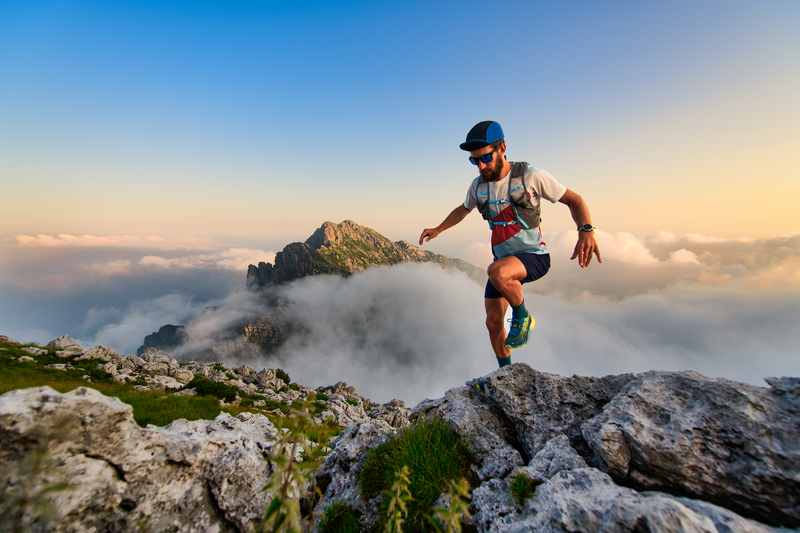
95% of researchers rate our articles as excellent or good
Learn more about the work of our research integrity team to safeguard the quality of each article we publish.
Find out more
ORIGINAL RESEARCH article
Front. Vet. Sci. , 04 May 2022
Sec. Veterinary Emergency and Critical Care Medicine
Volume 9 - 2022 | https://doi.org/10.3389/fvets.2022.865673
This article is part of the Research Topic Mechanical Ventilation in Anesthesia and Critical Care Small Animal Patients View all 7 articles
Objectives: To compare the effects of four levels of end-expiratory pressure [zero (ZEEP) and three levels of positive end-expiratory pressure (PEEP)] on the cardiovascular system and gas exchange of cats anesthetized with isoflurane and mechanically ventilated for 3 h with a tidal volume of 10 ml/kg.
Study Design: Prospective, randomized, controlled trial.
Animals: Six healthy male neutered purpose-bred cats.
Methods: Anesthesia was induced with isoflurane and maintained at 1.3 minimum alveolar concentration. PEEP of maximal respiratory compliance (PEEPmaxCrs) was identified in a decremental PEEP titration, and cats were randomly ventilated for 3 h with one of the following end-expiratory pressures: ZEEP, PEEPmaxCrs minus 2 cmH2O (PEEPmaxCrs−2), PEEPmaxCrs, and PEEPmaxCrs plus 2 cmH2O (PEEPmaxCrs+2). Cardiovascular and gas exchange variables were recorded at 5, 30, 60, 120, and 180 min (T5 to T180, respectively) of ventilation and compared between and within ventilation treatments with mixed-model ANOVA followed by Dunnet's and Tukey's tests (normal distribution) or Friedman test followed by the Dunn's test (non-normal distribution). Significance to reject the null hypothesis was considered p < 0.05.
Results: Mean arterial pressure (MAP—mmHg) was lower in PEEPmaxCrs+2 [63 (49–69); median (range)] when compared to ZEEP [71 (67–113)] at T5 and stroke index (ml/beat/kg) was lower in PEEPmaxCrs+2 (0.70 ± 0.20; mean ± SD) than in ZEEP (0.90 ± 0.20) at T60. Cardiac index, oxygen delivery index (DO2I), systemic vascular resistance index, and shunt fraction were not significantly different between treatments. The ratio between arterial partial pressure and inspired concentration of oxygen (PaO2/FIO2) was lower in ZEEP than in the PEEP treatments at various time points. At T180, DO2I was higher when compared to T5 in PEEPmaxCrs. Dopamine was required to maintain MAP higher than 60 mmHg in one cat during PEEPmaxCrs and in three cats during PEEPmaxCrs+2.
Conclusion: In cats anesthetized with isoflurane and mechanically ventilated for 3 h, all levels of PEEP mildly improved gas exchange with no significant difference in DO2I when compared to ZEEP. The PEEP levels higher than PEEPmaxCrs−2 caused more cardiovascular depression, and dopamine was an effective treatment. A temporal increase in DO2I was observed in the cats ventilated with PEEPmaxCrs. The effects of these levels of PEEP on respiratory mechanics, ventilation-induced lung injury, as well as in obese and critically ill cats deserve future investigation for a better understanding of the clinical use of PEEP in this species.
The application of positive end-expiratory pressure (PEEP) and alveolar recruitment maneuvers (ARM) during mechanical ventilation can increase functional residual capacity (FRC) and reduce or treat small airway closure and atelectasis in humans (1, 2) and dogs (3). Despite an improvement in arterial oxygenation related to PEEP in dogs (3), the same effect was not observed in other studies using the same species (4, 5). To the authors' best knowledge, no study on the effects of PEEP on gas exchange, cardiac output, and oxygen delivery in cats has been published. However, PEEP may have a beneficial effect on gas exchange in this species because atelectasis has been observed when anesthetized cats were ventilated with zero PEEP (ZEEP) (6, 7). Despite its potential benefits of improving FRC and gas exchange, PEEP can result in decreased mean arterial pressure (MAP) in cats (7). The cardiovascular depression caused by PEEP is mainly related to a decrease in cardiac index (CI) (8) as reported in dogs (5).
The PEEP of maximal respiratory system compliance (PEEPmaxCrs) achieved during a decremental PEEP titration has been recently used as a method to individualize PEEP in protocols of protective ventilation (9, 10). In healthy rats, PEEPmaxCrs promoted a better balance between alveolar overdistention and tidal recruitment/derecruitment when compared to higher PEEP or the absence of it (11). In addition, PEEP levels higher than PEEPmaxCrs seems to provide better prevention of atelectasis but at the expense of causing alveolar overdistension (11, 12) impairing cardiovascular function (5), and possibly causing redistribution of pulmonary blood with an increase in ventilation/perfusion (/) mismatch (13).
This study aimed to compare the effects of four levels on end-expiratory pressure (EEP): ZEEP, PEEPmaxCrs minus 2 cmH2O (PEEPmaxCrs−2), PEEPmaxCrs, and PEEPmaxCrs plus 2 cmH2O (PEEPmaxCrs+2) on the cardiovascular system, pulmonary gas exchange, and arterial oxygenation in isoflurane-anesthetized cats. We hypothesized that in isoflurane-anesthetized cats with healthy lungs mechanically ventilated for 3 h: (1) PEEPmaxCrs and PEEPmaxCrs+2 will provide higher arterial oxygenation than ZEEP and PEEPmaxCrs−2; and (2) PEEPmaxCrs+2 will decrease CI, MAP, and oxygen delivery index (DO2I) when compared to ZEEP.
Six healthy male neutered cats, 1–2 years old, weighing 5.1 ± 0.9 kg (mean ± standard deviation) were enrolled in this prospective, randomized, controlled crossover study. All 6 cats received all EEP treatments in separate days with a minimum of 7 days between experiments. Physical examination and routine basic blood work (packed cell volume and serum biochemistry) were performed to evaluate the cats' health status. All cats were housed in a room at the Teaching and Research Animal Care Services facility, University of California, Davis, United States. All cats were acclimatized to the laboratory conditions and handled 14 days before commencing the study. Cats were fed a commercial diet once a day and had access to water ad libitum. This study was approved by the Institutional Animal Care and Use Committee of the University of California Davis (n. 21985). Food, but not water, was withheld for 12 h before the experiments.
Each cat was anesthetized with 5% isoflurane (Isoflurane; Piramal Critical Care Inc., PA, USA) in oxygen (5 L/min) delivered by a Bain circuit into an acrylic chamber. Once the righting reflex was lost, the trachea was intubated with a 4.5-mm internal diameter cuffed tube (Sheridan/CF, Teleflex, NC, USA), and anesthesia was maintained during the whole experiment with 1.3 MAC of isoflurane (2.12 ETISO%) (14) in oxygen delivered from a circle breathing system. A 22-gauge catheter (BD Insyte, 2.5 cm, USA) was aseptically inserted in a cephalic vein for infusion of lactated Ringer's solution (Baxter Healthcare Corp., IL, USA) at 3 ml/kg/h. A pulse oximetry probe was positioned on the tongue and a lead II electrocardiogram was connected to evaluate heart rhythm. The cats were placed in dorsal recumbency during the whole experiment and rocuronium bromide (XGen Pharmaceuticals DJB, IL, USA) at a dose of 0.6 mg/kg was administered intravenously followed by a constant rate infusion of 0.6 mg/kg/h. If any sign of spontaneous ventilation was observed on the monitoring of airway pressure (Paw) or flow (), an additional dose of 0.1 mg/kg of rocuronium was administered intravenously. Stimulating electrodes were placed over the peroneal nerve and an accelerometer was attached to the paw to monitor the train-of-four ratio (TOF-Watch SX, Organon Ltd., Ireland). The train-of-four ratio was maintained below 0.3 during the whole experiment. Mechanical ventilation was performed during instrumentation in volume-control mode with a tidal volume (VT) of 10 mL/kg, inspiratory-to-expiratory time ratio (I:E ratio) of 1:2, FIO2 between 0.95 and 0.98, and the respiratory rate (fR) adjusted to maintain the end-tidal CO2 partial pressure (PETCO2) between 30 and 40 mmHg (baseline ventilatory settings) (Flow-I, C20, Getinge AB, USA). Inspired fraction of oxygen (FIO2) and end-tidal isoflurane concentration (ETISO) were measured with a gas analyzer calibrated before and during the experiments (AS/3, Datex-Ohmeda, Helsinki, Finland). A mainstream neonatal capnography sensor (NM3, Philips Healthcare, MA, USA) was placed between the pneumotachometer and the breathing system to measure PETCO2. Calibration curves for isoflurane, oxygen, and CO2 concentrations were obtained by linear regression using 3 different concentrations of primary gas standards (1.33, 2.00, and 3.50% for isoflurane; 60, 80, and 100% for O2; 5.0, 8.1, and 10.0% for CO2). A 4-Fr 5.5 cm sheath introducer (RCFN-4.0-18-5.5-RA1.5; Cook Medical, IN, USA) was aseptically inserted into the jugular vein using a modified Seldinger technique and sutured to the skin. A 4-Fr, 75 cm thermodilution catheter (AI-07044; Teleflex, NC, USA) was placed through the introducer until its tip was positioned in the pulmonary artery. Positioning was confirmed by fluoroscopy (Figure 1) with further adjustments in position made based on visualization of the pulmonary artery and central venous pressure (CVP) waveforms and the ability of the catheter to occlude the pulmonary artery during inflation of its balloon. The femoral or carotid artery was aseptically catheterized with a 22-gauge, 8 cm catheter (Arteriofix V, B Braun Meslsungen AG, Germany). Pressure transducers (Meritrans DTX plus, MeritMedical, Singapore) connected to non-compliant tubing filled with heparinized saline (2 U/ml) were positioned and zeroed at the level of the scapulohumeral joint, and attached to the arterial catheter, and the proximal and distal ports of the thermodilution catheter, for the recordings of MAP, CVP, and mean pulmonary artery (MPAP) pressure, respectively. Pressure transducers were calibrated against a mercury or water column before each experiment and connected to a data acquisition system as previously described (5). The dampening coefficient and natural frequency response of the blood pressure transducers-catheter were assessed at the beginning of the experiments by the fast flush test response, consisting of a quick opening of the flush valve of the transducer to the pressurized bag (~300 mmHg) with heparinized saline. The typical dampening coefficient of the system and natural frequency response were 0.3 (0.25 to 0.4) and 15 Hz (12 to 25 Hz), respectively. The thermodilution catheter thermistor was connected to a cardiac output monitor (AS/3, Datex-Ohmeda, Helsinki, Finland). Body temperature was measured by the thermistor of the thermodilution catheter and was maintained between 37.5 and 38.5°C by heating blankets. Thermodilution cardiac output was measured by the fast injection (1–2 s) of 1.5 ml of cold saline (0–2°C) through the proximal lumen of the pulmonary artery thermodilution catheter and the average of three measurements with <10% difference was reported.
Figure 1. Fluoroscopic image of the thermodilution catheter positioned at the pulmonary artery of one of the studied cats.
A Lilly heated pneumotachometer (8300 series; Hans Rudolph Ltd., KS, USA) coupled to a differential pressure transducer (DPL2.5—Hugo Sacks Elektronik—Harvard Apparatus GmbH, Germany) was connected between the breathing system and the endotracheal tube for the measurements of airflow (). Airway pressure (Paw) was measured by a differential pressure transducer (MPX 399/2, Hugo Sacks Elektronik—Harvard Apparatus GmbH, Germany) from a port between the endotracheal tube and the pneumotachometer. Volume (V) was calculated by the numerical integration of , zeroed at the beginning of each cycle. An esophageal balloon catheter was placed in the esophagus for the measurements of esophageal pressure (Peso) (P75, Hugo Sacks Elektronik—Harvard Apparatus GmbH, Germany) as a surrogate of pleural pressure. The position of the esophageal balloon catheter was verified by the occlusion method (15). The digital signals of Paw, , and Peso were continuously acquired at 400 Hz and displayed by a custom-made software (16) written in LabView (LabView 2019, NI, TX, USA), and saved on a personal computer. Values of Paw and Peso were calibrated by linear regression using 6 pressure points (−10, 0, 10, 20, 30, and 40 cmH2O) provided by a water column (reference method). The calibration of was performed by a modified flow-integration method (5, 17) using a 100-ml volumetric calibration syringe (5510 series—Hans Rudolph Ltd., KS, USA) containing oxygen and isoflurane concentrations similar to those used during the experiments (O2 94–99%; isoflurane 2.0–2.2%).
After instrumentation, an intravenous bolus of 10 ml/kg of lactated Ringer's solution was administered over 5 min. Then, an ARM was performed in pressure-control ventilation with 10 cmH2O difference between peak airway pressure and EEP in 4 ascending steps of EEP (0, 5, 10, 15, and 20 cmH2O), with each step maintained for 30 s. Following this ARM, a descendent PEEP titration from 10 to 0 cmH2O in steps of 2 cmH2O, maintained for 2 min each was performed in volume-control mode with VT of 10 ml/kg and the same fR used in the baseline ventilatory settings.
Immediately after the PEEP titration, respiratory system compliance (Crs) at each PEEP step was estimated offline using custom-made software (18) written in MATLab (MathWorks Inc., MA, USA). For this purpose, the multiple linear regression method was applied to the single compartment equation of motion of the respiratory system presented below:
where Rrs and Ers are respiratory system resistance and elastance, respectively, P0 is the Paw when V and are zero, and t is time. Respiratory system compliance (Crs) was calculated as 1/Ers.
The PEEP step associated with the highest Crs was assigned as PEEPmaxCrs, as previously described (5). After the PEEP titration, a second ARM identical to the first one was performed and the cats were mechanically ventilated for 3 h with one of the randomized (www.randomizer.org) EEP treatments (ZEEP, PEEPmaxCrs−2, PEEPmaxCrs, and PEEPmaxCrs+2). An intravenous constant rate infusion of dopamine, starting at 5 mcg/kg/min and increased as needed, was used to maintain MAP higher than 60 mmHg. Mechanical ventilation was performed in volume-control mode, VT of 10 mL/kg, I:E ratio of 1:2, FIO2 between 0.95 and 0.98, no inspiratory pause, an inspiratory rise time of 0%, and fR was adjusted to maintain PETCO2 between 28 and 35 mmHg. The cardiovascular and gas exchange data were collected at 5, 30, 60, 120, and 180 min (T5–T180, respectively) of mechanical ventilation with the investigated EEP. Arterial and mixed venous blood samples (1 ml) were simultaneously and anaerobically collected for the immediate measurement of their respective hemoglobin concentration (Hba and Hb), hemoglobin oxygen saturation (SaO2 and SO2) (OSM 3 co-oximeter, Radiometer, CA, USA), partial pressure of carbon dioxide (PaCO2 and PCO2), partial pressure of oxygen (PaO2 and PO2), lactate concentration, and pH (pHa and pH) (ABL825, Radiometer Medical ApS, Denmark). The blood gas values were corrected to the actual body temperature at the time of blood collection. Once the train-of-four ratio had been ≥100 % for more than 15 min and the cats resumed spontaneous ventilation, a bronchoalveolar lavage unrelated to this study was performed with 10 ml of saline (37°C) in the right caudal lung lobe under bronchoscopy guidance. After that, the delivery of isoflurane was stopped, and the cats were recovered from anesthesia. Meloxicam (VetOne, ID, USA) at a dose of 0.2 mg/kg was administered subcutaneously at the end of the experiment, and cats were returned to the vivarium. After the completion of the study, all cats were adopted to individuals pre-selected by the University of California, Davis IACUC.
Heart rate (HR), systolic (SAP), MAP, and diastolic (DAP) arterial pressures, CVP, MPAP, pulmonary artery occlusion pressure (PAOP), cardiac output (CO), fR, PETCO2, ETISO, and body temperature were measured during the experiments. Stroke index (SI), CI, systemic vascular resistance index (SVRI), pulmonary vascular resistance index (PVRI), arterial blood oxygen concentration (CaO2), mixed venous blood oxygen concentration (CO2), oxygen delivery index (DO2I), oxygen consumption index (VO2I), oxygen extraction ratio (O2ER), PaO2:FIO2, shunt fraction (Qs/Qt), and PaCO2 minus PETCO2 [P(a-ET)CO2] were calculated using standard formulae (19, 20).
The primary outcomes of the study were HR, MAP, CI, SI, MPAP, CVP, SVRI, PVRI, Qs/Qt, and DO2I. Because this is the very first study evaluating the cardiovascular effects of PEEP in cats, there was no previous data set to perform an optimal power analysis. Alternatively, cardiovascular data from a previous study performed in cats from the same colony and in similar laboratory conditions were used (21). Six cats were enough to detect a difference of 20–30% (effect size of 1.1) in the primary outcomes of this project between and within each EEP treatment with a power of 0.8151 and an alpha level of 0.05. The numeric data were verified for normality with the Shapiro–Wilk test. Normally and non-normally distributed data are reported as mean ± standard deviation (SD) and median (range), respectively. A Kruskal–Wallis test followed by Dunn's test was performed to compare the PEEPmaxCrs identified for each EEP treatment. All cardiovascular and respiratory variables were compared between treatments and within each treatment. For normally distributed data, a mixed model analysis of variance (using EEP treatment, timepoints, and their interaction as fixed effects and cat as a random effect) followed by Dunnett's test to compare each time point with T5 within each treatment, and Tukey's procedure for comparisons between treatments within the same time point were used. For non-normally distributed data, the Friedman test followed by Dunn's test for comparisons between each time point and T5 within the same treatment, and for comparisons between treatments within the same time point. The level of significance for all statistical analyses was p ≤ 0.05.
All cats recovered from all anesthetic episodes without complications. Data from one cat in PEEPmaxCrs at T180 were not included in the results due to arterial catheter malfunction during that time point. The order of treatment administration in the cats of the study is presented in Table 1.
PEEPmaxCrs was 4 (4–4), 4 (4–6), 4 (4–4), and 4 (4–6) cmH2O in ZEEP, PEEPmaxCrs−2, PEEPmaxCrs, and PEEPmaxCrs+2, respectively, with no significant difference between treatments. The values of body temperature, ETISO, VT, f , and EEP measured during the experiments are presented in Table 2. No significant differences between and within each EEP treatment were observed for body temperature, ETISO, and VT, while EEP was significantly different between EEP treatments at all time points (p < 0.0001), as expected from the study design.
Table 2. Temperature, end-tidal isoflurane concentration, and ventilator variables in six isoflurane-anesthetized cats mechanically ventilated for 3 h with a tidal volume of 10 ml/kg and four end-expiratory pressure (EEP) treatments: zero end-expiratory pressure (ZEEP), positive end-expiratory pressure (PEEP) of highest respiratory system compliance (PEEPmaxCrs), PEEPmaxCrs minus 2 cmH2O (PEEPmaxCrs−2), and PEEPmaxCrs plus 2 cmH2O (PEEPmaxCrs+2).
The cardiovascular results for each treatment are summarized in Table 3. MAP was significantly lower at T5 during PEEPmaxCrs+2 compared to ZEEP (p = 0.0492). At T60, SI was significantly lower during PEEPmaxCrs+2 compared to ZEEP (p = 0.0135). MPAP was significantly higher during PEEP maxCrs+2 compared to ZEEP at all timepoints (T5 p = 0.0107; T30 p = 0.002; T60, T120 and T180 p < 0.0001), when compared to PEEPmaxCrs−2 at T60 (p = 0.0209), T120 (p = 0.0149) and T180 (p = 0.0147), and when compared to PEEPmaxCrs at T60 (p = 0.0252). CVP was significantly lower during ZEEP compared to PEEPmaxCrs+2 at T5 (p = 0.0344) and T30 (p = 0.0292). PVRI was higher during PEEPmaxCrs compared to ZEEP at T30 (p = 0.0131), T120 (p = 0.036), and T180 (p =0.0202), and compared to PEEPmaxCrs−2 at T30 (p = 0.0202) and T180 (p = 0.0306). PVRI was also higher during PEEPmaxCrs+2 compared to ZEEP at T60 (p = 0.0453) and compared to PEEPmaxCrs−2 at T60 (p = 0.0202), T120 (p = 0.0453), and T180 (p = 0.0453). Dopamine was administered in one cat from 4 to 18 min of ventilation with PEEPmaxCrs [total dose 0.07 mg/kg (rate of 5 mcg/kg/min)], and in three cats during all timepoints with PEEPmaxCrs+2 [total dose 0.84 mg/kg (rate of 5 mcg/kg/min), total dose 1.17 mg/kg (rate of 5–10 mcg/kg/min), and total dose 0.46 mg/kg (rate of 5 mcg/kg/min)]. One cat in ZEEP (T30), PEEPmaxCrs (T5 and T30), and PEEPmaxCrs+2 (T5, T30, T60, and T120) had a MAP <60 mmHg either due to insufficient time for dopamine to exert its effect immediately after the ARM or because of the decrease in MAP caused by an additional dose of rocuronium administered close to the time point.
Table 3. Selected cardiovascular variables in six (unless indicated within parenthesis) isoflurane-anesthetized cats mechanically ventilated for 3 h with a tidal volume of 10 ml/kg and four end-expiratory pressure (EEP) treatments: zero end-expiratory pressure (ZEEP), positive end-expiratory pressure (PEEP) of highest respiratory system compliance (PEEPmaxCrs), PEEPmaxCrs minus 2 cmH2O (PEEPmaxCrs−2), and PEEPmaxCrs plus 2 cmH2O (PEEPmaxCrs+2).
Pulmonary gas exchange and oxygenation variables as well as arterial hemoglobin (Hba) and lactate concentrations are presented in Table 4. DO2I was significantly greater at T180 compared to T5 in the cats ventilated with PEEPmaxCrs (p = 0.05). Ventilation with ZEEP resulted in a lower PaO2/FIO2 compared to PEEPmaxCrs−2 (T120, p = 0.0121 and T180, p = 0.023), PEEPmaxCrs (T30, p = 0.0269 and T120, p = 0.0269), and PEEPmaxCrs+2 (T30, T60 and T120, p = 0.006; T180 p = 0.001).
Table 4. Pulmonary gas exchange, arterial hemoglobin (Hba) and lactate concentrations, and oxygenation variables in six (unless indicated within parenthesis) isoflurane-anesthetized cats mechanically ventilated for 3 h with a tidal volume of 10 ml/kg and four end-expiratory pressure treatments: zero end-expiratory pressure (ZEEP), positive end-expiratory pressure (PEEP) of highest respiratory system compliance (PEEPmaxCrs), PEEPmaxCrs minus 2 cmH2O (PEEPmaxCrs−2), and PEEPmaxCrs plus 2 cmH2O (PEEPmaxCrs+2).
The acid–base variables are presented in Table 5. The cats ventilated with ZEEP had a lower PaCO2 compared to PEEPmaxCrs+2 at T5 (p = 0.0349) and T30 (p = 0.0127), and to PEEPmaxCrs at T5 (p = 0.0432).
Table 5. Acid-base variables in six (unless indicated within parenthesis) isoflurane-anesthetized cats mechanically ventilated for 3 h with a tidal volume of 10 ml/kg and four end-expiratory pressure (EEP) treatments: zero end-expiratory pressure (ZEEP), positive end-expiratory pressure (PEEP) of highest respiratory system compliance (PEEPmaxCrs), PEEPmaxCrs minus 2 cmH2O (PEEPmaxCrs−2), and PEEPmaxCrs plus 2 cmH2O (PEEPmaxCrs+2).
Mechanical ventilation is commonly used during the anesthetic management of cats (22). However, the effects of different ventilatory settings on gas exchange and the cardiovascular system are poorly understood in this species. The present study aimed to partially fill this knowledge gap by investigating the effects on the cardiovascular system, and on gas exchange and arterial oxygenation of four different levels of EEP during 3 h of mechanical ventilation, which encompasses the duration of most anesthetic procedures performed in cats. The main findings of this study were that (1) all levels of PEEP studied minimally improved arterial oxygenation with no significant improvement in DO2I when compared to ZEEP; (2) PEEPmaxCrs and PEEPmaxCrs+2 were associated with lower MAP and higher requirements for dopamine to maintain MAP > 60 mmHg compared to ZEEP and PEEPmaxCrs−2; and (3) PEEPmaxCrs and PEEPmaxCrs+2 resulted in higher MPAP and PVRI than ZEEP and PEEPmaxCrs−2.
The ideal PEEP to be used in anesthetized patients has been a topic of debate. A fixed PEEP of 5 cmH2O after an ARM has been shown to prevent atelectasis and improve arterial oxygenation in dogs (3). However, variables commonly found in clinical cases such as obesity, surgical procedure (e.g., laparoscopic surgeries), and concurrent lung disease may alter the PEEP that can provide optimal improvement in pulmonary function. A recent guideline on lung-protective ventilation for surgical human patients (23) suggested that individualized mechanical ventilation settings including PEEP can improve clinical outcomes (24). One of the first methods described to individualize PEEP used the PEEPmaxCrs achieved during a decremental PEEP titration, which was associated with optimal cardiopulmonary function in critical human patients (25). In addition, PEEPmaxCrs promoted a better balance between preventing alveolar tidal recruitment/derecruitment and overdistention when compared to higher PEEP or ZEEP in lung-healthy rats (11). This beneficial effect of PEEPmaxCrs may explain why this level of PEEP was associated with improved clinical outcomes in critical human patients (10). Levels of PEEP higher and lower than PEEPmaxCrs such as PEEPmaxCrs−2 and PEEPmaxCrs+2 have been investigated to better understand the balance between the improvement in pulmonary function and the possible detrimental effects of PEEP, such as decrease in MAP and CI (5, 26). Although most clinical studies using PEEPmaxCrs have been performed in critical patients, healthy cats can develop atelectasis during anesthesia (6), which can predispose to ventilator-induced lung injury. Therefore, the results reported in this study may significantly contribute to the management of mechanical ventilation in lean healthy cats, as well as serve as reference for future studies in this species using PEEP in a variety of clinical conditions, including obesity and critical illness.
Despite its beneficial effects on pulmonary function, PEEP can significantly decrease CI and MAP, as recently demonstrated in dogs, especially when PEEP was higher than PEEPmaxCrs (5). Similar effects were found in cats, with PEEPmaxCrs+2 causing a more sustained and significant decrease in cardiovascular function as illustrated by the lower MAP at T5 when compared to ZEEP and by the need for dopamine to maintain MAP > 60 mmHg during the entire ventilation period in 3 out of 6 cats. As observed in dogs (5, 27, 28), high PEEP decreased SI, an important contributing factor for the more significant cardiovascular depression during PEEPmaxCrs+2 in the cats of this study. The administration of dopamine to maintain MAP > 60 mmHg masked the magnitude of the actual depression in SI, CI, and MAP caused by PEEP, particularly at PEEPmaxCrs+2. Dopamine was used in this experiment because it was considered unethical to tolerate severe hypotension in a survival study. Although PEEP decreases CI and MAP mainly by decreasing venous return with no apparent decrease in ventricular function (27), dopamine, at predominantly positive inotropic doses, is effective to treat the decrease in CI and MAP caused by PEEP in human patients with acute respiratory failure (29). At the doses used in the present study, dopamine has a predominant positive inotropic effect in cats (30, 31). However, selective venoconstriction caused by low doses of dopamine (32) promoting an increase in venous return is another possible mechanism for the improvement in CI and MAP in the cats ventilated with PEEPmaxCrs and PEEPmaxCrs+2. At the doses used in this study (5 and 10 mcg/kg/min), dopamine was effective at maintaining CI and MAP at values similar to spontaneously breathing cats anesthetized with comparable ETISO (33) and can be considered a good option to manage the cardiovascular depression caused by PEEP in cats.
Only one cat ventilated with PEEPmaxCrs required dopamine during the first 20 min of the ventilation protocol. This effect was likely related to the summation of cardiovascular depression caused by the ARM performed immediately before the ventilation protocol and the PEEP settings and has been reported in dogs (5). We attempted to minimize the influence of the cardiovascular depression caused by the ARM by administering a bolus of isotonic crystalloids in all cats immediately before the ARM, as described in dogs (34). A temporal improvement in CI due to fluid retention related to sustained positive pressure ventilation with PEEP (35) and/or a possible temporal decrease in the cardiovascular depression of isoflurane (36) could have also played a role in the lack of need for dopamine in this cat after 20 min of ventilation. However, both mechanisms of a temporal increase in CI take more than 20 min to occur (35, 36) and were deemed unlikely to have contributed. Interestingly, a temporal increase in CI was observed in dogs after 180 min of ventilation at different levels of EEP (5). Although a tendency (p = 0.0629) of higher CI was noted at T180 when compared to T5 with all studied EEPs, this effect was not detected in the present study because of three possible reasons: (1) small sample size and its associated low statistical power, (2) species-specific differences in the dynamics of fluid retention and isoflurane effects between dogs and cats, and (3) the confounding factor of the use of dopamine.
The augmented lung volume caused by PEEP can increase MPAP and PVRI (5, 28). In this study, PVRI was higher during ventilation with PEEPmaxCrs and PEEPmaxCrs+2 when compared to ZEEP and PEEPmaxCrs−2. Therefore, caution should be used when using those levels of PEEP in cats with right ventricular dysfunction and pulmonary hypertension. The increased right ventricle afterload associated with higher PVRI in PEEPmaxCrs and PEEPmaxCrs+2 was a probable contributor to the decrease in CI and MAP at these PEEP levels. In contrast with these findings, PVRI was less affected by PEEP in dogs since it only increased when a PEEP 4 cmH2O higher than PEEPmaxCrs was used (5). The use of dopamine and lower VT in the present study are the main methodological differences between the previous dog study and are unlikely to explain the increase in PVRI at lower PEEP levels in cats when compared to dogs. Intravenous dopamine at doses up to 20 mcg/kg/min was not associated with increased PVRI in cats (31). The highest dose used in this study was 10 mcg/kg/min. The lower VT used in the cats is expected to be associated with a lower PVRI as PVRI increases with VT (37). This difference can likely be related to a species-specific difference in the effects of PEEP, as data from the same laboratory indicates that PVRI is higher in cats than in dogs (19, 31).
The decreased atelectasis and increased FRC caused by PEEP have been associated with significant improvement in pulmonary gas exchange (1, 3). Nevertheless, the improvement in PaO2/FIO2 observed in all studied PEEP levels when compared to ZEEP are considered small, with minimal clinical significance since PaO2/FIO2 remained within normal limits with all treatments. The improvement in PaO2/FIO2 caused by PEEP was more important toward the end of the ventilation protocol and was likely caused by a faster temporal development of atelectasis and deterioration of respiratory mechanics in the cats ventilated with ZEEP compared to PEEP as previously demonstrated in dogs (3) and rats (11). When excessive, PEEP can cause alveolar overdistention and decrease pulmonary perfusion, which can ultimately impair pulmonary gas exchange as a consequence of increased / (alveolar dead space) (13). None of the PEEP treatments appeared to increase / in cats because no increase in P(a-ET)CO2 was observed with PEEP even at its highest level. Increased P(a-ET)CO2 has been commonly used as a marker of alveolar dead space but it has serious limitations in the presence of increased Qs/Qt, as demonstrated in anesthetized horses (38). Because Qs/Qt was normal in all EEP treatments, it is safe to assume that the lack of elevation in P(a-ET)CO2 caused by PEEP in this study indicated no increase in alveolar dead space. Similar effects of PEEP on PaO2/FIO2 and P(a-ET)CO2 were observed in dogs (5) and future studies are necessary to clarify the clinical significance of the mildly improved pulmonary gas exchange promoted by PEEP in healthy cats.
One of the clinical goals of improving arterial oxygenation in anesthetized patients is to increase CaO2 and consequently DO2I. In critically ill human patients, PEEPmaxCrs was associated with optimal cardiopulmonary function with improved DO2I (25). However, this was not achieved in healthy cats with any of the PEEP treatments. The CI depression caused by PEEP, especially at high levels, can be associated with a detrimental effect on DO2I (5). Interestingly, DO2I improved at T180 when compared to T5 in the cats ventilated with PEEPmaxCrs, probably due to a combination of nonsignificant improvements in CI and CaO2 over time observed with this level of PEEP. In dogs ventilated with PEEPmaxCrs and PEEPmaxCrs+2, a similar temporal improvement in DO2I was caused by a progressive increase in CI at the fourth hour of ventilation. There was a tendency of a temporal increase in DO2I at the other levels of PEEP and is possible that the small sample size used in this study did not provide enough power to reach statistical differences.
This study has important limitations that need to be accounted for when interpreting the results. As previously discussed, the use of dopamine to treat hypotension during the experiments has mitigated the decrease in CI and MAP due to PEEPmaxCrs and PEEPmaxCrs+2. However, the dose of dopamine required to maintain MAP > 60 mmHg provides an indirect but reliable assessment of the cardiovascular depression related to each treatment. In cats, ventilation with lower FIO2 (0.4) improved lung aeration distribution with less atelectasis and better gas exchange than at FIO2 close to 1.0 (6). Therefore, it is possible that the beneficial effect of PEEP on gas exchange observed in this study would not be achieved if the cats were ventilated with a lower FIO2 as previously reported in dogs (3). The results reported on healthy lean cats in dorsal recumbency should be taken with caution when applied to different body positions (e.g., lateral or sternal recumbency), cats with a different body condition score and critically ill cats, and especially cats with pulmonary disease because all these conditions are associated with altered respiratory mechanics and gas exchange where PEEPmaxCrs could be different than the conditions in this study. The administration of 10 ml/kg of lactated Ringer's solution before an ARM has been shown to minimize the decrease in MAP and CI in dogs (34) before the ARM. The same technique probably made the cats of the present study less sensitive to the preload effects of PEEP, and because of that, the cardiovascular effects of PEEP will likely be magnified in cats not receiving the same fluid bolus. At the first 30 min of ventilation, PaCO2 was higher in the cats ventilated with PEEPmaxCrs and PEEPmaxCrs+2 when compared to ZEEP and was probably an incidental finding due to the initial adjustments of RR after the ARM. These PaCO2 differences could have promoted an increase in sympathetic tone improving MAP and CI (39), masking further depression of CI and MAP by those PEEP values. This effect was unlikely to have affected our results since significant improvements of CI and MAP were only observed with differences of PaCO2 higher than approximately 17 mmHg (39). Other confounding factors such as ETISO and body temperature were well controlled during the experiments with no difference between treatments or time points. Finally, the cats of this study did not undergo any surgical procedure, which can produce significant changes in cardiovascular function, respiratory mechanics, and gas exchange, especially with intraabdominal or intrathoracic procedures. For instance, laparotomy and thoracotomy caused significant changes in lung compliance and resistance in rats (40, 41), which can lead to different requirements of PEEP to achieve optimal cardiopulmonary function.
In isoflurane-anesthetized lung-healthy cats ventilated for 3 h with a VT of 10 ml/kg after an ARM:
• none of the PEEP levels studied promoted clinically significant improvement in gas exchange;
• PEEPmaxCrs and PEEPmaxCrs+2 produced more cardiovascular depression, which was mild and limited to the first 20 min of ventilation in PEEPmaxCrs;
• none of the PEEP levels improved DO2I but a temporal increase on this variable was observed, particularly with PEEPmaxCrs;
• the cardiovascular effects of PEEPmaxCrs−2 were not significantly different than ZEEP;
• dopamine was effective at mitigating the cardiovascular depression produced by PEEP; and
• the effects of these levels of EEP on respiratory mechanics and ventilation-induced lung injury, as well as their use in different clinical situations, such as obese and critically ill cats, deserve future investigation.
The raw data supporting the conclusions of this article will be made available by the authors, without undue reservation.
The animal study was reviewed and approved by University of California Davis Institutional Animal Use and Care Committee.
MM: study execution, data acquisition, analysis, interpretation, and preparation of the manuscript, approved the final manuscript, and full access to all the data and responsible for integrity of the data and accuracy of data analysis. JS: study design, study execution, data acquisition, analysis, interpretation, and preparation of the manuscript, approved the final manuscript, and full access to all the data and responsible for integrity of the data and accuracy of data analysis. BP: study execution, data analysis, interpretation and preparation of the manuscript, and approved the final manuscript. AA: study execution, data acquisition, analysis, interpretation, and preparation of the manuscript, and approved the final manuscript. GM-R, FJ, and CB: data analysis, interpretation, and preparation of the manuscript and approved the final manuscript. All authors contributed to the article and approved the submitted version.
This work was supported by Center for Companion Animal Health (Resident Grant No. 38665 and Faculty Grant No. 44336), School of Veterinary Medicine, University of California, Davis.
The authors declare that the research was conducted in the absence of any commercial or financial relationships that could be construed as a potential conflict of interest.
All claims expressed in this article are solely those of the authors and do not necessarily represent those of their affiliated organizations, or those of the publisher, the editors and the reviewers. Any product that may be evaluated in this article, or claim that may be made by its manufacturer, is not guaranteed or endorsed by the publisher.
The authors would like to thank the Center of Companion Animal Health (University of California, Davis) and the University of California Library for contributing for paying the fees of this publication.
1. Tusman G, Bohm SH, Vazquez de. Anda GF, do Campo JL, Lachmann B. ‘Alveolar recruitment strategy' improves arterial oxygenation during general anaesthesia. Br J Anaesth. (1999) 82:8–13. doi: 10.1093/bja/82.1.8
2. Hedenstierna G. Oxygen and anesthesia: what lung do we deliver to the post-operative ward? Acta Anaesthesiol Scand. (2012) 56:675–85. doi: 10.1111/j.1399-6576.2012.02689.x
3. De Monte V, Grasso S, De Marzo C, Crovace A, Staffieri F. Effects of reduction of inspired oxygen fraction or application of positive end-expiratory pressure after an alveolar recruitment maneuver on respiratory mechanics, gas exchange, and lung aeration in dogs during anesthesia and neuromuscular blockade. Am J Vet Res. (2013) 74:25–33. doi: 10.2460/ajvr.74.1.25
4. De Monte V, Bufalari A, Grasso S, Ferrulli F, Crovace AM, Lacitignola L, et al. Respiratory effects of low versus high tidal volume with or without positive end-expiratory pressure in anesthetized dogs with healthy lungs. Am J Vet Res. (2018) 79:496–504. doi: 10.2460/ajvr.79.5.496
5. Soares JHN, Braun C, Machado ML, Oliveira RL, Henao-Guerrero N, Countermash-Ott S, et al. Cardiovascular function, pulmonary gas exchange and tissue oxygenation in isoflurane-anesthetized, mechanically ventilated beagle dogs with four levels of positive end-expiratory pressure. Vet Anaesth Analg. (2021) 48:324–33. doi: 10.1016/j.vaa.2021.01.007
6. Staffieri F, De Monte V, De Marzo C, Grasso S, Crovace A. Effects of two fractions of inspired oxygen on lung aeration and gas exchange in cats under inhalant anaesthesia. Vet Anaesth Analg. (2010) 37:483–90. doi: 10.1111/j.1467_2995.2010.00567.x
7. Henao-Guerrero N, Ricco C, Jones JC, Buechner-Maxwell V, Daniel GB. Comparison of four ventilatory protocols for computed tomography of the thorax in healthy cats. Am J Vet Res. (2012) 73:646–53. doi: 10.2460/ajvr.73.5.646
8. Luecke T, Pelosi P. Clinical review: positive end-expiratory pressure and cardiac output. Crit Care. (2005) 9:607–21. doi: 10.1186/cc3877
9. Suarez-Sipmann F, Bohm SH, Tusman G, Pesch T, Thamm O, Reissmann H, et al. Use of dynamic compliance for open lung positive end-expiratory pressure titration in an experimental study. Crit Care Med. (2007) 35:214–21. doi: 10.1097/01.CCM.0000251131.40301.E2
10. Pintado MC, de Pablo R, Trascasa M, Milicua JM, Sanchez-Garcia M. Compliance-guided versus Fio(2)-driven positive-end expiratory pressure in patients with moderate or severe acute respiratory distress syndrome according to the Berlin definition. Med Inten. (2017) 41:277–84. doi: 10.1016/j.medin.2016.08.009
11. Soares JHN, Carvalho AR, Bergamini BC, Gress MAK, Jandre FC, Zin WA, et al. Alveolar tidal recruitment/derecruitment and overdistension during four levels of end-expiratory pressure with protective tidal volume during anesthesia in a murine lung-healthy model. Lung. (2018) 196:335–42. doi: 10.1007/s00408-018-0096-8
12. Carvalho AR, Spieth PM, Pelosi P, Vidal Melo MF, Koch T, Jandre FC, et al. Ability of dynamic airway pressure curve profile and elastance for positive end-expiratory pressure titration. Intensive Care Med. (2008) 34:2291–9. doi: 10.1007/s00134-008-1301-7
13. Hedenstierna G, White FC, Mazzone R, Wagner PD. Redistribution of pulmonary blood flow in the dog with peep ventilation. J Appl Physiol Respir Environ Exerc Physiol. (1979) 46:278–87. doi: 10.1152/jappl.1979.46.2.278
14. Steffey EP, Howland Jr. D. Isoflurane potency in the dog and cat. Am J Vet Res. (1977) 38:1833–6
15. Lanteri CJ, Kano S, Sly PD. Validation of esophageal pressure occlusion test after paralysis. Pediatr Pulmonol. (1994) 17:56–62. doi: 10.1002/ppul.1950170110
16. Pino A, Kagami L, Jandre F, Giannella-Neto A editors. Das-Um Programa De AquisiçãO E Processamento De Sinais Para Engenharia Pulmonar. In: III CLAEB: Anais do 3° Congresso Latino Americano de Engenharia Biomédica (2004).
17. Yeh MP, Gardner RM, Adams TD, Yanowitz FG. Computerized determination of pneumotachometer characteristics using a calibrated syringe. J Appl Physiol Respir Environ Exerc Physiol. (1982) 53:280–5. doi: 10.1152/jappl.1982.53.1.280
18. Pino A, Costa J, Jandre F, Giannella A editors. MecâNica-Um Programa Para AnáLise Do Sistema Respiratório. In: Congresso Brasileiro de Engenharia Biomédica: Anais do XVIII Congresso Brasileiro de Engenharia Biomédica (2002).
19. Haskins S, Pascoe PJ, Ilkiw JE, Fudge J, Hopper K, Aldrich J. Reference cardiopulmonary values in normal dogs. Comp Med. (2005) 55:156–61.
20. Pypendop BH, Jones JH. Indexing cardiovascular and respiratory variables: allometric scaling principles. Vet Anaesth Analg. (2015) 42:343–9. doi: 10.1111/vaa.12276
21. Siao KT, Pypendop BH, Honkavaara J, Ilkiw JE. Hemodynamic effects of dexmedetomidine, with and without Mk-467, following intramuscular administration in cats anesthetized with isoflurane. Vet Anaesth Analg. (2017) 44:1101–15. doi: 10.1016/j.vaa.2017.02.010
22. Mosley C. Anesthesia equipment. In: Grimm KA, Lamont LA, Tranquilli WJ, Greene SA, Robertson SA, editors. Veterinary Anesthesia and Analgesia. 5th ed. Iowa: Wiley (2015). p. 23–85.
23. Young CC, Harris EM, Vacchiano C, Bodnar S, Bukowy B, Elliott RRD, et al. Lung-protective ventilation for the surgical patient: international expert panel-based consensus recommendations. Br J Anaesth. (2019) 123:898–913. doi: 10.1016/j.bja.2019.08.017
24. Pereira SM, Tucci MR, Morais CCA, Simoes CM, Tonelotto BFF, Pompeo MS, et al. Individual positive end-expiratory pressure settings optimize intraoperative mechanical ventilation and reduce postoperative atelectasis. Anesthesiology. (2018) 129:1070–81. doi: 10.1097/ALN.0000000000002435
25. Suter PM, Fairley B, Isenberg MD. Optimum end-expiratory airway pressure in patients with acute pulmonary failure. N Engl J Med. (1975) 292:284–9. doi: 10.1056/NEJM197502062920604
26. Camilo LM, Motta-Ribeiro GC, de Avila MB, Paula L, de Abreu MB, Carvalho AR, et al. Variable ventilation associated with recruitment maneuver minimizes tissue damage and pulmonary inflammation in anesthetized lung-healthy rats. Anesth Analg. (2018) 127:784–91. doi: 10.1213/ANE.0000000000003582
27. Marini JJ, Culver BH, Butler J. Effect of positive end-expiratory pressure on canine ventricular function curves. J Appl Physiol Respir Environ Exerc Physiol. (1981) 51:1367–74. doi: 10.1152/jappl.1981.51.6.1367
28. Marini JJ, Culver BH, Butler J. Mechanical effect of lung distention with positive pressure on cardiac function. Am Rev Respir Dis. (1981) 124:382–6. doi: 10.1164/arrd.1981.124.4.382
29. Hemmer M, Suter PM. Treatment of cardiac and renal effects of peep with dopamine in patients with acute respiratory failure. Anesthesiology. (1979) 50:399–403. doi: 10.1097/00000542-197905000-00005
30. Aramaki Y, Uechi M, Takase K. Comparison of the cardiovascular effects of intracellular cyclic adenosine 3',5'-monophosphate (camp)-modulating agents in isoflurane-anesthetized cats. J Vet Med Sci. (2002) 64:981–6. doi: 10.1292/jvms.64.981
31. Pascoe PJ, Ilkiw JE, Pypendop BH. Effects of increasing infusion rates of dopamine, dobutamine, epinephrine, and phenylephrine in healthy anesthetized cats. Am J Vet Res. (2006) 67:1491–9. doi: 10.2460/ajvr.67.9.1491
32. Marino RJ, Romagnoli A, Keats AS. Selective venoconstriction by dopamine in comparison with isoproterenol and phenylephrine. Anesthesiology. (1975) 43:570–2. doi: 10.1097/00000542-197511000-00016
33. Jaeger AT, Pypendop BH, Ahokoivu H, Honkavaara J. Cardiopulmonary effects of dexmedetomidine, with and without vatinoxan, in isoflurane-anesthetized cats. Vet Anaesth Analg. (2019) 46:753–64. doi: 10.1016/j.vaa.2019.05.012
34. Canfran S, Gomez de. Segura IA, Cediel R, Garcia-Fernandez J. Effects of fluid load on cardiovascular function during stepwise lung recruitment manoeuvre in healthy dogs. Vet J. (2013) 197:800–5. doi: 10.1016/j.tvjl.2013.05.013
35. Marshall BE, Berry AJ, Marshall C, Geer RT. Influence of ventilation on response to fluid load in dogs: body water and albumin distribution. Anesthesiology. (1982) 57:103–10. doi: 10.1097/00000542-198208000-00007
36. Floriano BP, Wagatsuma JT, Ferreira JZ, Abimussi CJ, Menegheti TM, Santos PS, et al. Effects on indicators of tissue perfusion in dogs anesthetized with isoflurane at two multiples of the minimum alveolar concentration. Am J Vet Res. (2016) 77:24–31. doi: 10.2460/ajvr.77.1.24
37. Cheifetz IM, Craig DM, Quick G, McGovern JJ, Cannon ML, Ungerleider RM, et al. Increasing tidal volumes and pulmonary overdistention adversely affect pulmonary vascular mechanics and cardiac output in a pediatric swine model. Crit Care Med. (1998) 26:710–6. doi: 10.1097/00003246-199804000-00020
38. Mosing M, Bohm SH, Rasis A, Hoosgood G, Auer U, Tusman G, et al. Physiologic factors influencing the arterial-to-end-tidal co2 difference and the alveolar dead space fraction in spontaneously breathing anesthetised horses. Front Vet Sci. (2018) 5:58. doi: 10.3389/fvets.2018.00058
39. Rasmussen JP, Dauchot PJ, DePalma RG, Sorensen B, Regula G, Anton AH, et al. Cardiac Function and Hypercarbia. Arch Surg. (1978) 113:1196–200. doi: 10.1001/archsurg.1978.01370220082013
40. Santos RL, Santos MA, Sakae RS, Saldiva PH, Zin WA. Effects of longitudinal laparotomy on respiratory system, lung, and chest wall mechanics. J Appl Physiol. (1992) 72:1985–90. doi: 10.1152/jappl.1992.72.5.1985
Keywords: mechanical ventilation, positive end-expiratory pressure (PEEP), cat, anesthesia, cardiovascular, gas exchange
Citation: Machado ML, Soares JHN, Pypendop BH, Aguiar AJA, Braun C, Motta-Ribeiro GC and Jandre FC (2022) Cardiovascular and Gas Exchange Effects of Individualized Positive End-Expiratory Pressures in Cats Anesthetized With Isoflurane. Front. Vet. Sci. 9:865673. doi: 10.3389/fvets.2022.865673
Received: 30 January 2022; Accepted: 18 March 2022;
Published: 04 May 2022.
Edited by:
Klaus Hopster, University of Pennsylvania, United StatesReviewed by:
Gabrielle Christine Musk, University of Western Australia, AustraliaCopyright © 2022 Machado, Soares, Pypendop, Aguiar, Braun, Motta-Ribeiro and Jandre. This is an open-access article distributed under the terms of the Creative Commons Attribution License (CC BY). The use, distribution or reproduction in other forums is permitted, provided the original author(s) and the copyright owner(s) are credited and that the original publication in this journal is cited, in accordance with accepted academic practice. No use, distribution or reproduction is permitted which does not comply with these terms.
*Correspondence: Joao H. N. Soares, amhzb2FyZXNAdWNkYXZpcy5lZHU=
Disclaimer: All claims expressed in this article are solely those of the authors and do not necessarily represent those of their affiliated organizations, or those of the publisher, the editors and the reviewers. Any product that may be evaluated in this article or claim that may be made by its manufacturer is not guaranteed or endorsed by the publisher.
Research integrity at Frontiers
Learn more about the work of our research integrity team to safeguard the quality of each article we publish.