- 1Quality and Authentication of Agricultural Products Unit, Knowledge and Valorization of Agricultural Products Department, Walloon Agricultural Research Centre, Gembloux, Belgium
- 2Haute Ecole Louvain-en-Hainaut, Montignies-sur-Sambre, Belgium
- 3Functional and Evolutionary Entomology, Gembloux Agro-Bio Tech, ULiège, Gembloux, Belgium
- 4Eurofins Biologie Moléculaire France, Eurofins, Nantes, France
- 5Biological Engineering Unit, Life Sciences Department, Walloon Agricultural Research Centre, Gembloux, Belgium
Use of edible insects as an alternative source of proteins in food and feed is increasing. These last years, numerous companies in Europe have started producing insects for food and feed purposes. In the European Union, the use of edible insects for human consumption falls within Regulation (EU) No. 2015/2283 on novel foods. For feed, Commission Regulation (EU) 2017/893 authorizes seven insect species as processed animal proteins for aquaculture. Methods of authentication are required to check the conformity of the products. In this study, we propose a real-time polymerase chain reaction (PCR) method for the specific detection of the lesser mealworm (Alphitobius diaperinus), one of the species included in the shortlist of authorized insects. The selected target is the cadherin gene with a single-copy (per haploid genome) illustrated by our experimental evidence. The PCR test amplified a 134-bp fragment of the cadherin gene. The qualitative method was assessed toward several performance criteria. Specificity was checked against 54 insect species next to other animal and plant species. The sensitivity, efficiency, robustness, and transferability of the PCR assay were also successfully tested. Finally, the applicability of the test was assessed on real-life processed samples (industrial meals) of A. diaperinus. The study also showed that there seems to be a huge confusion on the correct labeling of the marketed mealworms. We did not succeed to get Alphitobius laevigatus samples. They all appeared to belong to the A. diaperinus taxon.
Introduction
In recent years, edible insects are becoming an increasing alternative source of proteins. The main reason for it is that the food production rate is expected to be lower than the population growth (1). However, the insect consumption in European countries is limited because of strong impeding barriers associated with texture and appearance, much more than the taste (2, 3). Therefore, the incorporation of insects as ingredients in common food items such as sausages, protein bars, pâté, buns, and pastas is a way to overcome this problem (3).
Insects represent a promising strategy for enriching food in some nutrients, thereby achieving a better nutritional balance (4). In fact, the positive nutritional features of edible insects are the presence of high quantities of proteins, essential and non-essential amino acids, lipids, fibers, vitamins, and minerals (4, 5). Roncolini et al. (4) conducted a study to evaluate the use of lesser mealworm powder to replace a part of wheat flour as a means to enhance the protein and mineral content of crunchy snacks. The technological, microbiological, nutritional, and sensory characteristics of the fortified rusks were also evaluated in their study. A publication of Lacroix et al. (6) suggested the potential of lesser mealworm protein hydrolysates to serve as functional food ingredients to help improve glycemic regulation. Many insect species have been shown to have a high concentration not only of iron but also of zinc (3, 7). The rearing substrate can then modulate the insect nutritional quality (3). Insect growth rates, production efficiencies, and protein quality are influenced by substrates used to feed the insects but also depend on entomological species raised and rearing conditions (8, 9).
In animal production, the main protein source comes from either soybean (to feed cattle) or from fishmeal (to feed fish). However, soybean is associated with deforestation, use of genetically modified plant breeds, and massive use of pesticides, whereas industrial fishing to produce fishmeal depletes fish stocks (10). Insects are an ideal feed alternative because they are a good source of nutrients. Compared with conventional livestock, insects require less space for production (11), are expected to use less water (12), and emit less greenhouse gases (13), making them a more sustainable source of animal proteins (8).
Recently, seven insect species were authorized as processed animal proteins in aquafeed (14) and in pig and poultry feed (15): black soldier fly (Hermetia illucens L.), yellow mealworm (Tenebrio molitor L.), lesser mealworm (Alphitobius diaperinus), house cricket (Acheta domesticus L.), banded cricket [Gryllodes sigillatus (Walker)], field cricket (Gryllus assimilis F.), and common house fly (Musca domestica L.). Among these species, A. diaperinus can easily be reared to obtain protein flour for human consumption, as well as for feed material (16). In the meantime, the list of authorized insects as processed animal proteins was extended to silkworm (17).
Human consumption of edible insects in the European Union falls within Regulation (EU) No. 2015/2283 on novel foods (18). This means that food containing insects and their derived products must be subject to authorization once European Food Safety Authority (EFSA) has performed the risk assessments on their safety (4). In 2015, EFSA published a list of insect species potentially usable as food (19). Lesser mealworm (A. diaperinus) is included in this list. T. molitor larvae and A. domesticus are now assessed as safe novel food by EFSA (20–22), and dried larvae of the yellow mealworm are already authorized for placing on the market (23) with appropriate labeling of the food in which it is contained.
A. diaperinus is therefore used as feed for rearing animals and might be used for human consumption, once EFSA has given its approval for it as a safe novel food. A second species of the Alphitobius genus, Alphitobius laevigatus F., not listed by EFSA, is also marketed but it is only intended to feed house pets, including reptiles and amphibians.
Methods are required to check the conformity of these novel products. These last years, different methods of insect detection were developed. Mass spectrometry approaches were proposed for the detection of T. molitor and G. assimilis (24), H. illucens (25), A. domesticus, T. molitor, Locusta migratoria L., and A. diaperinus (25, 26), as well as the Drosophila genus (Fallén) (27). Mass spectrometry may also help to differentiate insect meals according to their taxonomic groups (28). However, an accurate identification by proteomic methods will only be achievable after more intensive sequencing efforts, given the obvious lack of proteomic data for insect species (24). An adapted sedimentation protocol concentrating the insect particles for their detection in feed by light microscopy was proposed by Veys and Baeten (29).
At present, real-time polymerase chain reaction (PCR) remains the reference technique for DNA detection in food or feed products (30–34). Therefore, detection methods by real-time PCR were also proposed for insects. Most PCR tests published were developed on mitochondrial DNA. This facilitates detection in processed food and feed in which the DNA may be degraded, as a mitochondrion contains several copies of its genome, and several mitochondria can be present in a single cell (35). This is applied for H. illucens detection in feed (36, 37), Oxya chinensis (Thunberg) in food (38), Bombyx mori L. in food (1) and feed (39), and G. sigillatus in food and feed (40). However, this multicopy characteristic is a disadvantage for quantitation purposes as the copy number per cell will be variable, depending on the considered tissue (36). This is why other publications focused on single-copy gene tests such as the real-time PCR test for T. molitor detection in food and feed (41) and B. mori in feed (39). In this study, we chose to focus on a chromosomal target that is a single copy per haploid genome.
This study proposes the first real-time PCR method for specific detection of lesser mealworm (A. diaperinus). The target used is the cadherin gene, which for A. diaperinus was characterized by Hua et al. (42). Its equivalent in T. molitor was already used as a target for the development of a real-time PCR test (41).
Materials and Methods
Samples
Insects were either collected in natural environment by trained entomologists, purchased from specialized companies, or provided by the Functional and Evolutionary Entomology laboratory of Gembloux Agro-Bio Tech (ULiège, Gembloux, Belgium). Insects were selected in order to cover several taxonomic groups or to have close relatives with a practical interest to the species considered. All insects were dead at the arrival at the laboratory. DNA extraction was mostly performed on a single individual, except for smaller insects for which several individuals were required.
Real-life processed samples (four industrial meals from different EU-based companies) of A. diaperinus were obtained through the International Producers of Insects for Food and Feed (IPIFF), but the origin of samples must remain anonymous.
For each A. diaperinus industrial meal, a mix containing 0.1% (in mass fraction) of A. diaperinus in a commercial fish feed (composition: fishmeal, fish oil, wheat gluten, protein concentrate extracted from pea, maize starch, yeast, lecithin, vitamins, and minerals) was prepared.
DNA Extraction
Genomic DNA was extracted and purified from all samples following the CTAB-based method described in Annex A.3.1 of the international standard ISO 21571:2005 (43). Plasmid DNA was isolated from bacterial cultures with the help of the Genopure Plasmid Maxi Kit (Roche Diagnostics GmbH, Mannheim, Germany). The quality and quantity of DNA extracted from samples were estimated spectrophotometrically using a Nanodrop ND-1000 spectrophotometer at 260 nm (A260) and 280 nm (A280) absorbance. DNA purity was determined using the A260/A280 ratio. The amplifiability of the DNA extract was successfully checked by real-time PCR with the 18S target for insects (41), rbcL (44) for plants, and with a generic fish target (45) for fish. Other species were tested with targets developed or evaluated within the European Union Reference Laboratory for Animal Proteins in Feedingstuff1 (EURL-AP, 2017) (32) or with the 18S target (46, 47). Ten nanograms of DNA was used in the PCRs.
The industrial meals and the feed mixes at 0.1% of A. diaperinus were extracted following the method recommended by EURL-AP and based on the adaptation of the protocol of the Wizard Magnetic DNA Purification System for Food kit (Promega, Madison, WI, USA). This method is described in the EURL-AP Standard Operating Procedure2 (EURL-AP, 2014). The quantities tested for this purpose are also in line with the EURL-AP SOP.
Primers and Probe for the Real-Time PCR
Eurogentec (Seraing, Belgium) synthesized the oligonucleotides. The primers and probe sequences developed for the detection of A. diaperinus are presented in Table 1. The probe for this latter method was labeled with the reporter dye FAM™ (6-carboxyfluorescein) at the 5′ end and the quencher dye TAMRA™ (tetramethyl-6-carboxyrhodamine) at the 3′ end. Table 1 also lists the primers used for the purpose of differentiating A. diaperinus from A. laevigatus.
Real-Time PCR Method
Real-time PCR (total reaction volume of 25 μL) was performed on a Lightcycler 480 (Roche Diagnostics Ltd., Rotkreuz, Switzerland) thermocycler using the Universal Mastermix provided by Diagenode (Seraing, Belgium). The reaction mixture included 12.5 μL of Master Mix, 1.7 μL of each primer (5 μM), 1.5 μL of probe (9 μM), 2.6 μL of bidistilled water, and 5 μL of DNA and distributed on 96-well reaction plates (Roche Diagnostics) for specific thermocyclers. Wells were covered with an adhesive film and centrifuged (2 min at 500 revolutions/min) to eliminate any air bubble in the well-bottoms. The thermal program was applied as follows: 2 min at 50°C; 10 min at 95°C; 50 cycles of 15 s at 95°C and 1 min at 60°C.
Specificity of the PCR Method
The specificity of the method was checked on 54 insect species of different taxonomic orders including 19 Coleoptera taxa other than A. diaperinus, 6 Diptera, 10 Orthoptera, 7 Hemiptera, 4 Hymenoptera, 5 Lepidoptera, 1 Neuroptera, and 1 Blattodea. One sample of larvae labeled buffalo worm and another sample of larvae considered as A. laevigatus were purchased from specialized companies. However, a Sanger sequencing trial (see Results) shows that these two samples belong to the A. diaperinus species and are therefore considered as such for the specificity test. The specificity tests were performed on two arachnids and six crustaceans, which like insects belong to the Arthropoda phylum, 1 mollusk and 33 species of vertebrates (12 terrestrial mammals, 6 sea mammals, 8 birds, 7 fish). The possibility of a cross-reaction with human DNA was also envisaged. Seven plant species frequently used in feed and food were included in the experimental setup (Table 2). Ten nanograms of DNA was used in the PCRs. Each DNA extract was tested at least in duplicate.
Cloning of the Target, Copy Number Determination of the Plasmid DNA, and Dilutions
The 134-bp target from the A. diaperinus cadherin gene was ligated into the 3.9-kb pCR® 2.1-TOPO plasmid vector (Invitrogen, Merelbeke, Belgium) following the TOPO® TA Cloning® kit instructions (Invitrogen). Plasmid DNA isolated from bacterial cultures was linearized with the HindIII restriction enzyme (Promega) and then purified using phenol–chloroform–isoamyl alcohol.
The quantity of recovered plasmid DNA was converted into copy numbers as usual (36, 48, 49), taking into consideration that (i) 1 unit of absorbance at 260 nm corresponds to a concentration of 50 μg/mL of double-stranded DNA, and (ii) the mean molar weight of one base pair is set at 635 Da (50).
The sensitivity, efficiency, and robustness of the PCR test were determined on diluted plasmid DNA. These dilutions were performed in water until an estimated copy number of 10,000 copies/5 μL was reached. Higher dilutions of the target DNA were prepared in a solution containing 50 ng/μL of salmon sperm DNA as background DNA. Low binding tubes were chosen to minimize DNA losses.
Copy Number Determination of A. diaperinus Genomic DNA and Dilutions
The quantity of genomic DNA corresponding to 36,000 target copies was estimated at 10.08 ng for A. diaperinus based on data from the animal genome size database (https://www.genome.size.com/results.php?page=3) at the University of Guelph (Ontario, Canada). The sensitivity (limit of detection [LOD]) and efficiency of the PCR test were determined on diluted genomic DNA. Genomic DNA dilutions were performed in a similar way as described for the cloned target.
Limit of Detection
Target sensitivity was evaluated following the recommendations of the former AFNOR XP V03-020-2 standard (51). This standard no longer exists, but the principles detailed in it are still valid. The absolute LOD was determined for the PCR assay (primers + probe + amplification program) on dilutions of plasmid material and on dilutions of genomic material.
The subsequent dilutions had to contain 50, 20, 10, 5, 2, 1, and 0.1 copies of the target. Six PCRs had to be achieved for each dilution. The method's LOD6 was the smallest copy number for which the six PCRs were positive, but only if the highest dilution supposed to contain the 0.1 copy per reaction generated a maximum of one positive PCR signal on six replicates. If more than one positive signal was observed for the 0.1 copy, the DNA quantities had to be revised. The copy number corresponding to LOD6 was then tested 60 times on the same plate (determination of the LOD95%). The LOD95% is validated as equal or below a given copy number if at least 95% of positive signals are recorded out of the 60 replicates. The highest acceptable copy number for LOD6 and LOD95% is 20 copies.
Efficiency
The efficiency of the PCR assay was calculated with a dilution series of genomic DNA and plasmid material at target levels of 5,000, 2,500, 1,000, 500, and 100 copies. Each dilution was analyzed in six replicates and on four runs. The efficiency has to be between 90 and 110% (52).
Digital PCR
The number of copies of the nuclear and plasmid DNA dilutions at approximately 500 copies/5 μL (5 μL being the volume of the DNA extracts added in the real-time PCR mix) were checked by digital PCR. Digital PCR was performed on the Biomark™ HD system (Fluidigm Corporation, South San Francisco, CA, USA) using the 12.765 Digital Array™. These digital arrays comprise 12 panels (12 wells, thus 12 samples), each of which is partitioned into 765 individual PCR of 6 nL. The reaction mixture included 4 μL of Universal Master Mix with passive reference (Diagenode), 0.15 μL of each primer (18.1 μM), 0.15 μL of probe (28.8 μM), 0.4 μL GE sample loading reagent (Fluidigm), and 3.15 μL of plasmid DNA. Eight microliters of reaction mix was dispensed into each sample inlet, and ~4.6 μL of this reaction mix was distributed throughout the partitions within each panel using an automated NanoFlex IFC Controller (Fluidigm Corporation) (53). Two arrays were analyzed with for each, 11 replicates of plasmid dilution and one no template control. The thermal program was as follows: 10-min activation step at 95°C, 50 cycles of 15 s at 95°C for denaturation and 60 s at 60°C for annealing and extension.
The number of target molecules per panel was determined using the BioMark HD Digital PCR software.
Robustness of the PCR Method
The method robustness was tested by introducing some slight deviations to the standard experimental conditions (54). Parameters considered were as usual (36, 52, 55): the annealing temperature (60 ± 1°C), the primer concentrations (standard or reduced by 30%), the probe concentration (standard or reduced by 30%), and the real-time PCR Master Mix volume (standard or ± 1 μL), which involves a final reaction volume of 25 ± 1 μL. Six replicates of the plasmid borne target at 20 copies/5 μL were tested in the conditions described in Table 3. The robustness was performed on two real-time PCR platforms: thermocycler Lightcycler 480 (Roche Diagnostics Ltd.) with Universal Mastermix by Diagenode and thermocycler QuantStudio™ 5 Real-Time PCR system (Applied Biosystems, Thermo Fisher Scientific, Foster City, CA, USA) with ABI TaqMan 2x Universal PCR Master Mix No AmpErase UNG (Applied Biosystems). The acceptance criterion is that all deviations to the standard protocol must give a positive result at a level of 20 copies of the target in the reaction (52).
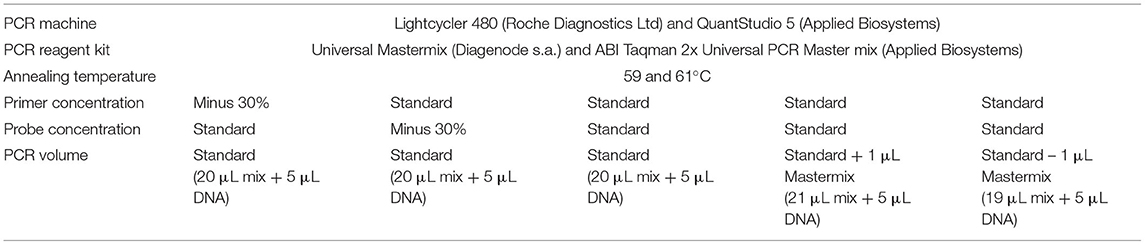
Table 3. Experimental conditions tested to evaluate the robustness of the described Alphitobius diaperinus PCR test.
Applicability of the PCR Method
The applicability of the PCR method was checked in duplicate on four real-life processed samples (industrial meals) of A. diaperinus produced in the EU and on a fish feed containing 0.1% in mass fraction of A. diaperinus industrial meal. Two DNA extracts with two dilutions were tested by PCR in duplicate.
Transferability of the PCR Method
The efficiency and the LOD (LOD6 and LOD95%) of the PCR assay were tested on genomic DNA in the laboratory of Eurofins Biologie Moléculaire France with conditions similar to those of the developer's laboratory (CRA-W, Gembloux, Belgium). The dilution series for the LOD test were carried out by this second laboratory starting from the solution at 500 copies/5 μL checked by digital PCR.
Real-time PCR was performed on thermocycler CFX96 Deep Well Real-time PCR Detection Systems (Bio-rad, Hercules, CA, USA) using the Applied Biosystems™ TaqMan™ Universal PCR Master Mix (Applied Biosystems). Reaction mixtures were distributed on Hard-Shell® 96-Well PCR Plates (Bio-rad) developed for the CFX96 thermocyclers. Wells were covered with adhesive film, and the plates were centrifuged to eliminate any air bubble in the well-bottoms.
Amplicon Preparation for Sanger Sequencing
The PCRs to generate amplicons that had to be checked by Sanger sequencing were done as follows. For the large cadherin target, the PCR contained 1 μL of DNA extract from the sample to be checked, 6 μL of 5 × GoTaq® Flexi Buffer (Promega), 3 μL of 2 mM dNTP mix (Thermo Scientific, Waltham, MA, USA), 3 μL of bovine serum albumin (BSA; Roth, Karlsruhe, Germany), 1.8 μL of 25 mM MgCl2 solution (Promega), 3 μL of 5 μM forward and reverse primers, 0.15 μL of 5 U/μL GoTaq® G2 Flexi DNA Polymerase (Promega), and nuclease-free water to 30 μL. The thermal cycling conditions were set as follows: initial denaturation at 95°C for 5 min followed by 35 cycles at 95°C for 30 s, 56°C for 30 s, and 72°C for 1 min, and a final extension at 72°C for 10 min.
To generate amplicons focused on the cytochrome c oxidase subunit I (COI) targets (Table 1), PCRs were set up in at similar way and contained 1 μL of DNA extract from the sample to be checked, 6 μL of 5x GoTaq® Flexi Buffer (Promega), 3 μL of 2 mM dNTP mix (Thermo Scientific), 3 μL of BSA (Roth), 1.8 μL of 25 mM MgCl2 solution (Promega), 3 μL of 5 μM forward and reverse primers, 0.15 μL of 5 U/μL GoTaq® G2 Flexi DNA Polymerase (Promega), and nuclease-free water to 30 μL. The thermal cycling conditions consisted in an initial denaturation at 95°C for 5 min followed by 35 cycles at 95°C for 30 s, 47°C (COI_Alphi_F/R) or 52°C (COI_Alphi_F2/R2) for 30 s and 72°C for 1 min, and a final extension at 72°C for 10 min.
Five microliters of PCR products were run on a 1.2% agarose gel to check amplicon quality. The remaining 25 μL of PCR products was sent to Eurofins Genomics (Constance, Germany) for Sanger sequencing.
Results
The cadherin gene of A. diaperinus was used to select a piece of DNA that is specific to the considered insect species. Appropriate primers and probe were designed to amplify a 134-bp fragment of the cadherin gene (Table 1). The latter is considered as a single-copy gene in several insect species such as the Lepidopteran Ostrinia nubilalis (Hübner) (56) or the Coleoptera Diabrotica virgifera virgifera (Le Conte) (57), which is an advantage for quantitation purposes.
The specificity was first investigated in silico using the Blast tool available on the National Center for Biotechnology Information (NCBI) database. The different blasts and the alignments of DNA sequences with other Coleopteran (58) performed indicated that the PCR test should be specific to the target species A. diaperinus. The alignment with the closest relative within the NCBI database is the T. molitor sequence. It shows some similarity but not enough for allowing primers and probes to hybridize (Supplementary File 1). Specificity was also experimentally tested on DNA from A. diaperinus of various origins as well as on 53 other non-target insect species, including 19 Coleoptera. Positive results were obtained only with all samples of A. diaperinus. No signal was obtained with the 53 other insect species, the 42 other animal species (arachnids, crustaceans, mollusk and vertebrates), and the seven plant species tested (Table 2).
A difficulty that appeared was to know if the test enables to distinguish A. diaperinus from A. laevigatus, a closely related species commercialized as feed product for non-farmed animals. Products labeled as A. laevigatus or as buffalo worm were tested, and a clear positive signal with the PCR test for A. diaperinus was observed. A doubt existed, however, if these samples really belonged to the A. laevigatus species. To check this hypothesis, Sanger sequencing was applied on PCR products of eight collected samples of Alphitobius (Supplementary File 2). Three targets were considered. The first one focused on a larger cadherin target (Table 1), which completely contains the smaller target of 134 bp. The two other targets focused on COI regions known as more variable and considered as suitable to allow a distinction between A. diaperinus and A. laevigatus according to published data. All Sanger sequencings were successful and showed identical sequences for the eight samples (Supplementary Files 2–5).
The sequences obtained for the cadherin target corresponded to the A. diaperinus sequence published by Hua et al. (2014-KC470207.1)3 (Supplementary File 3). The alignment of sequenced COI fragments showed that all analyzed specimens belong to the A. diaperinus species when compared with A. diaperinus and A. laevigatus reference sequences. The first COI portion corresponded to the A. diaperinus sequence published by Hong et al. (2020-NC_049092.1)4 (Supplementary File 4); this COI region is, however, not available for A. laevigatus. Reference sequences are available for both Alphitobius species for the second COI region considered. Results for that target showed that all samples of Alphitobius tested belonged to the A. diaperinus species (Supplementary File 5).
The amplification efficiency, LOD, and robustness were evaluated on plasmid DNA. The efficiency and LOD were also determined on genomic DNA.
To check that the number of copies in the dilutions used to assess the performance criteria was correct, the dilutions at approximately 500 copies/5 μL were estimated by digital PCR on a Biomark™ HD system. For the genomic DNA, the average obtained over the 10 measurements by digital PCR was 339 copies/5 μL with a variation coefficient at 9.45% (Table 4). This mean copy number measured was slightly lower than the expected value (based on the genome size of A. diaperinus and considering the cadherin gene as a single-copy gene). The difference between these values did not exceed a factor of 2, corresponding to the subsequent dilution. These results therefore confirm that the cadherin gene is a single-copy gene per haploid genome as mentioned in other studies (56, 57). However, in order to be closer to the expected values to evaluate the efficiency and LOD, new dilutions on the genomic DNA were carried out, taking into account the results obtained in digital PCR. On these new dilutions, the average obtained over the 22 measurements by digital PCR was 498 copies/5 μL with a variation coefficient at 9.21% (Table 5). The mean copy number measured was estimated at the expected value and it is from this dilution series that the efficiency and sensitivity were evaluated.
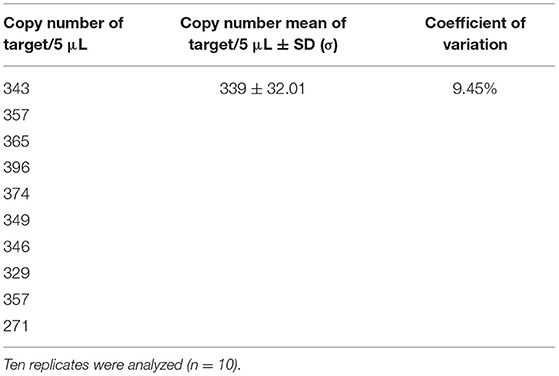
Table 4. Copy numbers obtained on dilution of genomic DNA at ~500 copies/5 μL by digital PCR on a Biomark™ HD system.
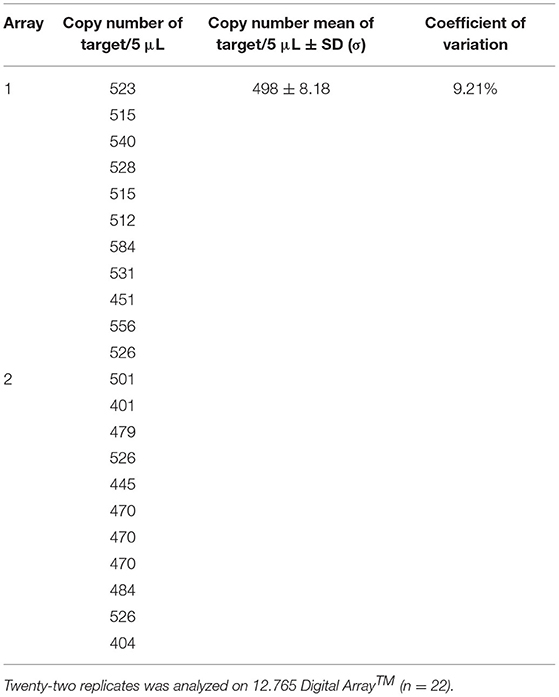
Table 5. Copy numbers obtained on dilution slightly adapted of genomic DNA at ~500 copies/5 μL by digital PCR on a Biomark™ HD system.
The copy number of the plasmid material (linearized) was also checked, and the average copy obtained over the 22 measurements by digital PCR was 446 copies/5 μL with a variation coefficient at 9.26% (Table 6). The mean copy number measured was estimated at the expected value, and it is from this dilution series that the efficiency, sensitivity, and robustness were evaluated.
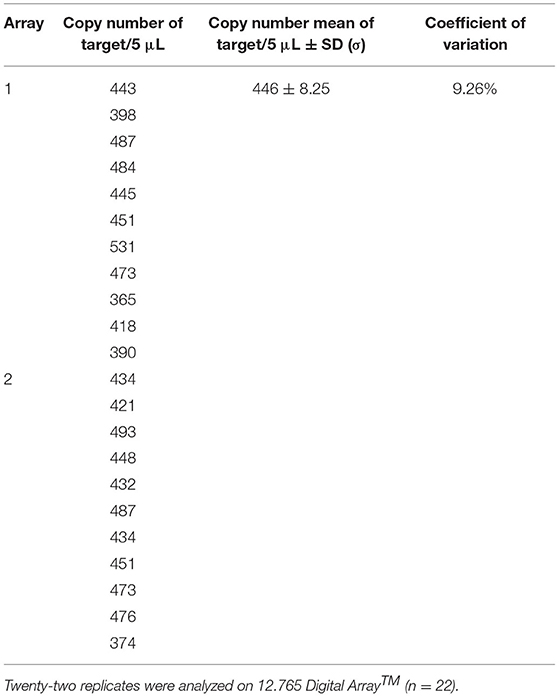
Table 6. Copy numbers obtained on dilution of plasmid DNA at ~500 copies/5 μL by digital PCR on a Biomark™ HD system.
The PCR efficiency was evaluated at 102.5% on plasmid DNA and 100.0% on genomic DNA. This was calculated, taking into account the mean Cq (quantification cycle) values obtained at the different copy numbers tested (from 5,000 to 10), and no outliers were encountered (Tables 7, 8). When calculated per plate, the efficiency was always higher than 90% and therefore met the acceptance criterion proposed by Broeders et al. (52).
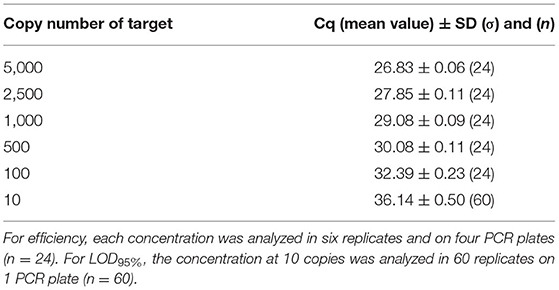
Table 7. Cq values obtained on dilutions of plasmid material used for efficiency calculation and for LOD95%.
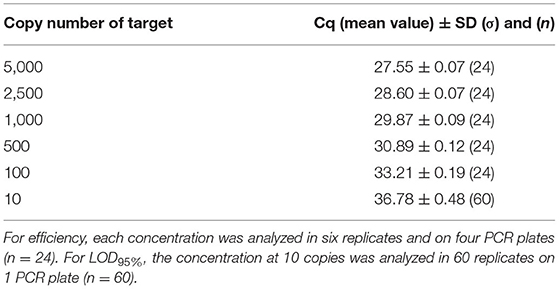
Table 8. Cq values obtained on dilutions of genomic material used for efficiency calculation and for LOD95%.
Concerning the sensitivity testing, the LOD6 was estimated at five copies following the AFNOR XP V03-020-2 standard approach (51) and also at five copies for the LOD95% with 57/60 positive signals on plasmid DNA and 58/60 positive signals on genomic DNA. Sixty of 60 positive signals were obtained at the level of 10 copies with a mean Cq value of 36.14 cycles on plasmid DNA and 36.78 cycles on genomic DNA. Therefore, the PCR test easily reaches the recommended performance criterion (≤ 20 copies).
The PCR method robustness was also evaluated on plasmid DNA, with success. All tested deviations to the standard protocol delivered positive results at the level of 20 copies in the PCR.
Positive signals were obtained on industrial samples (PAPs of A. diaperinus) showing the applicability of the PCR test on real-life samples (Table 9).
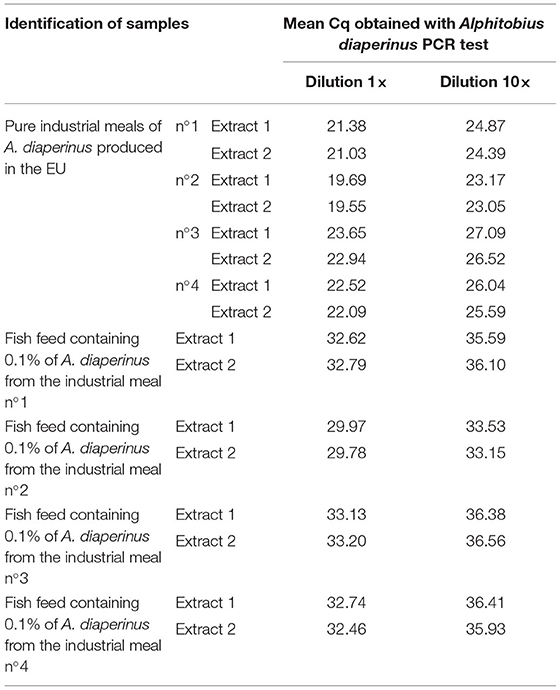
Table 9. Mean Cq obtained with the Alphitobius diaperinus PCR test on processed samples from A. diaperinus and on mixes containing 0.1% in mass fraction of A. diaperinus in a commercial fish feed (n = 2).
The applicability was tested on a commercial fish feed adulterated with a low content of processed A. diaperinus. The commercial fish feed was first tested as free of A. diaperinus and the amplifiability of the DNA extracts obtained from this fish feed was checked with a 18S rDNA target (46, 47).
The four mixes of fish feed containing 0.1% of different processed A. diaperinus meals were tested and gave positive results with the cadherin PCR test (Table 9). The 10-fold dilutions provided evidence that there was no inhibitory effect of the feed matrix on the amplification of the A. diaperinus target.
Concerning the transferability, the PCR efficiency was evaluated at 90.0% on genomic DNA in a second laboratory and therefore met the acceptance criterion proposed by Broeders et al. (52). Table 10 indicates the mean Cq values obtained with the different copy numbers tested (from 5,000 to 10), and no outliers were encountered. When calculated per plate, the efficiency was higher than 90% for three plates and slightly below for the fourth one with an efficiency at 87.7%. The LOD6 was estimated at five copies following the former AFNOR XP V03-020-2 standard approach (51) and at 10 copies for the LOD95% with 60/60 positive signals. The mean Cq value at 10 copies is of 37.08 cycles. The PCR test easily reached the recommended performance criterion (≤ 20 copies). The transferability of the method was therefore demonstrated.
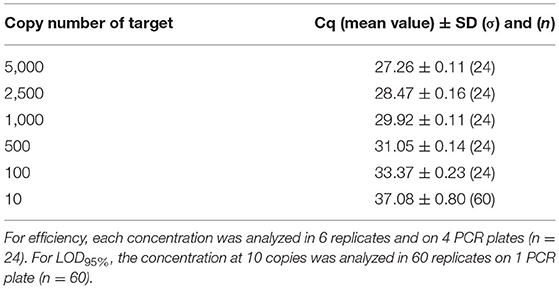
Table 10. Cq values obtained on dilutions of genomic material used for efficiency calculation and for LOD95% for the transferability test.
Discussion
The study describes a specific, sensitive, and robust test to detect A. diaperinus. With the recent authorization in the EU legislation to use eight insect species, among which lesser mealworm, in aquafeed, pig, and poultry feed, the interest of such a PCR test is increasing.
During the search for the target and the validation study, special care was taken to be able to distinguish lesser mealworm from other representatives of the Tenebrionidae family (Supplementary File 1). Blasting the sequences of the developed primers and of the targeted fragment against the NCBI nucleotide database showed that the PCR test is specific to the target species A. diaperinus. Unfortunately, cadherin gene sequences for other Alphitobius species are not available in the NCBI and DNA Data Bank of Japan databases. Sequence alignments were therefore not possible. The other Alphitobius species are, however, not produced nor marketed, with the exception of A. laevigatus.
It seems that there is an important confusion at commercial level between A. diaperinus and A. laevigatus. Indeed, A. diaperinus is known under the common names of lesser mealworm for the larvae (12, 14, 25, 42, 59) and darkling beetle at adult stage (19, 60, 61), whereas A. laevigatus is known as black fungus beetle5 (37, 62, 63). Especially as larvae, the name of buffalo worm can be used to designate both species (7, 9, 64–70). A. laevigatus looks like A. diaperinus in size and shape3 (62, 63). In a review on Tenebrionidae in France, Bonneau (71) also clearly points out that there are confusions between A. diaperinus, A. laevigatus, Alphitobius piceus Olivier, and Alphitobius ovatus Herbst.
This confusion seems also to occur at the level of the sequences available in the NCBI database. Two COI sequences published by (72, 73) (accession no. KM435102.1 and KM652640.1) attributed to A. diaperinus show 99% identity with A. laevigatus [KP410252.1, (74)] but only 88% with A. diaperinus [NC_049092.1, (75)].
This probably also explains that the ordered samples of A. laevigatus were wrongly labeled by their providers. The Sanger sequencing revealed that these samples were in reality A. diaperinus samples. No real commercial sample of A. laevigatus was found, and even if we did not check with all possible sources, it seems that it is not that easy to find marketed A. laevigatus larvae. That is the reason why from a merely practical point of view we consider that the 134-bp cadherin target published in this study is specific to A. diaperinus. Nevertheless, from a scientific viewpoint, it is not impossible that the target is specific only at genus level. It could not be checked because of a lack of appropriate samples to do so.
The applicability of the PCR method was also successfully tested on real-world samples. This validates the implementation of the method on industrial samples.
Finally, it should be stressed that the target is present only once per haploid genome, which makes it a suitable target for possible quantification purposes. This would require to have a general insect target, present only once per haploid genome. To our knowledge, such a target has unfortunately not yet been identified.
Conclusions
The developed PCR method based on the cadherin gene fits for the purpose of detection of A. diaperinus in feed. Indeed, the efficiency met the required criterion, the specificity gave good results, and only A. diaperinus was detected with respect to the insect, animal, and plant species tested. The acceptance criteria were also reached for sensitivity (LOD6 and LOD95%) and robustness. The PCR method was applicable on real-life samples from industry even when A. diaperinus was present at 0.1% in mass fraction in a fish feed. Finally, the transferability of the method in a second laboratory was also demonstrated by testing the efficiency and LOD.
The developed method was primarily aimed for application on feed. However, it is not excluded that several of the PCR tests developed in the study might be helpful to taxonomists in a better delineation of the several taxons within the genus Alphitobius.
Data Availability Statement
The original contributions presented in the study are included in the article/Supplementary Material, further inquiries can be directed to the corresponding author.
Author Contributions
AM, HS, BD, and FD contributed to the design and implementation of the research. AM, HS, BD, JM, and FD designed and performed the experiments. SG and J-FM participated to the transferability study. AM, JM, BD, SG, J-FM, and FD analyzed the data and interpreted the results. AM wrote the manuscript with the help of FD. GB, BD, FF, SG, J-FM, and OF provided valuable comments to improve the quality of the manuscript. All authors contributed to the article and approved the submitted version.
Funding
This research was financially supported by the European Commission in the frame of Horizon 2020 Public-Private Partnership Bio-Based Industries Joint Undertaking (topic BBI.2018.F2 – Large-scale production of proteins for food and feed applications from alternative, sustainable sources) through the FARMYNG project.
Conflict of Interest
The authors declare that the research was conducted in the absence of any commercial or financial relationships that could be construed as a potential conflict of interest.
Publisher's Note
All claims expressed in this article are solely those of the authors and do not necessarily represent those of their affiliated organizations, or those of the publisher, the editors and the reviewers. Any product that may be evaluated in this article, or claim that may be made by its manufacturer, is not guaranteed or endorsed by the publisher.
Acknowledgments
The authors would like to thank Céline Aerts (of the Microbiology team, Unit 12, CRA-W) for her technical help. They would also like to thank the IPIFF for the industrial meals provided.
Supplementary Material
The Supplementary Material for this article can be found online at: https://www.frontiersin.org/articles/10.3389/fvets.2022.718806/full#supplementary-material
Footnotes
1. ^www.eurl.craw.eu/wp-content/uploads/2021/01/EURL-AP-SOP-Ruminant-PCR-FINAL-V1.2.pdf
2. ^www.eurl.craw.eu/wp-content/uploads/2021/01/EURL-AP-SOP-DNA-extraction-V1.1.pdf
3. ^https://www.ncbi.nlm.nih.gov/nuccore/KC470207.1
4. ^https://www.ncbi.nlm.nih.gov/nuccore/NC_049092.1
5. ^https://www.ams.usda.gov/sites/default/files/media/StoredGrainInsectsReference2017.pdf
References
1. Kim M-J, Jung S-K, Kim S-Y, Kim H-Y. Development of detection method for edible silkworm (Bombyx mori) using real-time PCR. Food Control. (2018) 94:295–9. doi: 10.1016/j.foodcont.2018.07.021
2. Sogari G, Menozzi D, Mora C. Sensory-liking expectations and perceptions of processed and unprocessed insect products. Int J Food Syst Dyn. (2018) 9:314–20. doi: 10.18461/ijfsd.v9i4.942
3. Van Huis A. Insects as food and feed, a new emerging agricultural sector: a review. J Insects Food Feed. (2020) 6:27–44. doi: 10.3920/JIFF2019.0017
4. Roncolini A, Milanović V, Aquilanti L, Cardinali F, Garofalo C, Sabbatini R, et al. Lesser mealworm (Alphitobius diaperinus) powder as a novel baking ingredient for manufacturing high-protein, mineral-dense snacks. Food Res Int. (2020) 131:109031. doi: 10.1016/j.foodres.2020.109031
5. Rumpold BA, Schlüter OK. Nutritional composition and safety aspects of edible insects. Mol Nutr Food Res. (2013) 57:802–23. doi: 10.1002/mnfr.201200735
6. Lacroix IME, Dávalos Terán I, Fogliano V, Wichers HJ. Investigation into the potential of commercially available lesser mealworm (A. diaperinus) protein to serve as sources of peptides with DPP-IV inhibitory activity. Int J Food Sci Tech. (2019) 54:696–704. doi: 10.1111/ijfs.13982
7. Mwangi MN, Oonincx DGAB, Stouten T, Veenenbos M, Melse-Boonstra A, Dicke M, et al. Insects as sources of iron and zinc in human nutrition. Nutr Res Rev. (2018) 31:248–55. doi: 10.1017/S0954422418000094
8. Van Broekhoven S, Oonincx DGAB, van Huis A, van Loon JJA. Growth performance and feed conversion efficiency of three edible mealworm species (Coleoptera: Tenebrionidae) on diets composed of organic by-products. J Insect Physiol. (2015) 73:1–10. doi: 10.1016/j.jinsphys.2014.12.005
9. Lamsal B, Wang H, Pinsirodom P, Dossey AT. Applications of insect-derived protein ingredients in food and feed industry. J Am Oil Chem Soc. (2018) 96:2. doi: 10.1002/aocs.12180
10. Thevenot A, Rivera JL, Wilfart A, Maillard F, Hassouna M, Senga-Kiesse T, et al. Mealworm meal for animal feed: environmental assessment and sensitivity analysis to guide future prospects. J Clean Prod. (2017) 170:1260–7. doi: 10.1016/j.jclepro.2017.09.054
11. Nesic K, Zagon J. Insects – a promising feed and food protein source? Meat Tech. (2019) 60:56–67. doi: 10.18485/meattech.2019.60.1.8
12. Van Huis A. Potential of insects as food and feed in assuring food security. Annu Rev Entomol. (2012) 58:563–83. doi: 10.1146/annurev-ento-120811-153704
13. Oonincx DGAB, van Itterbeeck J, Heetkamp MJW, van den Brand H, van Loon JJA, van Huis A. An exploration on greenhouse gas and ammonia production by insect species suitable for animal or human consumption. PLoS ONE. (2010) 5:e14445. doi: 10.1371/journal.pone.0014445
14. European Commission. Commission regulation (EU) 2017/893. Amending Annexes I and IV to Regulation (EC) No 999/2001 of the European Parliament and of the Council and Annexes X, XIV and XV to Commission Regulation (EU) No 42/2011 as Regards the Provisions on Processed Animal Protein. O. J. E. U. L 138 (2017). p. 92–116.
15. European Commission. Commission regulation (EU) 2021/1372 of 17 August 2021 amending Annex IV to Regulation (EC) No 999/2001 of the European Parliament and of the Council as Regards the Prohibition to Feed Non-ruminant Farmed Animals, Other Than Fur Animals, With Protein Derived From Animals. O. J. E. U. L 295 (2021). p. 1–17.
16. Ricciardi C, Baviera C. Role of carbohydrates and proteins in maximizing productivity in Alphitobius diaperinus (Coleopteran tenebrionidae). J Zool. (2016) 99:97–105. doi: 10.19263/Redia-99.16.13
17. European Commission. Commission Regulation (EU) 2021/1925 of 5 November 2021 Amending Certain Annexes to Regulation (EU) No 142/2011 as Regards the Requirements for Placing on the Market of Certain Insect Products and the Adaptation of a Containment Method. O.J.E.U. L 393 (2021). p. 4–6.
18. European Commission. Regulation (EU) 2015/2283 of the European Parliament and of the Council of 25 November 2015 on Novel Foods, Amending Regulation (EU) No 1169/2011 of the European Parliament and of the Council and Repealing Regulation (EC) No 258/97 of the European Parliament and of the Council and Commission Regulation (EC) No 1852/2001. O. J. E. U. L 327 (2015). p. 1–22.
19. EFSA Scientific Committee. Scientific opinion on a risk profile related to production and consumption of insects as food and feed. EFSA J. (2015) 13:4257. doi: 10.2903/j.efsa.2015.4257
20. EFSA NDA Panel. Scientific Opinion on the safety of dried yellow mealworm (Tenebrio molitor larva) as a novel food pursuant to Regulation (EU) 2015/2283. EFSA J. (2021) 19:6343. doi: 10.2903/j.efsa.2021.6343
21. EFSA NDA Panel. Scientific Opinion on the safety of frozen and dried formulations from whole yellow mealworm (Tenebrio molitor larva) as a novel food pursuant to Regulation (EU) 2015/2283. EFSA J. (2021) 19:6778. doi: 10.2903/j.efsa.2021.6778
22. EFSA NDA Panel. Scientific Opinion on the safety of frozen and dried formulations from whole house crickets (Acheta domesticus) as a Novel food pursuant to Regulation (EU) 2015/2283. EFSA J. (2021) 19:6779. doi: 10.2903/j.efsa.2021.6779
23. European Commission. Commission Implementing Regulation (EU) 2021/882 of 1 June 2021 Authorising the Placing on the Market of Dried Tenebrio Molitor Larva as a Novel Food Under Regulation (EU) 2015/2283 of the European Parliament and of the Council, and Amending Commission Implementing Regulation (EU) 2017/2470. O.J.E.U. L 194 (2021). p. 16–18.
24. Francis F, Mazzucchelli G, Baiwir D, Debode F, Berben G, Caparros Megido R. Proteomics based approach for edible insect fingerprinting in novel food: differential efficiency according to selected model species. Food Control. (2020) 112:107135. doi: 10.1016/j.foodcont.2020.107135
25. Leni G, Prandi B, Varani M, Faccini A, Caligiani A, Sforza S. Peptide fingerprinting of Hermetia illucens and Alphitobius diaperinus: identification of insect species-specific marker peptides for authentication in food and feed. Food Chem. (2020) 320:126681. doi: 10.1016/j.foodchem.2020.126681
26. Ulrich S, Kühn U, Biermaier B, Piacenza N, Schwaiger K, Gottschalk C, et al. Direct identification of edible insects by MALDI-TOF mass spectrometry. Food Control. (2017) 76:96–101. doi: 10.1016/j.foodcont.2017.01.010
27. Feltens R, Görner R, Kalkhof S, Gröger-Arndt H, von Bergen M. Discrimination of different species from the genus Drosophila by intact protein profiling using matrix-assisted laser desorption ionization mass spectrometry. BMC Evol Biol. (2010) 10:95. doi: 10.1186/1471-2148-10-95
28. Belghit I, Lock E-J, Fumière O, Lecrenier M-C, Renard P, Dieu M, et al. Species-Specific discrimination of insect meals for aquafeeds by direct comparison of tandem mass spectra. Animals. (2019) 9:222. doi: 10.3390/ani9050222
29. Veys P, Baeten V. Protocol for the isolation of processed animal proteins from insects in feed and their identification by microscopy. Food Control. (2018) 92:496–504. doi: 10.1016/j.foodcont.2018.05.028
30. Debode F, Janssen E, Berben G. Physical degradation of genomic DNA of soybean flours does not impair relative quantification of its transgenic content. Eur Food Res Technol. (2007) 226:273–80. doi: 10.1007/s00217-006-0536-1
31. Debode F, Marien A, Janssen E, Bragard C, Berben G. The influence of amplicon length on real-time PCR results. Biotechnol Agron Soc Environ. (2017) 21:3–11. Available online at: https://popups.uliege.be/1780-4507/index.php?id=13461
32. Marien A, Fumière O, Debode F, Hulin J, Berben G. The horse meat scandal – The European analytical response. In: Burns M, Foster L, Walker M, editors. DNA Techniques to Verify Food Authenticity: Applications in Food Fraud. London: Royal Society of Chemistry (2019). p. 177–88.
33. Grohmann L, Seiler C. Standardization of DNA-based methods for food authenticity testing. In: Burns M, Foster L, Walker M, editors. DNA Techniques to Verify Food Authenticity: Applications in Food Fraud. London: Royal Society of Chemistry (2019). p. 227–34.
34. Hirst B, Fernandez-Calvino L, Weiss T. Commercial DNA testing. In: Burns M, Foster L, Walker M, editors. DNA Techniques to Verify Food Authenticity: Applications in Food Fraud. London: Royal Society of Chemistry (2019). p. 264–82.
35. Cavelier L, Johannisson A, Gyllensten U. Analysis of mtDNA copy number and composition of single mitochondrial particles using flow cytometry and PCR. Exp Cell Res. (2000) 259:79–85. doi: 10.1006/excr.2000.4949
36. Marien A, Debode F, Aerts C, Ancion C, Francis F, Berben G. Detection of Hermetia illucens by real-time PCR. J Insects Food Feed. (2018) 4:115–22. doi: 10.3920/JIFF2017.0069
37. Zagon J, di Rienzo V, Potkura J, Lampen A, Braeuning A. A real-time PCR method for the detection of black soldier fly (Hermetia illucens) in feedstuff. Food Control. (2018) 91:440–8. doi: 10.1016/j.foodcont.2018.04.032
38. Kim S-Y, Kim M-J, Jung S-K, Kim H-Y. Development of a fast real-time PCR assay based on TaqMan probe for identification of edible rice grasshopper (Oxya chinensis) in processed food products. Food Res Int. (2019) 116:441–6. doi: 10.1016/j.foodres.2018.08.059
39. Zarske M, Zagon J, Schmolke S, Seidler T, Braeuning A. Detection of silkworm (Bombyx mori) and Lepidoptera DNA in feeding stuff by real-time PCR. Food Control. (2021) 126:108059. doi: 10.1016/j.foodcont.2021.108059
40. Daniso E, Tulli F, Cardinaletti G, Cerri R, Tibaldi E. Molecular approach for insect detection in feed and food: the case of Gryllodes sigillatus. Eur Food Res Technol. (2020) 246:2373–81. doi: 10.1007/s00217-020-03573-1
41. Debode F, Marien A, Gérard A, Francis F, Fumière O, Berben G. Development of real-time PCR targets for the detection of Tenebrio molitor in food and feed. Food Addit Contam Part A. (2017) 34:1421–6. doi: 10.1080/19440049.2017.1320811
42. Hua G, Park Y, Adang MJ. Cadherin AdCad1 in Alphitobius diaperinus larvae is a receptor of Cry3Bb toxin from Bacillus thuringiensis. Insect Biochem Mol. (2014) 45:11–7. doi: 10.1016/j.ibmb.2013.10.007
43. ISO 21571:2005. Foodstuffs – Methods of Analysis for the Detection of Genetically Modified Organisms and Derived Products – Nucleic acid Extraction. Geneva: International Organisation for Standardization (2005).
44. Debode F, Janssen E, Marien A, Berben G. DNA detection by conventional and real-time PCR after extraction from vegetable oils. J Am Oil Chem Soc. (2012) 89:1249–57. doi: 10.1007/s11746-012-2007-0
45. Debode F, Marien A, Ledoux Q, Janssen E, Ancion C, Berben G. Detection of ornamental transgenic fish by real-time PCR and fluorescence microscopy. Transgenic Res. (2020) 29:283–94. doi: 10.1007/s,11248-020-00197-9
46. Garikipati DK, Gahr SA, Rodgers BD. Identification, characterization, and quantitative expression analysis of rainbow trout myostatin-1a and myostatin-1b genes. J Endocrinol. (2006) 190:879–88. doi: 10.1677/joe.1.06866
47. Debode F, Janssen E, Marien A, Devlin RH, Lieske K, Mankertz J, et al. Detection of transgenic Atlantic and Coho salmon by real-time PCR. Food Anal Method. (2017) 11:2396–406. doi: 10.1007/s12161-018-1214-1
48. Debode F, Marien A, Janssen E, Berben G. Design of multiplex calibrant plasmids, their use in GMO detection and the limit of their applicability for quantitative purposes owing to competition effects. Anal Bioanal Chem. (2010) 396:2151–64. doi: 10.1007/s00216-009-3396-2
49. Debode F. Développement de Méthodologies Pour la Détection des Plantes Génétiquement Modifiées (Doctoral thesis). AGRO, UCL, 367/2017 (2017). 391 p. Available online at: http://hdl.handle.net/2078.1/186329 (accessed January 01, 2021).
50. Sambrook J, Fritsch EF, Maniatis T. Molecular Cloning: A Laboratory Manual. 2nd ed. New York, NY: Cold Spring Harbor Laboratory Press (1989).
51. AFNOR. Détection et Quantification des Organismes Végétaux Génétiquement Modifiés et Produits Dérivés. Partie 2: Méthodes Basées sur la Réaction de Polymérisation en Chaîne. Saint-Denis La Plaine: AFNOR; Report No.: AFNOR Standard XP-V-03-020-2 (2008).
52. Broeders S, Huber I, Grohmann L, Berben G, Taverniers I, Mazzara M, et al. Guidelines for validation of qualitative real-time PCR methods. Trends Food Sci Technol. (2014) 37:115–26. doi: 10.1016/j.tifs.2014.03.008
53. Corbisier P, Bhat S, Partis L, Rui Dan Xie V, Emslie KR. Absolute quantification of genetically modified MON810 maize (Zea mays L.) by digital polymerase chain reaction. Anal Bioanal Chem. (2010) 396:2143–50. doi: 10.1007/s00216-009-3200-3
54. CCMAS. Guidelines on Performance Criteria and Validation of Methods for Detection, Identification and Quantification for Specific DNA Sequences and Specific Proteins in Foods (Rep. No. CAC/GL 74-2010). Codex committee on methods of analysis and sampling (2010).
55. Debode F, Janssen E, Bragard C, Berben G. Detection by real-time PCR and pyrosequencing of the cry1Ab and cry1Ac genes introduced in GM constructions. Food Addit Contam Part A. (2017) 34:1398–409. doi: 10.1080/19440049.2017.1317925
56. Bel Y, Ferré J, Escriche B. Quantitative real-time PCR with SYBR Green detection to assess gene duplication in insects: study of gene dosage in Drosophila melanogaster (diptera) and in Ostrinia nubilalis (lepidoptera). BMC Res Notes. (2011) 4:84. doi: 10.1186/1756-0500-4-84
57. Nekl ER, Siqueira H, Moriyama H, Siegfried BD. A novel cadherin-like gene from Western corn rootworm, Diabrotica virgifera virgifera (Coleoptera: Chrysomelidae). In: The 2005 ESA (Entomological Society of America) Annual Meeting and Exhibition, 18 December, Lauderdale, Florida. Presentation D0546 (2005). Available online at: https://esa.confex.com/esa/2005/techprogram/paper_22465.htm (accessed November 30, 2020).
58. Zhang S, Che L, Li Y, Liang D, Pang H, Slipinski A, et al. Evolutionary history of Coleoptera revealed by extensive sampling of genes and species. Nat Commun. (2018) 9:205. doi: 10.1038/s41467-017-02644-4
59. Blickenstaff CC. Common names of insects approved by the entomological society of America. Bull Entomol Soc Am. (1965) 11:287–320. doi: 10.1093/besa/11.4.287
60. Goodwin MA, Waltman WD. Transmission of eimeria, viruses, and bacteria to chicks: darkling beetles (Alphitobius diaperinus) as vectors of pathogens. J Appl Poult Res. (1996) 5:51–5. doi: 10.1093/japr/5.1.51
61. Hazeleger WC, Bolder NM, Beumer RR, Jacobs-Reitsma WF. Darkling beetles (Alphitobius diaperinus) and their larvae as potential vectors for the transfer of Campylobacter jejuni and Salmonella enterica serovar paratyphi B variant Java between successive broiler flocks. Appl Environ Microbiol. (2008) 74:6887–91. doi: 10.1128/AEM.00451-08
62. Preiss FJ, Davidson JA. Characters for separating late-stage larvae, pupae, and adults of Alphitobius diaperinus and A. laevigatus (Coleoptera: Tenebrionidae). Ann Entomol Soc Am. (1970) 63:807–9. doi: 10.1093/aesa/63.3.807
63. USDA. Stored-Grain Insect Reference. Washington, DC: Federal Grain Inspection Service (2016). p. 64. Available online at: https://www.ams.usda.gov/sites/default/files/media/StoredGrainInsectsReference2017.pdf
64. Vlieger L, Brakefield PM, Müller C. Effectiveness of the defence mechanism of the turnip sawfly, Athalia rosae (Hymenoptera: Tenthredinidae), against predation by lizards. Bull Entomol Res. (2004) 94:283–9. doi: 10.1079/BER2004299
65. Vlieger L, Brakefield PM. The deflection hypothesis: eyespots on the margins of butterfly wings do not influence predation by lizards. Biol J Linn Soc. (2007) 92:661–7. doi: 10.1111/j.1095-8312.2007.00863.x
66. Van Rooij P, Martel A, D'Herde K, Brutyn M, Croubels S, Ducatelle R, et al. Germ tube mediated invasion of Batrachochytrium dendrobatidis in Amphibian skin is host dependent. PLoS ONE. (2012) 7:e41481. doi: 10.1371/journal.pone.0041481
67. Poma G, Cuykx M, Amato E, Calaprice C, Focant JF, Covaci A. Evaluation of hazardous chemicals in edible insects and insect-based food intended for human consumption. Food Chem. Toxicol. (2017) 100:70–9. doi: 10.1016/j.fct.2016.12.006
68. Lammers P, Ullmann LM, Fiebelkorn F. Acceptance of insects as food in Germany: is it about sensation seeking, sustainability consciousness, or food disgust? Food Qual Pref. (2019) 77:78–88. doi: 10.1016/j.foodqual.2019.05.010
69. Murefu TR, Macheka L, Musundire R, Manditsera FA. Safety of wild harvested and reared edible insects: a review Food Control. (2019) 101:209–24. doi: 10.1016/j.foodcont.2019.03.003
70. Watson C, Schlösser C, Vögerl J, Wichern F. Excellent excrement? Frass impacts on a soil's microbial community, processes and metal bioavailability. Appl Soil Ecol. (2021) 168:104110. doi: 10.1016/j.apsoil.2021.104110
71. Bonneau P. Contribution à la rédaction d'un catalogue des Ténébrionidae de France. L'Entomologiste. (1988) 44:201–12.
72. Zheng SZ, Zhan GH, Gao Y, Fan XH. Direct submission to NCBI on 23rd of August 2014. (2014). Available online at: https://www.ncbi.nlm.nih.gov/nuccore/KM435102.1 (accessed November 24, 2021).
73. Zheng SZ, Zhan GH, Gao Y, Fan XH. Direct submission to NCBI on 19th of September 2014. (2014). Available online at: https://www.ncbi.nlm.nih.gov/nuccore/KM652640.1 (accessed November 24, 2021).
74. Zheng SZ, Zhan GH, Gao Y, Fan XH. Direct submission to NCBI on 06th of January 2015. (2015). Available online at: https://www.ncbi.nlm.nih.gov/nuccore/KP410252.1 (accessed November 24, 2021).
Keywords: insect, Alphitobius diaperinus, lesser mealworm, Coleoptera, detection, real-time PCR, cadherin, feed
Citation: Marien A, Sedefoglu H, Dubois B, Maljean J, Francis F, Berben G, Guillet S, Morin J-F, Fumière O and Debode F (2022) Detection of Alphitobius diaperinus by Real-Time Polymerase Chain Reaction With a Single-Copy Gene Target. Front. Vet. Sci. 9:718806. doi: 10.3389/fvets.2022.718806
Received: 01 June 2021; Accepted: 10 January 2022;
Published: 09 March 2022.
Edited by:
Nils Th. Grabowski, Leibniz University Hannover, GermanyReviewed by:
Christos Athanassiou, University of Thessaly, GreeceChristos Rumbos, University of Thessaly, Greece
Copyright © 2022 Marien, Sedefoglu, Dubois, Maljean, Francis, Berben, Guillet, Morin, Fumière and Debode. This is an open-access article distributed under the terms of the Creative Commons Attribution License (CC BY). The use, distribution or reproduction in other forums is permitted, provided the original author(s) and the copyright owner(s) are credited and that the original publication in this journal is cited, in accordance with accepted academic practice. No use, distribution or reproduction is permitted which does not comply with these terms.
*Correspondence: Aline Marien, YS5tYXJpZW4mI3gwMDA0MDtjcmEud2FsbG9uaWUuYmU=