- 1Groupe de Recherche en Écologie Buccale, Faculté de Médecine Dentaire, Université Laval, Quebec City, QC, Canada
- 2Centre de Recherche en Infectiologie Porcine et Avicole, Fonds de Recherche du Québec–Nature et Technologies, Saint-Hyacinthe, QC, Canada
- 3Groupe de Recherche sur les Maladies Infectieuses du Porc, Faculté de Médecine Vétérinaire, Université de Montréal, Saint-Hyacinthe, QC, Canada
Actinobacillus pleuropneumoniae is the causal agent of porcine pleuropneumonia, a highly contagious and often deadly respiratory disease that causes major economic losses in the swine industry worldwide. The aim of the present study was to investigate the hydrogen peroxide (H2O2)-dependent antagonistic activity of Streptococcus pluranimalium 2N12 (pig nasal isolate) against A. pleuropneumoniae. A fluorimetric assay showed that S. pluranimalium produces H2O2 dose- and time-dependently. The production of H2O2 increased in the presence of exogenous lactate, suggesting the involvement of lactate oxidase. All 20 strains of A. pleuropneumoniae tested, belonging to 18 different serovars, were susceptible to H2O2, with minimal inhibitory concentrations and minimal bactericidal concentrations ranging from 0.57 to 2.3 mM. H2O2, as well as a culture supernatant of S. pluranimalium, killed planktonic cells of A. pleuropneumoniae. Treating the culture supernatant with catalase abolished its bactericidal property. H2O2 was also active against a pre-formed biofilm-like structure of A. pleuropneumoniae albeit to a lesser extent. A checkerboard assay was used to show that there were antibacterial synergistic interactions between H2O2 and conventional antibiotics, more particularly ceftiofur. Based on our results and within the limitations of this in vitro study, the production of H2O2 by S. pluranimalium could be regarded as a potential protective mechanism of the upper respiratory tract against H2O2-sensitive pathogens such as A. pleuropneumoniae.
Introduction
Actinobacillus pleuropneumoniae is a Gram-negative facultative anaerobic encapsulated coccobacillus. It is classified into two biotypes: biotype 1 requires nicotinamide adenine dinucleotide (NAD) to grow while biotype 2 is NAD independent (1). Based on their antigenic composition and the properties of their lipopolysaccharides (LPS) and capsular polysaccharides, nineteen serovars of A. pleuropneumoniae have been described to date (2, 3). This bacterium is transmitted from pig to pig mainly by direct oral and nasal contact or by bioaerosols following the introduction of asymptomatic carrier pigs into a herd or piggery (2). A. pleuropneumoniae is a respiratory pathogen that colonizes the tonsils and nasal cavities of pigs and causes porcine pleuropneumonia, a highly contagious and often deadly respiratory disease responsible for significant economic losses in the swine industry worldwide (1). This disease, for which the clinical features range from peracute to chronic, is characterized by the presence of hemorrhagic, fibrinous, and necrotic lung lesions. The serovars most often isolated from infections differ depending on the region of the world. In North America, serovars 5 and 7 are predominant while serovars 2 and 9/11 are most often isolated in European countries (2, 4–7). The use of antibiotics at the onset of the disease is the most effective treatment for diminishing the severity of the clinical symptoms, the death rate, and the spread of infections (1, 2).
A. pleuropneumoniae produces a broad array of virulence factors that play crucial roles in the infectious process of porcine pleuropneumonia by contributing to colonization, evasion of the immune defense mechanisms, and induction of lung lesions (2, 8, 9). The most important and well-known virulence factors are the Apx toxins (I to III), which belong to the family of Repeats in ToXin (RTX) toxins (9, 10). They cause the lysis of different cell types, including red blood cells, alveolar epithelial cells, neutrophils, and macrophages (9, 10).
A wide variety of bacterial species are present in the upper respiratory tract of pigs (11), and are likely to have positive or negative interactions with A. pleuropneumoniae. To the best of our knowledge, the negative interactions, such as competition and antagonism, that occur in the porcine respiratory tract and that involve A. pleuropneumoniae have not been thoroughly investigated to date. Antagonism is related to the ability of microorganisms to produce antimicrobial substances, including bacteriocins, organic acids, and hydrogen peroxide (H2O2) (12, 13). Preliminary analyses in our laboratory showed that Streptococcus pluranimalium 2N12, which was isolated from a pig nasal sample, is able to antagonize the growth of A. pleuropneumoniae in a deferred growth inhibition assay. In the present study, we investigated the H2O2-mediated antagonistic activity of S. pluranimalium 2N12 against A. pleuropneumoniae.
Materials and Methods
Bacteria and Growth Conditions
The A. pleuropneumoniae strains used in this study (Table 1) were grown in Todd Hewitt Broth (THB; BD-Canada, Mississauga, ON, Canada) supplemented with NAD (20 μg/mL; THB-NAD) and incubated in an anaerobic chamber (80% N2, 10% CO2, 10% H2) at 37°C. S. pluranimalium 2N12, which was isolated by our laboratory from the nasal cavity of a healthy pig, was identified by 16S rRNA gene sequencing. It was cultivated in THB and was incubated at 37°C in aerobic conditions.
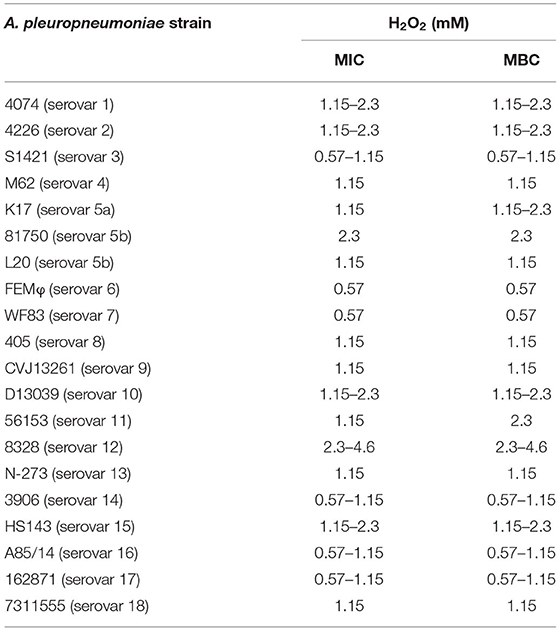
Table 1. Minimum inhibitory concentration (MIC) and minimum bactericidal concentration (MBC) values of H2O2 against A. pleuropneumoniae.
Deferred Growth Inhibition Assay for Detecting the Antagonistic Activity of S. pluranimalium Against A. pleuropneumoniae
Two-μL aliquots of an overnight broth culture of S. pluranimalium 2N12 were applied on the surface of THB agar plates. After a 24-h incubation at 37°C to allow bacterial growth, the plates were overlaid with soft THB-NAD agar (0.75%, w/v) that had been inoculated with an overnight culture of either A. pleuropneumoniae K17 (biotype 1, serovar 5a), 81750 (biotype 1, serovar 5b), or L20 (biotype 1, serovar 5b) (700 μL of bacterial culture in 7 mL of soft THB-NAD agar), and were incubated for a further 24 h at 37°C. The inhibitory zones (in mm) were measured from the edge of the S. pluranimalium growth to the margin of the inhibition area. The effect of adding catalase (100 units/mL) to the THB agar plates when growing S. pluranimalium was evaluated. Assays were performed in triplicate, and the means ± standard deviations (SD) were calculated.
Determination of H2O2 Production
The quantification of H2O2 production by S. pluranimalium 2N12 was assessed using the LAPTg medium described by Alvarez et al. (14). LAPTg medium contains 1.5% (w/v) peptone, 1% (w/v) tryptone, 1% (w/v) yeast extract, 1% (w/v) glucose, and 0.1% (v/v) Tween 80. The pH was adjusted to 6.5. Briefly, S. pluranimalium was cultivated overnight in LAPTg, harvested by centrifugation, and suspended in the same medium at an optical density at 660 nm (OD660) of 0.6 (~3 × 109 colony-forming units [CFU]/mL), 0.4, 0.2, and 0.1. The bacteria were incubated at 37°C with shaking (120 RPM), and samples were taken after 0, 1, and 2 h. In one experiment using an inoculum with an OD660 of 0.1, the incubation was extended to 24 h. H2O2 in the cell-free supernatants was determined using a fluorimetric H2O2 assay kit (Sigma-Aldrich Canada Co., Oakville, ON, Canada) according to the manufacturer's instructions. Assays were performed in triplicate in three independent experiments, and the means ± SD were calculated.
Effect of Lactate on H2O2 Production
The effect of exogenous lactate on H2O2 production by S. pluranimalium 2N12 was assessed. Lactate was added at concentrations ranging from 1 to 25 mM to the LAPTg medium, and the production of H2O2 was quantified after a 2-h incubation following inoculation (OD660 = 0.4) as described above. Assays were performed in triplicate in three independent experiments, and the means ± SD were calculated.
Determination of Minimum Inhibitory Concentrations and Minimum Bactericidal Concentrations of H2O2
Minimum inhibitory concentrations (MICs) and minimum bactericidal concentrations (MBCs) of H2O2 (Sigma-Aldrich Canada Co.) against twenty strains of A. pleuropneumoniae (Table 1) belonging to eighteen different serovars were determined as follows. Briefly, 24-h bacterial cultures (A. pleuropneumoniae) in THB-NAD were diluted in fresh broth medium to obtain an OD660 of 0.2. Equal volumes (100 μL) of bacteria and two-fold serial dilutions of 0.1% H2O2 (corresponding to 29.5 mM) in THB-NAD were mixed in the wells of a 96-well microplate. Control wells with no bacteria or no H2O2 were also prepared. The microplates were incubated (without agitation) at 37°C for 24 h in an anaerobic chamber prior to determining bacterial growth by recording the OD660 using an xMark Microplate spectrophotometer (Bio-Rad Laboratories Inc., Mississauga, ON, Canada). The MIC value was defined as the lowest concentration of H2O2 that completely prevented bacterial growth. The MBC values were determined by subculturing 5-μL aliquots from each well that exhibited no visible growth on THB-NAD agar plates, which were incubated for 48 h at 37°C in an anaerobic chamber. The MBC value was defined as the lowest concentration of H2O2 at which no colonies formed. Assays were performed in triplicate in three independent experiments.
Killing Assay of Planktonic A. pleuropneumoniae by H2O2 and a Culture Supernatant of S. pluranimalium
In a first assay, cells from overnight cultures of A. pleuropneumoniae 81750 and K17 were harvested by centrifugation and were suspended in LAPTg medium to obtain an OD660 of 0.4. The bacterial suspensions (2 mL) were mixed with 6 mL of H2O2 (in LAPTg medium) to obtain a final concentration of 2.3 mM. The mixtures were incubated anaerobically for 4 h at 37°C prior to assessing bacterial viability by determining the number of CFUs. In a second assay, S. pluranimalium 2N12 was cultivated overnight in THB, harvested by centrifugation, and suspended in LAPTg medium to an OD660 of 0.4. The bacterial suspension was incubated for 2 h with shaking at 37°C. The cell-free culture supernatant was then harvested, adjusted to pH 7.0, and filter-sterilized. The amount of H2O2 in the supernatant was quantified using the fluorimetric assay described above. The A. pleuropneumoniae suspensions (2 mL, OD660 of 0.4) were mixed with 6 mL of the S. pluranimalium culture supernatant. The mixture was anaerobically incubated at 37°C for 4 h, and bacterial viability was assessed by determining the CFUs. The effect of pre-treating the culture supernatant of S. pluranimalium with catalase (100 units/mL) for 1 h at room temperature prior to performing the assay was assessed. All the assays were performed in triplicate, and the means ± SD were calculated. Given the above viable count methodology, the minimum detection limit is 101 CFU/mL.
Killing Assay of a Biofilm-Like Structure of A. pleuropneumoniae by H2O2 and a Culture Supernatant of S. pluranimalium
A. pleuropneumoniae 81750 and K17 biofilm-like structures were pre-formed overnight (THB-NAD, 37°C, anaerobic chamber) in the wells of a 96-well clear bottom black wall microplate (Greiner Bio-One North America, Monroe, NC, USA). After removing planktonic and loosely-attached bacteria by aspiration, the biofilm-like structures were exposed (4 h, 37°C, anaerobic chamber) to either H2O2 (0.57, 1.15, 2.3, and 4.6 mM) or a culture supernatant of S. pluranimalium 2N12 prepared as described above. Biofilm-like structure viability was assessed using the FilmTracer LIVE/DEAD Biofilm Viability kit (Life Technologies Corporation, Eugene, OR, USA) according to the manufacturer's protocol. Assays were performed in triplicate, and the means ± SD were calculated.
Effect of H2O2 on the Activity of Conventional Antibiotics Against A. pleuropneumoniae
Interactions between H2O2 and conventional antibiotics (ceftiofur, penicillin G, tetracycline) used to treat A. pleuropneumoniae infections were evaluated using the checkerboard technique (15). H2O2 (from 4.6 mM) was serially diluted two-fold in THB-NAD (100 μL) along the ordinate of a 96-well microplate, while antibiotics (from 2 μg/mL) were serially diluted two-fold in THB-NAD (100 μL) along the abscissa. A cell suspension of A. pleuropneumoniae 81750 prepared in THB-NAD and adjusted to an OD660 of 0.2 was used as the inoculum. The microplate wells were inoculated with 100 μL of bacterial suspension, and the microplates were incubated at 37°C for 24 h. Wells with no bacteria or compounds were used as controls. After the incubation period, bacterial growth was assessed visually. The lowest concentration at which no growth occurred was considered the MIC. The fractional inhibitory concentration index (FICI) was calculated as follows: FICI = FICA + FICB = (MICH2O2 in combination/MICH2O2 alone) + (MICAntibiotic in combination/MICAntibiotic alone). An FICI ≤ 0.5 was considered as having a synergistic effect, an FICI > 0.5 and ≤ 1.0 as having an additive effect, an FICI > 1.0 and ≤ 4.0 as having no effect, and an FICI > 4.0 as having an antagonistic effect (15). Two independent assays were performed.
Effect of Catalase on the Growth of and Biofilm Formation by S. pluranimalium
The effect of catalase (6.25, 12.5, 25, 50, 100, and 200 units/mL) on the growth of and biofilm formation by S. pluranimalium 2N12 was assessed in a 96-well microplate. Bacteria were cultivated for 20 h, and growth was monitored by recording the OD660 using a Synergy 2 microplate reader (BioTek Instruments, Winooski, VT, USA). Planktonic and poorly-attached bacterial cells were then removed by aspiration with a 26G needle. Biofilms were stained with 100 μL of 0.01% crystal violet for 15 min, washed with distilled water, and dried at 37°C for 2 h. To release biofilm-embedded crystal violet, 100 μL of 75% ethanol was added, and the plate was shaken for 15 min. The biofilm biomass was estimated by recording the absorbance at 550 nm (A550) using an xMark Microplate spectrophotometer. Assays were performed in triplicate in three independent experiments, and the means ± SD were calculated.
Statistical Analysis
Statistical analyses were performed using a one-way ANOVA analysis of variance with a post-hoc Bonferroni multiple comparison test (GraphPad Software Inc., San Diego, CA, USA). All results were considered statistically significant at p < 0.01.
Results
The ability of S. pluranimalium 2N12 to exert an antagonistic effect on A. pleuropneumoniae was first examined using a deferred growth inhibition assay. As reported in Table 2, A. pleuropneumoniae 81750 and L20 strains, which belong to serovar 5b, displayed inhibitory zones of 4.0 mm and 6.3 mm, respectively. A. pleuropneumoniae K17 (serovar 5a) appeared less sensitive, showing an inhibitory zone of 1.3 mm. The deferred growth inhibition assay was then performed in the presence of catalase to determine whether the inhibition of A. pleuropneumoniae may have resulted from the production of H2O2 by S. pluranimalium. Adding catalase (100 units/mL) resulted in a significant reduction or complete abolition of the inhibitory zones (Table 2).
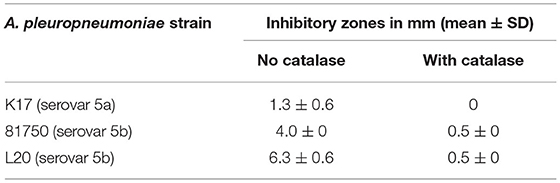
Table 2. Inhibitory zones produced by S. pluranimalium 2N12 against A. pleuropneumoniae in a deferred growth inhibition assay.
As H2O2 is a potential inhibitory compound that is active against A. pleuropneumoniae, its production by S. pluranimalium 2N12 was confirmed by quantifying H2O2 levels in a culture supernatant (LAPTg medium) using a fluorimetric assay. As shown in Figure 1A, S. pluranimalium dose- and time-dependently produced H2O2. A low bacterial inoculum (OD660 of 0.1) resulted in the production of 56.3 μM of H2O2 after a 2-h incubation, while the use of a higher bacterial inoculum (OD660 of 0.6) was associated with the production of 845.5 μM of H2O2, a 15-fold increase compared to that of the low bacterial inoculum. It was then determined whether a longer incubation time for the low bacterial inoculum (OD660 of 0.1) resulted in the production of higher amounts of H2O2. The results reported in Figure 1B show that extending the incubation to 24 h was associated with lower amounts of H2O2 compared to the 6-h incubation time.
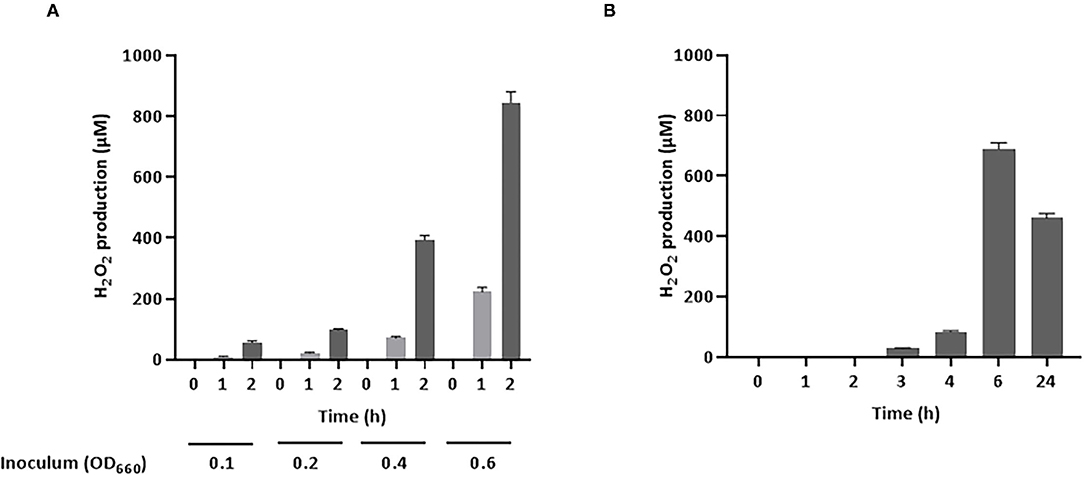
Figure 1. Production of H2O2 by S. pluranimalium 2N12. (A): Dose- and time-dependent effect. (B): Time-dependent effect. H2O2 was quantified using a fluorimetric assay. Assays were performed in triplicate in two independent experiments, and the means ± SD were calculated.
Lactate oxidase is a bacterial enzyme that converts lactate into pyruvate and H2O2. Figure 2 indicates that exogenous lactate added to the LAPTg culture medium dose-dependently increased the amount of H2O2 produced by S. pluranimalium. More specifically, the presence of 25 mM lactate increased the production of H2O2 1.57-fold compared to the control (no lactate), suggesting that the production of H2O2 by S. pluranimalium relies, at least in part, on the activity of lactate oxidase.
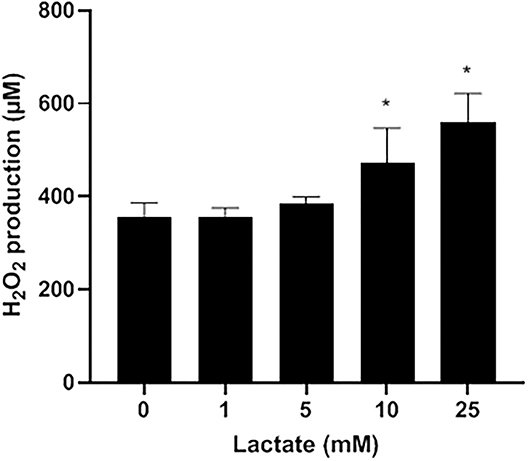
Figure 2. Dose-dependent effect of exogenous lactate on the production of H2O2 by S. pluranimalium 2N12. H2O2 was quantified using a fluorimetric assay. Assays were performed in triplicate in two independent experiments, and the means ± SD were calculated. *Significantly different at p < 0.01 compared to the control (no lactate).
The antibacterial activity of H2O2 against A. pleuropneumoniae strains belonging to eighteen different serovars was evaluated by determining the MIC and MBC values. As reported in Table 1, all the strains tested had MICs and MBCs ranging from 0.57 mM (corresponding to 0.002% [v/v]) to 2.3 mM (corresponding to 0.008% [v/v]) for H2O2.
The killing of planktonic A. pleuropneumoniae (strains 81750 and K17) caused by either H2O2 or a culture supernatant of S. pluranimalium (treated or not with catalase) after a 4-h incubation was monitored. As reported in Figure 3A, H2O2 used at a concentration of 2.3 mM, which corresponded to the MBC, reduced the viability of A. pleuropneumoniae 81750 (initial concentration: 5 × 106 CFU/mL) and K17 (initial concentration: 4.45 × 106 CFU/mL) below the minimum detection limit (101 CFU/mL). On the other hand, in the control assay (no H2O2), the bacterial concentrations increased slightly. Similarly, a culture supernatant of S. pluranimalium (containing 0.5 mM H2O2) significantly reduced the number of planktonic A. pleuropneumoniae cells. Figure 3B shows that the CFUs for A. pleuropneumoniae 81750 dropped from 4.45 × 106 CFU/mL to 2 ± 0.6 × 103 CFU/mL, and from 3.4 ± 0.4 × 107 CFU/mL to below the minimum detection limit (101 CFU/mL) for A. pleuropneumoniae K17. Treating the culture supernatant of S. pluranimalium with catalase, which led to a residual concentration of 0.04 mM H2O2, completely abolished its bactericidal activity.
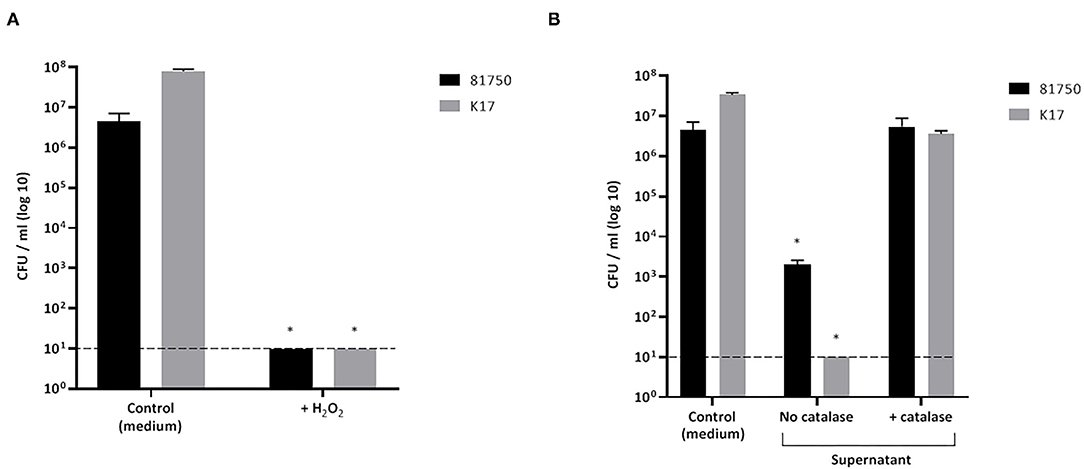
Figure 3. Effect of H2O2 at the minimal bactericidal concentration (A) and a culture supernatant of S. pluranimalium 2N12 treated or not with catalase (B) on the killing of planktonic cells of A. pleuropneumoniae 81750 and K17. Bacterial viability was assessed by determining colony-forming units (CFU) after an exposure time of 4 h. The broken horizontal line indicates the minimum detection limit (101 CFU/mL). Assays were performed in triplicate in three independent experiments, and the means ± SD were calculated. *Significantly different at p < 0.01 compared to the control (no H2O2 or no S. pluranimalium culture supernatant).
The ability of H2O2 and the culture supernatant of S. pluranimalium to cause the killing and desorption of biofilm-like structures of A. pleuropneumoniae 81750 and K17 following a 4-h treatment was then assessed. When used at a high concentration (MBC or two-fold MBC), H2O2 slightly but significantly decreased the viability of both A. pleuropneumoniae biofilm-like structures (Figure 4A). More specifically, at two-fold MBC (4.6 mM), viability was reduced by 11.5 ± 1.4% and 8.3 ± 2.7% for strains 81750 and K17, respectively. While H2O2 did not induce biofilm-like structure desorption for A. pleuropneumoniae 81750, it caused a dose-dependently desorption of the A. pleuropneumoniae K17 biofilm-like structure (Figure 4B). At two-fold MBC (4.6 mM), the biofilm-like biomass was reduced by 64.4 ± 1.3%. The same effects on A. pleuropneumoniae biofilm-like structure killing and desorption were investigated using the culture supernatant of S. pluranimalium containing 0.5 mM H2O2. As reported in Figure 5, no significant effects were observed.
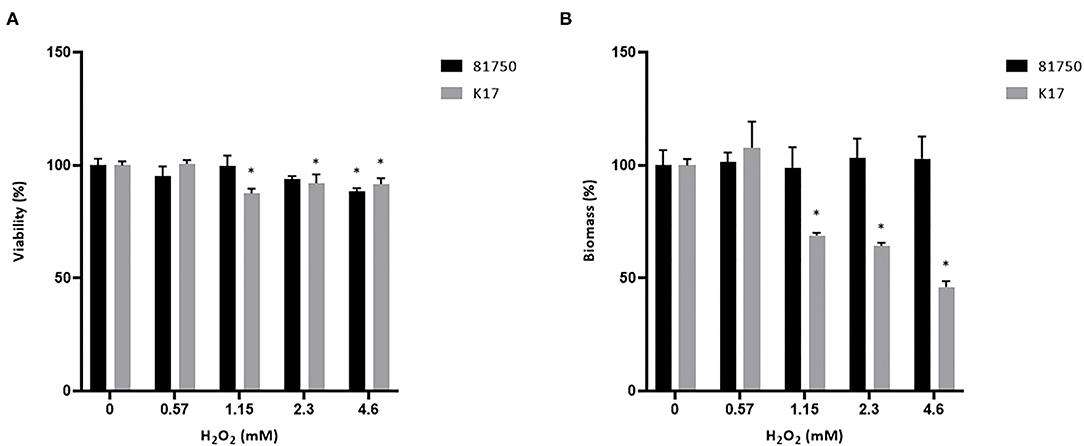
Figure 4. Dose-dependent effect of H2O2 on the viability (A) and biomass (B) of A. pleuropneumoniae 81750 and K17 biofilm-like structures following an exposure time of 4 h. Viability was assessed using the FilmTracer LIVE/DEAD Biofilm Viability kit. Biofilm-like structure biomass was determined by crystal violet staining. Assays were performed in triplicate in three independent experiments, and the means ± SD were calculated. *Significantly different at p < 0.01 compared to the control (no H2O2).
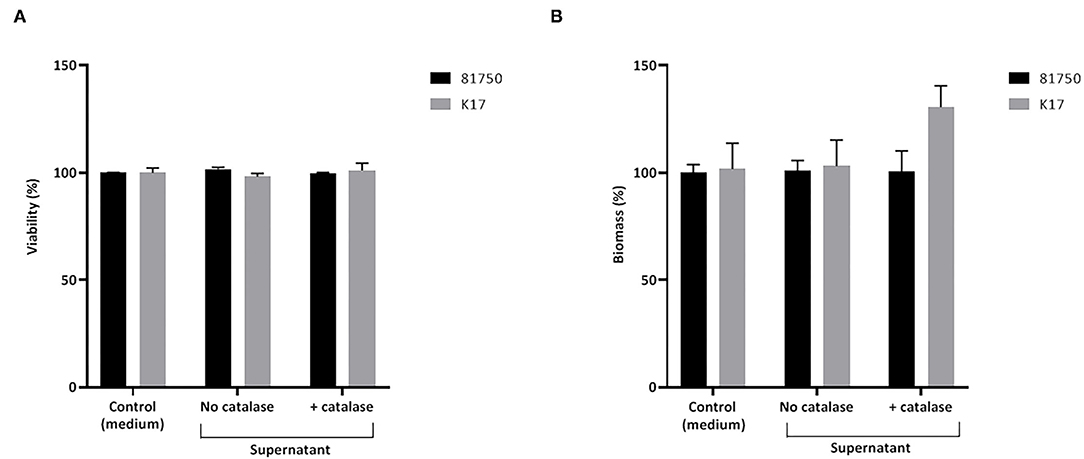
Figure 5. Effect of a culture supernatant of S. pluranimalium 2N12, treated or not with catalase, on the viability (A) and biomass (B) of A. pleuropneumoniae 81750 and K17 biofilms. Biofilm viability was assessed using the FilmTracer LIVE/DEAD Biofilm Viability kit. Biofilm biomass was determined by crystal violet staining. Assays were performed in triplicate in three independent experiments, and the means ± SD were calculated.
The effect of H2O2 on the activity of conventional antibiotics, including ceftiofur, penicillin G, and tetracycline, against A. pleuropneumoniae 81750 and K17 was then evaluated. As reported in Table 3, synergistic effects were observed for both strains when H2O2 was used in combination with ceftiofur. Synergistic interactions were only observed for strain K17 with the H2O2/penicillin G and H2O2/tetracycline combinations.
Preliminary assays showed that S. pluranimalium has the ability to form a biofilm. We thus investigated whether H2O2 contributes to biofilm formation by growing S. pluranimalium in the presence of various amounts of catalase. On the one hand, the presence of catalase increased the bacterial growth rate, and the highest concentration tested (200 units/mL) also appeared to increase the final biomass as shown by the higher final OD660 (Figure 6A). On the other hand, the presence of catalase dose-dependently reduced biofilm formation (Figure 6B). More specifically, when added at a concentration of 200 units/mL, catalase decreased the formation of a biofilm by 76.3 ± 1.3%.
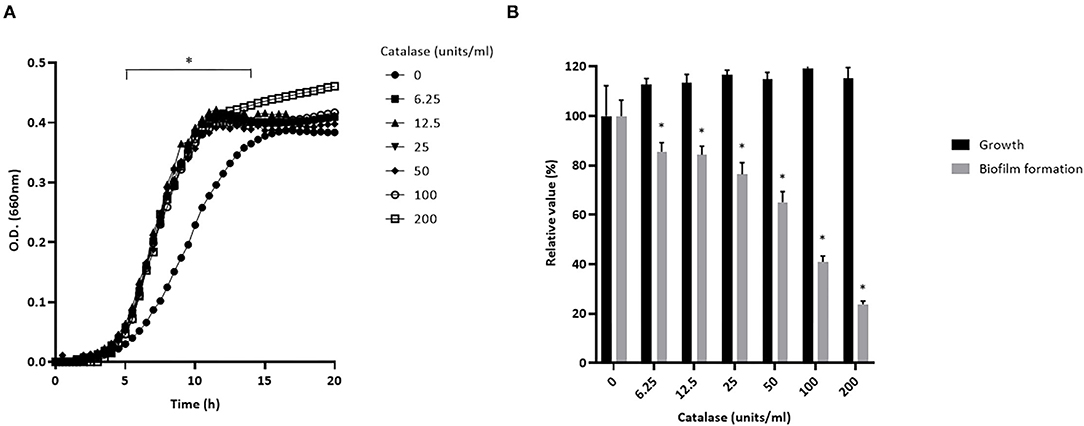
Figure 6. Dose-dependent effect of catalase on the growth (A) and biofilm formation (B) of S. pluranimalium 2N12. Bacterial growth was monitored by recording the optical density at 660 nm (OD660). Biofilm biomass was quantified by crystal violet staining. Assays were performed in triplicate in three independent experiments, and the means ± SD were calculated. *Significantly different at p < 0.01 compared to the control (no catalase).
Discussion
A large number of different microorganisms reside in the upper respiratory tract of pigs (11). In the healthy state, these microbial communities live in homeostasis and it can be hypothesized that they protect the animals against external pathogens that may reach this site. This protective effect may rely on the ability of certain commensal bacteria to produce antimicrobial compounds such as bacteriocins, organic acids, and hydrogen peroxide that are active against pathogenic microorganisms (12, 13). In the present study, we investigated the antagonistic activity of S. pluranimalium 2N12 against A. pleuropneumoniae.
We first confirmed an antimicrobial activity of S. pluranimalium 2N12 against A. pleuropneumoniae, which was related to H2O2 production. This is supported by the fact that the ability of a culture supernatant of S. pluranimalium 2N12 to induce the killing of planktonic cells of A. pleuropneumoniae was totally eliminated following a treatment with catalase. Moreover, commercial H2O2 was highly bactericidal for all serovars of A. pleuropneumoniae tested, with MBC values ranging from 0.57 to 2.3 mM. The deleterious effects of H2O2 on A. pleuropneumoniae may be linked to the generation of hydroxyl radicals in the presence of Fe(II) once it enters the cells (16), which results in the oxidation of macromolecules such as DNA and proteins.
Lactate oxidase (LctO) is a common H2O2-producing enzyme in bacteria that catalyzes the formation of pyruvate and H2O2 from lactate and oxygen (17). In the present study, the addition of exogenous lactate increased the production of H2O2 by S. pluranimalium, providing support for the key role of LctO. However, other pathways such as those involving pyruvate oxidase and amino acid oxidase (17), which may also contribute to generating H2O2, should not be excluded. Studies are currently in progress in our laboratory to investigate how environmental parameters modulate H2O2 production by S. pluranimalium.
As H2O2 is a harmful byproduct of aerobic metabolism and as S. pluranimalium does not produce catalase, it must possess a molecular mechanism to neutralize H2O2. Similarly to S. pluranimalium, Streptococcus pneumoniae produces large amounts of H2O2 as a byproduct of its metabolism (18). Several mechanisms have been suggested to explain how S. pneumoniae defends against the H2O2-mediated oxidative stress, including (i) scarcity of proteins with iron-sulfur clusters which can be damaged by reactive oxygen species (ROS) in an iron-dependent manner, (ii) expression of ferritin-like proteins known as Dps (DNA-binding protein from starved cells) or Dpr (Dps-like peroxide resistance), and (iii) production of thiol peroxidase (TpxD) activity (18). The exact mechanism that allows protection of S. pluranimalium from H2O2 needs to be characterized.
Evidence was brought that H2O2 production by S. pluranimalium is involved in its ability to form a biofilm given that the presence of catalase dose-dependently prevented biofilm formation. H2O2-mediated biofilm formation has been previously reported for Streptococcus sanguinis and Streptococcus gordonii, which are primary colonizers of human dental biofilms (19, 20). It has been proposed that H2O2 induces the release of extracellular bacterial DNA, without autolysis, that promotes cell-to-cell adhesion and biofilm formation (20).
A. pleuropneumoniae has the ability to form a biofilm (21, 22) that enhances its resistance to antibiotics compared to planktonic cells (23). We showed that treating a pre-formed biofilm-like structure of A. pleuropneumoniae with H2O2 at a concentration corresponding to the MBC (2.3 mM) reduces its viability. No killing of the biofilm-like structure was obtained with a culture supernatant of S. pluranimalium, which is likely related to the fact that the supernatant contained a low amount of H2O2 (0.5 mM). Additional studies are required to demonstrate the killing effect of H2O2 using more relevant biofilm models of A. pleuropneumoniae.
The effect of H2O2 on the activity of conventional antibiotics used to treat A. pleuropneumoniae infections was assessed using the checkerboard technique. Synergistic interactions between H2O2 and some antibiotics, including ceftiofur, penicillin G, and tetracycline, were demonstrated, particularly against A. pleuropneumoniae K17. This observation was in agreement with the study of Sgibnev and Kremleva (24) who investigated the influence of various microbial metabolites on the antibiotic sensitivity of bacteria. The authors reported that H2O2 was the most effective bacterial metabolite for increasing the sensitivity of both Gram-positive and Gram-negative bacteria to several antibiotics. They proposed that H2O2 may cause a shift in the balance of pro-oxidants and antioxidants in bacteria and that the resulting oxidative stress enhances the effects of antibiotics on the target bacteria.
Based on our results, the production of H2O2 by S. pluranimalium could be regarded as a potential protection mechanism of the upper respiratory tract against H2O2-sensitive pathogens such as A. pleuropneumoniae. Interestingly, previous studies have documented the role of H2O2 produced by commensal streptococci colonizing the oral cavity in controlling cariogenic bacteria (mainly Streptococcus mutans) (25–27). It is worth mentioning that the presence of catalase positive staphylococci in the upper respiratory tract of pigs (11) may attenuate the beneficial impact of H2O2.
The resistance of A. pleuropneumoniae to various antibiotics, including tetracycline, ampicillin, and penicillin, is on the increase and is a growing concern (28–30). The identification of alternative strategies for controlling A pleuropneumoniae infections is thus of great interest. In this regard, further studies are required to explore the possibility of using S. pluranimalium as a probiotic to antagonize respiratory pathogens such as A. pleuropneumoniae.
Conclusions
Based on our results, the production of H2O2 by S. pluranimalium could be regarded as a potential protective mechanism of the upper respiratory tract against H2O2-sensitive pathogens such as A. pleuropneumoniae.
Data Availability Statement
The original contributions presented in the study are included in the article/supplementary material, further inquiries can be directed to the corresponding author.
Author Contributions
MF, MG, and DG conceived and designed the experiments. KV performed the experimental assays and the statistical analysis. DG drafted and finalized the manuscript. All authors contributed to the article and approved the submitted version.
Funding
This study was supported by Fonds de recherche du Québec—Nature et technologies (2018-PR-205462).
Conflict of Interest
The authors declare that the research was conducted in the absence of any commercial or financial relationships that could be construed as a potential conflict of interest.
Publisher's Note
All claims expressed in this article are solely those of the authors and do not necessarily represent those of their affiliated organizations, or those of the publisher, the editors and the reviewers. Any product that may be evaluated in this article, or claim that may be made by its manufacturer, is not guaranteed or endorsed by the publisher.
References
1. Gottschalk M. Actinobacillosis. In: Karriker L, Ramirez A, Schwartz K, Stevenson G, Zimmerman J. editors. Diseases of Swine. 10th ed. Hoboken, NJ: Wiley (2012). p. 653–69.
2. Sassu EL, Bossé JT, Tobias TJ, Gottschalk M, Langford PR, Hennig-Pauka I. Update on Actinobacillus pleuropneumoniae–knowledge, gaps and challenges. Transbound Emerg Dis. (2017) 65:72–90. doi: 10.1111/tbed.12739
3. Stringer OW, Bossé JT, Lacouture S, Gottschalk M, Fodor L, Angen Ø, et al. Proposal of Actinobacillus pleuropneumoniae serovar 19, and reformulation of previous multiplex PCRs for capsule-specific typing of all known serovars. Vet Microbiol. (2021) 255:109021. doi: 10.1016/j.vetmic.2021.109021
4. Lacouture S, Gottschalk M. Distribution of Actinobacillus pleuropneumoniae (from 2015 to June 2020) and Glaesserella parasuis (from 2017 to June 2020) serotypes isolated from diseased pigs in Quebec. Can Vet J. (2020) 61:1261–3.
5. Dubreuil JD, Jacques M, Mittal KR, Gottschalk M. Actinobacillus pleuropneumoniae surface polysaccharides: their role in diagnosis and immunogenicity. Anim Health Res Vet. (2000) 1:73–93. doi: 10.1017/S1466252300000074
6. Schumerk L, Hoeltig D, Waldmann KH, Valentin-Weigand P, Rohde J. Sero- and apx-typing of German Actinobacillus pleuropneumoniae field isolates from 2010 to 2019 reveals a predominance of serovar 2 with regular apx-profile. Vet Res. (2021) 52:10. doi: 10.1186/s13567-020-00890-x
7. Sarkozi R, Makrai L, Fodor L. Actinobacillus pleuropneumoniae serotypes in Hungary. Acta Vet Hung. (2018) 66:343–9. doi: 10.1556/004.2018.031
8. Bossé JT, Janson H, Sheehan BJ, Beddek AJ, Rycroft AN, Kroll JS, et al. Actinobacillus pleuropneumoniae: pathobiology and pathogenesis of infection. Microbes Infect. (2002) 4:225–35. doi: 10.1016/S1286-4579(01)01534-9
9. Chiers K, De Waele T, Pasmans F, Ducatelle R, Haesebrouck F. Virulence factors of Actinobacillus pleuropneumoniae involved in colonization, persistence and induction of lesions in its porcine host. Vet Res. (2010) 41:65. doi: 10.1051/vetres/2010037
10. Schaller A, Kuhnert P. de la Puente-Redondo VA, Nicolet J, Frey J. Apx toxins in Pasteurellaceae species from animals. Vet Microbiol. (2000) 74:365–76. doi: 10.1016/S0378-1135(00)00204-2
11. Pirolo M, Espinosa-Gongora C, Bogaert D, Guardabassi L. The porcine respiratory microbiome: recent insights and future challenges. Anim Microbiome. (2021) 3:9. doi: 10.1186/s42523-020-00070-4
12. Kanmani P, Satish Kumar R, Yuvaraj N, Paari KA, Pattukumar V, Arul V. Probiotics and its functionally valuable products–a review. Crit Rev Food Sci Nut. (2013) 53:641–58. doi: 10.1080/10408398.2011.553752
13. Khaneghah AM, Abhari K, Es I, Soares MB, Oliveira RBA, Hosseini H, et al. Sant'Ana AS. Interactions between probiotics and pathogenic microorganisms in hosts and food: A review. Trends Food Sci Technol. (2020) 95:205–18. doi: 10.1016/j.tifs.2019.11.022
14. Alvarez MF, Medina R, Pasteris SE, Strasser de Saad AM, Sesma F. Glycerol metabolism of Lactobacillus rhamnosus ATCC 7469: cloning and expression of two glycerol kinase genes. J Mol Microbiol Biotechnol. (2004) 7:170–81. doi: 10.1159/000079826
15. Eliopoulos GM, Moellering RC. Antimicrobial combinations. In: Lorian V, editor. Antibiotics in Laboratory Medicine. Baltimore, MD: The Williams and Wilkins Co. (1996), p. 330–96.
16. Imlay JA. Pathways of oxidative damage. Annu Rev Microbiol. (2003) 57:395–418. doi: 10.1146/annurev.micro.57.030502.090938
17. Redanz S, Cheng X, Giacaman RA, Pfeifer CS, Merritt J, Kreth J. Live and let die: hydrogen peroxide production by the commensal flora and its role in maintaining a symbiotic microbiome. Mol Oral Microbiol. (2018) 33:337–52. doi: 10.1111/omi.12231
18. Yesilkaya H, Farshchi Andisi V, Andrew PW, Bijlsma JJE. Streptococcus pneumoniae and reactive oxygen species: an unsual approach to living with radicals. Trends Microbiol. (2013) 21:187–95. doi: 10.1016/j.tim.2013.01.004
19. Duan D, Scoffield JA, Zhou X, Wu H. Fine-tuned production of hydrogen peroxide promotes biofilm formation of Streptococcus parasanguinis by a pathogenic cohabitant Aggregatibacter actinomycetemcomitans. Environ Microbiol. (2016) 18:4023–36. doi: 10.1111/1462-2920.13425
20. Kreth J, Vu H, Zhang Y, Herzberg MC. Characterization of hydrogen peroxide-induced DNA release by Streptococcus sanguinis and Streptococcus gordonii. J Bacteriol. (2009) 191:6281–91. doi: 10.1128/JB.00906-09
21. Hathroubi S, Loera-Muro A, Guerrero-Barrera AL, Tremblay YND, Jacques M. Actinobacillus pleuropneumoniae biofilms: role in pathogenicity and potential impact for vaccination development. Anim Health Res Rev. (2017) 19:17–30. doi: 10.1017/S146625231700010X
22. Labrie J, Pelletier-Jacques G, Deslandes V, Ramjeet M, Auger E, Nash JHE, et al. Effects of growth conditions on biofilm formation by Actinobacillus pleuropneumoniae. Vet Res. (2010) 41:03. doi: 10.1051/vetres/2009051
23. Izano EA, Sadovskaya I, Vinogradov E., Mulks MH, Velliyagounder K, Ragunath C, et al. Poly-N-acetylglucosamine mediates biofilm formation and antibiotic resistance in Actinobacillus pleuropneumoniae. Microb Pathog. (2007) 43:1–9. doi: 10.1016/j.micpath.2007.02.004
24. Sgibnev A, Kremleva E. Influence of hydrogen peroxide, lactic acid, and surfactants from vaginal lactobacilli on the antibiotic sensitivity of opportunistic bacteria. Probiotics Antimicrob Proteins. (2017) 9:131–41. doi: 10.1007/s12602-016-9238-6
25. Giacaman RA, Torres S, Gomez Y, Munoz-Sandoval C, Kreth J. Correlation of Streptococcus mutans and Streptococcus sanguinis colonization and ex vivo hydrogen peroxide production in carious lesion-free and high caries adults. Arch Oral Biol. (2015) 60:154–9. doi: 10.1016/j.archoralbio.2014.09.007
26. Herrero ER, Slomka V, Bernaerts K, Boon N, Hernandez-Sanabria E, Passoni BB, et al. Teughels. Anti-microbial effects pf commensal oral species are regulated by environmental factors. J Dent. (2016) 47:23–33. doi: 10.1016/j.jdent.2016.02.007
27. Kreth J, Zhang Y, Herzberg MC. Streptococcal antagonism in oral biofilms: Streptococcus sanguinis and Streptococcus gordonii interference with Streptococcus mutans. J Bacteriol. (2008) 190:4632–40. doi: 10.1128/JB.00276-08
28. Archambault M, Harel J, Goure J, Tremblay YD, Jacques M. 2012. Antimicrobial susceptibilities and resistance genes of Canadian isolates of Actinobacillus pleuropneumoniae. Microb Drug Resist. (2012) 18:198–206. doi: 10.1089/mdr.2011.0150
29. Michael GB, Bossé TJ, Schwarz S. 2017. Antimicrobial resistance in Pasteurellaceae of veterinary origin Microbiol Spectr. (2012) 6:3. doi: 10.1128/microbiolspec.ARBA-0022-2017
Keywords: Actinobacillus pleuropneumoniae, Streptococcus pluranimalium, porcine pleuropneumonia, swine infection, hydrogen peroxide, antagonism
Citation: Vaillancourt K, Frenette M, Gottschalk M and Grenier D (2021) Streptococcus pluranimalium 2N12 Exerts an Antagonistic Effect Against the Swine Pathogen Actinobacillus pleuropneumoniae by Producing Hydrogen Peroxide. Front. Vet. Sci. 8:787241. doi: 10.3389/fvets.2021.787241
Received: 30 September 2021; Accepted: 16 November 2021;
Published: 08 December 2021.
Edited by:
Fabrizio Bertelloni, University of Pisa, ItalyReviewed by:
Patrick Blackall, The University of Queensland, AustraliaCiro Cesar Rossi, Federal University of Rio de Janeiro, Brazil
Andrew N. Rycroft, Royal Veterinary College (RVC), United Kingdom
Copyright © 2021 Vaillancourt, Frenette, Gottschalk and Grenier. This is an open-access article distributed under the terms of the Creative Commons Attribution License (CC BY). The use, distribution or reproduction in other forums is permitted, provided the original author(s) and the copyright owner(s) are credited and that the original publication in this journal is cited, in accordance with accepted academic practice. No use, distribution or reproduction is permitted which does not comply with these terms.
*Correspondence: Daniel Grenier, ZGFuaWVsLmdyZW5pZXJAZ3JlYi51bGF2YWwuY2E=