cortex in epilepsy: evidence from human Ictal Centromedian recordings in patients assessed for deep brain stimulation. Int J Neural Syst. (2017) 27:18. doi: 10.1142/S0129065717500101
114. Stavropoulos I, Pak HL, Valentin A. Neuromodulation in super-refractory status epilepticus. J Clin Neurophysiol. (2021) 38:494–502. doi: 10.1097/WNP.0000000000000710
115. Lee CY, Lim SN, Wu TN, Lee ST. Successful treatment of refractory status epilepticus using anterior thalamic nuclei deep brain stimulation. World Neurosurg. (2017) 99:14–8. doi: 10.1016/j.wneu.2016.11.097
116. Yuan L, Zhang SH, Liang SS, Liu N, Yu XM, Liang SL. Deep brain stimulation of the anterior nucleus of the thalamus in a patient with super-refractory convulsive status epilepticus. Epileptic Disord. (2019) 21:379–84. doi: 10.1684/epd.2019.1086
117. Imbach LL, Baumann C, Poryazova R, Geissler O, Brugger P, Mothersill I, et al. Anticonvulsive effect of anterior thalamic deep brain stimulation in superrefractory status epilepticus crucially depends on active stimulation zone - a single case observation. Epilepsia. (2019) 60:100–1. doi: 10.1016/j.seizure.2019.08.015
118. Lehtimaki K, Mottonen T, Jarventausta K, Katisko J, Tahtinen T, Haapasalo J, et al. Outcome based definition of the anterior thalamic deep brain stimulation target in refractory epilepsy. Brain Stimul. (2016) 9:268–75. doi: 10.1016/j.brs.2015.09.014
119. Valentin A, Nguyen HQ, Skupenova AM, Agirre-Arrizubieta Z, Jewell S, Mullatti N, et al. Centromedian thalamic nuclei deep brain stimulation in refractory status epilepticus. Brain Stimul. (2012) 5:594–8. doi: 10.1016/j.brs.2011.10.002
120. Gregg NM, Sladky V, Nejedly P, Mivalt F, Kim I, Balzekas I, et al. Thalamic deep brain stimulation modulates cycles of seizure risk in epilepsy. Sci Rep. (2021) 11:12. doi: 10.1038/s41598-021-03555-7
121. Vedam-Mai V, Deisseroth K, Giordano J, Lazaro-Munoz G, Chiong W, Suthana N, et al. Proceedings of the eighth annual deep brain stimulation think tank: advances in optogenetics, ethical issues affecting DBS research, neuromodulatory approaches for depression, adaptive neurostimulation, and emerging DBS technologies. Front Hum Neurosci. (2021) 15:765150. doi: 10.3389/fnhum.2021.765150
122. Maeda F, Kleiner-Fisman G, Pascual-Leone A. Motor facilitation while observing hand actions: specificity of the effect and role of observer's orientation. J Neurophysiol. (2002) 87:1329–35. doi: 10.1152/jn.00773.2000
123. Peng Z, Zhou C, Xue S, Bai J, Yu S, Li X, et al. Mechanism of repetitive transcranial magnetic stimulation for depression. Shanghai Arch Psychiatry. (2018) 30:84–92. doi: 10.11919/j.issn.1002-0829.217047
124. Badawy RA, Freestone DR, Lai A, Cook MJ. Epilepsy: ever-changing states of cortical excitability. Neuroscience. (2012) 222:89–99. doi: 10.1016/j.neuroscience.2012.07.015
125. Kramer MA, Cash SS. Epilepsy as a disorder of cortical network organization. Neuroscientist. (2012) 18:360–72. doi: 10.1177/1073858411422754
126. Wagner TA, Zahn M, Grodzinsky AJ, Pascual-Leone A. Three-dimensional head model simulation of transcranial magnetic stimulation. IEEE Transac Biomed Eng. (2004) 51:1586–98. doi: 10.1109/TBME.2004.827925
127. Bestmann S, Baudewig J, Siebner HR, Rothwell JC, Frahm J. Functional MRI of the immediate impact of transcranial magnetic stimulation on cortical and subcortical motor circuits. Eur J Neurosci. (2004) 19:1950–62. doi: 10.1111/j.1460-9568.2004.03277.x
128. Chouinard PA, Van Der Werf YD, Leonard G, Paus T. Modulating neural networks with transcranial magnetic stimulation applied over the dorsal premotor and primary motor cortices. J Neurophysiol. (2003) 90:1071–83. doi: 10.1152/jn.01105.2002
129. Valero-Cabré A, Payne BR, Rushmore J, Lomber SG, Pascual-Leone A. Impact of repetitive transcranial magnetic stimulation of the parietal cortex on metabolic brain activity: a 14C-2DG tracing study in the cat. Exp Brain Res. (2005) 163:1–12. doi: 10.1007/s00221-004-2140-6
130. Theodore WH. Transcranial magnetic stimulation in epilepsy. Epilepsy Curr. (2003) 3:191–7. doi: 10.1046/j.1535-7597.2003.03607.x
131. Thordstein M, Constantinescu R. Possibly lifesaving, noninvasive, EEG-guided neuromodulation in anesthesia-refractory partial status epilepticus. Epilepsy Behav. (2012) 25:468–72. doi: 10.1016/j.yebeh.2012.07.026
132. Liu A, Pang T, Herman S, Pascual-Leone A, Rotenberg A. Transcranial magnetic stimulation for refractory focal status epilepticus in the intensive care unit. Seizure. (2013) 22:893–6. doi: 10.1016/j.seizure.2013.06.014
133. VanHaerents S, Herman ST, Pang T, Pascual-Leone A, Shafi MM. Repetitive transcranial magnetic stimulation; A cost-effective and beneficial treatment option for refractory focal seizures. Clin Neurophysiol. (2015) 126:1840–2. doi: 10.1016/j.clinph.2014.12.004
134. Tergau F, Naumann U, Paulus W, Steinhoff BJ. Low-frequency repetitive transcranial magnetic stimulation improves intractable epilepsy. Lancet. (1999) 353:2209. doi: 10.1016/S0140-6736(99)01301-X
135. Daniele O, Brighina F, Piazza A, Giglia G, Scalia S, Fierro B. Low-frequency transcranial magnetic stimulation in patients with cortical dysplasia - a preliminary study. J Neurol. (2003) 250:761–2. doi: 10.1007/s00415-003-1080-6
136. Brasil-Neto JP, de Araújo DP, Teixeira WA, Araújo VP, Boechat-Barros R. Experimental therapy of epilepsy with transcranial magnetic stimulation: lack of additional benefit with prolonged treatment. Arquivos Neuro Psiquiatr. (2004) 62:21–5. doi: 10.1590/S0004-282X2004000100004
137. Tsuboyama M, Kaye HL, Rotenberg A. Review of transcranial magnetic stimulation in epilepsy. Clin Ther. (2020) 42:1155–68. doi: 10.1016/j.clinthera.2020.05.016
138. Lefaucheur JP, André-Obadia N, Antal A, Ayache SS, Baeken C, Benninger DH, et al. Evidence-based guidelines on the therapeutic use of repetitive transcranial magnetic stimulation (rTMS). Clin Neurophysiol. (2014) 125:2150–206. doi: 10.1016/j.clinph.2014.05.021
139. Seynaeve L, Devroye A, Dupont P, Van Paesschen W. Randomized crossover sham-controlled clinical trial of targeted low-frequency transcranial magnetic stimulation comparing a figure-8 and a round coil to treat refractory neocortical epilepsy. Epilepsia. (2016) 57:141–50. doi: 10.1111/epi.13247
140. Poma R, Ives J, Rotenberg A, Pascual-Leone A. Repetitive transcranial magnetic stimulation in 3 epileptic dogs: techniques of stimulation and results. Epilepsia. (2006) 47:337. doi: 10.1016/j.yebeh.2008.09.007
141. Ferrarelli F, Massimini M, Sarasso S, Casali A, Riedner BA, Angelini G, et al. Breakdown in cortical effective connectivity during midazolam-induced loss of consciousness. Proc Natl Acad Sci USA. (2010) 107:2681–6. doi: 10.1073/pnas.0913008107
142. Waelbers T, Peremans K, Vermeire S, Duchateau L, Dobbeleir A, Audenaert K, et al. The effect of medetomidine on the regional cerebral blood flow in dogs measured using Technetium-99m-Ethyl Cysteinate Dimer SPECT. Res Vet Sci. (2011) 91:138–43. doi: 10.1016/j.rvsc.2010.08.003
143. Newberg LA, Milde JH, Michenfelder JD. The cerebral metabolic effects of isoflurane at and above concentrations that suppress cortical electrical activity. Anesthesiology. (1983) 59:23–8. doi: 10.1097/00000542-198307000-00005
144. Waelbers T, Polis I, Vermeire S, Dobbeleir A, Eersels J, De Spiegeleer B, et al. Effect of ketamine on the regional cerebral blood flow and binding index of the 5-HT2A receptor radioligand 123I-R91150 in the canine brain. J Vet Behav. (2015) 10:332–7. doi: 10.1016/j.jveb.2015.03.009
145. Muller PA, Dhamne SC, Vahabzadeh-Hagh AM, Pascual-Leone A, Jensen FE, Rotenberg A. Suppression of motor cortical excitability in anesthetized rats by low frequency repetitive transcranial magnetic stimulation. PLoS ONE. (2014) 9:e91065. doi: 10.1371/journal.pone.0091065
146. Dockx R, Peremans K, Vlerick L, Van Laeken N, Saunders JH, Polis I, et al. Anaesthesia, not number of sessions, influences the magnitude and duration of an aHF-rTMS in dogs. PLoS ONE. (2017) 12:e0185362. doi: 10.1371/journal.pone.0185362
147. Elger CE, Mormann F. Seizure prediction and documentation–two important problems. Lancet Neurol. (2013) 12:531–2. doi: 10.1016/S1474-4422(13)70092-9
148. Cook MJ, O'Brien TJ, Berkovic SF, Murphy M, Morokoff A, Fabinyi G, et al. Prediction of seizure likelihood with a long-term, implanted seizure advisory system in patients with drug-resistant epilepsy: a first-in-man study. Lancet Neurol. (2013) 12:563–71. doi: 10.1016/S1474-4422(13)70075-9
149. Morrell MJ. In response: the RNS System multicenter randomized double-blinded controlled trial of responsive cortical stimulation for adjunctive treatment of intractable partial epilepsy: knowledge and insights gained. Epilepsia. (2014) 55:1470–1. doi: 10.1111/epi.12736
150. Gregg NM, Marks VS, Sladky V, Lundstrom BN, Klassen B, Messina SA, et al. Anterior nucleus of the thalamus seizure detection in ambulatory humans. Epilepsia. (2021) 62:e158–64. doi: 10.1111/epi.17047
151. Baldassano S, Zhao X, Brinkmann B, Kremen V, Bernabei J, Cook M, et al. Cloud computing for seizure detection in implanted neural devices. J Neural Eng. (2019) 16:026016. doi: 10.1088/1741-2552/aaf92e
152. Kremen V, Brinkmann BH, Kim I, Guragain H, Nasseri M, Magee AL, et al. Integrating brain implants with local and distributed computing devices: a next generation epilepsy management system. IEEE J Transl Eng Health Med. (2018) 6:2500112. doi: 10.1109/JTEHM.2018.2869398
153. Sladky V, Nejedly P, Mivalt F, Brinkmann BH, Kim I St., Louis EK, et al. Distributed brain co-processor for neurophysiologic tracking and adaptive stimulation: application to drug resistant epilepsy. bioRxiv. (2021).
154. Dumanis SB, French JA, Bernard C, Worrell GA, Fureman BE. Seizure forecasting from idea to reality. Outcomes of the my seizure gauge epilepsy innovation institute workshop. eNeuro. (2017) 4:ENEURO.0349-17.2017. Available online at: https://www.eneuro.org/content/eneuro/4/6/ENEURO.0349-17.2017.full.pdf
155. Brinkmann BH, Wagenaar J, Abbot D, Adkins P, Bosshard SC, Chen M, et al. Crowdsourcing reproducible seizure forecasting in human and canine epilepsy. Brain. (2016) 139:1713–22. doi: 10.1093/brain/aww045
156. Kuhlmann L, Karoly P, Freestone DR, Brinkmann BH, Temko A, Barachant A, et al. Epilepsyecosystem.org: crowd-sourcing reproducible seizure prediction with long-term human intracranial EEG. Brain. (2018) 141:2619–30. doi: 10.1093/brain/awy210
157. Baud MO, Rao VR. Gauging seizure risk. Neurology. (2018) 91:967–73. doi: 10.1212/WNL.0000000000006548
158. Karoly PJ, Rao VR, Gregg NM, Worrell GA, Bernard C, Cook MJ, et al. Cycles in epilepsy. Nat Rev Neurol. (2021) 17:267–84. doi: 10.1038/s41582-021-00464-1
159. Baud MO, Kleen JK, Mirro EA, Andrechak JC, King-Stephens D, Chang EF, et al. Multi-day rhythms modulate seizure risk in epilepsy. Nat Commun. (2018) 9:88. doi: 10.1038/s41467-017-02577-y
160. Stirling RE, Cook MJ, Grayden DB, Karoly PJ. Seizure forecasting and cyclic control of seizures. Epilepsia. (2021) 62:S2–14. doi: 10.1111/epi.16541
161. Gregg NM, Nasseri M, Kremen V, Patterson EE, Sturges BK, Denison TJ, et al. Circadian and multiday seizure periodicities, and seizure clusters in canine epilepsy. Brain Commun. (2020) 2:fcaa008. doi: 10.1093/braincomms/fcaa008
162. Langdon-Down M, RBW. Time of day in relation to convulsions in epilepsy. Lancet. (1929) 213. doi: 10.1016/S0140-6736(00)79288-9
163. Boon P, De Cock E, Mertens A, Trinka E. Neurostimulation for drug-resistant epilepsy: a systematic review of clinical evidence for efficacy, safety, contraindications and predictors for response. Curr Opin Neurol. (2018) 31:198–210. doi: 10.1097/WCO.0000000000000534
164. Santos-Valencia F, Almazan-Alvarado S, Rubio-Luviano A, Valdes-Cruz A, Magdaleno-Madrigal VM, Martinez-Vargas D. Temporally irregular electrical stimulation to the epileptogenic focus delays epileptogenesis in rats. Brain Stimul. (2019) 12:1429–38. doi: 10.1016/j.brs.2019.07.016
165. Chen N, Zhang JG, Han CL, Meng FG. Hippocampus chronic deep brain stimulation induces reversible transcript changes in a macaque model of mesial temporal lobe epilepsy. Chin Med J. (2021) 134:1845–54. doi: 10.1097/CM9.0000000000001644
166. Keck M, van Dijk RM, Deeg CA, Kistler K, Walker A, von Rüden EL, et al. Proteomic profiling of epileptogenesis in a rat model: focus on cell stress, extracellular matrix and angiogenesis. Neurobiol Dis. (2018) 112:119–35. doi: 10.1016/j.nbd.2018.01.013
167. Ghotbeddin Z, Moazedi AA, Yadollahpour A, Rendi F, Jalilifar M. Improving cognitive task in kindled rats by using low frequency stimulation during epileptogenesis. Metabolic Brain Dis. (2018) 33:1525–31. doi: 10.1007/s11011-018-0260-0
168. Simonin C, Tir M, Devos D, Kreisler A, Dujardin K, Salleron J, et al. Reduced levodopa-induced complications after 5 years of subthalamic stimulation in Parkinson's disease: a second honeymoon. J Neurol. (2009) 256:1736–41. doi: 10.1007/s00415-009-5195-2
169. Nakajima A, Oyama G, Jo T, Shimo Y, Umemura A, Nakajima M, et al. Rescue pallidal stimulation for diphasic and stimulation-induced dyskinesia after successful subthalamic stimulation for Parkinson's disease. Neurol Clin Neurosci. (2017) 5:127–8. doi: 10.1111/ncn3.12127
170. Biggio F, Gorini G, Utzeri C, Olla P, Marrosu F, Mocchetti I, et al. Chronic vagus nerve stimulation induces neuronal plasticity in the rat hippocampus. Int J Neuropsychopharmacol. (2009) 12:1209–21. doi: 10.1017/S1461145709000200
171. Vezzani A, French J, Bartfai T, Baram TZ. The role of inflammation in epilepsy. Nat Rev Neurol. (2011) 7:31–40. doi: 10.1038/nrneurol.2010.178
172. Vezzani A, Friedman A, Dingledine RJ. The role of inflammation in epileptogenesis. Neuropharmacology. (2013) 69:16–24. doi: 10.1016/j.neuropharm.2012.04.004
173. Bonaz B, Picq C, Sinniger V, Mayol JF, Clarencon D. Vagus nerve stimulation: from epilepsy to the cholinergic anti-inflammatory pathway. Neurogastroenterol Motil. (2013) 25:208–21. doi: 10.1111/nmo.12076
174. Bonaz B, Sinniger V, Pellissier S. The vagus nerve in the neuro-immune axis: implications in the pathology of the gastrointestinal tract. Front Immunol. (2017) 8:1452. doi: 10.3389/fimmu.2017.01452
175. Bie B, Wang Z, Chen Y, Sheng L, Li H, You H, et al. Vagus nerve stimulation affects inflammatory response and anti-apoptosis reactions via regulating miR-210 in epilepsy rat model. Neuroreport. (2021) 32:783–91. doi: 10.1097/WNR.0000000000001655
176. Tang Y, Dong X, Chen G, Ye W, Kang J, Tang Y, et al. Vagus nerve stimulation attenuates early traumatic brain injury by regulating the NF-kappaB/NLRP3 signaling pathway. Neurorehabil Neural Repair. (2020) 34:831–43. doi: 10.1177/1545968320948065
177. Meneses G, Bautista M, Florentino A, Diaz G, Acero G, Besedovsky H, et al. Electric stimulation of the vagus nerve reduced mouse neuroinflammation induced by lipopolysaccharide. J Inflamm. (2016) 13:33. doi: 10.1186/s12950-016-0140-5
178. Qi R, Wang M, Zhong Q, Wang L, Yang X, Huang B, et al. Chronic vagus nerve stimulation (VNS) altered IL-6, IL-1β, CXCL-1 and IL-13 levels in the hippocampus of rats with LiCl-pilocarpine-induced epilepsy. Brain Res. (2022) 1780:147800. doi: 10.1016/j.brainres.2022.147800
179. Chen YC, Zhu GY, Wang X, Shi L, Du TT, Liu DF, et al. Anterior thalamic nuclei deep brain stimulation reduces disruption of the blood-brain barrier, albumin extravasation, inflammation and apoptosis in kainic acid-induced epileptic rats. Neurol Res. (2017) 39:1103–13. doi: 10.1080/01616412.2017.1379241
180. Amorim BO, Covolan L, Ferreira E, Brito JG, Nunes DP, de Morais DG, et al. Deep brain stimulation induces antiapoptotic and anti-inflammatory effects in epileptic rats. J Neuroinflammation. (2015) 12:162. doi: 10.1186/s12974-015-0384-7
181. Ding M, Lang Y, Shu H, Shao J, Cui L. Microbiota–gut–brain axis and epilepsy: a review on mechanisms and potential therapeutics. Front Immunol. (2021) 12:742449. doi: 10.3389/fimmu.2021.742449
182. Haney MM, Ericsson AC, Lever TE. Effects of intraoperative vagal nerve stimulation on the gastrointestinal microbiome in a mouse model of amyotrophic lateral sclerosis. Comp Med. (2018) 68:452–60. doi: 10.30802/AALAS-CM-18-000039
183. Aizawa Y, Morishita J, Kano M, Kanazawa M, Fukudo S. Modification of rectal function and emotion by repetitive transcranial magnetic stimulation in humans. Neurosci Res. (2021) 168:54–63. doi: 10.1016/j.neures.2021.05.013
184. Roganovic M, Pantovic S, Dizdarevic S. Role of the oxidative stress in the pathogenesis of epilepsy. Neurol. Sci. Neurophysiology. (2019) 36:1–8. doi: 10.5152/NSN.2019.11632
185. Medina-Fernandez FJ, Escribano BM, Padilla-Del-Campo C, Drucker-Colin R, Pascual-Leone A, Tunez I. Transcranial magnetic stimulation as an antioxidant. Free Radic Res. (2018) 52:381–9. doi: 10.1080/10715762.2018.1434313
186. Chen M, Zhou X, Yu L, Liu Q, Sheng X, Wang Z, et al. Low-level vagus nerve stimulation attenuates myocardial ischemic reperfusion injury by antioxidative stress and antiapoptosis reactions in canines. J Cardiovasc Electrophysiol. (2016) 27:224–31. doi: 10.1111/jce.12850
Keywords: drug-resistant epilepsy, dogs, vagus nerve stimulation, deep brain stimulation, transcranial magnetic stimulation, seizure, epileptogenesis
Citation: Nowakowska M, Üçal M, Charalambous M, Bhatti SFM, Denison T, Meller S, Worrell GA, Potschka H and Volk HA (2022) Neurostimulation as a Method of Treatment and a Preventive Measure in Canine Drug-Resistant Epilepsy: Current State and Future Prospects. Front. Vet. Sci. 9:889561. doi: 10.3389/fvets.2022.889561
Received: 04 March 2022; Accepted: 23 May 2022;
Published: 16 June 2022.
Edited by:
Monica Aleman, University of California, Davis, United StatesReviewed by:
Alejandra Mondino, North Carolina State University, United StatesCopyright © 2022 Nowakowska, Üçal, Charalambous, Bhatti, Denison, Meller, Worrell, Potschka and Volk. This is an open-access article distributed under the terms of the Creative Commons Attribution License (CC BY). The use, distribution or reproduction in other forums is permitted, provided the original author(s) and the copyright owner(s) are credited and that the original publication in this journal is cited, in accordance with accepted academic practice. No use, distribution or reproduction is permitted which does not comply with these terms.
*Correspondence: Marta Nowakowska, bWFydGEubm93YWtvd3NrYUBtZWR1bmlncmF6LmF0; Holger A. Volk, aG9sZ2VyLnZvbGtAdGloby1oYW5ub3Zlci5kZQ==
Disclaimer: All claims expressed in this article are solely those of the authors and do not necessarily represent those of their affiliated organizations, or those of the publisher, the editors and the reviewers. Any product that may be evaluated in this article or claim that may be made by its manufacturer is not guaranteed or endorsed by the publisher.
Research integrity at Frontiers
Learn more about the work of our research integrity team to safeguard the quality of each article we publish.