- 1Henan Key Laboratory of Innovation and Utilization of Unconventional Feed Resources, Henan University of Animal Husbandry and Economy, Zhengzhou, China
- 2College of Veterinary Medicine, Henan Agricultural University, Zhengzhou, China
- 3School of Life Science and Engineering, Foshan University, Foshan, China
- 4Key Laboratory of Animal Immunology of the Ministry of Agriculture, Henan Provincial Key Laboratory of Animal Immunology, Henan Academy of Agricultural Sciences, Zhengzhou, China
Porcine reproductive and respiratory syndrome virus (PRRSV), one of the most serious animal pathogens in the world, has caused enormous global swine industry losses. An in-depth investigation of the PRRSV-host interaction would be beneficial for preventing and controlling PRRSV infections and transmission. In this study, we performed label-free quantitative proteomic assays to investigate proteome dynamics of porcine alveolar macrophages (PAMs) during infection with highly pathogenic PRRSV (HP-PRRSV) strain HN07-1. Analysis of the results led to identification of 269 significantly differentially expressed host cellular proteins, of which levels of proteins belonging to the eukaryotic translation initiation factor (eIF) family were found to be decreased in abundance in HP-PRRSV-infected PAMs. Furthermore, knockdown of eIF5A expression was demonstrated to markedly suppress HP-PRRSV propagation, as reflected by reduced progeny virus titers in vitro. These results highlight the importance of eIF5A in PRRSV infection, while also demonstrating that PAMs down-regulate eIF5A expression as a host cell antiviral strategy. Results of the current study deepen our understanding of PRRSV pathogenesis and provide novel insights to guide development of effective strategies to combat the virus.
Introduction
Porcine reproductive and respiratory syndrome (PRRS), a highly contagious disease caused by PRRS virus (PRRSV) infection, leads to reproductive disorders in sows and respiratory symptoms in pigs of all ages (1). PRRS was first discovered in North America in 1987 before it spread around the world, to cause substantial economic swine industry losses (2). In 2006, a severe epidemic of highly pathogenic (HP)-PRRS occurred in China that induced high fever in pigs and was associated with high mortality regardless of age. Importantly, the viral strain that was responsible for the epidemic possessed a 30-amino-acid discontinuous deletion within PRRSV non-structural protein 2 (nsp2) (3, 4).
PRRSV belongs to the family Arteriviridae (genus Betaarterivirus) within the taxonomic order Nidovirales and possesses a single-stranded positive-sense RNA genome of 15.4 kb (5). All PRRSV isolates are classified into two genotypes: PRRSV-1 and PRRSV-2 (6). PRRSV-2 strains were predominant in China. The cell tropism of PRRSV, which is highly limited, includes porcine alveolar macrophages (PAMs) that act as primary host cells to support viral infection (7). Importantly, PRRSV infection is a complex process such that mechanisms associated with PRRSV infection have not yet been fully clarified in spite of intensive research efforts.
Quantitative proteomic techniques, which are classified as label-based and label-free methods, have been used to research viral infections such as influenza virus (8), human respiratory syncytial virus (9), hepatitis B virus (10), porcine circovirus type 2 (11), foot and mouth disease virus (12), infectious bursal disease virus (13), African swine fever virus (14, 15). Consequently, results of these studies have created a foundation of knowledge on which to build future investigations to better understand pathogenesis of other viruses, including PRRSV.
In recent years, researchers have studied PRRSV infection using quantitative proteomic approaches. For example, Fang et al. (16) used an acetylation-based antibody enrichment technique and a tandem mass tag label high-affinity purification liquid chromatography-mass spectrometry (LC-MS/MS) method to study acetylome regulation of antiviral activities in PRRSV-infected PAMs. A few years earlier, Zhang et al. (17) had generated a broad-spectrum ubiquitination modification map of PRRSV-infected PAMs using ubiquitination antibody enrichment in combination with MS technology. Slightly earlier, Li et al. (18) conducted label-free quantitative proteomics to detect differentially secreted proteins in supernatants of PRRSV-infected PAMs and compared the results to those obtained for supernatants of uninfected PAMs. These studies not only enhanced our understanding of PRRSV infection, but also identified potential targets of antiviral drugs.
In order to better understand the proteome alterations of host cells during PRRSV infection, a label-free quantitative approach coupled with LC-MS/MS was applied to analyze the altered proteins in HP-PRRSV HN07-1-infected PAMs. The results of these experiments revealed that at 24 h post-infection (hpi), expression levels of 269 host cellular proteins were found to be significantly altered. Subsequent bioinformatic analyses revealed that these differentially expressed proteins were enriched for functional terms corresponding to several biological processes and KEGG pathways. Interestingly, expression levels of translation-related proteins, including eukaryotic translation initiation factors (eIFs), were significantly down-regulated after HP-PRRSV HN07-1 infection, with one such factor, eIF5A, found to be essential for viral replication. The results of this work uncovered for the first time the importance of eIF5A during virus infection, while also providing insights into host-pathogen interactions that occur during PRRSV infection to guide future development of effective antiviral strategies.
Materials and Methods
Cells and Virus
PAMs were collected from 4-week-old pathogen-free piglets that had been previously confirmed to be uninfected with PRRSV, porcine circovirus type 2, foot-and-mouth disease virus, pseudorabies virus, classical swine fever virus, and porcine parvovirus. The PAMs collection procedure was approved by the Ethical and Animal Welfare Committee of the Key Laboratory of Animal Immunology of the Ministry of Agriculture of China (permit no. 2018005). Collected PAMs were cultured in RPMI-1640 medium (Hyclone, China) containing 10% fetal bovine serum (FBS, Gibco, USA), 100 μg/mL streptomycin, and 100 units/mL penicillin (Hyclone, China) in a humidified incubator maintained at 37°C with 5% CO2. MARC-145 cells were purchased from ATCC and passaged in our laboratory. CRL-2843-CD163 cells were obtained from Professor Enmin Zhou of the College of Veterinary Medicine, Northwest Agriculture and Forestry University. The HP-PRRSV HN07-1 strain (GenBank: KX766378.1) that was used in this study was isolated and identified by the Key Laboratory of Animal Immunology of the Ministry of Agriculture, Henan Provincial Key Laboratory of Animal Immunology, Henan Academy of Agricultural Sciences (19).
Virus Inoculation
PAMs were infected with HP-PRRSV HN07-1 at a multiplicity of infection (MOI) of 0.1 then the cells were fixed in ice-cold 95% methyl alcohol at 12, 24, 36, and 48 hpi. Next, cells were blocked in 5% skim milk in phosphate buffer solution with Tween-20 (PBST) at 4°C for 12 h. Thereafter, an immunofluorescence assay (IFA) was conducted to detect viral propagation at different time points post-infection using anti-PRRSV nucleocapsid (N) protein antibody as a probe (GeneTex, USA). Thereafter, cells were incubated with fluorescein isothiocyanate (FITC)-labeled goat-anti-mouse IgG (Sigma, USA) as secondary antibody. After incubation with primary and secondary antibodies, the cells were washed with PBST then 4′,6-diamidino-2-phenylindole-dihydrochloride (DAPI, Beyotime, China) was added to cells followed by incubation at room temperature for 30 min to stain the nuclei. Next, an OLYMPUS IX 81 confocal microscope equipped with a digital camera was used to capture the fluorescent images. Then the one-step growth curve of HP-PRRSV HN07-1 in PAMs was plotted based on virus titers obtained at 12, 24, 36, 48, 60, and 72 hpi.
Sample Preparation, Protein Isolation, and Protein Digestion
PRRSV-infected and uninfected PAMs were gently washed with PBS, then were treated with 0.25% trypsin-EDTA (Solarbio, China) for 2 min. Next, cell suspensions were centrifuged at 1,200 rpm for 6 min then the cells were stored at −80°C until needed for further investigations. Protein extractions from PAMs were conducted as follows: frozen protein pellets were homogenized in lysis buffer (8 M urea, 2 M thiourea, 4% CHAPS, 20 mM Tris base, 30 mM dithiothreitol (DTT), and 2% Bio-Lyte) on ice. After that, samples were subjected to ultrasonic treatment followed by centrifugation at 13,500 rpm at 4°C for 20 min. Thereafter, each supernatant was removed then precipitated with ice-cold acetone at −20°C for 30 min then centrifuged twice (13,500 rpm for 10 min at 4°C) to pellet the proteins. Next, each pellet was collected and dissolved in 40 mM NH4HCO3 in which DTT was added to a final concentration of 100 mM, and iodoacetamide was also added to a final concentration of 50 mM. Thereafter, reductive alkylation was allowed to proceed for 1 h in dark at room temperature. Protein concentrations were quantified using the Bradford assay (20).
To conduct protein digestions, denatured proteins were reduced in 100 mM DTT and alkylated with 50 mM iodoacetamide to prevent reformation of disulfide bonds. Next, samples were digested with sequencing grade modified trypsin (Promega, USA) then were incubated at 37°C for 14 h. Finally, peptides were pooled and dried using a Speed-Vac system (RVC 2–18, Marin Christ, Germany) then were analyzed via MS/MS.
LC–MS/MS Analysis
Each dried sample was dissolved in 1 formic acid aqueous solution and centrifuged at 14,000 rpm for 20 min at 4°C. Next, each supernatant was gently transferred to a clean tube to avoid creation of bubbles. Prior to MS analysis, digested peptides were suspended in 15 μL of 0.1% formic acid then 10 μL of each peptide sample was subjected to LC-MS/MS analysis using an Easy nLC1000 System (Thermo Fisher Scientific, USA) coupled to a Q Exactive Orbitrap Spectrometer (Thermo Fisher Scientific) that was equipped with a nanoelectrospray ion source (capillary temperature 275°C, spray voltage 2.3 kV, and S-Lens RF 55%). After addition of loading solvent (2% acetonitrile and 0.1% formic acid in H2O) to the tryptic digests, the samples were loaded onto an Easy-Spray column filled with 2 μm C18 resin (75 μm ×50 cm, 100 Å, Thermo Fisher Scientific). Peptides were separated over a period of 130 min using a gradient consisting of 3 to 30% acetonitrile (containing 0.1% formic acid) using an analytical column packed with 3 μm C18 (75 μm × 15 cm, 100 Å, Thermo Fisher Scientific). The mass spectrometer was tuned to positive ion mode, MS scan control was maintained using Xcalibur software 2.2 (Thermo Fisher Scientific), MS data acquisition was data-dependent, repetition count was set to 1, exclusion duration was set to 30 s, and dynamic exclusion was enabled. MS1 precursor scan (m/z 300–2,000) acquisition was carried out in the orbitrap with a nominal resolution of 30,000 at m/z 400. Next, MS/MS fragmentation of the top 20 most intense multiply charged precursor ions was conducted using higher energy collisional dissociation with 35% normalized fragmentation energy. MS2 scans (m/z 100–2,000) were performed using the orbitrap mass analyzer at a resolution setting of 15,000 at m/z 400 and a starting m/z setting of 100.
Data Analysis and Protein Quantification
MS raw data files were retrieved using Xcalibur 2.2 (Thermo Fisher Scientific) and searched using PEAKS 7.5 against the Sus Scrofa database, which contained 38,431 entries when it was downloaded in July 2020 from the NCBI-ref database (21). Search parameters were set as follows: parent mass error tolerance of 20.0 ppm, fragment mass error tolerance of 0.05 Da, enzyme was trypsin. No specific cleavage site was selected for the peptide, maximum missed cleavages per peptide was set to 2, carbamidomethyl (C, +57.02) was selected as the fixed modification, and oxidation (M, +15.99) was selected as the variable modification. Each peptide had at most three types of posttranslational modifications. Protein selection parameters were as follows: false discovery rate (FDR) was ≤1.0% (-10l g P ≥ 20.0) and unique peptide with one spectrum was ≥1. The results of database retrieval were quantitatively analyzed using PEAKS Q and the peptide rate was calculated according to peak area. The conditions were set as follows: retention time shift tolerance of 1 min, mass error tolerance of 15 ppm, unique peptide ≥1, charge between 2 and 8, fold change of proteins and peptides was ≥1.5, and significance of ≥3 (P ≤ 0.05).
Bioinformatics Analysis
All identified proteins were used as inputs for functional analysis using ClueGO V2.1.7, a Cytoscape plug-in (http://www.ici.upmc.fr/cluego/), that comprehensively identifies proteins that are involved in various biological signal pathways and protein interactions (22). Protein FASTA files were blasted against the Sus scrofa database using GI numbers. Right-sided hypergeometric enrichment was conducted as a statistical test using the Bonferroni step-down correction method (P ≤ 0.05) using parameters of gene ontology score range of three to eight, Kappa threshold set to 0.4, and initial group size set to one. Protein-protein interaction (PPI) networks were constructed using another Cytoscape plug-in, GeneMANIA (23), which uses many functional association data, including protein and genetic interactions.
Real-Time PCR (RT-PCR)
Samples of total RNA of mock- and PRRSV-infected PAMs were prepared using TRIzol reagent (Invitrogen, USA) at 12 and 24 hpi, then cDNAs were generated from total RNA preparations using a reverse transcriptase kit according to the manufacturer's instructions (Takara, Japan). β-Actin served as an internal reference to normalize the data. Primer sequences are listed in Supplementary Table 1. RT-PCR assays were conducted using an Applied Biosystems 7,500 Fast RT-PCR System with 20 μL reactions (performed in triplicate) prepared that contained SYBR Green Premix 10 μL (ROCHE, Switzerland), 0.6 μL of each primer, and 6.8 μL of H2O. Each experiment was performed independently three times.
UV-Inactivation of PRRSV
The virus solution was irradiated by exposure to ultraviolet light of wavelength 254 nm that was emitted by a low-intensity ultraviolet lamp (120 mJ/cm2). Irradiation was conducted at room temperature for 30 min to inactivate the virus; the effectiveness of inactivation was assessed using RT-PCR.
Western Blot (WB)
Mock-infected, PRRSV-infected, and UV-inactivated PRRSV-treated PAMs were harvested at 0, 6, 12, and 24 hpi then the cells were lysed in RIPA buffer (Solarbio) containing 1% PMSF (Solarbio) for 30 min on ice followed by measurement of protein concentrations. For WB analysis, cell lysates containing equivalent concentrations of total protein were subjected to 12% SDS-PAGE then the separated proteins were transferred to 0.45 μm polyvinylidene difluoride (PVDF) membranes (Millipore, USA). After membranes were blocked in 5% skim milk at 4°C overnight, membranes were incubated with polyclonal antibodies specific for myxovirus-resistant protein 1 (Mx1) (Proteintech, China), tetratricopeptide repeats 3 (IFIT3) (Proteintech), PRRSV N (GeneTex, USA), or monoclonal antibody (mAb) specific for signal transducer and activator of transcription 1 (STAT1), eIF5A, eIF4E, eIF4H, 4EBP1, β-Actin (Cell Signaling Technology, USA). After being washed with PBST for three times, the membranes were treated with horseradish peroxidase (HRP)-conjugated goat anti-rabbit IgG or goat anti-mouse IgG secondary antibody (Abbkine, USA). A chemiluminescence kit (Beyotime) was used to detect signals resulting from antibodies binding to membrane-bound proteins.
SiRNA Transfection
SiRNAs targeting eIF5A, eIF4E and the negative control (NC) were synthesized by Gene Pharma (Shanghai, China) as described in Supplementary Table 2. CRL-2843-CD163 cells or PAMs were transfected with each indicated siRNA at a final concentration of 0.2 mM using Lipofectamine RNAiMAX (Invitrogen, USA) according to the manufacturer's instructions. Effects of transfected siRNAs after 24, 36, and 48 h were verified by RT-PCR using primers that are listed in Supplementary Table 1 and by WB analysis based on binding of mAb probes to eIF5A and eIF4E proteins. The rescue experiment was carried out according to a previous study (24), the siRNA targeting the 3′untranslated region (UTR) of eIF5A which was used in rescue experiment was synthesized by Gene Pharma (sequence is listed in Supplementary Table 2) and was used in the eIF5A knockdown experiment conducted in CRL-2843-CD163 cells. Cytotoxicity was assessed at 24, 36, and 48 h after siRNA transfection by adding MTS reagent to cells followed by incubation of cells at 37°C for 1 h. Absorbance was measured at 490 nm.
Effect of SiRNA-eIF5A on PRRSV Infection
After CRL-2843-CD163 cells or PAMs were subjected to eIF4E and eIF5A knockdown with appropriate siRNAs (or NC control), the cells were inoculated with HP-PRRSV HN07-1 (MOI = 0.1) and harvested at 12 and 24 hpi for RT-PCR analysis. CRL-2843-CD163 cells or PAMs after eIF5A knockdown were inoculated with HP-PRRSV HN07-1 (MOI = 0.1) and harvested at 24 hpi for IFA and WB analyses. PAMs after eIF5A knockdown were infected with HP-PRRSV HN07-1 (MOI = 0.1) and harvested at 48 hpi for determination of the median tissue culture infective dose (TCID50), which was conducted as follows: MARC-145 cells were cultured in 96-well plates overnight in DMEM (Solarbio) containing 10% FBS. Next, the cells were inoculated with diluted PRRSV at a MOI of 0.1 followed by incubation at 37°C for 3 h. After the cells were washed, DMEM containing 2% FBS was added to each well-then viral yields were calculated based on TCID50 values determined at 48 hpi as per the Reed-Munch method (25).
EIF5A Rescue Experiments
To determine whether recombinant eIF5A expression could reverse suppression of PRRSV infection due to eIF5A knockdown, we cloned eIF5A into a eukaryotic expression vector. Briefly, cDNA encoding eIF5A was amplified via PCR from full-length eIF5A cDNA (Gene ID: 100517970) using the primers listed in Supplementary Table 1. Next, the amplified PCR product was isolated and inserted into the 3*Flag-CMV-7.1 eukaryotic expression vector (Sigma) between the HindIII and XbaI sites. The recombinant 3*Flag-CMV-eIF5A eukaryotic expression vector was validated by the Shanghai Sangon Biotech Co. Ltd. (Shanghai, China). WB was used to confirm expression of 3*Flag-CMV-eIF5A protein in cells using anti-eIF5A mAb and anti-Flag mAb (Sigma). After siRNA targeting the 3′UTR of eIF5A was transfected into CRL-2843-CD163 cells, recombinant 3*Flag-CMV-eIF5A was transfected into the same cells then PRRSV propagation in the CRL-2843-CD163 cells was evaluated by RT-PCR, IFA, WB, and TCID50 assay. Cytotoxicity of 3*Flag-CMV-eIF5A after it was transfected into CRL-2843-CD163 cells (after eIF5A knockdown) was assessed at 24, 36, and 48 h post-transfection using the same method as described above.
Statistical Analysis
Experimental data were expressed as the mean and ± standard deviation (SD) based on triplicate samples then data were analyzed via Student's t-test using GraphPad Prism software (v8.0). Statistical significance is indicated in the figures as *, P < 0.05; **, P < 0.01; ***, P < 0.001; ns, not significant.
Results
Propagation Kinetics of HP-PRRSV HN07-1 in PAMs
First, PAMs were infected with HP-PRRSV HN07-1 at a MOI of 0.1. Next, to determine the appropriate time point for proteomic analysis, IFA was conducted of infected cells using a mAb probe specific for the viral N protein in order to determine the kinetics of HP-PRRSV HN07-1 infection in PAMs. As shown in Figure 1A, specific immunofluorescence became visible as early as 12 hpi that indicated that PRRSV propagation began at that time, with fluorescence significantly increasing after 24 hpi. At 36 hpi, fluorescence reached its maximum value then decreased at 48 hpi (Figure 1A). Based on these results, the one-step growth curve for HP-PRRSV HN07-1 in PAMs was plotted and it revealed that viral titers of HP-PRRSV HN07-1 reached a maximum of approximately 107.2 TCID50/mL at 36 hpi then gradually declined (Figure 1B). Based on IFA and growth curve results, we chose the time point of 24 hpi (before the titer peaked at 36 hpi) for use in subsequent proteomic analyses.
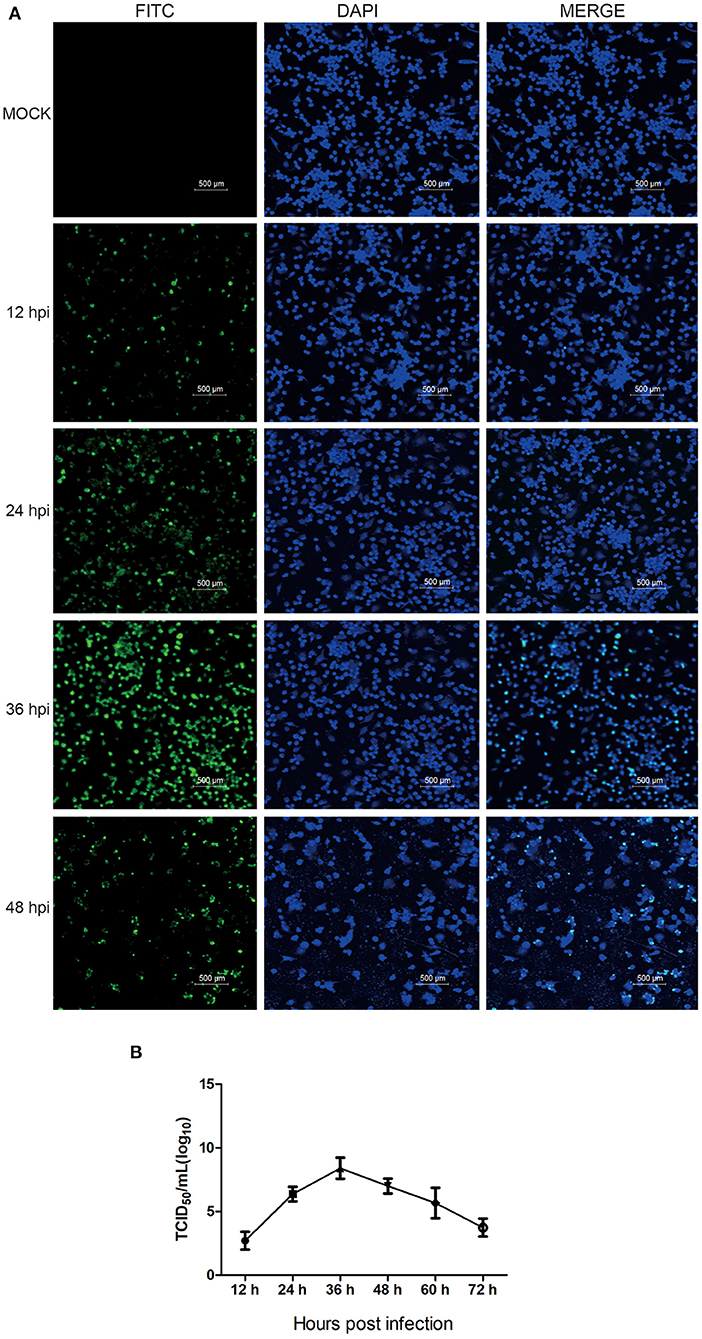
Figure 1. The propagation kinetics of HP-PRRSV HN07-1 in PAMs. (A) PAMs were infected with HP-PRRSV HN07-1 at MOI = 0.1 for 12, 24, 36, and 48 h or were mock-infected for 36 h as a control. Fluorescent images were recorded using an OLYMPUS IX 81 confocal microscope based on detection of virus using anti-PRRSV N protein antibody. (B) The one-step growth curve of HP-PRRSV HN07-1 in PAMs based on viral titers at 12, 24, 36, 48, 60, and 72 hpi.
LC-MS/MS Detection
Using mock-infected and HP-PRRSV HN07-1-infected PAMs, label-free quantitative proteome analysis was performed on triplicate samples then the data for each sample were analyzed using Peaks 7.5 software tool in order to conduct database searching and quantitative analysis. The results revealed detection of total numbers of peptides (29,854, 26,947) and proteins (3794, 3558) in mock-infected and HP-PRRSV HN07-1-infected PAMs, respectively (Supplementary Tables 3–6). All proteomic data were deposited into the ProteomeXchange Consortium (http://proteomecentral.proteomexchange.org) iProX partner repository (26) using the dataset identifier PXD026209.
Protein Quantification
Next, the quantitative function tool of PEAKS 7.5 (Bioinformatics Solutions Inc.) was used to examine clusters of significantly different proteins identified in HP-PRRSV HN07-1-infected PAMs at 24 hpi (>1.5-fold, P < 0.05). Ultimately, 269 significantly differentially expressed proteins were identified after HP-PRRSV HN07-1 infection (P < 0.05), of which 46 proteins were significantly up-regulated and 223 proteins were significantly down-regulated (Tables 1, 2). Importantly, for samples tested in triplicate, the heatmap indicated good repeatability (Figure 2). More information can be found in Supplementary Table 7.
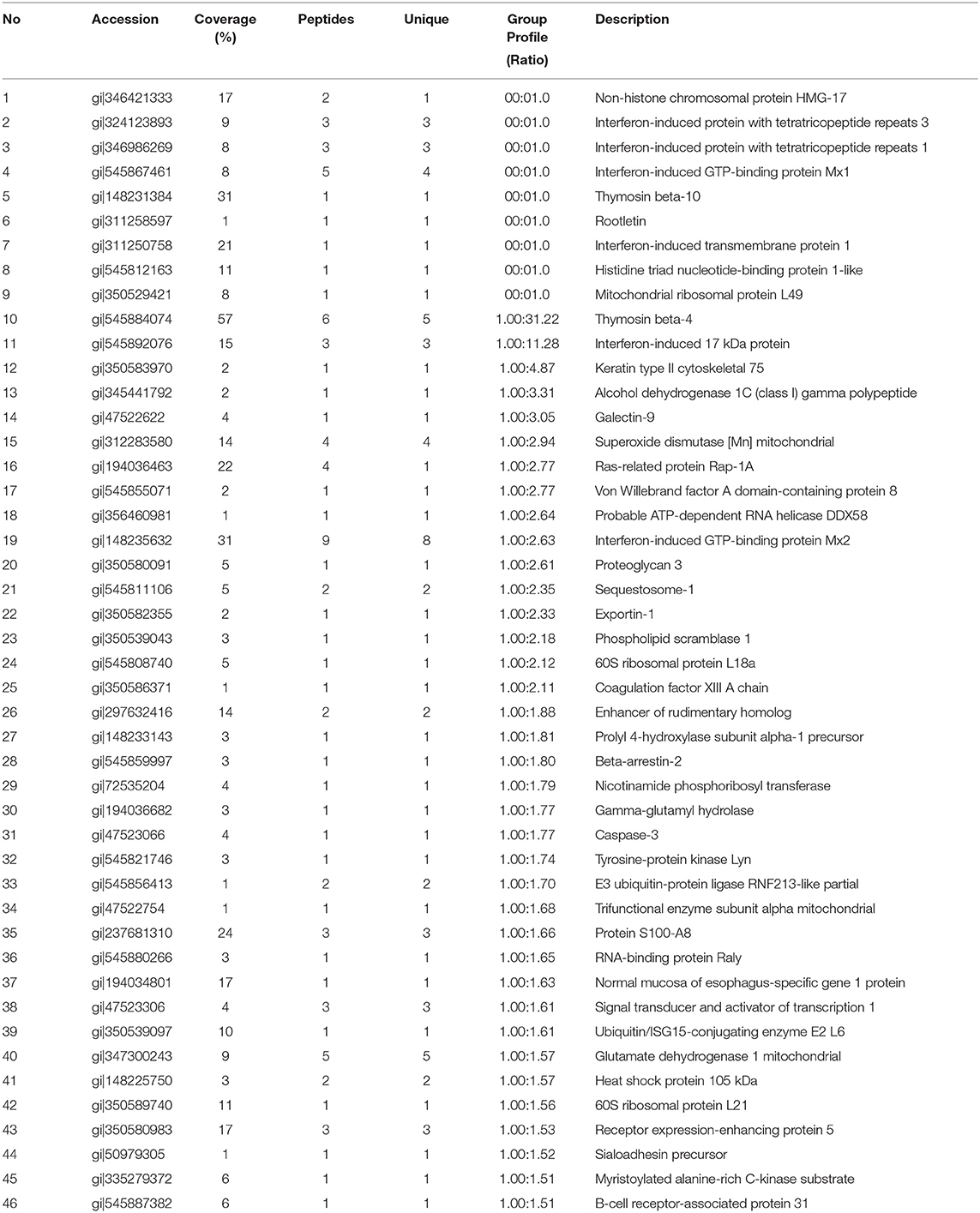
Table 1. The significantly up-regulated proteins in HP-PRRSV HN07-1-infected PAMs identified by LC-MS/MS.

Table 2. The significantly down-regulated proteins in HP-PRRSV HN07-1-infected PAMs identified by LC-MS/MS.
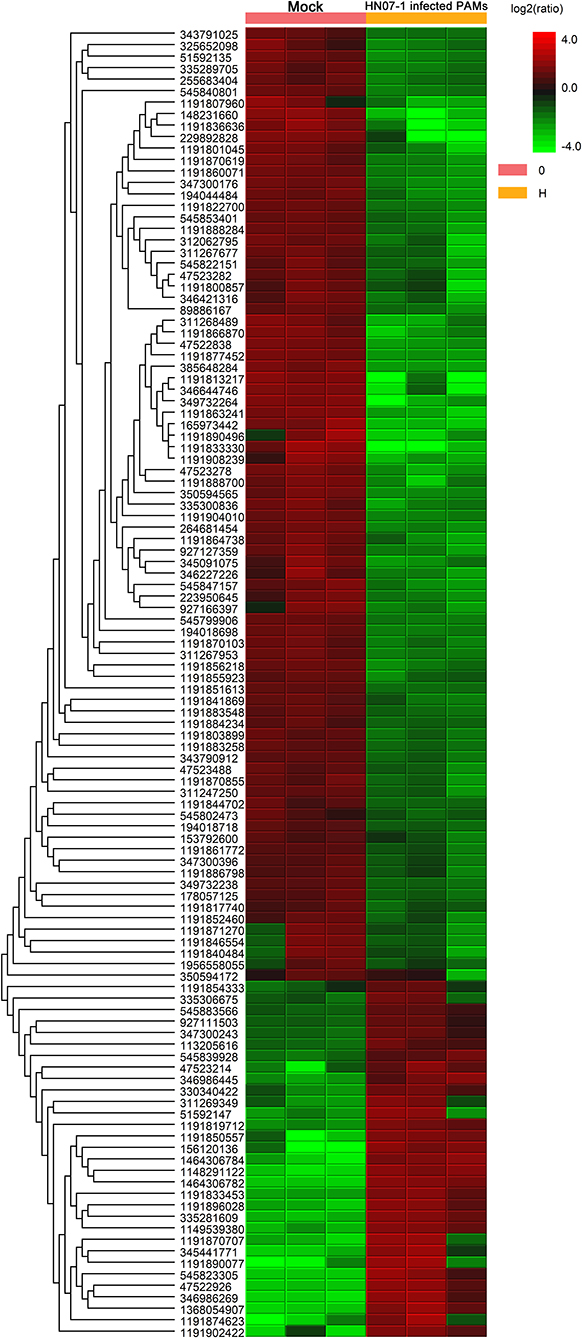
Figure 2. Clustering analysis of significantly differentially expressed proteins in HP-PRRSV HN07-1-infected PAMs. Unsupervised hierarchical clustering of differentially expressed (fold change ≥2 and P < 0.05) proteins in HP-PRRSV HN07-1-infected PAMs. The columns represent mock cells and HP-PRRSV-HN07-1-infected PAMs for three replicate samples, while rows represent different proteins. Up-regulated and down-regulated proteins are indicated by red and green colors, respectively, with color intensity reflecting magnitudes of protein expression level changes, as shown in the legend at the upper right. More information can be found in Supplementary Table 7.
Bioinformatics Analysis Based on ClueGo
ClueGo V2.1.7 was used to generate functionally grouped annotation networks so that we could functionally categorize significantly differentially expressed proteins associated with HP-PRRSV HN07-1 infection. As shown in Figure 3A, up-regulated proteins were mainly associated with functional terms such as response to interferon (IFN)-α (P = 1.03 × 10−5), IFN-β (P = 1.18 × 10−5), positive regulation of interleukin (IL)-8 production (P = 1.34 × 10−4), positive regulation of IL-1β production (P = 4.22 × 10−2), and regulation of Fc receptor-mediated stimulatory signaling pathway (P = 2.38 × 10−7). Down-regulated proteins were mainly associated with functional terms such as cytoplasmic translation (P = 1.78 × 10−04), translation (P = 1.02 × 10−11), translational initiation (P = 1.07 × 10−14), and antigen processing and presentation of exogenous peptide antigen (P = 2.8 × 10−06) (Figure 3B). Additional data are presented in Supplementary Tables 8, 9.
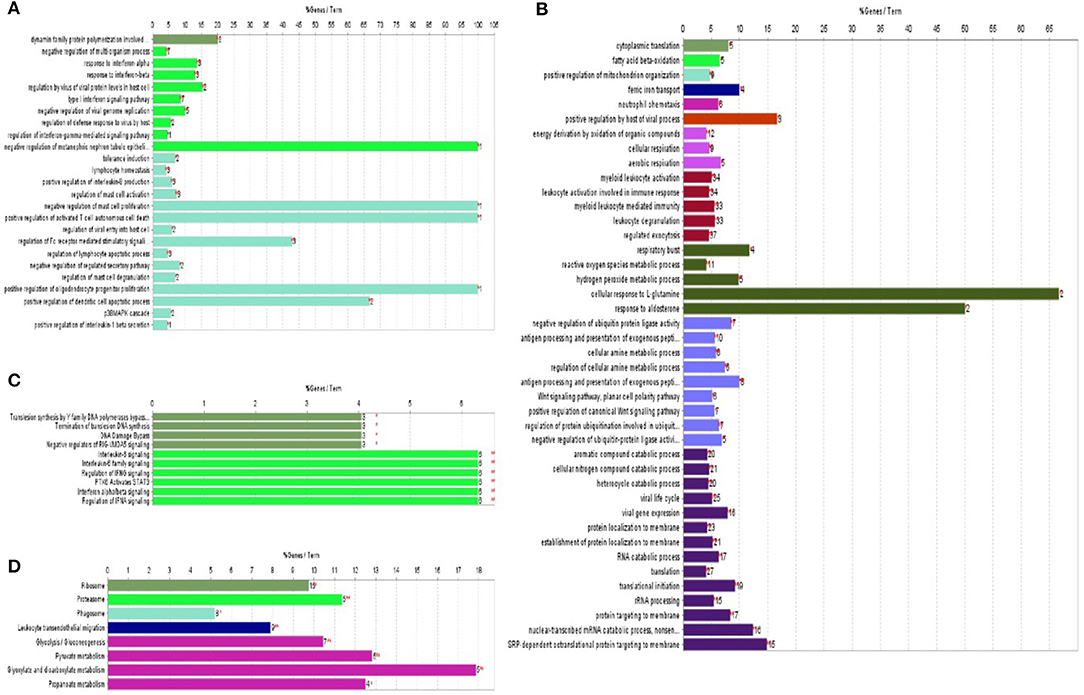
Figure 3. ClueGo was applied to enrich the biological process and KEGG pathways of significantly differentially expressed proteins identified in HP-PRRSV HN07-1-infected PAMs. (A) Biological process enrichment terms of significantly up-regulated proteins. (B) Biological process enrichment terms of significantly down-regulated proteins. (C) KEGG pathway enrichment terms of significantly up-regulated proteins. (D) KEGG pathway enrichment terms of significantly down-regulated proteins. The same color indicates similar functional categories. *P < 0.05, **P < 0.01. More information can be found in Supplementary Tables 8–11.
ClueGo V 2.1.7 was next used to conduct KEGG pathway analysis to explore potential functional networks of differentially expressed proteins. Up-regulated proteins were mainly enriched for KEGG pathway terms such as IL-6 signaling (P = 4.46 × 10−06), regulation of IFN signaling (P = 4.45757089317539 × 10−06), and termination of translesion DNA synthesis (P = 1.8771367 × 10−02) (Figure 3C). Down-regulated proteins were mainly enriched for the KEGG term ribosome (P = 7.5 × 10−08) (Figure 3D). Additional data are shown in Supplementary Tables 10, 11. Taken together, bioinformatic analysis results revealed that after HP-PRRSV HN07-1 infection, host innate immunity-related proteins and pathways were almost all up-regulated, while translation-related proteins and processes were dramatically down-regulated.
PPI Network Analysis
To gain additional insights into possible functional interactions among the identified proteins, PPI networks were constructed using GeneMANIA (23) based on a large set of applicable association data pertaining to protein and genetic interactions (Figure 4). Due to the fact that the pig genome database is under-annotated, gene identifications for identified significantly differentially regulated proteins (listed in Tables 1, 2) were converted to human protein GI numbers. Next, predicted and integrated known PPI data sets from the Homo sapiens genome were input into GeneMANIA. Thereafter, GO categories were enriched for the input dataset using a false discovery rate (FDR)-corrected hypergeometric test.The results were compared to the background set of GO annotations for the entire Homo sapiens genome, with predicted, genetic, and physical interactions enabled during creation of the networks. The top 20 related genes and 20 attributes were displayed using GO biological process-based weighting, then Cytoscape was used to depict the networks. Figure 4A shows the interaction network for up-regulated proteins in HP-PRRSV HN07-1-infected PAMs that highlights interactions between host innate immunity and IFN I-related signaling pathway proteins. Figure 4B shows the interaction network for down-regulated proteins in HP-PRRSV HN07-1-infected PAMs that highlights the importance of interactions involving host proteins related to protein translation.
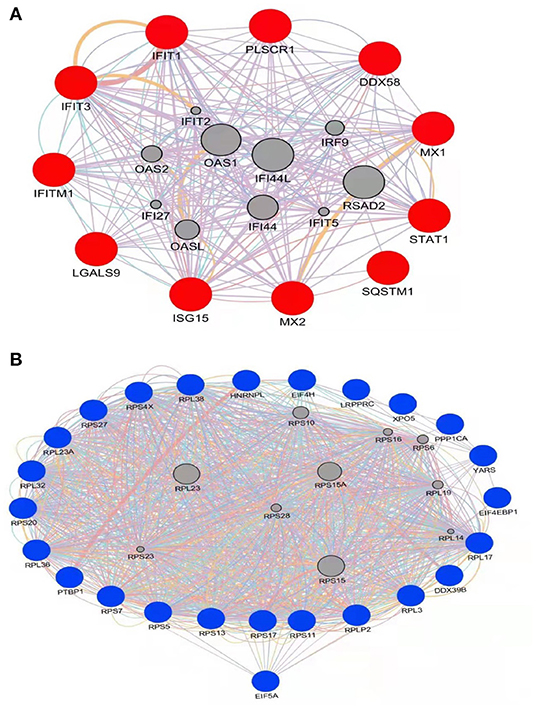
Figure 4. PPI network with significantly differentially expressed proteins in HP-PRRSV infected-PAMs using the GeneMANIA tool. (A) Significantly up-regulated proteins are shown in red; (B) Significantly down-regulated proteins are shown in blue. Edge: interaction between two different proteins.
RT-PCR and WB Analyses of Up-Regulated IFN I-Mediated Signaling Pathways
Notably, proteins in IFN I-mediated signaling pathways were found to be up-regulated in our study. Given the importance of IFN-related proteins in host antiviral responses, we conducted RT-PCR to measure mRNA-level expression of genes that encode IFN-induced proteins, such as retinoic acid-inducible gene I (RIG-I), IFIT1, IFIT3, Mx1, Mx2, STAT1, interferon-stimulated gene 15 (ISG15), and interferon-induced transmembrane protein 1 (IFITM1) in HP-PRRSV HN07-1-infected or mock-infected PAMs. Intriguingly, abundances of mRNAs corresponding to these proteins were found to be significantly increased after HP-PRRSV HN07-1 infection (Figure 5A), with increased levels of Mx1, IFIT3, and STAT1 proteins confirmed by WB analysis (Figure 5B). Importantly, these results aligned with our MS results.
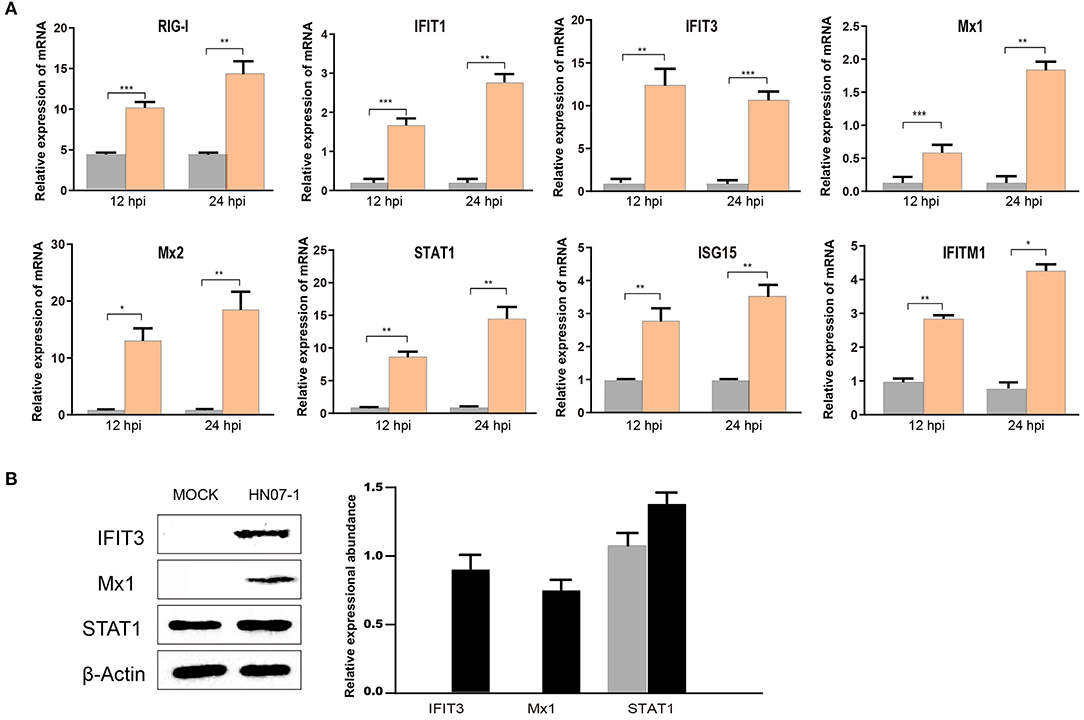
Figure 5. Confirmation of up-regulated proteins using RT-PCR and WB analyses. (A) PAMs were infected with HP-PRRSV HN07-1 at MOI = 0.1 or mock-infected. Samples were collected at 24 hpi for RT-PCR detection for analysis of relative mRNA abundances of RIG-I, IFIT1, IFIT3, Mx1, Mx2, ISG15, STAT1, and IFITM1. (B) PAMs were infected with HP-PRRSV at MOI = 0.1 or mock-infected. Samples were collected at 24 hpi for WB to analyze protein expression levels of STAT1, IFIT3, Mx1, and β-Actin. *P < 0.05; **P < 0.01; ***P < 0.001; ns, not significant.
Decreased EIF5A Abundance After HP-PRRSV HN07-1 Infection
Translation and translational initiation related proteins were found down-regulated in the current study. The protein levels of eIF5A, eIF4E, eIF4E-binding protein 1(4EBP1) and eIF4H in HP-PRRSV HN07-1-infected PAMs at 0, 6, 12 and 24 hpi were determined by WB. The protein levels of eIF5A, 4EBP1, and eIF4H were significantly decreased after 6 hpi, whereas that of eIF4E was not significantly altered (Figure 6A). Considering that eIF5A expression was down-regulated most significantly after PRRSV HN07-1 infection along with the fact that this protein has not been extensively researched in the field of virology, we chose eIF5A as a target for further study and used eIF4E as a control. Next, we tested eIF5A dynamics after PAMs were exposed to UV-inactivated PRRSV, with the results revealing that the eIF5A level remained stable in PAMs treated with UV-inactivated virions (Figure 6B). Interestingly, transcription-level expression of eIF5A in HP-PRRSV HN07-1-infected PAMs remained almost unchanged at all time points (0, 6, 12, and 24 hpi) (Figure 6C).
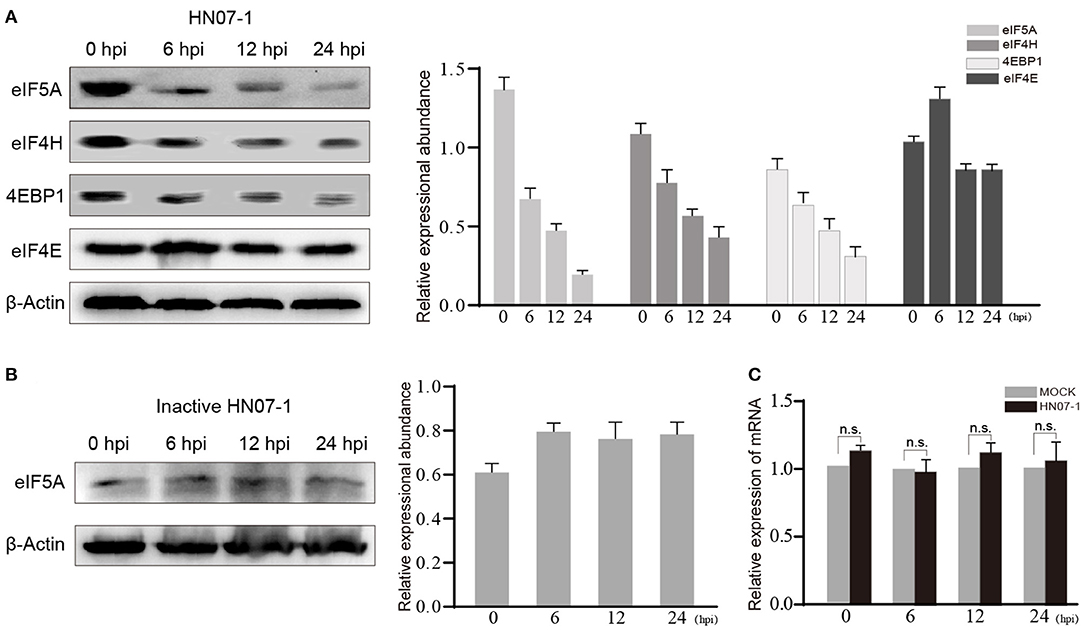
Figure 6. The protein level of eIF5A was decreased after HP-PRRSV HN07-1 infection. (A) PAMs were infected with HP-PRRSV HN07-1 at MOI = 0.1 or mock-infected. The cells were collected at 0, 6, 12, and 24 hpi then total proteins of cells were analyzed via WB to measure protein levels of eIF5A, 4EBP1, eIF4H, and eIF4E. (B) PAMs were treated with UV-inactivated PRRSV then the cells were harvested at 0, 6, 12, and 24 hpi, then total proteins from cells were analyzed via WB to measure protein levels of eIF5A. (C) PAMs were infected with HP-PRRSV HN07-1 at MOI = 0.1 or mock-infected. The cells were collected at 0, 6, 12, and 24 hpi then total RNA preparations from the cells were analyzed via RT-PCR to detect transcription-level expression of eIF5A.
Effect of EIF5A Knockdown on PRRSV Propagation in vitro
We next studied the biological significance of eIF5A on PRRSV infection using the CRL-2843-CD163 cell line and PAMs. CRL-2843-CD163, a cell line that stably expresses CD163, was obtained by transfection of immortalized PAMs (CRL-2843) with DNA encoding the host PRRSV receptor CD163; thus, these cells can be readily infected with PRRSV. As shown in Figure 7A, RT-RCR and WB were carried out to verify effects of eIF4E and eIF5A knockdown in CRL-2843-CD163 cells. At 24 h after transfection, RT-PCR results indicated that transcriptional expression levels of eIF5A and eIF4E were dramatically reduced. WB analysis indicated that expression levels of eIF5A and eIF4E proteins were markedly decreased at 48 h after transfection (Figure 7A).
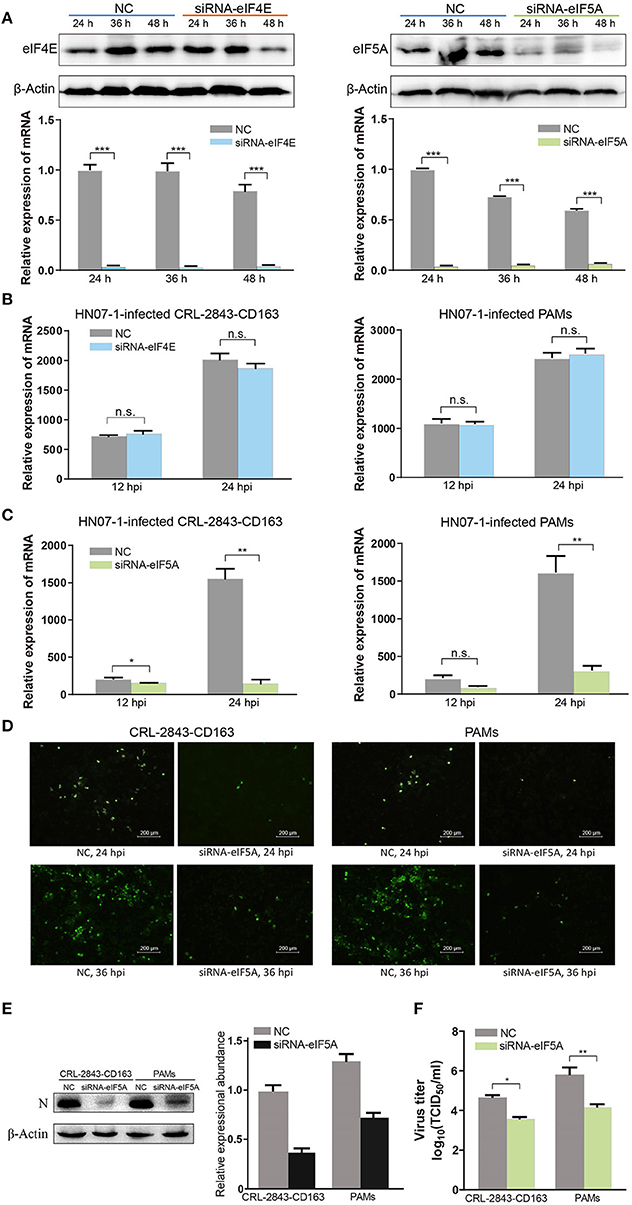
Figure 7. EIF5A is important for PRRSV infection in vitro. (A) SiRNA-eIF4E and siRNA-eIF5A were transfected into CRL-2843-CD163 cells for 24, 36, or 48 h, with NC transfected as the control. The knockdown effect was validated by RT-PCR and WB. (B) The eIF4E knockdown CRL-2843-CD163 cells or PAMs were inoculated with HP-PRRSV HN07-1 (MOI = 0.1) and harvested at 12 and 24 hpi for RT-PCR analysis. (C) The eIF5A knockdown CRL-2843-CD163 cells or PAMs were inoculated with HP-PRRSV HN07-1 (MOI = 0.1) and harvested at 12 and 24 hpi for RT-PCR analysis. (D) HP-PRRSV HN07-1 (MOI = 0.1) was used to inoculate eIF5A knockdown CRL-2843-CD163 cells or PAMs and harvested at 24 hpi for IFA analysis with anti-PRRSV N protein antibody. (E) HP-PRRSV HN07-1 (MOI = 0.1) was used to inoculate eIF5A knockdown CRL-2843-CD163 cells or PAMs then cells were harvested at 24 hpi for WB analysis with anti-PRRSV N protein antibody. (F) HP-PRRSV HN07-1 (MOI = 0.1) was used to inoculate eIF5A knockdown CRL-2843-CD163 cells or PAMs then cells were harvested at 48 hpi. Viral yields were determined based on TCID50 values in MARC-145 cells. Each experiment was carried out three times independently and yielded consistent findings. *P < 0.05; **P < 0.01; ***P < 0.001; ns, not significant.
Next, CRL-2843-CD163 cells and PAMs were transfected with small interfering RNA (siRNA)-eIF4E and siRNA-eIF5A for 48 h, then were infected with HP-PRRSV HN07-1 and harvested at 24 hpi. Thereafter, RT-PCR was applied to verify the effects of transfected siRNAs on HP-PRRSV HN07-1 replication in vitro. The results showed that PRRSV replication in cells with knockdown of eIF4E expression (both CRL-2843-CD163 cells and PAMs) was not significantly different from that of the NC group (Figure 7B). By contrast, viral propagation in cells with knockdown of eIF5A expression was significantly inhibited (Figure 7C). In addition, expression of PRRSV N protein, as measured using IFA, was considerably suppressed in cells that were knockdown of eIF5A expression (both CRL-2843-CD163 cells and PAMs) (Figure 7D). Moreover, WB results revealed that N protein expression was dramatically reduced in cells after eIF5A knockdown (both CRL-2843-CD163 cells and PAMs) (Figure 7E). Furthermore, TCID50 values were much lower in cells after eIF5A knockdown (both CRL-2843-CD163 cells and PAMs) than in the NC group (Figure 7F).
Recombinant EIF5A Rescue of the SiRNA-EIF5A Inhibitory Effect
To further determine whether overexpression of recombinant eIF5A could reverse the eIF5A knockdown-induced inhibitory effect on PRRSV infection, we transfected specific siRNA targeting the eIF5A 3′UTR into CRL-2843-CD163 cells to knock down endogenous eIF5A expression. Next, the cells were transfected with 3*Flag-CMV-eIF5A (Figure 8A) to restore eIF5A expression (as Flag-tagged eIF5A) while inhibiting endogenous eIF5A expression. Thereafter, the modified CRL-2843-CD163 cells were inoculated with HP-PRRSV HN07-1 (MOI = 0.1) then PRRSV propagation was assessed via RT-PCR, IFA, and WB and virus titer determinations. The results revealed that restoration of eIF5A expression in CRL-2843-CD163 cells after eIF5A knockdown rescued PRRSV propagation (Figure 8). Meanwhile, cytotoxicity assays showed that cell viability was not adversely affected by siRNA transfection or restoration of eIF5A expression (Supplementary Figures 1, 2). Taken together, all of these results demonstrated that eIF5A was required for PRRSV propagation in vitro.
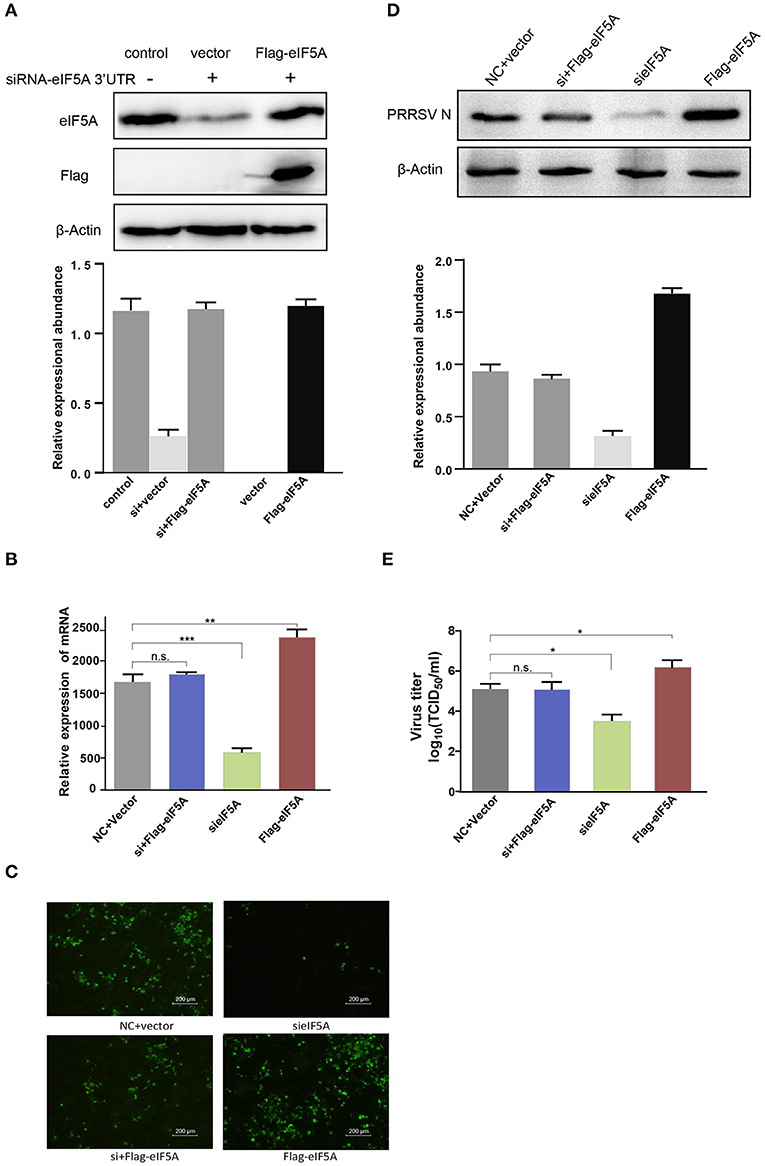
Figure 8. Flag-tagged eIF5A rescued the inhibitory effect of eIF5A knockdown on PRRSV propagation. (A) WB analysis of endogenous eIF5A in CRL-2843-CD163 cells and recombinant Flag-tagged eIF5A in CRL-2843-CD163 cells with eIF5A knockdown. Endogenous eIF5A was knocked down by siRNA targeting of the eIF5A 3′UTR in CRL-2843-CD163 cells. HP-PRRSV HN07-1 (MOI = 0.1) was added to endogenous eIF5A knockdown CRL-2843-CD163 cells with recombinant Flag-tagged eIF5A overexpression. PRRSV propagation was validated by (B) RT-PCR, (C) IFA and (D) WB, (E) Virus titers were also determined. Each experiment was performed three times independently and all had similar results. *P < 0.05; **P < 0.01; ***P < 0.001; ns, not significant.
Discussion
PAMs are known target cells of PRRSV infection. Therefore, it is of great significance to study the interaction between PRRSV and PAMs in order to clarify mechanisms involved in viral infection and propagation. Toward this goal, label-free LC-MS/MS can serve as a powerful, quantitative proteomic method that offers many advantages over traditional proteomic methods, including high sensitivity, high coverage, and high accuracy (27, 28). In our study, the proteome of HP-PRRSV HN07-1-infected PAMs was investigated using label-free LC-MS/MS, with uninfected PAMs serving as the control. Ultimately, a total of 269 differentially expressed proteins were identified, among which 46 proteins were significantly up-regulated and 223 proteins were significantly down-regulated (Tables 1, 2).
Importantly, our results revealed that up-regulated proteins were mainly enriched in IFN-I signaling pathways. RT-PCR further confirmed that specific genes associated with these pathways were significantly up-regulated at 24 hpi, such as RIG-I, IFIT1, IFIT3, Mx1, Mx2, STAT1, ISG15, and IFITM1 (Figure 5A). Moreover, expression of several these proteins (IFIT3, Mx1, and STAT1) was confirmed in HP-PRRSV HN07-1-infected PAMs at 24 hpi by WB analysis (Figure 5B). Taken together, these results indicated that PRRSV infection activated the host innate immune system, a result that was consistent with previously reported results. For example, the expression of cytoplasmic virus sensing receptors RIG-I and melanoma differentiation-associated gene five were found to be significantly increased in PRRSV-infected lungs (29). Furthermore, PRRSV infection of MARC-145 cells had been shown to up-regulate expression of Mx2, which when overexpressed was shown to suppress PRRSV replication. In addition, antiviral activity mediated by IFN-β was found to be reduced when Mx2 expression was knocked down, with Mx2 protein observed to reduce PRRSV replication through its interaction with the viral N protein (30). Importantly, infection of PAMs by PRRSV vaccine strains promoted the secretion of extracellular ISG15 from infected PAMs. This observation prompted researchers to introduce recombinant DNA encoding ISG15 into PAMs, after which PAMs expressed ISG15 then entered an antiviral state whereby PRRSV propagation was blocked (31). IFITM3 overexpression had been shown to inhibit PRRSV replication. Meanwhile, endogenous IFITM3 silencing had been shown to promote PRRSV replication. Additionally, it had also been reported that IFITM3 was S-palmitoylated and ubiquitinated and that both of these posttranslational changes contribute to the anti-PRRSV effect of IFITM3 (32).
In contrast to the results mentioned above for up-regulated proteins, down-regulated proteins were mainly enriched for functional terms related to translation-associated and translational initiation-associated processes. More specifically, levels of eIF5A, eIF5B, eIF1A, eIF4H proteins, and ribosomal proteins 40S and 60S were significantly decreased in PAMs after HP-PRRSV HN07-1-infection (Table 2). These results may be explained the host antiviral defense strategy involved the shutting down of translation-related protein synthesis to restrain virus propagation. Indeed, this concept is supported by results of numerous research studies that have shown that levels of eIFs and other host translation-related proteins were significantly decreased in cells after viral infections. For example, after infection with swine transmissible gastroenteritis virus, results of quantitative proteomic experiments revealed that expression levels of eIF3 protein and ribosomal subunit proteins 40S and 60S were considerably decreased in PK-15 cells (33). As another example, during infection with the extremely pathogenic porcine epidemic diarrhea virus (PEDV), the level of eIF2 protein was drastically decreased in Vero cells (34). As yet another example, the host translation system was shown to be repressed after PRRSV infection, with nsp2 and its transmembrane domain found to be responsible for inducing translation shutdown (35). Furthermore, an investigation of proteomic changes associated with organ infection with the PEDV YN144 strain indicated that expression levels of hnRNPA1 and eIF4G1 proteins were decreased in the PEDV YN144-infected group, suggesting that both proteins might be connected to PEDV YN144 strain pathogenicity (36).
Notably, here protein-level expression of eIF5A in PAMs infected with HP-PRRSV HN07-1 was found to be decreased via WB analysis (Figure 6), indicating that this protein might play a role in PRRSV propagation. Meanwhile, after HP-PRRSV HN07-1 infection, eIF5A protein-level expression was down-regulated in PAMs but not in PAMs exposed to UV-inactivated virions, prompting us to speculate that down-regulation of eIF5A expression in PAMs after PRRSV infection was caused by viral replication rather than viral invasion. Interestingly, transcription-level expression of eIF5A was not altered in HP-PRRSV HN07-1-infected PAMs at 0, 6, 12, and 24 hpi even though the eIF5A protein level was decreased in HP-PRRSV HN07-1-infected PAMs, warranting further study.
EIFs are important eukaryotic protein translation proteins. EIF2 inhibits protein translation, reduces the levels of early stress proteins and misfolded proteins that are produced in the endoplasmic reticulum (ER), and relieves ER stress (37). Meanwhile, eIF3 mediates ribosome binding to specific RNAs during formation of the translation initiation complex (38), while eIF4E binds to the 5′ methylated cap structure of eukaryotic mRNA and participates in the formation of the translation initiation complex (39). In fact, in recent years results of several studies have shown that eIFs are closely associated with viral replication, including results reported by Regina Cencic et al. showing that blocking the functional link between eIF4E and eIF4G greatly decreased replication of human coronavirus (40). In other studies, viral suppression of replication was observed after silencing of eIF4G1 protein expression during infections with vesicular stomatitis virus and influenza virus (41, 42).
EIF5A, which is also known as eIF4D, was first isolated from immature red blood cells (43). It is an acidic protein with a molecular mass of 17–21 kDa that is fairly well-conserved from yeast to humans (44). The function of eIF5A during translation has been widely studied in recent years, with results of studies showing that eIF5A binds to a region of the ribosome that is associated with its translation function, where it acts to promotes the elongation of numerous non polyproline-specific tripeptide sequences (45, 46). EIF5A also binds to 3′-terminal polyadenylation tails of eukaryotic mRNAs and plays a vital role in termination of translation (47). Another critical function of eIF5A is to mediate nucleocytoplasmic transport of mRNA and ensure the balanced distribution of mRNA in the cell nucleus and cytoplasm (48).
In a previous study, eIF5A was observed to play a pivotal role in human immunodeficiency virus (HIV) replication (49). In addition, Ruhl et al. reported that eIF5A participated in HIV replication in combination with HIV assistant factor regulator of expression of Rev protein (50). Subsequently, Hofmann et al. discovered that Rev protein shuttles back and forth between the nucleus and cytoplasm of host cells as part of its primary function, whereby it controls nuclear transport of non-spliced and incompletely spliced viral mRNAs as an eIF5A-dependent process (51). Meanwhile, mutation of eIF5A has been shown to significantly suppress mRNA nuclear export and inhibit HIV replication in vitro without affecting cell propagation and metabolic activity (52). In addition, expression of eIF5A was found to be down-regulated after Fe overload, while expression of the NEF protein of HIV was considerably down-regulated and HIV replication was reduced in vitro when eIF5A expression was reduced due to shRNA effects (53). Taken together, these results show that eIF5A plays a role in HIV replication, while eIF5A effects on PRRSV replication have not yet been reported.
Replication of the PRRSV RNA genome is a multi-step process involving the assembly of replication and transcription complexes that consist of viral and cell-derived components (54–56). In this study, we first investigated the role of eIF5A in PRRSV replication in vitro. Notably, PRRSV propagation was significantly inhibited after knockdown of eIF5A expression in CRL-2843-CD163 cells and PAMs even though eIF4E knockdown did not affect viral propagation (Figure 7). However, suppression of HP-PRRSV infection after eIF5A knockdown could be reversed by restoration of host cell expression of eIF5A (Figure 8). Although these results are intriguing, they raise additional questions regarding the mechanisms underlying eIF5A involvement in PRRSV propagation. Thus, experiments are currently underway in our laboratory to answer these questions toward the development of novel anti-viral strategies and more effective anti-viral drugs to combat PRRSV.
Conclusion
In summary, here dynamic changes in the proteome of HP-PRRSV HN07-1-infected PAMs were analyzed using label-free LC-MS/MS techniques, resulting in identification of a total of 269 significantly differentially expressed host proteins. Interestingly, expression of one of these proteins, eIF5A, was down-regulated in PAMs after HP-PRRSV HN07-1 infection, while PRRSV replication was significantly inhibited and the viral titer was suppressed considerably by eIF5A knockdown in vitro. Taken together, these results demonstrated that eIF5A participates in PRRSV infection and created a foundation for further exploration of mechanisms toward the development of antiviral strategies to control and prevent PRRSV infection.
Data Availability Statement
The datasets presented in this study can be found in online repositories. The names of the repository/repositories and accession number(s) can be found in the article/Supplementary Material.
Ethics Statement
The animal study was reviewed and approved by The Ethical and Animal Welfare Committee of the Key Laboratory of Animal Immunology of the Ministry of Agriculture of China.
Author Contributions
HL, RL, and SQ designed the experiments. HL, BW, DJ, PJ, MZ, and XL performed the experiments and analyzed the data. HL wrote the paper. RL and SQ revised the article, and all authors approved the final manuscript.
Funding
This study was supported by grants from National Natural Science Foundation of China (31902284, 31902279), Funding scheme for young teachers in colleges and universities in Henan province (2020GGJS258), Doctoral Research Initiation Fund of Henan University of Animal Husbandry and Economy (2019HNUAHEDF040).
Conflict of Interest
The authors declare that the research was conducted in the absence of any commercial or financial relationships that could be construed as a potential conflict of interest.
Publisher's Note
All claims expressed in this article are solely those of the authors and do not necessarily represent those of their affiliated organizations, or those of the publisher, the editors and the reviewers. Any product that may be evaluated in this article, or claim that may be made by its manufacturer, is not guaranteed or endorsed by the publisher.
Supplementary Material
The Supplementary Material for this article can be found online at: https://www.frontiersin.org/articles/10.3389/fvets.2022.861137/full#supplementary-material
References
1. Rossow KD. Porcine reproductive and respiratory syndrome. Vet Pathol. (1998) 35:1–20. doi: 10.1177/030098589803500101
2. Neumann EJ, Kliebenstein JB, Johnson CD, Mabry JW, Bush EJ, Seitzinger AH, et al. Assessment of the economic impact of porcine reproductive and respiratory syndrome on swine production in the United States. J Am Vet Med Assoc. (2005) 227:385–92. doi: 10.2460/javma.2005.227.385
3. Tian K, Yu X, Zhao T, Feng Y, Cao Z, Wang C, et al. Emergence of fatal PRRSV variants: unparalleled outbreaks of atypical PRRS in China and molecular dissection of the unique hallmark. PLoS ONE. (2007) 2:e526. doi: 10.1371/journal.pone.0000526
4. Tong GZ, Zhou YJ, Hao XF, Tian ZJ, An TQ, Qiu HJ. Highly pathogenic porcine reproductive and respiratory syndrome, China. Emerg Infect Dis. (2007) 13:1434–6. doi: 10.3201/eid1309.070399
5. Lefkowitz EJ, Dempsey DM, Hendrickson RC, Orton RJ, Siddell SG, Smith DB. Virus taxonomy: the database of the international committee on taxonomy of viruses (ICTV). Nucleic Acids Res. (2018) 46:D708–17. doi: 10.1093/nar/gkx932
6. Wu C, Gu G, Zhai T, Wang Y, Yang Y, Li Y, et al. Broad neutralization activity against both PRRSV-1 and PRRSV-2 and enhancement of cell mediated immunity against PRRSV by a novel IgM monoclonal antibody. Antiviral Res. (2020) 175:104716. doi: 10.1016/j.antiviral.2020.104716
7. Duan X, Nauwynck HJ, Pensaert MB. Virus quantification and identification of cellular targets in the lungs and lymphoid tissues of pigs at different time intervals after inoculation with porcine reproductive and respiratory syndrome virus (PRRSV). Vet Microbiol. (1997) 56:9–19. doi: 10.1016/S0378-1135(96)01347-8
8. Emmott E, Wise H, Loucaides EM, Matthews DA, Digard P, Hiscox JA. Quantitative proteomics using SILAC coupled to LC-MS/MS reveals changes in the nucleolar proteome in influenza a virus-infected cells. J Proteome Res. (2010) 9:5335–45. doi: 10.1021/pr100593g
9. Munday DC, Hiscox JA, Barr JN. Quantitative proteomic analysis of A549 cells infected with human respiratory syncytial virus subgroup B using SILAC coupled to LC-MS/MS. Proteomics. (2010) 10:4320–34. doi: 10.1002/pmic.201000228
10. Sokolowska I, Dorobantu C, Woods AG, Macovei A, Branza-Nichita N, Darie CC. Proteomic analysis of plasma membranes isolated from undifferentiated and differentiated HepaRG cells. Proteome Sci. (2012) 10:47. doi: 10.1186/1477-5956-10-47
11. Fan H, Ye Y, Luo Y, Tong T, Yan G, Liao M. Quantitative proteomics using stable isotope labeling with amino acids in cell culture reveals protein and pathway regulation in porcine circovirus type 2 infected PK-15 cells. J Proteome Res. (2012) 11:995–1008. doi: 10.1021/pr200755d
12. Ye Y, Yan G, Luo Y, Tong T, Liu X, Xin C, et al. Quantitative proteomics by amino acid labeling in foot-and-mouth disease virus (FMDV)-infected cells. J Proteome Res. (2013) 12:363–77. doi: 10.1021/pr300611e
13. Zheng X, Hong L, Shi L, Guo J, Sun Z, Zhou J. Proteomics analysis of host cells infected with infectious bursal disease virus. Mol Cell Proteomics. (2008) 7:612–25. doi: 10.1074/mcp.M700396-MCP200
14. Alfonso P, Rivera J, Hernaez B, Alonso C, Escribano JM. Identification of cellular proteins modified in response to African swine fever virus infection by proteomics. Proteomics. (2004) 4:2037–46. doi: 10.1002/pmic.200300742
15. Ai Q, Lin X, Xie H, Li B, Liao M, Fan H. Proteome analysis in PAM cells reveals that african swine fever virus can regulate the level of intracellular polyamines to facilitate its own replication through ARG1. Viruses. (2021) 13:1236. doi: 10.3390/v13071236
16. Fang J, Qiao S, Wang K, Li R, Wang L, Li H, Zhang G. Quantitative Proteomic Analysis of Global Protein Acetylation in PRRSV-Infected Pulmonary Alveolar Macrophages. Proteomics. (2021) 21:e2000019. doi: 10.390/v13071236
17. Zhang H, Fang L, Zhu X, Wang D, Xiao S. Global analysis of ubiquitome in PRRSV-infected pulmonary alveolar macrophages. J Proteomics. (2018) 184:16–24. doi: 10.1016/j.jprot.2018.06.010
18. Li Y, Wu Z, Liu K, Qi P, Xu J, Wei J, et al. Proteomic analysis of the secretome of porcine alveolar macrophages infected with porcine reproductive and respiratory syndrome virus. Proteomics. (2017) 17:80. doi: 10.1002/pmic.201700080
19. Qiao S, Feng L, Bao D, Guo J, Wan B, Xiao Z, et al. Porcine reproductive and respiratory syndrome virus and bacterial endotoxin act in synergy to amplify the inflammatory response of infected macrophages. Vet Microbiol. (2011) 149:213–20. doi: 10.1016/j.vetmic.2010.11.006
20. Bradford MM. A rapid and sensitive method for the quantitation of microgram quantities of protein utilizing the principle of protein-dye binding. Anal Biochem. (1976) 72:248–54. doi: 10.1006/abio.1976.9999
21. Zhang J, Xin L, Shan B, Chen W, Xie M, Yuen D, et al. PEAKS DB: de novo sequencing assisted database search for sensitive and accurate peptide identification. Mol Cell Proteomics. (2012) 11:M111010587. doi: 10.1074/mcp.M111.010587
22. Bindea G, Mlecnik B, Hackl H, Charoentong P, Tosolini M, Kirilovsky A, et al. ClueGO: a Cytoscape plug-in to decipher functionally grouped gene ontology and pathway annotation networks. Bioinformatics. (2009) 25:1091–3. doi: 10.1093/bioinformatics/btp101
23. Warde-Farley D, Donaldson SL, Comes O, Zuberi K, Badrawi R, Chao P, et al. The GeneMANIA prediction server: biological network integration for gene prioritization and predicting gene function. Nucleic Acids Res. (2010) 38:W214–220. doi: 10.1093/nar/gkq537
24. Liu Y, Lu N, Yuan B, Weng L, Wang F, Liu Y J, Zhang Z. The interaction between the helicase DHX33 and IPS-1 as a novel pathway to sense double-stranded RNA and RNA viruses in myeloid dendritic cells. Cell Mol Immunol. (2014) 11:49–57. doi: 10.1038/cmi.2013.40
25. Nadgir SV, Hensler HR, Knowlton ER, Rinaldo CR, Rappocciolo G, Jenkins FJ. Fifty percent tissue culture infective dose assay for determining the titer of infectious human herpesvirus 8. J Clin Microbiol. (2013) 51:1931–4. doi: 10.1128/JCM.00761-13
26. Ma J, Chen T, Wu S, Yang C, Bai M, Shu K, et al. iProX: an integrated proteome resource. Nucleic Acids Res. (2019) 47:D1211–7. doi: 10.1093/nar/gky869
27. Wang H, Alvarez S, Hicks LM. Comprehensive comparison of iTRAQ and label-free LC-based quantitative proteomics approaches using two Chlamydomonas reinhardtii strains of interest for biofuels engineering. J Proteome Res. (2012) 11:487–501. doi: 10.1021/pr2008225
28. Trinh HV, Grossmann J, Gehrig P, Roschitzki B, Schlapbach R, Greber UF, et al. iTRAQ-based and label-free proteomics approaches for studies of human adenovirus infections. Int J Proteomics. (2013) 2013:581862. doi: 10.1155/2013/581862
29. Xiao S, Mo D, Wang Q, Jia J, Qin L, Yu X, et al. Aberrant host immune response induced by highly virulent PRRSV identified by digital gene expression tag profiling. BMC Genomics. (2010) 11:544. doi: 10.1186/1471-2164-11-544
30. Wang H, Bai J, Fan B, Li Y, Zhang Qand Jiang P. The interferon-Induced Mx2 inhibits porcine reproductive and respiratory syndrome virus replication. J Interferon Cytokine Res. (2016) 36:129–39. doi: 10.1089/jir.2015.0077
31. Liu H, Shi B, Zhang Z, Zhao B, Zhao G, Li Y, et al. PRRSV vaccine strain-induced secretion of extracellular ISG15 stimulates porcine alveolar macrophage antiviral response against PRRSV. Viruses. (2020) 12:1009. doi: 10.3390/v12091009
32. Zhang A, Duan H, Zhao H, Liao H, Du Y, Li L, et al. Interferon-induced transmembrane protein 3 is a virus-associated protein which suppresses porcine reproductive and respiratory syndrome virus replication by blocking viral membrane fusion. J Virol. (2020) 94:20. doi: 10.1128/JVI.01350-20
33. An K, Fang L, Luo R, Wang D, Xie L, Yang J, et al. Quantitative proteomic analysis reveals that transmissible gastroenteritis virus activates the JAK-STAT1 signaling pathway. J Proteome Res. (2014) 13:5376–90. doi: 10.1021/pr500173p
34. Guo X, Hu H, Chen F, Li Z, Ye S, Cheng S, et al. iTRAQ-based comparative proteomic analysis of Vero cells infected with virulent and CV777 vaccine strain-like strains of porcine epidemic diarrhea virus. J Proteomics. (2016) 130:65–75. doi: 10.1016/j.jprot.2015.09.002
35. Li Y, Fang L, Zhou Y, Tao R, Wang D, Xiao S. Porcine reproductive and respiratory syndrome virus infection induces both eIF2alpha phosphorylation-dependent and -independent host translation shutoff. J Virol. (2018) 92:18. doi: 10.1128/JVI.00600-18
36. Li Z, Chen F, Ye S, Guo X, Muhanmmad Memon A, Wu M, et al. comparative proteome analysis of porcine jejunum tissues in response to a virulent strain of porcine epidemic diarrhea virus and its attenuated strain. Viruses. (2016) 8:2032. doi: 10.3390/v8120323
37. Teske BF, Wek SA, Bunpo P, Cundiff JK, McClintick JN, Anthony TG, et al. The eIF2 kinase PERK and the integrated stress response facilitate activation of ATF6 during endoplasmic reticulum stress. Mol Biol Cell. (2011) 22:4390–405. doi: 10.1091/mbc.E11-06-0510
38. Sadato D, Ono T, Gotoh-Saito S, Kajiwara N, Nomura N, Ukaji M, et al. Eukaryotic translation initiation factor 3 (eIF3) subunit e is essential for embryonic development and cell proliferation. FEBS Open Bio. (2018) 8:1188–201. doi: 10.1002/2211-5463.12482
39. Mishra RK, Datey A, Hussain T. mRNA Recruiting eIF4 factors involved in protein synthesis and its regulation. Biochemistry. (2020) 59:34–46. doi: 10.1021/acs.biochem.9b00788
40. Cencic R, Desforges M, Hall DR, Kozakov D, Du Y, Min J, et al. Blocking eIF4E-eIF4G interaction as a strategy to impair coronavirus replication. J Virol. (2011) 85:6381–9. doi: 10.1128/JVI.00078-11
41. Welnowska E, Castello A, Moral P, Carrasco L. Translation of mRNAs from vesicular stomatitis virus and vaccinia virus is differentially blocked in cells with depletion of eIF4GI and/or eIF4GII. J Mol Biol. (2009) 394:506–21. doi: 10.1016/j.jmb.2009.09.036
42. Yanguez E, Castello A, Welnowska E, Carrasco L, Goodfellow I, Nieto A. Functional impairment of eIF4A and eIF4G factors correlates with inhibition of influenza virus mRNA translation. Virology. (2011) 413:93–102. doi: 10.1016/j.virol.2011.02.012
43. Smit-McBride Z, Schnier J, Kaufman RJ, Hershey JW. Protein synthesis initiation factor eIF-4D. Functional comparison of native and unhypusinated forms of the protein. J Biol Chem. (1989) 264:18527–30.
44. Gordon ED, Mora R, Meredith SC, Lee C, Lindquist SL. Eukaryotic initiation factor 4D, the hypusine-containing protein, is conserved among eukaryotes. J Biol Chem. (1987) 262:16585–9.
45. Pelechano V, Alepuz P. eIF5A facilitates translation termination globally and promotes the elongation of many non polyproline-specific tripeptide sequences. Nucleic Acids Res. (2017) 45:7326–38. doi: 10.1093/nar/gkx479
46. Abe T, Nagai R, Shimazaki S, Kondo S, Nishimura S, Sakaguchi Y, et al. In vitro yeast reconstituted translation system reveals function of eIF5A for synthesis of long polypeptide. J Biochem. (2020) 167:451–62. doi: 10.1093/jb/mvaa022
47. Henderson A, Hershey JW. The role of eIF5A in protein synthesis. Cell Cycle. (2011) 10:3617–8. doi: 10.4161/cc.10.21.17850
48. Meneguello L, Barbosa NM, Pereira KD, Proenca ARG, Tamborlin L, Simabuco FM, et al. The polyproline-motif of S6K2: eIF5A translational dependence and importance for protein-protein interactions. J Cell Biochem. (2019) 120:6015–25. doi: 10.1002/jcb.27888
49. Kaiser A. Translational control of eIF5A in various diseases. Amino Acids. (2012) 42:679–84. doi: 10.1007/s00726-011-1042-8
50. Ruhl M, Himmelspach M, Bahr GM, Hammerschmid F, Jaksche H, Wolff B, et al. Eukaryotic initiation factor 5A is a cellular target of the human immunodeficiency virus type 1 Rev activation domain mediating trans-activation. J Cell Biol. (1993) 123:1309–20.
51. Hofmann W, Reichart B, Ewald A, Muller E, Schmitt I, Stauber RH, et al. Cofactor requirements for nuclear export of Rev response element (RRE)- and constitutive transport element (CTE)-containing retroviral RNAs. An unexpected role for actin. J Cell Biol. (2001) 152:895–910. doi: 10.1083/jcb.152.5.895
52. Bevec D, Jaksche H, Oft M, Wohl T, Himmelspach M, Pacher A, et al. Inhibition of HIV-1 replication in lymphocytes by mutants of the Rev cofactor eIF-5A. Science. (1996) 271:1858–60. doi: 10.1126/science.271.5257.1858
53. Mancone C, Grimaldi A, Refolo G, Abbate I, Rozera G, Benelli D, et al. Iron overload down-regulates the expression of the HIV-1 Rev cofactor eIF5A in infected T lymphocytes. Proteome Sci. (2017) 15:18. doi: 10.1186/s12953-017-0126-0
54. van der Meer Y, van Tol H, Locker JK, Snijder EJ. ORF1a-encoded replicase subunits are involved in the membrane association of the arterivirus replication complex. J Virol. (1998) 72:6689–98. doi: 10.1128/JVI.72.8.6689-6698.1998
55. Pedersen KW, van der Meer Y, Roos N, Snijder EJ. Open reading frame 1a-encoded subunits of the arterivirus replicase induce endoplasmic reticulum-derived double-membrane vesicles which carry the viral replication complex. J Virol. (1999) 73:2016–26. doi: 10.1128/JVI.73.3.2016-2026.1999
Keywords: proteome, eIF5A, PRRSV, infection, PAMs
Citation: Li H, Wan B, Jiang D, Ji P, Zhao M, Li X, Li R and Qiao S (2022) Proteomic Investigation Reveals Eukaryotic Translation Initiation Factor 5A Involvement in Porcine Reproductive and Respiratory Syndrome Virus Infection in vitro. Front. Vet. Sci. 9:861137. doi: 10.3389/fvets.2022.861137
Received: 24 January 2022; Accepted: 18 March 2022;
Published: 13 April 2022.
Edited by:
Yulong Gao, Harbin Veterinary Research Institute (CAAS), ChinaReviewed by:
Dang Wang, Huazhong Agricultural University, ChinaKun Zhang, Virginia Commonwealth University, United States
Copyright © 2022 Li, Wan, Jiang, Ji, Zhao, Li, Li and Qiao. This is an open-access article distributed under the terms of the Creative Commons Attribution License (CC BY). The use, distribution or reproduction in other forums is permitted, provided the original author(s) and the copyright owner(s) are credited and that the original publication in this journal is cited, in accordance with accepted academic practice. No use, distribution or reproduction is permitted which does not comply with these terms.
*Correspondence: Rui Li, bGlydWk4NjA2MjBAc2luYS5jb20=; Songlin Qiao, Y2RqNTY1QGdtYWlsLmNvbQ==