- 1Departmento de Cirurgia Veterinária e Reprodução Animal, Faculdade de Medicina Veterinária e Zootecnia, Universidade Estadual Paulista, Botucatu, Brazil
- 2Department of Clinical Studies, Ontario Veterinary College, University of Guelph, Guelph, ON, Canada
Resuscitative fluid therapy aims to increase stroke volume (SV) and cardiac output (CO) and restore/improve tissue oxygen delivery in patients with circulatory failure. In individualized goal-directed fluid therapy (GDFT), fluids are titrated based on the assessment of responsiveness status (i.e., the ability of an individual to increase SV and CO in response to volume expansion). Fluid administration may increase venous return, SV and CO, but these effects may not be predictable in the clinical setting. The fluid challenge (FC) approach, which consists on the intravenous administration of small aliquots of fluids, over a relatively short period of time, to test if a patient has a preload reserve (i.e., the relative position on the Frank-Starling curve), has been used to guide fluid administration in critically ill humans. In responders to volume expansion (defined as individuals where SV or CO increases ≥10–15% from pre FC values), FC administration is repeated until the individual no longer presents a preload reserve (i.e., until increases in SV or CO are <10–15% from values preceding each FC) or until other signs of shock are resolved (e.g., hypotension). Even with the most recent technological developments, reliable and practical measurement of the response variable (SV or CO changes induced by a FC) has posed a challenge in GDFT. Among the methods used to evaluate fluid responsiveness in the human medical field, measurement of aortic flow velocity time integral by point-of-care echocardiography has been implemented as a surrogate of SV changes induced by a FC and seems a promising non-invasive tool to guide FC administration in animals with signs of circulatory failure. This narrative review discusses the development of GDFT based on the FC approach and the response variables used to assess fluid responsiveness status in humans and animals, aiming to open new perspectives on the application of this concept to the veterinary field.
Introduction
Patients that are critically ill or that are undergoing major surgery are frequently presented with inadequate tissue oxygen delivery due to poor circulating volume. Under these conditions, rapid volume expansion via intravenous fluid administration should optimize tissue perfusion but such benefit is not without risks. Iatrogenic hypervolemia due to a liberal fluid administration strategy causes tissue/lung edema and worsens patient outcome (1). Otherwise, insufficient fluid administration due to a restrictive fluid therapy strategy can perpetuate hypovolemia/poor tissue perfusion and increase the rate of complications such as renal failure and death (1). According to guidelines published in veterinary medicine, when signs of circulatory failure/hypovolemia are present, aggressive fluid administration can be initiated via rapid administration of isotonic crystalloids using 25% of the “shock dose” (15–20 ml/kg in dogs and 10 mL/kg in cats), while monitoring the patient's the response (e.g., blood pressure, capillary refill time, lung sounds) (2). Based on the response to initial volume expansion, additional aliquots (25% of shock dose) can be administered, or a synthetic colloid can be considered if 50% of crystalloid shock volume did not cause sufficient improvement (2). However, there are opened questions regarding the goals and endpoints that should guide volume resuscitation in animals.
In the human medical field, fluid resuscitation guided by assessment of fluid responsiveness [i.e., the ability of an individual to increase stroke volume (SV) and cardiac output (CO) in response to volume expansion] appears to reduce patient mortality, duration of stay in the intensive care unit (ICU), and duration of mechanical ventilation (3). The use of a fluid challenge approach (i.e., the rapid administration of a relatively small amount of fluids) guided assessment of fluid responsiveness status allows rapid replacement of intravascular volume deficits as long as the individual has a cardiac preload reserve (i.e., is positioned on the ascending limb of the Frank-Starling curve). Because additional amounts of fluids are administered only if the individual presents an improvement in SV and CO, the fluid challenge approach can improve tissue perfusion and minimize the risks associated with hypervolemia and fluid overload (1, 3). This article will review the physiological basis of goal-directed fluid therapy (GDFT), the fluid challenge approach for treating circulatory failure, and the target variables used to evaluate the response to volume resuscitation. Most of the current literature originates from research on the human side, whether conducted in the clinical setting, or from bench research in animals. More recently, veterinary studies have used this information in several animal species. This article aims to critically review the development of the fluid challenge approach in human and veterinary medicine, with the objective of shedding light on the possible applications of this concept to the veterinary field.
References from PubMed database since January 2000 were included in this narrative review. Primary search terms were fluid challenge, GDFT, and fluid responsiveness. Human and animal studies (dogs and cats, and other species if relevant) involving the above mentioned terms were selected. To approach the target variables used to assess the response to a fluid challenge, studies comparing newer CO measurement technologies with reference methods of CO measurement in humans, dogs, and cats were selected. Studies published before 2000 considered seminal for understanding the current state of knowledge were also included in the review.
The Physiological Basis of Goal Directed Fluid Therapy
The venous system is composed of highly compliant capacitance vessels (i.e., vessels that can accommodate a large volume of blood without a large increase in intravascular pressure) which contain ~70% of blood volume (4, 5). Based on its physiological role in the circulation, blood volume is subdivided into unstressed and stressed volumes (Vu and Vs, respectively), which represent 70% and 30% of total blood volume, respectively (4, 5). The Vu is the blood volume that maintains the vessels minimally opened and does not influence transmural pressure (i.e., the difference between intravascular pressure and the pressure exerted on the outside of the vessel wall). The Vs is the blood volume that stretches the vessel walls, effectively increasing transmural pressure (4, 5). The Vs represents the blood that moves fast within the circulatory system and is the major determinant of venous return (VR) (4, 5). During steady state conditions, VR from the systemic and pulmonary circulations equals CO. Venous return is the product of the gradient between mean circulatory filling pressure (MCFP) and right atrial pressure (RAP), divided by the resistance to blood flow in the venous circulation (Figure 1A). The effects of a fluid challenge on VR, SV, and CO may be the result of an unpredictable distribution of the infused fluid between the Vs and Vu, which may explain why ~50% of critically ill human patients do not respond to a fluid challenge with significant increases in SV and/or CO (6). If intravenous fluids are distributed mainly to Vu there will be no increase in VR, SV, and CO (6). Otherwise, a fluid challenge will result in the desired increase in VR, SV, and CO if it augments MCFP via an effective increase Vs (Figure 1B). Another frequently unappreciated physiological concept is the fact that fluids do not hold the monopoly of improving VR, SV, and CO. Venoconstriction may also shift blood from Vu to Vs, thereby, increasing VR due to an increase in MCFP (Figure 1C). Lower doses of vasopressor agents (e.g., norepinephrine and phenylephrine) may increase VR, SV, and CO through a venoconstrictive effect (6, 7). Otherwise, higher doses of vasopressors, in spite of increasing VR and SV through venoconstriction, will also induce significant arterial vasoconstriction, which could eventually lead to a decrease in CO (6). The negative impact of vasopressors on blood flow/CO can be suspected if a substantial increase in arterial pressure induced by these drugs is paralleled by clinically relevant bradycardia, which is probably caused by activation of the baroreceptor reflex.
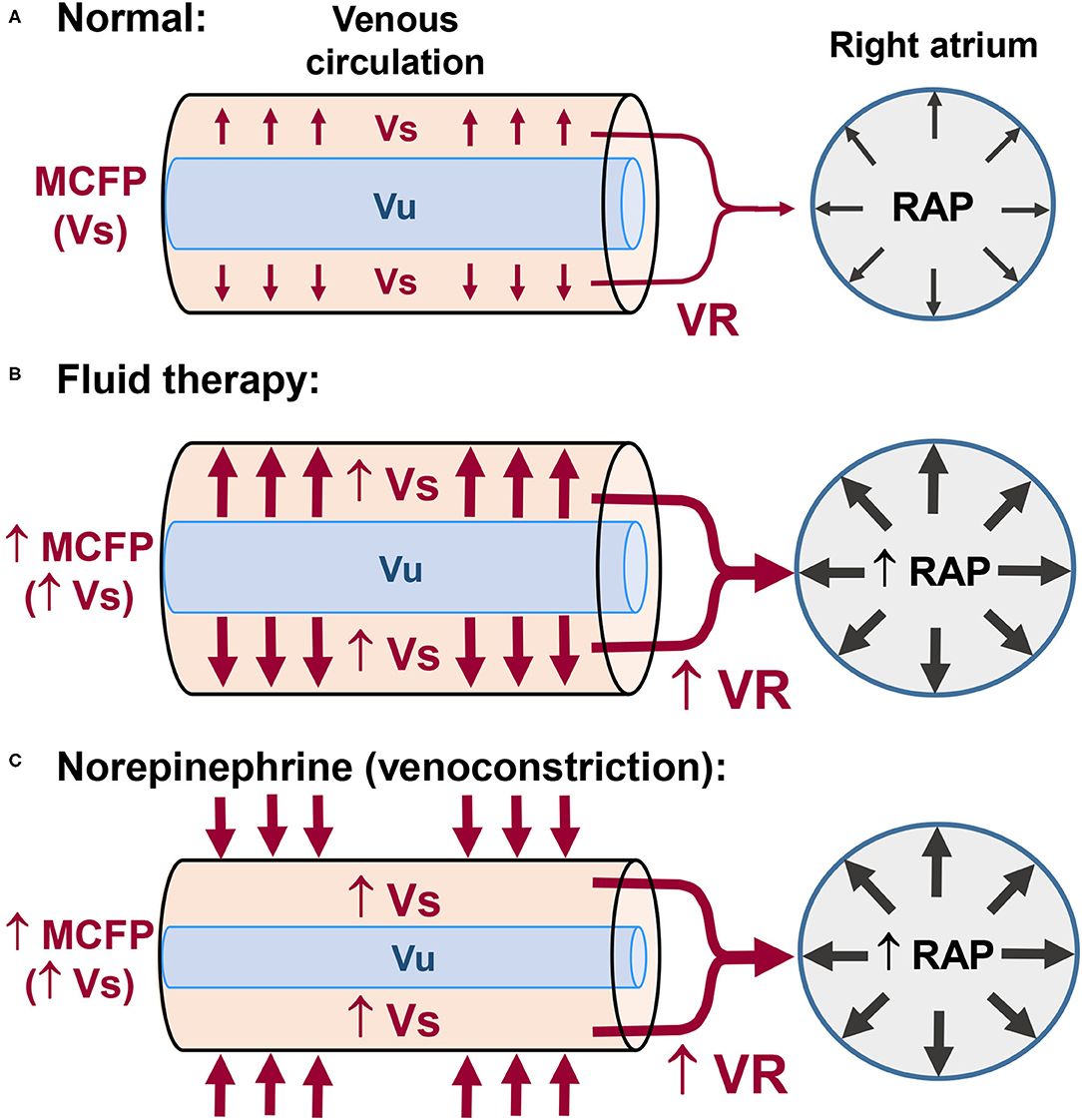
Figure 1. Control of venous return (VR) in normal subjects and the effects of fluid therapy and of vasopressors on VR. (A) The unstressed blood volume (Vu) maintains the vessels minimally opened. The stressed blood volume (Vs) exerts pressure on the vessel walls and is responsible for the mean circulatory filling pressure (MCFP). The pressure gradient between the upstream veins (MCFP) and right atrial pressure (RAP) is the major driving force responsible for VR of blood from the systemic circulation to the right side of the heart. (B) Intravenous fluid administration may increase VR and RAP (preload) via increases in Vs and MCFP. (C) Venoconstriction induced by low doses of vasopressors (e.g., norepinephrine) may also increase VR and RAP via increases in Vs and MCFP.
What is Fluid Responsiveness and How Can it Be Assessed?
In conscious patients with signs of acute circulatory failure (e.g., hypotension, increased blood lactate levels), intravascular volume expansion is beneficial only if it significantly increases SV and CO. Titration of intravenous fluids to maximize SV and CO, while avoiding hyper- or hypovolemia is an ongoing paradigm in the medical field. The Frank-Starling curve provides the physiological basis for understanding the concept of fluid responsiveness. To test the fluid responsiveness status of an individual, a change in preload must be provoked and the observed response in SV or CO recorded (8). Responders to volume expansion, whose heart is operating in the ascending limb of the Frank-Starling curve, are defined as those individuals where SV or CO increase at least 10–15% after an increase in preload induced by test dose of fluids (i.e., a fluid challenge) or by a passive leg raise test (9–11). The passive leg raise test in humans involves raising both legs to a 45° angle in relation to the trunk to temporarily increase cardiac preload by diverting part of the blood volume (~300 ml) from the legs to the trunk/central circulation. This test allows determining the fluid responsiveness status of an individual without the need to administer fluids (11). In veterinary medicine, determination of fluid responsiveness has been restricted to the evaluation of changes in SV or CO in response to a fluid challenge in anesthetized or conscious dogs (12–22).
Dynamic assessment of fluid responsiveness relies on changes in preload induced by mechanical ventilation and the resulting change in surrogates of SV (23–29). Differently from the fluid challenge approach, which relies on a test dose of fluids to determine responsiveness status, heart/lung interactions during mechanical ventilation allows identifying the relative position of an individual in the Frank-Starling curve (i.e., fluid responsiveness status) a priori to fluid administration (23–29). Because preload changes induced by volume-controlled ventilation cause larger SV variations in patients positioned in the ascending limb of the Frank-Starling curve, responders to volume expansion can be identified if respiratory induced variations in SV are increased above a certain threshold. Surrogates of SV used for dynamic assessment of fluid responsiveness include respiratory variation in peak aortic flow velocity (ΔVpeak), pulse pressure variation (PPV), systolic pressure variation (SPV). plethysmographic variability index (PVI) and stroke volume variation derived from pulse contour analysis (SVVPCA) (29).
In contrast with dynamic preload indexes, static markers of preload that reflect cardiac filling pressures (central venous pressure and pulmonary artery occlusion pressure), or that reflect end-diastolic volume, are not reliable to predict fluid responsiveness and have limited usefulness to guide fluid administration (30–32). Accurate prediction of fluid responsiveness by PPV and other dynamic preload indexes demands that patients are under volume-controlled mechanical ventilation with tidal volumes ≥8 ml/kg, without heart rhythm irregularities and without spontaneous breathing efforts (33). According to a meta-analysis, PPV was superior to predict responsiveness than other dynamic preload indexes in human patients under mechanical ventilation with tidal volumes ranging from 8 to 12 ml/kg (31). Although, PPV values >12.5% accurately discriminate responders from non-responders to a fluid challenge (31), this index has limited clinical application in the ICU setting because of inaccurate prediction of responsiveness in patients ventilated with low tidal volumes (<8 ml/kg) (34, 35). The ability of ΔVpeak, PPV, SPV, PVI, and SVVPCA to predict the response to a fluid challenge has been assessed in anesthetized mechanically ventilated dogs (12–16, 18, 21, 22). In canine studies PPV thresholds > 11–16% were able to identify responders to volume expansion (13–16, 18, 21, 22). The large variation in PPV thresholds reported by the veterinary literature can be explained in part by differences in the fluid challenge protocol, in the methods used for assessing the response to volume expansion, and in mechanical ventilation adjustments.
Although, dynamic preload indexes are effective tools to predict fluid responsiveness, the necessity for patients to be under mechanical ventilation without cardiac arrhythmias and spontaneous breathing efforts is a major limiting factor to their use in clinical practice. In critically ill spontaneously breathing human patients, the response to volume expansion can be predicted by ultrasound assessment of respiratory induced variations in inferior vena cava diameter (i.e., inferior vena cava collapsibility index) (36, 37). In a heterogeneous population of hospitalized dogs, ultrasonographic indexes showed different results, the “caudal” vena cava collapsibility index could not predict fluid responsiveness; while the “caudal” vena cava to aortic diameter ratio (CVC/Ao ratio), showed reasonable ability to discriminate responsiveness status a priori to volume expansion (19). The CVC/Ao ratio appears useful to detect early stages of volume depletion in dogs, but with variable results (38–41).
The fluid Challenge Approach and the End-Point of Fluid Ressuscitation
A fluid challenge is the rapid administration of a relatively small volume of fluid to test if an individual has a cardiac preload reserve. Inasmuch as small amounts of fluids are titrated “to effect,” based on pre-established goals, the fluid challenge also provides volume replacement with lower risk of volume overload (9, 10). Percent changes in SV or CO are the target hemodynamic variables and individuals where SV or CO increased by more than 10–15% from values recorded before the fluid challenge are characterized as responders to volume expansion (i.e., are positioned in the ascending limb of the Frank-Starling Curve). With the fluid challenge traditionally used in adult humans (500 ml of artificial colloids or crystalloids), it has been recognized that 52% of patients in the ICU and 63% of patients in the operating room show preload dependency (i.e., are responders to volume expansion) (32). More recent a meta-analysis has confirmed that only 50% of critically ill human individuals positively respond to a fluid challenge (42).
In individuals with signs of poor tissue perfusion that are responders to the first fluid challenge (SV or CO increase ≥10–15% above baseline), maximization of SV and CO can be achieved by administering additional fluid challenges (9, 10). Before a decision is made on whether fluid resuscitation should be continued, SV or CO values recorded after the previous fluid challenge is taken as the new baseline to determine percent changes in SV or CO. Stepwise fluid resuscitation can be interrupted once the individual becomes a non-responder to volume expansion (i.e., until increases in SV or CO are <10–15%). Because the only excess fluid administered corresponds to the volume of one fluid challenge, the risk of volume overload can be minimized (9, 10, 42).
One question that remains opened is the end-point of fluid resuscitation in individuals that show preload dependence. Unless there is evidence of significant hypovolemia, it has been the suggested in humans admitted for emergency care that the volume of intravenous fluids should be initially limited to 20–30 ml/kg before vasopressors are considered to treat hypotension (7). The updated guidelines of the surviving sepsis campaign recommends intravenous administration of 30 ml/kg of crystalloids during the first hour in septic human patients with hypotension and elevated plasma lactate (43, 44). Although, fluids are titrated to maximize SV and CO in GDFT (i.e., until the individual is in the transition between the ascending and the flat portion of the Frank-Starling curve), a maximum cumulative volume of fluids can be predefined in GDFT protocols to minimize the risk of fluid overload (45). In patients that are hypotensive from hypovolemia and sepsis, normalization of arterial pressure could be determinant for terminating fluid resuscitation, even if assessment of fluid responsiveness still shows a state of preload dependence (45). Otherwise, vasopressor therapy should be initiated within the first hour if initial fluid administration is not sufficient to achieve the hemodynamic resuscitation goals (43–45) (Figure 2).
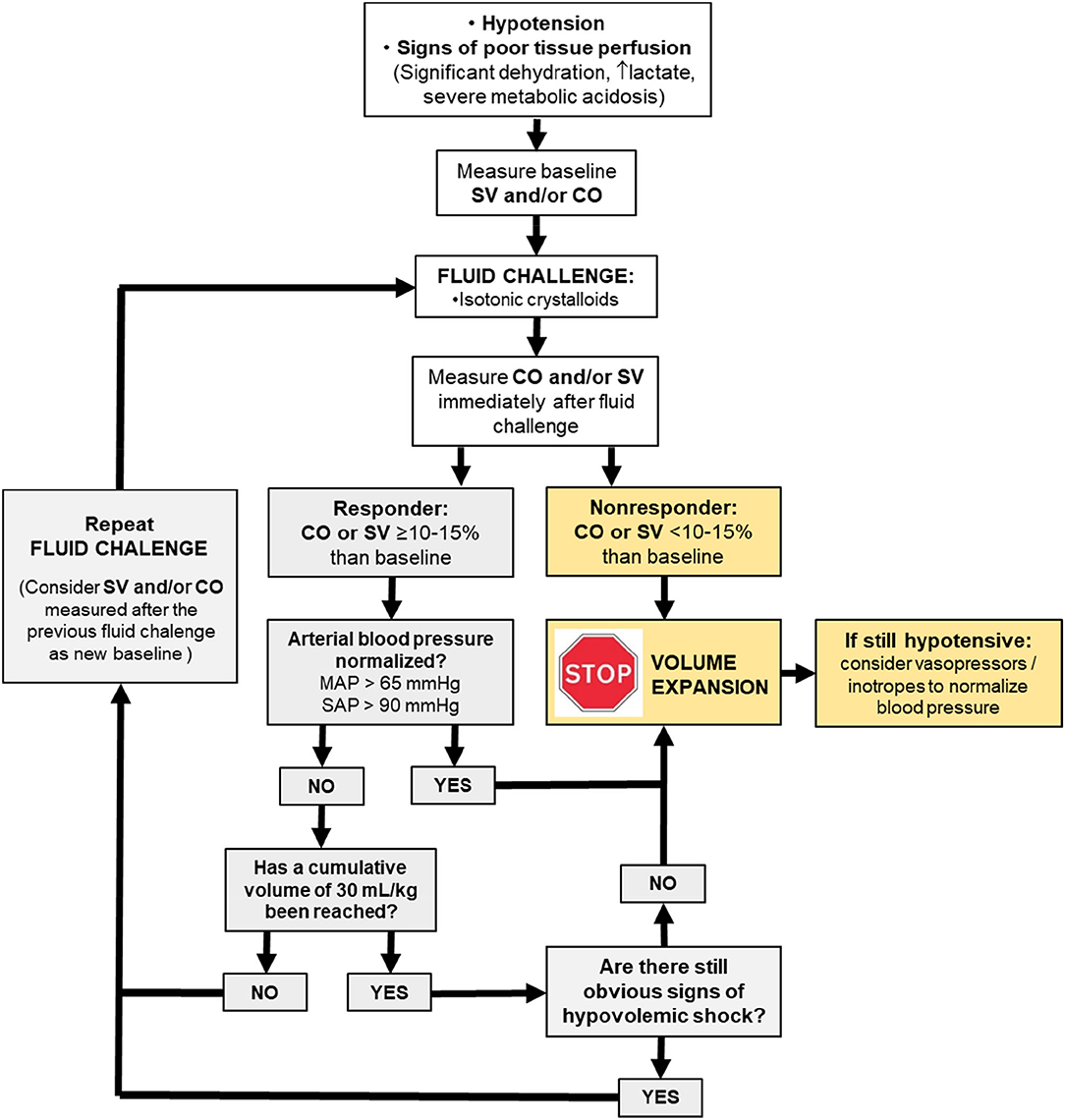
Figure 2. Physiologically driven fluid resuscitation protocol incorporating the fluid challenge approach to maximize stroke volume (SV) and cardiac output (CO). MAP, meant arterial pressure; SAP, systolic arterial pressure.
In individuals admitted for emergency care with signs of circulatory failure, hypotension is often caused by poor circulating volume that may be associated or not with sepsis-induced loss of vasomotor tone. Otherwise, hypotension during anesthesia is often caused by drug-induced decreases in systemic vascular resistance (SVR) and/or CO and does not imply that there is a circulating volume deficit (46). Although, it has been recognized that responders to volume expansion are not necessarily hypovolemic (28), hypotension-inducing hemorrhage in isoflurane anesthetized dogs can lead to a state of preload dependence, as suggested by increases in PPV and PVI from pre-hemorrhage values (47, 48). In case of intraoperative hypotension where the suspected cause is poor circulating volume, a fluid challenge approach may be attempted but cardiovascular support with sympathomimetics should not be delayed due to the dose-dependent depressant effects of inhalant anesthetics and/or neuraxial anesthesia on contractility and vasodilation (46). A drug with alpha-1 and beta-1 effects is ideal to improve both arterial blood pressure and CO, respectively (46). Constant rate infusions of vasopressor drugs, such as norepinephrine and dexmedetomidine, should be carefully used as these drugs can mask hypotension and increases in PPV and SPV caused by poor circulating volume, probably by shifting blood from the unstressed to stressed volume via a venoconstrictive action (47, 49).
According to a systematic review of literature, the fluid challenge most commonly used in adult human patients with signs of poor tissue perfusion consisted of 500 ml of crystalloids or colloids (~7 ml/kg to an adult patient weighing 70 kg), administered during 20–30 min (42). The target variable most commonly monitored after the fluid challenge in humans was an increase in CO ≥ 15% (42). Because traditionally used fluid challenges might result in some risk of volume overload, a mini-fluid challenge technique, based on the use of smaller volumes of fluids administered at faster rates has been used to predict if patients will respond to a “standard” fluid challenge (Table 1). Use of a mini-fluid challenge to test for a cardiac preload reserve can reduce the risk of fluid overload that would otherwise occur with the administration of a traditional fluid challenge. In critically ill adult humans, rapid administration of 50–150 ml of fluids during 10 s to 2 min reasonably predicts the response to larger volumes of fluids (250–500 ml) (50–55). In children undergoing elective surgery, however, a 3 ml/kg mini-fluid challenge administered during 2 min weakly predicted the effects of a 15 ml/kg volume of lactated Ringer's solution (LRS) (56). Recent meta-analysis concluded that a mini-fluid challenge reliably predicts fluid responsiveness status in the operating room and in the ICU setting (57).
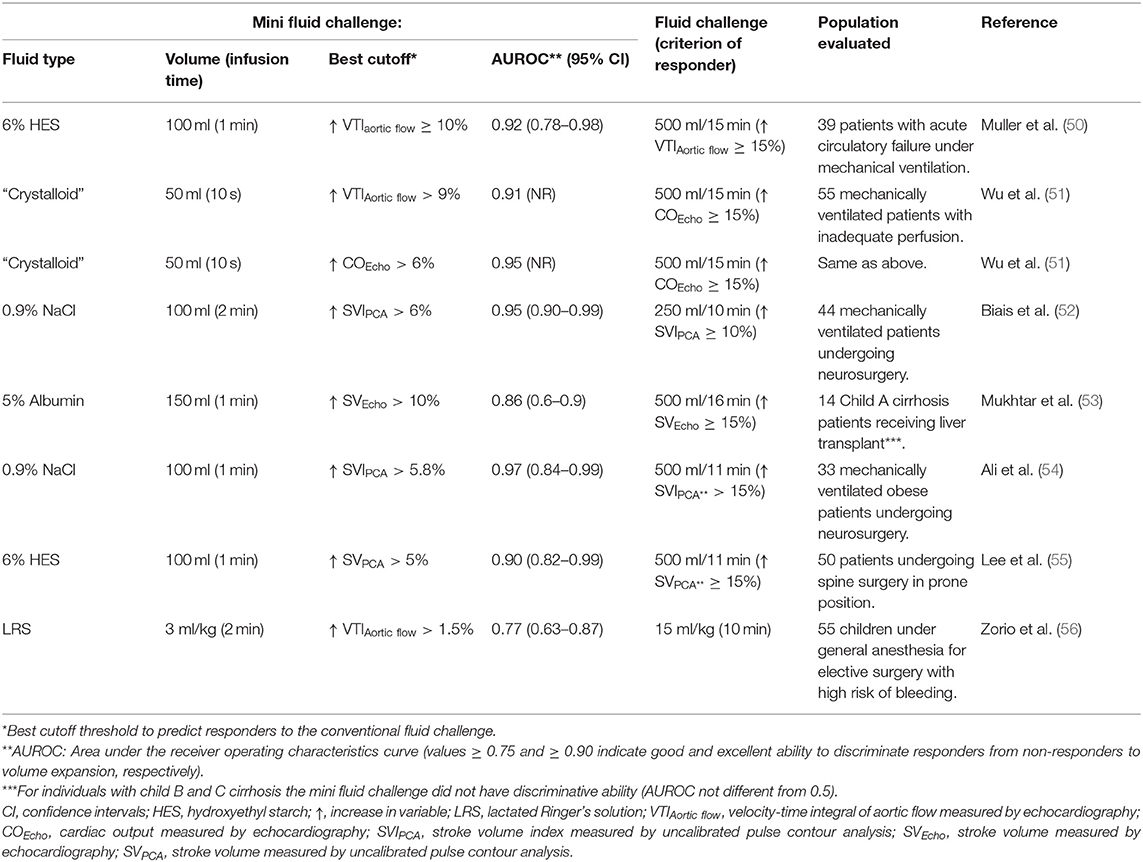
Table 1. Clinical studies using a mini-fluid challenge to predict the response to a standard fluid challenge in humans.
A summary of studies evaluating responsiveness using fluid challenges in small animals is presented in Table 2. Several reports in veterinary medicine have evaluated fluid responsiveness via a fluid challenge approach in healthy/normovolemic dogs under anesthesia (12, 15, 16, 20–22). In other studies a fluid challenge has been administered in dogs anesthetized for orthopedic/oncologic surgery that presented intraoperative hypotension (mean arterial pressure <65 mmHg) (13, 18), or in dogs anesthetized for abdominal surgeries potentially associated with hemodynamic instability that were predicted as responders to volume expansion based on PPV values >13% (14).
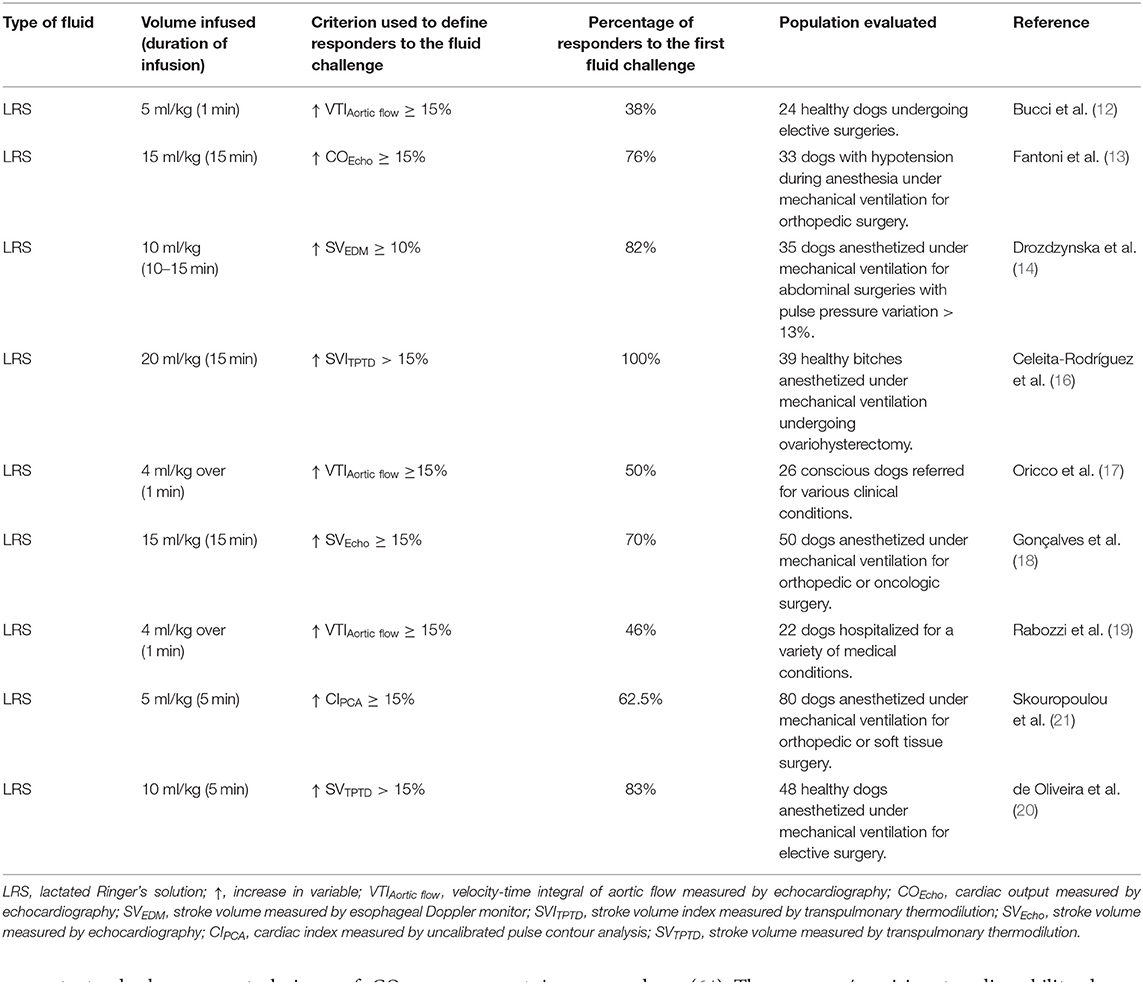
Table 2. Fluid challenge techniques with crystalloids used to evaluate fluid responsiveness in dogs.
The volume and duration of infusion of fluid challenges administered to small animal patients in the literature varies considerably. Fluid responsiveness status in dogs has been assessed via administration of LRS at doses ranging from 10 to 20 ml/kg administered over 5–15 min (13, 14, 16, 18, 20, 22). In one study, a 10 ml/kg bolus of 6% hydroxyethyl starch (HES) was administered during 13 min in anesthetized dogs (15). Smaller volumes of isotonic crystalloids (LRS), administered at faster (4–5 ml/kg during 1 min) (12, 17, 19) or slower rates (5 ml/kg during 5 min) (21) have been used to evaluate fluid responsiveness status in canine species. Although, one might define these smaller volumes of LRS as mini fluid challenges, such definition might not be appropriate because there have been no published studies evaluating the ability of these volumes to predict the response to fluid challenges traditionally used in canine species (15–20 ml/kg of LRS).
The criteria used to define responders to volume expansion in veterinary studies has been an increase in CO or SV ≥10–15% (Table 2). Transthoracic echocardiography (12, 15, 17, 19), transesophageal echocardiography (13, 18), esophageal Doppler (14), transpulmonary thermodilution (16, 20, 22), and uncalibrated pulse contour analysis (21) have been used to monitor fluid responsiveness status in anesthetized dogs. While it has been recognized that only 50% of critically ill human individuals positively respond to a fluid challenge with substantial increases in CO or SV (32, 42), the percentage of responders to a single fluid challenge with crystalloids in canine studies has been reported to range from 38 to 100% (Table 2). This large variability could be partly related to the fact that fluid responsiveness was evaluated in animals presenting with varying clinical conditions, which may lead to an altered fluid responsiveness status due to varying degrees of systemic illness. The proportion of responders/non-responders to volume expansion in these studies could also have been influenced by the use of fluid challenges administered at different volumes and rates, and to the use of monitoring devices that differ in their ability accurately to detect CO and/or SV changes induced by volume expansion (58).
Which Target Variable Should Be Used to Assess Fluid Responsiveness Status by a Fluid Challenge?
In spite of recommendations for monitoring CO or SV changes as the main response variable during GDFT, large scale clinical trials reported that arterial blood pressure was still the most commonly used target variable in humans (59). However, evaluation of changes in arterial pressure as a surrogate of CO changes could be misleading even in individuals presenting with hypotension from hypovolemia because arterial elastance/resistance can be significantly altered (20, 59, 60). Data in veterinary medicine is lacking, but it appears that arterial blood pressure is commonly used as the main target variable in dogs and cats admitted with signs of shock. Despite its limitations, arterial pressure is the preferred method to assess the response to a fluid challenge if measurement of SV and CO or its surrogates are not available. In veterinary medicine, systolic arterial pressure (SAP) measured by a Doppler ultrasound device has the advantage of allowing a subjective assessment of the quality of the peripheral perfusion. The pulsatile arterial blood flow detected by the Doppler ultrasound may be inaudible or muffled in dogs and cats with severe circulatory failure, but it often improves after a first fluid challenge, allowing measurement of SAP. In the presence of signs of shock (SAP <90 mmHg), an increase of at least 5–10 mmHg in SAP induced by a fluid challenge can be used to define a responder volume expansion. In a similar way as for SV and CO, as long as the response remains positive, fluid challenges are repeated until the target SAP is achieved. If an animal does not respond to a fluid challenge with increases in SAP, fluid resuscitation should be stopped and other alternatives to stabilize blood pressure considered. In a retrospective study of dogs admitted to a veterinary care facility with signs of circulatory failure (SAP <90 mmHg), when bolus fluid administration resulted in normalization of SAP within the first hour of fluid resuscitation (Doppler SAP ≥ 90 mmHg) there was lower probability of death compared to animals that remained hypotensive in response to rapid volume resuscitation (61).
The lack of implementation of CO or SV changes to guide fluid administration in patients with circulatory failure could be attributed to difficulties in monitoring CO and SV in a practical and reliable way. A clinically useful method of CO and SV measurement should be accurate (i.e., provide values that are close to a reference value), precise (i.e., provide values in close proximity when repeated measurements are performed during steady state conditions), and provide good ability to track changes in the target variable (62, 63). According to current standards, a new technique of CO measurement is interchangeable with a reference method if the alternative technique presents acceptable agreement and good trending ability with the reference standard (62, 63). Percentage error (PE), calculated as 2 times the standard deviation of the bias between methods divided by the average CO of the population, evaluates the precision of agreement between methods. It has been suggested that PE values <30% denote an acceptable agreement between the alternative method and the reference standard used to measure CO (62). Polar plot analysis evaluates ability of an alternative method to track changes in the reference standard based on directional changes (increase or decrease in CO) and on the magnitude of changes in CO, with mean polar angle ≤ ±5° and radial limits of agreement ≤ ±30° denoting good trending ability (63).
In addition to meeting the criteria of accuracy and precision, a device should also be operator independent and allow real time CO and SV monitoring via minimally invasive or non-invasive procedures (64). The accuracy/precision, trending ability, degree of invasiveness, operator dependence, and ability to provide real time CO and SV estimations of hemodynamic monitors currently available, with emphasis on their clinical application in fluid therapy guided by hemodynamic goals is discussed below.
Indicator Dilution Cardiac Output Techniques
Indicator dilution techniques are relatively independent of the operator skill and are considered the most accurate and precise methods of CO measurement available for clinical use (65). However, due to varying degrees of invasiveness and some other technical difficulties, indicator dilution techniques have not been routinely implemented to evaluate fluid responsiveness during fluid resuscitation of patients with signs of circulatory failure. Indicator dilution techniques provide intermittent CO and SV monitoring that need to be updated by the generation of new indicator dilution curves via injection of a thermal or a chemical indicator, which could make evaluation of fluid responsiveness less practical.
Pulmonary Artery Thermodilution
Among the indicator dilution methods available, the pulmonary artery thermodilution (PATD) technique has been the clinical gold standard method for CO monitoring since its introduction in the 1970's (65). Pulmonary artery thermodilution measures right ventricular blood flow after a known volume of thermal indicator (ice-cold or room temperature 5% dextrose) is injected into the vena cava/right atrium for recording the change in blood temperature over time (thermodilution curve) in the pulmonary artery, via a fast-response thermistor located in the tip of a pulmonary artery catheter (PAC) (65). Cardiac output measured by PATD (COPATD) was used to evaluate the response to a fluid challenge in mechanically ventilated human individuals with acute circulatory failure related to sepsis (23, 26, 30) or during anesthesia for major surgery (66). However, questionable improvement in patient outcome in face of the higher degree of invasiveness and the inherent risks involved with placement of a PAC, including intra-cardiac knotting of the catheter and pulmonary artery rupture/thrombosis, has fueled a debate on whether the PAC should still be used (67, 68). For these reasons, advanced hemodynamic monitoring by means of a PAC in human ICUs has declined in favor of other less invasive/non-invasive methods of CO monitoring (67–69). In veterinary medicine, insertion of PACs for hemodynamic monitoring has been largely restricted to experimental studies.
Transpulmonary Thermodilution
Introduced during the 1990's in the medical field, transpulmonary thermodilution (TPTD) is a less invasive indicator dilution technique than PATD because changes in blood temperature induced by the thermal indicator are measured by a thermistor tipped catheter inserted into a central artery of the systemic circulation (e.g., femoral artery) (65, 69). Experimental studies have shown that the TPTD technique shows good ability to track changes in CO. A strong correlation (correlation coefficient = 0.95) between TPTD and the gold standard reference method (perivascular flow probe around the pulmonary artery) has been reported during CO changes induced by hemorrhage and volume replacement (70).
A summary of studies in small animals evaluating the ability of CO measured by TPTD (COTPTD) to track changes in CO measured by the reference standard (COPATD) using polar plot analysis is presented in Table 3 (71–73). Based on experimental studies in dogs, where a range of CO values was obtained by increasing depth of anesthesia and inotropic (dobutamine) administration, it has been suggested that COTPTD can be used in replacement of COPATD because of an acceptable precision of agreement and good trending ability between these two techniques (73). Another report showed a marginal trending ability between COTPTD and COPATD in healthy anesthetized dogs (72). However, results were limited by the fact that the anesthetic protocol was designed for another purpose and no specific procedures were made to induce changes in CO (72). In cats, limited data from a small number of animals (n = 3) showed that COTPTD overestimated COPATD. However, there was an acceptable precision of agreement and trending ability based on polar plot analysis (71).
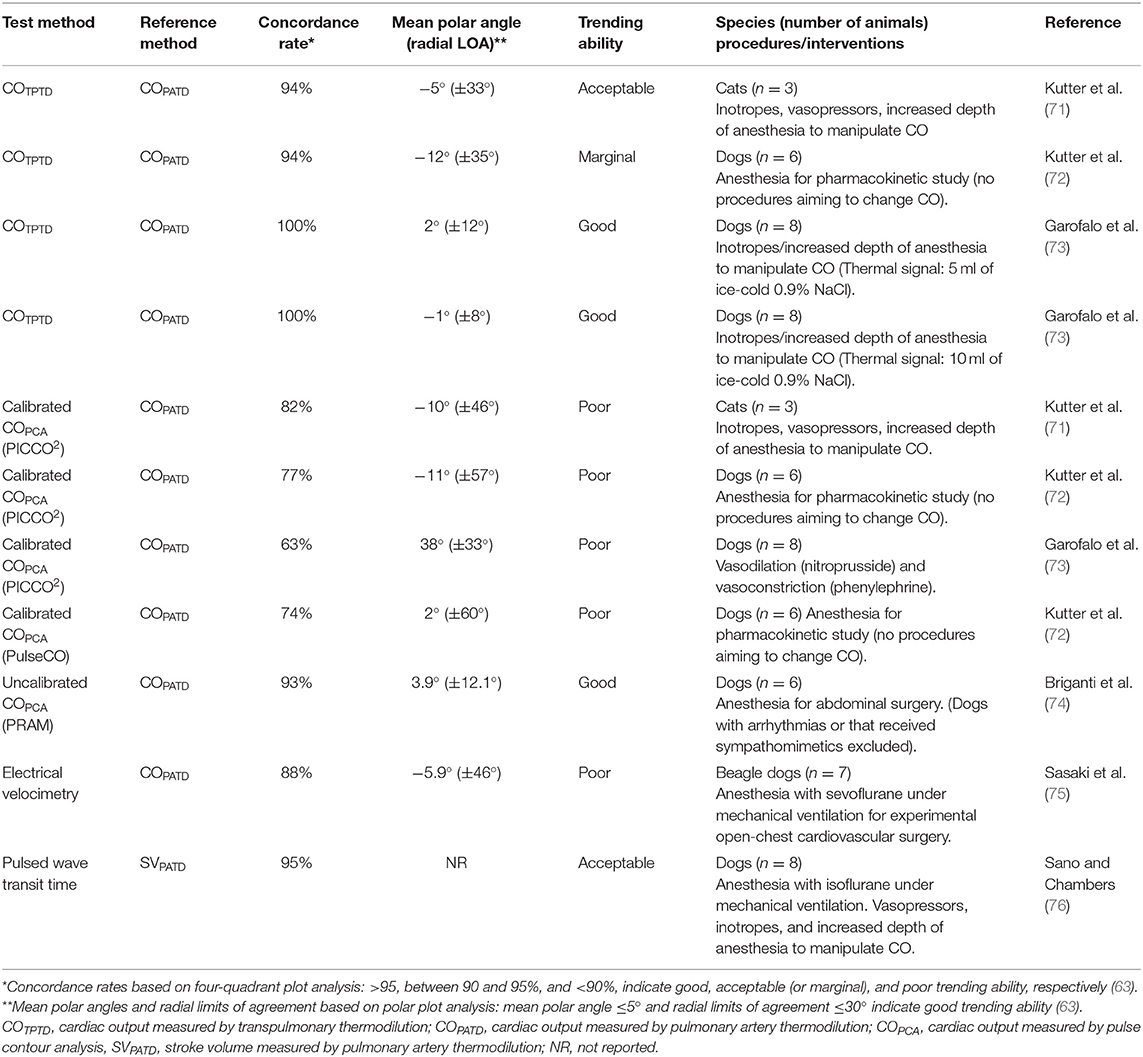
Table 3. Ability of several cardiac output monitors to track changes in cardiac output measured by a reference method induced by in dogs and cats.
Investigations have used the TPTD technique as a reference method to determine “true” fluid responsiveness status in humans (58, 77–79) and, more recently, in veterinary medicine (16, 20, 22). Averaging triplicate COTPTD measurements results in adequate precision (i.e., variability of repeated measurements <10%) to evaluate fluid responsiveness. The minimum percent change in CO that can be trusted as significant and not related to the imprecision of the method [i.e. the least significant change (LSC)] was reported decrease from 20% with single COTPTD measurements to 12% with triplicate COTPTD measurements in humans, which is adequate to detect responders to volume expansion (CO increases ≥ 15%) (80). Averaging the three closest sequential COTPTD values from a series of five measurements in anesthetized dogs, resulted in a higher level of precision (LSC = 5.1%) than previously reported in humans (LSC = 12%), which could allow reliable detection of changes in CO ≥ 10% (16).
In spite of its acceptable accuracy/precision, COTPTD monitoring during the fluid resuscitation phase is limited by the fact that it requires not only a central venous catheter for injection of the thermal indicator (ideally ice-cold physiological saline), but also a thermistor tipped catheter placed in a central artery (e.g., femoral artery) (65, 69). Although, placing the thermistor tipped catheter in a peripheral artery seems an attractive alternative, this procedure is not recommended because loss of thermal signal in the periphery will lead to errors in CO measurement (overestimation of CO due to a decrease in the area under the thermodilution curve). Placement of the thermistor tipped catheter in a peripheral (metatarsal) artery in dogs failed to generate thermodilution curves necessary to measure COTPTD (81). Another disadvantage is the fact that COTPTD monitoring does not provide real time CO assessment and the need for rapid injection of ice-cold thermal signal to generate 3 thermodilution curves can result in an additional intravascular volume load. For use in small animals, the TPTD technique could be further limited by the high cost of the thermodilution catheter, which was designed for single use. Also the risk of displacement of the catheter from its insertion site (femoral artery) and hematoma formation should be considered as potential complications (72).
Lithium Dilution
Lithium dilution is a less invasive technique compared to PATD and TPTD because it does not demand catheterization of a central vein or a central artery. Injection of the chemical indicator (lithium chloride) can be performed through a peripheral venous catheter in humans and dogs (82, 83). The lithium dilution curve (plot of the lithium concentration over time) is measured in arterial blood withdrawn from a peripheral artery catheter into a lithium sensor located outside the patient (84). Although, PE (precision of agreement) calculations have not been reported in earlier studies, subjective assessment of Bland Altman analysis results has led authors to conclude that this technique provides reasonably good agreement with COPATD in humans and animals, including dogs and cats (82–87). However, in addition to the fact that this technique demands catheterization of a peripheral artery (which may carry greater difficulty in hypovolemic/hypotensive patients), repeated lithium chloride injections for measuring CO in hemodynamically unstable patients may lead to inaccurate results due to lithium accumulation/recirculation (86). The lithium dilution technique does not seem a suitable technique for repeatedly assessing fluid responsiveness status in smaller veterinary patients, such as cats and small dogs, because the amount of arterial blood withdrawn into the lithium sensor (~4.6 ml per measurement) can become significant if several measurements are performed over time (87).
Pulse Contour Analysis Cardiac Output Techniques
While indicator dilution techniques provide intermittent CO values that need to be updated by the generation of new indicator dilution curves via injection of additional amounts of thermal (e.g., ice-cold 5% dextrose) or chemical (lithium chloride) indicators, the pulse contour analysis (PCA) method provides continuous, real time estimation of CO and SV.
Real time estimation of CO and SV could be particularly convenient to evaluate the response to a fluid challenge during volume resuscitation (88). However, PCA methods with potential application in animals demand catheterization of a central or a peripheral artery, which may carry greater difficulty in animals with signs of shock. The major limitation of PCA devices is the poor ability to track changes in CO during conditions of hemodynamic instability associated with changes in SVR in humans (89, 90) and animals (72, 73, 91). Studies evaluating the ability of calibrated and uncalibrated PCA monitors to track changes in COPATD in small animals are summarized in Table 3. Interpretation of these studies should consider if their design incorporated or not manipulations aiming to induce changes in SVR, since the performance of PCA methods can be altered by hemodynamic instability associated with vasoconstriction/vasodilation.
Calibrated Contour Analysis Methods
The same monitors that measure CO intermittently by indicator dilution techniques (TPTD and lithium dilution) also provide real time CO and SV estimations by the calibrated PCA method. This technique is based on analysis of the arterial pressure waveform to estimate real time CO and SV using proprietary algorithms, after calibration of the system with COTPTD (PiCCO2 system and VolumeView/EV1000 system) or with CO measured by lithium dilution (LIDCOplus and PulseCO systems). Studies have suggested that the calibrated PCA method could be useful to evaluate the response to a fluid challenge in humans (77, 79, 92). Cardiac output measured by a calibrated PCA system (PiCCO2) showed good correlation with changes in COPATD and COTPTD induced by volume expansion (correlation coefficient ≥ 0.70) (77, 92).
The ability of less invasive and non-invasive methods of CO monitoring to discriminate fluid responsiveness status is presented in Table 4. In septic human patients, receiver operating characteristics (ROC) curve analysis showed that continuous CO measured by calibrated PCA (PICCO2 system) could discriminate responders (COTPTD increases ≥ 15%) from non-responders (COTPTD increases <15%) to volume expansion (77, 79). However, CO and SV estimations by calibrated PCA devices may become unreliable in case of significant changes in SVR (73, 89) and the ability of calibrated PCA devices to track changes in COTPTD induced by a fluid challenge has been reported as poor (58). Although, the drift in CO estimations can be corrected by frequent recalibration of calibrated PCA systems with the indicator dilution method (94), these devices may not meet minimum criteria of accuracy, precision, and trending ability to detect changes in COTPTD induced by a fluid challenge (58).
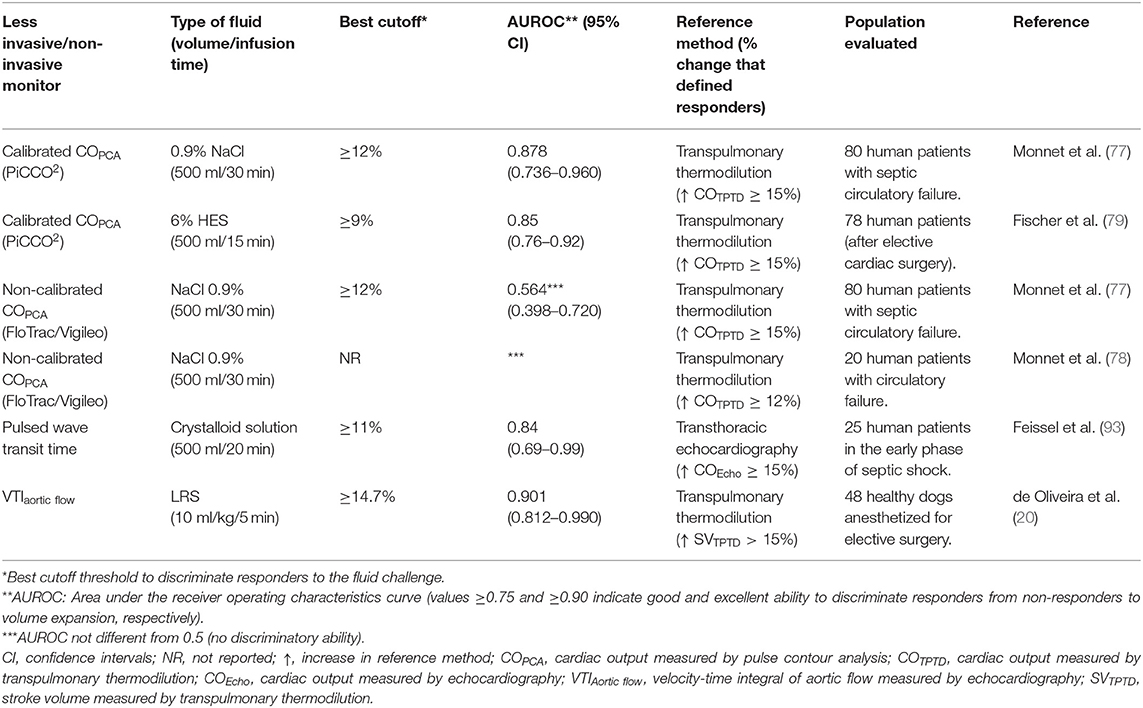
Table 4. Ability of less invasive/non-invasive methods of cardiac output and stroke volume monitoring to discriminate responders from non-responders to volume expansion induced by a fluid challenge.
Uncalibrated Pulse Contour Analysis Methods
Real time CO estimations can be provided by analysis of the arterial pressure waveform without requiring previous calibration of the system with a reference technique [FloTrac/Vigileo, LiDCOrapid, pulse recording analytical method (PRAM), ProAQT/Pulsioflex]. Real time SV monitoring with an uncalibrated PCA device (ProAQT/Pulsioflex) was used to evaluate the ability of a mini fluid challenge (50 and 100 ml of physiological saline) to predict the response to 250 ml of physiological saline in mechanically ventilated human patients undergoing neurosurgery (52). However, uncalibrated PCA devices may not discriminate fluid responsiveness status based on ROC curve analysis (77, 78) (Table 4), and have shown poor ability to track changes in CO induced by a fluid challenge (58, 78, 95, 96) (Table 5).
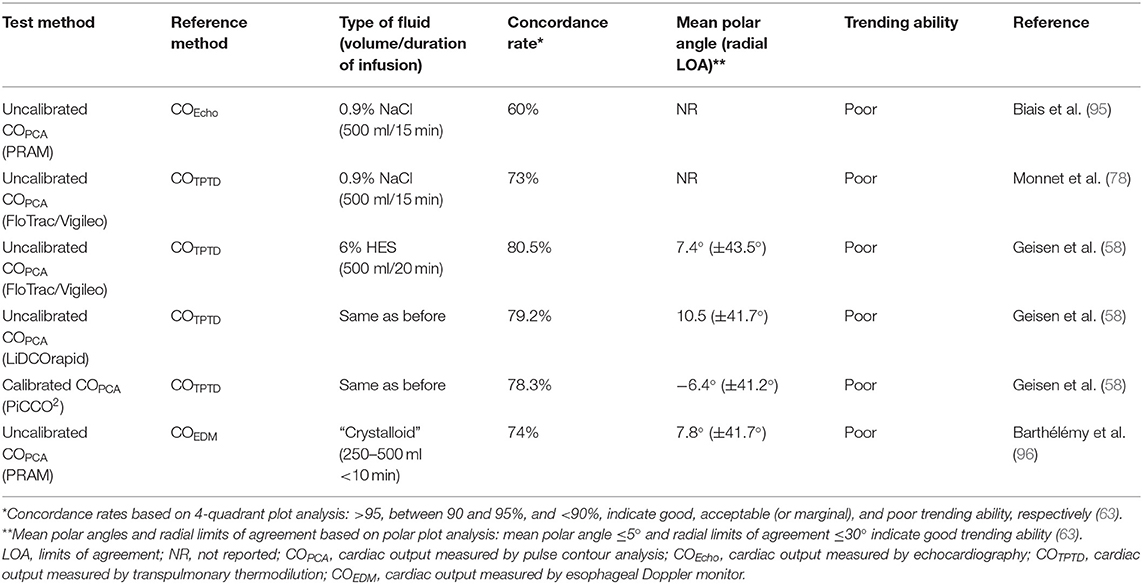
Table 5. Ability of several monitors to track changes in cardiac output induced by a fluid challenge in humans.
In spite of continuous changes in the algorithm of one uncalibrated PCA device (FloTrac/Vigileo) over the recent years, studies have shown a poor agreement and limited ability to track phenylephrine-induced changes in CO measured by echocardiography (97) and by PATD (98). Studies point out that this uncalibrated PCA device overestimates CO measured by indicator dilution methods (PATD and Lithium dilution) and is unreliable to monitor CO in dogs (99, 100).
For another uncalibrated PCA device (PRAM), there are conflicting results regarding its ability to track changes in CO measured by a reference method. Earlier studies in pigs, where a range of CO values were induced by hemorrhage and inotropic administration (dobutamine), reported an acceptable agreement (PE <30%) between PRAM and CO measured by an aortic flow probe and between PRAM and PATD (101). However, this uncalibrated PCA device has also shown an unacceptable agreement/poor ability to track changes in CO measured by thermodilution techniques in humans and in animals (90, 92). Other studies have reported that this device was unable to track changes in CO induced by volume expansion in humans (95, 96). In veterinary medicine, a preliminary study performed in anesthetized dogs reported that the PRAM method showed good precision, acceptable concordance, and good trending ability with COPATD (74). However, results were limited by the fact that its reliability was not determined during conditions of hemodynamic instability/changes in SVR.
Echocardiography
Point of care echocardiography, in addition to its role in guiding fluid challenge administration, has the unique advantage of allowing to rule out other causes of shock, such as circulatory failure caused by pericardial effusion or by decreased myocardial contractility/systolic dysfunction, where fluid resuscitation is contraindicated. Cardiac output and SV measured by echocardiography (COEcho and SVEcho, respectively) can be used to evaluate the response to a fluid challenge. While transthoracic echocardiography is useful in conscious individuals with signs of circulatory failure, transesophageal echocardiography is a practical means of monitoring the response to a fluid challenge in anesthetized patients during surgery or in unconscious/sedated individuals under mechanical ventilation. According to this technique SVEcho is firstly measured by multiplying the area of the left ventricular outflow tract (LVOT) during mid systole (the point of maximal opening of aortic valve leaflets) by the velocity time integral (VTI) of the LVOT. Because the LVOT area during mid systole does not to change over the respiratory and cardiac cycle, one single measurement of the LVOT area is usually performed for a patient as SV will vary exclusively as function of changes in aortic flow VTI (102–104). Parallel alignment between the Doppler beam and the aortic outflow, with an angle between the Doppler and LVOT as close to 0° as possible (ideally <20°) is critical for obtaining reliable aortic outflow measurements by echocardiography (105). The left parasternal five-chamber view has been used to evaluate aortic flow VTI in dogs and cats (106). However, in canine species, a subcostal (or subxiphoid) view may be preferable in point-of-care settings because it does not demand the use of a echocardiography examination table and more accurately measures peak aortic velocities, resulting in higher VTI values than the five-chamber view (106) (Figure 3).
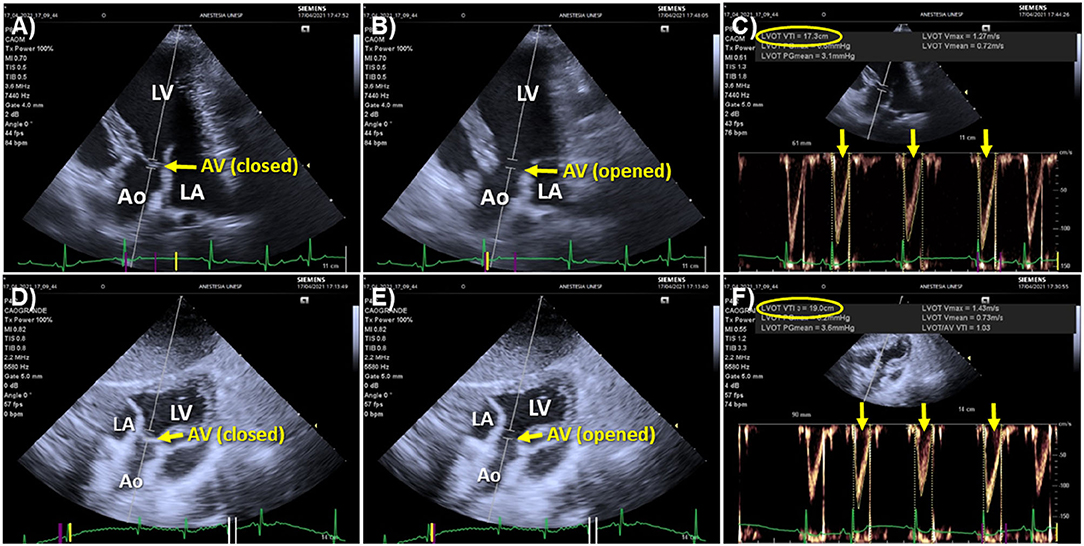
Figure 3. Echocardiographic windows used to measure aortic flow velocity-time integral (VTI) in dogs. (A) Left parasternal, apical five-chamber view optimized for visualizing the left ventricular outflow tract (LVOT) with the aortic valve closed during diastole. The Doppler beam is aligned as parallel as possible with the aortic outflow and the Doppler sampler is positioned just below the mitral annulus (point of insertion of aortic valve leaflets). (B) The same image with the aortic valve (AV) opened during systole. (C) Acceleration of aortic flow over time measured by positioning the Doppler cursor as shown in (A,B). The aortic flow VTI averaged of 3 sequential heartbeats (yellow arrows) using an automated algorithm was 17.3 cm (yellow oval). (D) Subxiphoid view of the LVOT showing alignment of the Doppler beam with the aortic outflow and the placement of the Doppler sampler with the AV closed during diastole. (E) The same image with the AV opened during systole. (F) Measurement of aortic flow VTI according to the subxiphoid view. The VTI averaged from 3 sequential heartbeats (yellow arrows) was 19.0 cm (yellow oval). Comparison between the five-chamber (A,B) and subxiphoid views (D,E) shows that the latter allowed better alignment of the Doppler beam with the aortic outflow, which contributed to a higher VTI measured from the subxiphoid view. Ao, aorta; AV, aortic valve; LV, left ventricle; LA, left atrium.
Velocity time integral measurements are sensitive to operator experience, cardiac arrhythmias and heart valve defects. Given the fact that echocardiographic measurements are highly operator dependent, a question frequently raised is the need for a certified specialist to reliably monitor COEcho. Studies have shown that COEcho measured by physicians with basic training in critical care echocardiography present good reproducibility and acceptable precision of agreement (PE = 17%) with COPATD (107). Providing that correct LVOT area and aortic flow VTI measurements are performed, transthoracic echocardiography has been considered an accurate and precise technique (PE <30%) to measure CO when compared with COPATD in critically ill humans (105). Polar plot analysis has also shown that CO measured by transthoracic echocardiography presents a good ability to track changes in COPATD (105), which is an important prerequisite for a technique used to assess the response to a fluid challenge. Beat by beat irregularities of left ventricular SV caused by arrhythmias will lead to a substantial error if COEcho and SVEcho is calculated from one single VTI measurement. Accuracy of measurements can be improved by averaging as many VTIs as possible in the presence of heart rhythm irregularities (108). Cardiac output measured by trans-esophageal echocardiography has been shown to present a good precision of agreement with COPATD (PE <30%) in dogs that were normotensive and hypotensive during anesthesia (109). The ability of transthoracic and transesophageal echocardiography to track changes in COPATD has not been reported in small animals.
Cardiac output and SV values derived from transthoracic or transesophageal echocardiography have been used to discriminate responders from non-responders to volume expansion in the human medical field (25, 51, 53, 95, 110) and in dogs (13, 18). Because SV will change only as a function of changes in aortic flow, percent changes in aortic flow VTI have been used as a surrogate of SV to evaluate fluid responsiveness status in humans (36, 50, 56, 111) and dogs (12, 17, 19). More recently, in addition to the use of aortic flow VTI to track changes in SV, use of the “minute distance,” calculated as the VTI times the heart rate, has been proposed as a surrogate of CO in point-of-care settings (104).
Although, percent changes in aortic flow VTI have been used as a surrogate of SV changes in GDFT protocols, there have been no studies evaluating the ability of VTI to track changes in SV determined by a reference method (e.g., thermodilution CO). It must be borne in mind that the reliability of VTI measurements is highly operator dependent. To account for operator related errors, studies evaluating fluid responsiveness with echocardiography should ideally report intra and inter-operator agreement (112). The reliability of repeated measures performed by one single observer (repeatability) and between observers (reproducibility) can be assessed by intraclass correlation coefficients. Values between 0.6–0.74 and 0.75–1 suggest good and excellent correlation, respectively (112).
When VTI is used to evaluate fluid responsiveness, one important question that arises is the level of precision (i.e., the proximity of repeated measurements) of aortic flow measurements obtained during stable hemodynamic conditions. If the precision of the method is poor, it may not be able to correctly identify responders to volume expansion (80). In human patients with a regular heart rhythm, averaging the VTI from 3 sequential heartbeats is recommended for achieving an adequate precision (108). In the presence of a regular heart rhythm, the minimum percent change in VTI between two successive measurements that can be trusted as significant (i.e., the least significant change) was 11%, which would be adequate to detect increases in VTI ≥ 15% induced by a fluid challenge (108). Otherwise, in individuals with an irregular heart rhythm, evaluation of fluid responsiveness by VTI carries greater difficulty because of beat-by-beat variations on SV and aortic flow. Under these circumstances, averaging VTI from 5 sequential heartbeats or more may be necessary to achieve an adequate level of precision of the method (108).
The ability of a method to detect changes in SV induced by a fluid challenge can be inadequate during conditions of hemodynamic instability/irregular heart rhythm because the method's variance is composed not only by the precision of the method but also by the method's general variability about the true values (defined as “trueness”) (113). Therefore, relatively small physiologic variations in CO and SV values can worsen the precision of the method and impair the ability of VTI to detect smaller changes in CO and SV induced by a fluid challenge (113). Fear, anxiety and discomfort caused by physical restraining, which is often necessary performing transthoracic echocardiography in conscious animals, can result in changes in sympathetic tone and interfere with the level of precision of VTI measurements. In anesthetized dogs, percent changes in aortic flow VTI induced by a fluid challenge > 14.7% showed good ability to discriminate responders to volume expansion, defined as dogs where SV measured by TPTD increased > 15% after the fluid challenge (20) (Table 4). The close proximity between the optimal cutoff threshold determined from the ROC curve (percent change in VTI > 14.7%) and the percent change in SV used to define “true” responders to volume expansion, suggest that changes in aortic flow VTI can be used as a surrogate of SV to evaluate fluid responsiveness in dogs (20). However, percent changes in VTI associated with a high probability of false negative and/or false positive results (zone of diagnostic uncertainty) was reported to range from 11.8 to 17.6%, which could be related to errors in obtaining proper alignment of the pulsatile Doppler with the aortic flow (20). In conscious dogs with a variety of clinical conditions, baseline aortic flow VTI (measurements obtained prior to a fluid challenge) ≤ 10.3 cm predicted responders to volume expansion with high sensitivity (84.9%) and specificity (100%) (17).
Measuring the VTI demands several keystrokes and could be time-consuming, which may be less practical in the emergency setting. However, with the recent development of algorithms incorporated into ultrasound machines, it has become possible to perform automated VTI measurements. Use of tools that allow automated VTI measurements has the potential for optimizing evaluation of fluid responsiveness in the emergency settings (Figure 4).
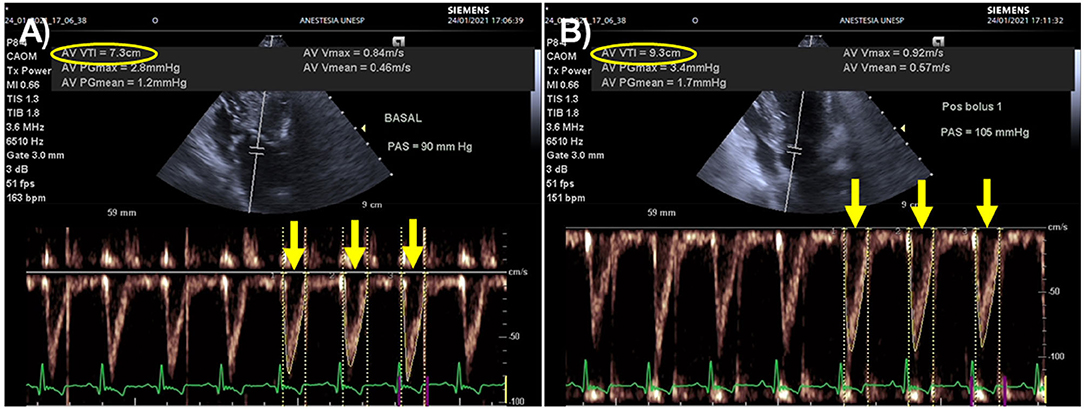
Figure 4. Effects of a fluid challenge with 10 ml/kg of lactated Ringer's solution administered over 5 min on aortic flow velocity time integral (VTI) recorded from an apical five-changer view in a dog with blunt trauma. An automated algorithm was used to measure aortic flow VTI as the average of 3 sequential heartbeats (yellow arrows). (A) Heart rate (HR), Doppler systolic arterial pressure (SAP), and aortic flow VTI before the fluid challenge were 163 beats/min, 90 mmHg and 7.3 cm (yellow oval), respectively. (B) After the fluid challenge, HR, Doppler SAP and aortic flow VTI were 151 beats/min, 105 mmHg and 9.3 cm (yellow oval). This animal was a responder to volume expansion because aortic flow VTI increased 27% (from 7.3 to 9.3 cm). The minute distance (HR times VTI), a surrogate index used to track changes in cardiac output, increased 17% after the fluid challenge (from 1197 to 1404 cm).
Velocity time integral measurements are overestimated in the presence of moderate-to-severe aortic regurgitation and sub-aortic obstruction (aortic stenosis) (104). Dynamic LVOT obstruction may be observed during severe hypovolemia/inotropic stimulation, in dogs with sub-aortic stenosis, and cats with hypertrophic cardiomyopathy. In the presence of these conditions, it is not possible to determine if an increase in aortic flow VTI results from increased SV or from increased regurgitant volume (aortic regurgitation) or from subaortic/LVOT stenosis (104) (Figure 5).
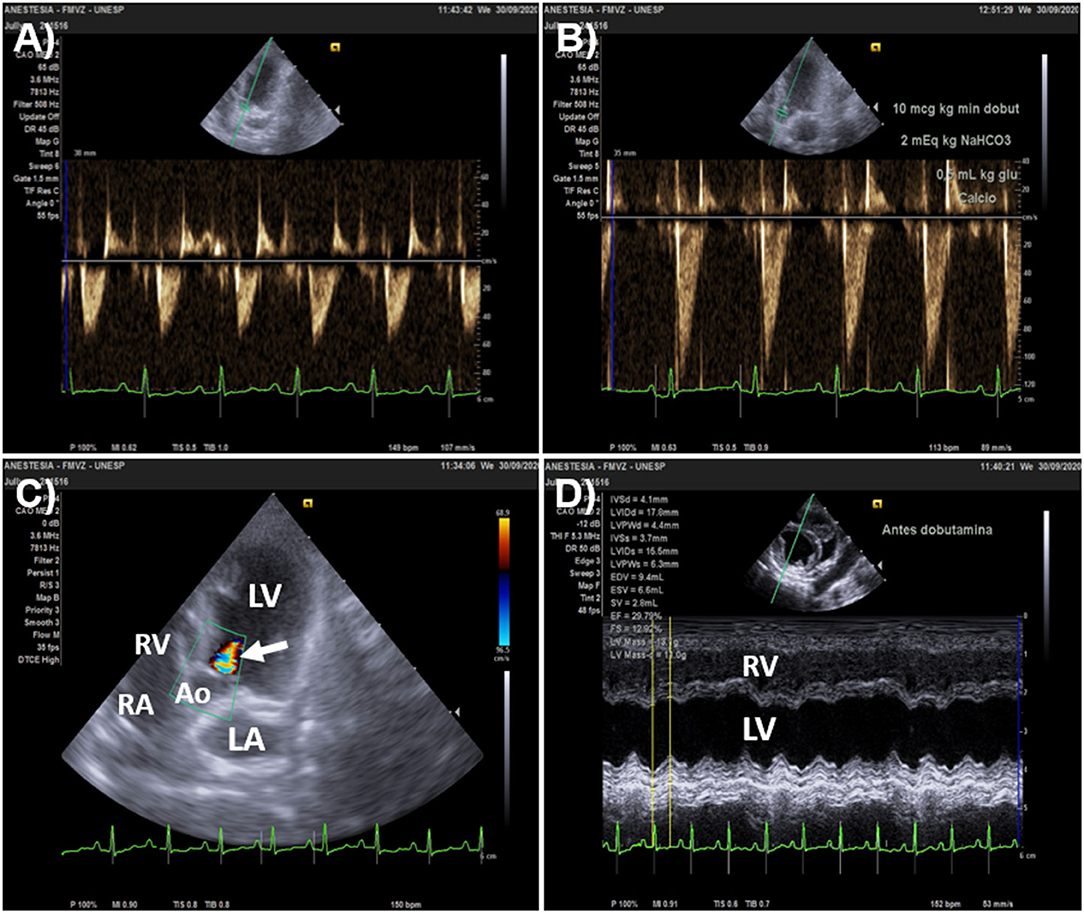
Figure 5. Overestimation of stroke volume (SV) changes by aortic flow velocity-time integral (VTI) in a 3.0 kg Pinscher dog that presented aortic regurgitation. The animal was admitted with signs of acute circulatory failure (unconsciousness of sudden onset, absent peripheral pulses and muffled heart sounds). (A) Baseline heart rate (HR) and aortic flow VTI (left parasternal, five-chamber apical view) was 149 beats/min and 5.1 cm, respectively. Doppler systolic arterial pressure (SAP) was undetectable. The minute distance (HR times VTI) was 760 cm. (B) After dobutamine was administered by constant rate infusion (10 μg/kg/min), HR was 113 beats/min and aortic flow VTI increased by 100% (from 5.1 to 10 cm); while minute distance and SAP were 1113 cm 170 mmHg, respectively. (C) The 100% increase in VTI detected after dobutamine overestimated percent increases in SV because of a significant aortic regurgitation shown by color Doppler (arrow). (D) M-mode echocardiography of the short axis of the heart (right parasternal view) upon admission showed that ejection fraction (EF) and fractional shortening (FS) were 29.8 and 12.9%, respectively, denoting severe systolic dysfunction (defined as EF < 40% and FS < 20%). Inotropic support was initially chosen to increase SV and cardiac output instead of fluid challenge administration in this animal because of severe left ventricular systolic dysfunction. Ao, aorta; RV, right ventricle; RA, right atrium; LA, left atrium; LV, left ventricle.
Esophageal Doppler
The esophageal Doppler technique measures blood flow velocity in the descending aorta (or caudal aorta in quadrupeds) via a Doppler transducer inserted into the thoracic esophagus. The velocity of blood flow in the descending aorta plotted over time is used to determine the stroke distance (distance of the envelope that contains blood flow velocity vs. time curve measured in centimeters) (114–116). The SV measured by the esophageal Doppler method (SVEDM) is calculated by stroke distance times the cross sectional area of the aorta. A nomogram developed for human patients (based on body weight, height and body surface area) estimates the cross sectional area of the aorta, assuming a 45° angle between the aorta and the esophageal probe (114–116). The algorithm for SV determination also assumes that the proportion of the blood flow/SV generated by the left ventricle at each heartbeat to the descending aorta remains fixed at 70% and CO measured by the esophageal Doppler method (COEDM) is calculated by the multiplying SV/stroke distance by the heart rate (114–116).
The esophageal Doppler monitor showed poor precision of agreement with the reference standard (PATD) in pediatric patients with congenital heart defects and in patients undergoing coronary artery bypass surgery (PE = 54 and 70%, respectively) (114, 115). Although, COEDM cannot be considered interchangeable with COPATD, this device can show an acceptable/marginal trending ability with COPATD, suggesting that this method could be used to guide volume expansion in goal directed fluid therapy (115). The use of esophageal Doppler monitoring to guide fluid administration reduced the rate of postoperative complications in patients undergoing major abdominal surgery compared to fluid therapy guided by conventional parameters (e.g., central venous pressure and arterial pressure) (116).
Similarly to human studies, it has been reported that COEDM does not present an acceptable agreement with COPATD in dogs (PE = 39%) (117). Otherwise, an abstract reported a close relationship between stroke distance measured by the esophageal Doppler and SV measured by thermodilution in dogs undergoing hemorrhage and re-transfusion of shed blood (correlation coefficient = 0.9), suggesting that this monitoring device might be a useful surrogate marker of SV changes induced by a fluid challenge (118). Additional studies are necessary to establish the clinical usefulness of the esophageal Doppler as a tool to monitor fluid responsiveness in dogs and cats.
Electrical Velocimetry
Electrical velocimetry (or transthoracic electrical bioimpedance) is a non-invasive method of continuous CO monitoring based on impedance cardiography. Measurement of left ventricular SV and CO is based on a transcutaneous electrical AC voltage applied to the chest to calculate changes in resistance during the cardiac cycle (119–125). Studies in humans have compared electrical velocimetry with PATD, TPTD, transthoracic, and transesophageal echocardiography with conflicting results (119–125). A recent meta-analysis concluded that electrical velocimetry cannot replace thermodilution or transthoracic echocardiography to measure CO because of an unacceptable precision of agreement (mean PE > 30%) with these methods (126). The clinical utility of electrical velocimetry is also limited in veterinary medicine. In sevoflurane-anesthetized dogs, an electrical velocimetry device showed a marginally acceptable agreement (PE = 30.4%) and a poor ability to track changes in COPATD (75), which could limit the usefulness of this method to evaluate the response to a fluid challenge in dogs (Table 3).
Pulse Wave Transit Time
Pulse wave transit time (PWTT) is another recently developed technique that provides continuous, non-invasive CO estimations. This method measures the time elapsed between the R wave of the electrocardiogram, which corresponds to the beginning of ventricular systole, and the appearance of the corresponding pulse wave on a pulse oximeter. Measurement of CO by PWTT is based on the principle that the transit time of pulsatile blood flow, detected by a pulse oximeter, is inversely proportional to CO (76, 93, 127, 128). However, studies have shown that PWTT has an unacceptable agreement with COPATD in dogs (PE = 61–63%) and humans (PE = 69%) (93, 127). Cardiac output measured by PWTT has also shown a poor agreement (PE = 47%) and poor trending ability (concordance rate = 74%) with COTPTD (128). In spite of its poor agreement and poor trending ability with reference methods, percent changes in CO measured by PWTT ≥ 11% showed reasonable ability to discriminate the response to a 500 ml fluid challenge (area under the ROC curve = 0.84) during the early phase septic shock humans (93). This observation, and the good ability of PWTT to detect a 15% increase in COPATD in dogs (76), raises the possibility that this monitoring tool might be useful evaluate fluid responsiveness (Tables 3, 4).
Controversies of GDFT and the Risk of Fluid Overload
The benefits of volume expansion in hypovolemic individuals cannot be achieved without risks. Although, the physiological principles of GDFT make good sense, evidence of benefit on major outcomes/mortality has been considered inconsistent in the human medical field (6). Otherwise, meta-analysis of 13 clinical trials enrolling 1642 patients, concluded that application of the concept of fluid responsiveness to patients that require acute volume resuscitation appeared to reduce mortality, duration of ICU stay, and duration of mechanical ventilation (3). However, conclusions of this study may be restricted to high-risk surgery, which comprised 12 of 13 trials analyzed (3). While it appears that GDFT in patients undergoing major surgery is beneficial, evidence of an improved outcome of GDFT in septic individuals is lacking (129). In human patients with septic shock there is an association between the volume of fluids administered during resuscitation and the degree of damage to the endothelial glycocalyx, a carbohydrate-rich layer lining vascular endothelial cells that prevents leakage of fluids from the intravascular to the extravascular compartment (130). Theoretically, administering fluids until individuals are at the flat portion of the Frank-Starling curve might further damage the endothelial glycocalyx in septic patients. More recently, dynamic assessment of fluid responsiveness to guide fluid or vasopressor administration in patients with septic shock decreased the total amount of intravenous fluids, lowered the risk of renal and respiratory failure when compared to usual care (45).
Damage to the Endothelial Glycocalyx and Lung Edema Secondary to Volume Expansion
Repeated fluid challenge administration until the heart is operating on the flat portion of the Frank-Starling curve may potentially lead to tissue/lung edema. Increases in cardiac filling pressures induced by fluid administration stimulates the release of atrial natriuretic peptide by myocardial cells (131), which is a physiological mechanism whereby the organism promotes elimination of excess fluid. However, it has been demonstrated that increased plasma atrial natriuretic peptide also promotes shedding of the endothelial glycocalyx, which may lead to tissue edema (132).
Pulmonary edema is a major complication of fluid overload that can be objectively evaluated in human critical care settings by measuring extra-vascular lung water index (EVLWI) and pulmonary vascular permeability index (PVPI) in patients monitored with TPTD technology (133, 134). In human subjects EVLWI > 10 ml/kg and PVPI <2 are suggestive of cardiogenic pulmonary edema; whereas, EVLWI > 10 ml/kg and PVPI > 3 suggests that pulmonary edema is likely caused by increased pulmonary vascular permeability, such as in acute respiratory distress syndrome (133, 134). The superimposition of the Marik-Phillips curve and the Frank-Starling curve, which depict the relationship between EVLWI and SV with preload, respectively, allows understanding why there is an increased the risk of pulmonary edema if the heart is operating near the flat portion of the Frank-Starling curve (135) (Figure 6).
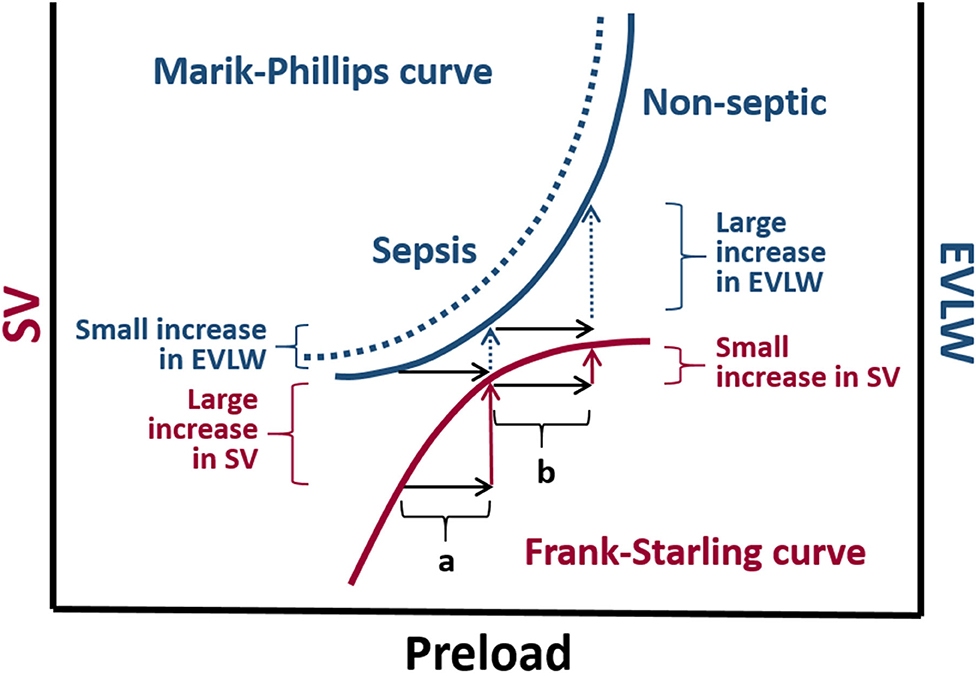
Figure 6. The Marik-Phillips curve and the Frank-Starling curve correlating changes in extra vascular lung water (EVLW) and stroke volume (SV) with preload, respectively. For individuals whose heart is operating on the ascending limb of the Frank-Starling curve, an increase in preload induced by a fluid challenge (a) does not substantially increase EVLW. If a fluid challenge is administered to individuals whose heart is operating on the flat portion of the Frank-Starling curve, the increase in preload (b) may result in a large increase in EVLW. Due to endothelial glycocalyx damage associated with sepsis, larger increases in EVLW can be expected in septic individuals (dashed curve).
Based on experimental studies healthy anesthetized dogs, it appears that reference values of EVLWI in humans cannot be extrapolated to canine species, as mean EVLWI values of 12 ml/kg have been reported in healthy anesthetized dogs receiving a standard fluid rate of 2 ml/kg/hour of LRS (136). In normovolemic dogs undergoing elective surgery, mean EVLWI values were in the range of 9 to 10 ml/kg and were not significantly increased by one or two fluid challenges with 20 ml/kg of LRS administered over 15 min (16). Although, EVLWI did not increase after volume expansion, an increased incidence of peripheral edema in the form of chemosis and edema of the tongue was recorded the group of animals that were positioned at the flat portion of the Frank-Starling curve after receiving two 20 ml/kg crystalloid boluses (16).
Use of Point of Care Echocardiography to Evaluate the Risk of Pulmonary Edema Secondary to Volume Expansion
Hydrostatic pulmonary edema may occur if a fluid challenge is administered to individuals presenting a restrictive left ventricular filling pattern. Features of diastolic dysfunction leading to a restrictive filling pattern include an excessive acceleration of early transmitral flow (E wave), and a decreased longitudinal myocardial fiber lengthening at the level of the mitral annulus during early diastole (e' wave), measured by pulse wave Doppler and by tissue Doppler imaging, respectively (137). The E/e' ratio has been in humans used as an estimate of left ventricular filling pressures (LVFP), with values > 14 (e' averaged from the septal and lateral mitral annulus) identifying elevated LVFP with high specificity (low false positive rate) (137).
Myxomatous mitral valve disease and dilated cardiomyopathy are among the heart diseases that lead to elevated LVFP and increased risk of pulmonary edema from bolus fluid administration in canine species (138). In cats, hypertrophic, restrictive, and dilated cardiomyopathy may be associated with elevated LVFP and pulmonary edema (139). The E/e' ratio (e' averaged from the septal and lateral mitral annulus) >12.4 shows a reasonably good ability to discriminate dogs with congestive heart failure (area under the ROC curve = 0.79) (138). However, compared to the E/e' ratio, the ratio between the E wave and the isovolumic relaxation time (IRVT) showed higher accuracy (area under the ROC curve = 0.97) to identify congestive heart failure in canine species (138). In dogs with experimentally induced fluid overload, E/IVRT ratio values > 2.2 are able to identify left atrial pressure values > 15 mmHg with high sensitivity (90%) and specificity (100%) (140). Excessive increases in LVFP due to congestive heart failure in dogs are associated with E/IVRT ratio > values 2.5 and excessively accelerated E waves (>120 cm/s) (138, 141).
Although, data are lacking in veterinary medicine, left ventricular diastolic dysfunction has been recognized as a common feature in humans with sepsis or septic shock (142). Systolic dysfunction (defined as a decrease in left ventricular ejection fraction to <50%) may not be associated with mortality in sepsis. Otherwise, diastolic dysfunction, characterized by a decrease in longitudinal fiber lengthening during early diastole (e') and an increase in E/e' ratio, has been reported to occur more frequently than systolic dysfunction (50 vs. 30% of patients) and has been associated with higher mortality in septic human patients (142, 143). The prevalence of diastolic dysfunction dogs and cats with sepsis/septic shock deserves further investigation as it can impact the prognosis and fluid resuscitation strategies in the emergency setting.
In the presence of echocardiographic changes suggestive of elevated LVFP a restrictive fluid therapy strategy should be considered (Figure 7). Otherwise, recognition of elevated LVFP does not rule out the need for volume replacement. Under these circumstances, the clinician should consider that range of optimal left ventricular filling pressure is relatively narrow and the left ventricle can be easily overfilled (103).
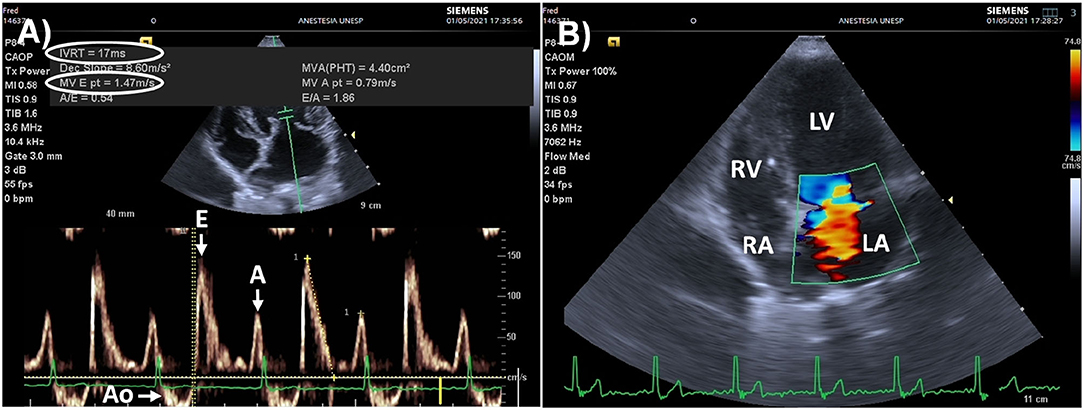
Figure 7. Point of care echocardiography in an 8 kg Schnauzer dog presented for surgery due to a bleeding mass located in the upper lip that presented elevated left ventricular filling pressures (LVFP), secondary to myxomatous mitral valve disease. (A) Pulsed wave Doppler of mitral inflow (left apical, four/five-chamber view) showing marked acceleration of the early passive diastolic filling wave (E wave = 147 cm/s, normal range: 53–108 cm/s). The isovolumic relaxation time (IVRT) was low (17 ms, normal range: 43–63 ms), and the E/IVRT ratio was markedly increased (8.64, upper reference value: 2.5), indicating high LVFP due to congestive heart failure. (B) Color Doppler (left apical, four-chamber view) showing mitral regurgitation during mid systole. This animal was anesthetized as an emergency procedure with the recommendation for a restrictive fluid therapy strategy. Inotropes/vasopressors instead of fluid boluses were recommended to manage intraoperative hypotension. The congestive heart failure was later managed by a cardiologist. Ao, aorta; E, early passive diastolic filling wave, A, late diastolic filling wave induced by atrial contraction; RV, right ventricle; RA, right atrium; LV, left ventricle; LA, left atrium.
What is the Ideal Fluid Challenge?
An ideal fluid challenge should reliably discriminate responders from non-responders to volume expansion by means of the smallest volume possible to minimize this risk of fluid overload. An excessively large volume of IV fluids used to test the preload dependency increases the risk of tissue edema. Otherwise, if the volume of fluid used as a “challenge” is excessively small, the resultant increase preload might not be enough to identify responders to volume expansion, yielding false negative results. In hemodynamically stable post-cardiac surgery human patients the administration of progressively increasing volumes of isotonic crystalloids (1 to 4 ml/kg over 5 min) increased the proportion of responders from 20% (1 ml/kg) to 65% (4 ml/kg) (144). Therefore, the ideal fluid challenge is the minimal volume of fluid that still allows identification of all “true” responders to volume expansion.
Not only the volume of fluid, but also the duration of the infusion influences the recognition of responders to volume expansion. Meta-analysis studies in humans have shown that more prolonged infusion times (>30 min) decreases the proportion of responders to volume expansion (145). A third factor that can influence the identification of responders to volume expansion is the timing of the assessment of the change in CO and/or SV. In septic humans, the number of responders to a fluid challenge recorded upon conclusion of a 500 ml crystalloid fluid challenge (individuals where aortic flow VTI increased > 15% from baseline) was decreased by 44% when fluid responsiveness was reassessed 20 min later (111). The increase in preload induced by crystalloid solutions is transient as they rapidly redistribute from the intravascular to the extravascular compartment (146). Persistence of isotonic crystalloids within the intravascular space is likely to be poorer in septic patients because of endothelial glycocalyx damage and low colloid oncotic pressure associated with hypoalbuminemia (130). Meta-analysis studies have not detected an influence of the type of fluid (colloids vs. crystalloids) on the proportion of responders to volume expansion (145). However, fluid challenges with artificial colloids have the potential to induce longer lasting increases in intravascular volume and cardiac preload than similar volumes of isotonic crystalloids.
Experimental evidence suggests that administration of 25% of the “shock dose” of crystalloids in dogs (20 ml/kg) can increase the risk of tissue edema because a larger cumulative volume of fluids may be administered until animals are recognized as non-responders to volume expansion (16). Lower volumes of crystalloids (10 ml/kg over 5 min) may be favored as standard fluid challenge because smaller cumulative volume of fluid is likely to be administered until dogs become non-responders (20). Because longer infusion times of crystalloids result in rapid redistribution of fluid from the intravascular compartment to other compartments (111, 146), infusion times should be kept as short as possible (e.g., 5 to 10 min). The standard crystalloid bolus in cats with signs of shock has traditionally been limited to 10 ml/kg over 15 min because of this species present a smaller circulating blood volume (60 ml/kg of body weight) in comparison to dogs (80 ml/kg of body weight) and because of a perceived higher risk of volume overload in this species. However, clinical data on the use fluid challenges is lacking in cats and crystalloid volumes lower than 10 ml/kg are probably adequate in this species.
Conclusions
Knowledge on the assessment of fluid responsiveness has experienced a significant development during the past two decades in the human medical field. In patients admitted with signs of circulatory failure, fluid resuscitation guided by a fluid challenge approach has the potential to improve patient outcome. Considering the principle of “less is more,” to minimize the risk of fluid overload, it has been recognized that smaller volumes of fluids, or mini-fluid challenges, may allow identification of responders to volume expansion induced by a standard fluid challenge. To reduce the risk of edema from fluid overload, recommendations for the use of 25% of the “shock dose” should be revised in favor of smaller volumes of crystalloids as standard fluid challenge (up to 10 ml/kg over 5–10 min in dogs and <10 ml/kg over 10–15 min in cats). When using the fluid challenge approach, it should be recognized that the response variable measured before and after the fluid challenge (i.e., the method of CO and SV measurement or its surrogates) will significantly impact the identification of responders to volume expansion. In veterinary medicine, goal-directed fluid therapy aiming to maximize CO and SV is in its infancy. Among the CO and SV measurement technologies that could be used to assess the response to a fluid challenge, aortic flow VTI obtained by transesophageal and transthoracic echocardiography appears to be a promising tool to evaluate fluid responsiveness in animals. Esophageal Doppler monitoring might be particularly useful to guide fluid administration in anesthetized dogs and cats or in animals under mechanical ventilation in the ICU. Future studies are needed to assess fluid responsiveness status in dogs and cats with signs of circulatory failure and the potential benefits that could be achieved by maximizing CO and SV via fluid administration in high-risk small animal patients.
Author Contributions
FT-N: manuscript conception and writing (~70% of manuscript text). AV: Manuscript preparation, writing (~30% of manuscript text), and critical review. Both authors contributed to the article and approved the submitted version.
Funding
Partial funding for this article was provided by PROAP-CAPES, Ministry of Education, Brazil (Grant number: AUXPE 1088/2020).
Conflict of Interest
The authors declare that the research was conducted in the absence of any commercial or financial relationships that could be construed as a potential conflict of interest.
Publisher's Note
All claims expressed in this article are solely those of the authors and do not necessarily represent those of their affiliated organizations, or those of the publisher, the editors and the reviewers. Any product that may be evaluated in this article, or claim that may be made by its manufacturer, is not guaranteed or endorsed by the publisher.
Acknowledgments
Images reproduced in Figure 5 were obtained in collaboration with Angelica Alfonso and Maria Lucia Gomes Lourenço from the Cardiology Section of Faculdade de Medicina Veterinária e Zootecnia–UNESP, Brazil.
References
1. Corcoran T, Rhodes JE, Clarke S, Myles PS, Ho KM. Perioperative fluid management strategies in major surgery: a stratified meta-analysis. Anesth Analg. (2012) 114:640–51. doi: 10.1213/ANE.0b013e318240d6eb
2. Davis H, Jensen T, Johnson A, Knowles P, Meyer R, Rucinsky R, et al. 2013 AAHA/AAFP fluid therapy guidelines for dogs and cats. J Am Anim Hosp Assoc. (2013) 49:149–59. doi: 10.5326/JAAHA-MS-5868
3. Bednarczyk JM, Fridfinnson JA, Kumar A, Blanchard L, Rabbani R, Bell D, et al. Incorporating dynamic assessment of fluid responsiveness into goal-directed therapy: a systematic review and meta-analysis. Crit Care Med. (2017) 45:1538–45. doi: 10.1097/CCM.0000000000002554
4. Gelman S. Venous function and central venous pressure: a physiologic story. Anesthesiology. (2008) 108:735–48. doi: 10.1097/ALN.0b013e3181672607
5. Magder S. Volume and its relationship to cardiac output and venous return. Crit Care. (2016) 20:271. doi: 10.1186/s13054-016-1438-7
6. Gelman S, Bigatello L. The physiologic basis for goal-directed hemodynamic and fluid therapy: the pivotal role of the venous circulation. Can J Anaesth. (2018) 65:294–308. doi: 10.1007/s12630-017-1045-3
7. Spiegel R. Stressed vs. unstressed volume and its relevance to critical care practitioners. Clin Exp Emerg Med. (2016) 3:52–4. doi: 10.15441/ceem.16.128
8. Cherpanath TG, Geerts BF, Lagrand WK, Schultz MJ, Groeneveld AB. Basic concepts of fluid responsiveness. Neth Heart J. (2013) 21:530–6. doi: 10.1007/s12471-013-0487-7
9. Cecconi M, Parsons AK, Rhodes A. What is a fluid challenge? Curr Opin Crit Care. (2011) 17:290–5. doi: 10.1097/MCC.0b013e32834699cd
10. Carsetti A, Cecconi M, Rhodes A. Fluid bolus therapy: monitoring and predicting fluid responsiveness. Curr Opin Crit Care. (2015) 21:388–94. doi: 10.1097/MCC.0000000000000240
11. Monnet X, Teboul JL. Passive leg raising: five rules, not a drop of fluid! Crit Care. (2015) 19:18. doi: 10.1186/s13054-014-0708-5
12. Bucci M, Rabozzi R, Guglielmini C, Franci P. Respiratory variation in aortic blood peak velocity and caudal vena cava diameter can predict fluid responsiveness in anaesthetised and mechanically ventilated dogs. Vet J. (2017) 227:30–5. doi: 10.1016/j.tvjl.2017.08.004
13. Fantoni DT, Ida KK, Gimenes AM, Mantovani MM, Castro JR, Patrício GCF, et al. Pulse pressure variation as a guide for volume expansion in dogs undergoing orthopedic surgery. Vet Anaesth Analg. (2017) 44:710–8. doi: 10.1016/j.vaa.2016.11.011
14. Drozdzynska MJ, Chang YM, Stanzani G, Pelligand L. Evaluation of the dynamic predictors of fluid responsiveness in dogs receiving goal-directed fluid therapy. Vet Anaesth Analg. (2018) 45:22–30. doi: 10.1016/j.vaa.2017.06.001
15. Sano H, Seo J, Wightman P, Cave NJ, Gieseg MA, Johnson CB, et al. Evaluation of pulse pressure variation and pleth variability index to predict fluid responsiveness in mechanically ventilated isoflurane-anesthetized dogs. J Vet Emerg Crit Care. (2018) 28:301–9. doi: 10.1111/vec.12728
16. Celeita-Rodríguez N, Teixeira-Neto FJ, Garofalo NA, Dalmagro TL, Girotto CH, Oliveira GCV, et al. Comparison of the diagnostic accuracy of dynamic and static preload indexes to predic fluid responsiveness in mechanically ventilated, isoflurane anesthetized dogs. Vet Anaesth Analg. (2019) 46:276–88. doi: 10.1016/j.vaa.2018.12.004
17. Oricco S, Rabozzi R, Meneghini C, Franci P. Usefulness of focused cardiac ultrasonography for predicting fluid responsiveness in conscious, spontaneously breathing dogs. Am J Vet Res. (2019) 80:369–77. doi: 10.2460/ajvr.80.4.369
18. Gonçalves LA, Otsuki DA, Pereira MA, Nagashima JK, Ambrosio AM, Fantoni DT. Comparison of pulse pressure variation versus echocardiography-derived stroke volume variation for prediction of fluid responsiveness in mechanically ventilated anesthetized dogs. Vet Anaesth Analg. (2020) 47:28–37. doi: 10.1016/j.vaa.2019.08.047
19. Rabozzi R, Oricco S, Meneghini C, Bucci M, Franci P. Evaluation of the caudal vena cava diameter to abdominal aortic diameter ratio and the caudal vena cava respiratory collapsibility for predicting fluid responsiveness in a heterogeneous population of hospitalized conscious dogs. J Vet Med Sci. (2020) 82:337–44. doi: 10.1292/jvms.19-0028
20. de Oliveira GCV, Teixeira-Neto FJ, Dalmagro TL, Alfonso A, Celeita-Rodríguez N, Lobo CPC, et al. Use of aortic flow indexes derived from transthoracic echocardiography to evaluate response to a fluid challenge in anesthetized dogs. Vet Anaesth Analg. (2021) 48:187–97. doi: 10.1016/j.vaa.2020.12.006
21. Skouropoulou D, Lacitignola L, Di Bella C, Stabile M, Acquafredda C, Brienza N, et al. Intraoperative assessment of fluid responsiveness in normotensive dogs under isoflurane anaesthesia. Vet Sci. (2021) 8:26. doi: 10.3390/vetsci8020026
22. Dalmagro TL, Teixeira-Neto FJ, Celeita-Rodríguez N, Garofalo NA, López-Castañeda B, Nascimento-Junior PD. Comparison between pulse pressure variation and systolic pressure variation measured from a peripheral artery for accurately predicting fluid responsiveness in mechanically ventilated dogs. Vet Anaesth Analg. (2021) 48:501–8. doi: 10.1016/j.vaa.2021.01.009
23. Michard F, Boussat S, Chemla D, Anguel N, Mercat A, Lecarpentier Y, et al. Relation between respiratory changes in arterial pulse pressure and fluid responsiveness in septic patients with acute circulatory failure. Am J Respir Crit Care Med. (2000) 162:134–8. doi: 10.1164/ajrccm.162.1.9903035
24. Michard F, Teboul JL. Using heart-lung interactions to assess fluid responsiveness during mechanical ventilation. Crit Care. (2000) 4:282–9. doi: 10.1186/cc710
25. Feissel M, Michard F, Mangin I, Ruyer O, Faller JP, Teboul JL. Respiratory changes in aortic blood velocity as an indicator of fluid responsiveness in ventilated patients with septic shock. Chest. (2001) 119:867–73. doi: 10.1378/chest.119.3.867
26. Kramer A, Zygun D, Hawes H, Easton P, Ferland A. Pulse pressure variation predicts fluid responsiveness following coronary artery bypass surgery. Chest. (2004) 126:1563–8. doi: 10.1378/chest.126.5.1563
27. Michard F. Changes in arterial pressure during mechanical ventilation. Anesthesiology. (2005) 103:419–28 doi: 10.1097/00000542-200508000-00026
28. Michard F, Lopes MR, Auler JO Jr. Pulse pressure variation: beyond the fluid management of patients with shock. Crit Care. (2007) 11:131. doi: 10.1186/cc5905
29. Monnet X, Marik PE, Teboul JL. Prediction of fluid responsiveness: an update. Ann Intensive Care. (2016) 6:111. doi: 10.1186/s13613-016-0216-7
30. Osman D, Ridel C, Ray P, Monnet X, Anguel N, Richard C, et al. Cardiac filling pressures are not appropriate to predict hemodynamic response to volume challenge. Crit Care Med. (2007) 35:64–8. doi: 10.1097/01.CCM.0000249851.94101.4F
31. Marik PE, Cavallazzi R, Vasu T, Hirani A. Dynamic changes in arterial waveform derived variables and fluid responsiveness in mechanically ventilated patients: a systematic review of the literature. Crit Care Med. (2009) 37:2642–7. doi: 10.1097/CCM.0b013e3181a590da
32. Marik PE, Cavallazzi R. Does the central venous pressure predict fluid responsiveness? An updated meta-analysis and a plea for some common sense. Crit Care Med. (2013) 41:1774–81. doi: 10.1097/CCM.0b013e31828a25fd
33. Michard F, Chemla D, Teboul JL. Applicability of pulse pressure variation: how many shades of grey? Crit Care. (2015) 19:144. doi: 10.1186/s13054-015-0869-x
34. Yang X, Du B. Does pulse pressure variation predict fluid responsiveness in critically ill patients? A systematic review and meta-analysis. Crit Care. (2014) 18:650. doi: 10.1186/s13054-014-0650-6
35. Alvarado Sánchez JI, Caicedo Ruiz JD, Diaztagle Fernández JJ, Ospina-Tascón GA, Cruz Martínez LE. Use of pulse pressure variation as predictor of fluid responsiveness in patients ventilated with low tidal volume: a systematic review and meta-analysis. Clin Med Insights Circ Respir Pulm Med. (2020) 14:1179548420901518. doi: 10.1177/1179548420901518
36. Muller L, Bobbia X, Toumi M, Louart G, Molinari N, Ragonnet B, et al. Respiratory variations of inferior vena cava diameter to predict fluid responsiveness in spontaneously breathing patients with acute circulatory failure: need for a cautious use. Crit Care. (2012) 16:R188. doi: 10.1186/cc11672
37. Corl KA, George NR, Romanoff J, Levinson AT, Chheng DB, Merchant RC, et al. Inferior vena cava collapsibility detects fluid responsiveness among spontaneously breathing critically-ill patients. J Crit Care. (2017) 41:130–7. doi: 10.1016/j.jcrc.2017.05.008
38. Cambournac M, Goy-Thollot I, Violé A, Boisvineau C, Pouzot-Nevoret C, Barthélemy A. Sonographic assessment of volaemia: development and validation of a new method in dogs. J Small Anim Pract. (2018) 59:174–82. doi: 10.1111/jsap.12759
39. Kwak J, Yoon H, Kim J, Kim M, Eom K. Ultrasonographic measurement of caudal vena cava to aorta ratios for determination of volume depletion in normal beagle dogs. Vet Radiol Ultrasound. (2018) 59:203–11. doi: 10.1111/vru.12566
40. Marshall KA, Thomovsky EJ, Brooks AC, Johnson PA, Lim CK, Heng HG. Ultrasound measurements of the caudal vena cava before and after blood donation in 9 greyhound dogs. Can Vet J. (2018) 59:973–80.
41. Herreria-Bustillo VJ, Fitzgerald E, Humm KR. Caval-aortic ratio and caudal vena cava diameter in dogs before and after blood donation. J Vet Emerg Crit Care. (2019) 29:643–6. doi: 10.1111/vec.12900
42. Messina A, Longhini F, Coppo C, Pagni A, Lungu R, Ronco C, et al. Use of the fluid challenge in critically ill adult patients: a systematic review. Anesth Analg. (2017) 125:1532–43. doi: 10.1213/ANE.0000000000002103
43. Rhodes A, Evans LE, Alhazzani W, Levy MM, Antonelli M, Ferrer R, et al. Surviving sepsis campaign: international guidelines for management of sepsis and septic shock: 2016. Intensive Care Med. (2017) 43:304–77. doi: 10.1007/s00134-017-4683-6
44. Levy MM, Evans LE, Rhodes A. The surviving sepsis campaign bundle: 2018 update. Crit Care Med. (2018) 46:997–1000. doi: 10.1097/CCM.0000000000003119
45. Douglas IS Alapat PM Corl KA Exline MC Forni LG Holder AL. Fluid response evaluation in sepsis hypotension and shock: a randomized clinical trial. Chest. (2020) 158:1431–45. doi: 10.1016/j.chest.2020.04.025
46. Valverde A. Fluid resuscitation for refractory hypotension. Front Vet Sci. (2021) 8:621696. doi: 10.3389/fvets.2021.621696
47. Diniz MS, Teixeira-Neto FJ, Cândido TD, Zanuzzo FS, Teixeira LR, Klein AV, et al. Effects of dexmedetomidine on pulse pressure variation changes induced by hemorrhage followed by volume replacement in isoflurane-anesthetized dogs. J Vet Emerg Crit Care. (2014) 24:681–92. doi: 10.1111/vec.12246
48. Klein AV, Teixeira-Neto FJ, Garofalo NA, Lagos-Carvajal AP, Diniz MS, Becerra-Velásquez DR. Changes in pulse pressure variation and plethysmographic variability index caused by hypotension-inducing hemorrhage followed by volume replacement in isoflurane-anesthetized dogs. Am J Vet Res. (2016) 77:280–7. doi: 10.2460/ajvr.77.3.280
49. Nouira S, Elatrous S, Dimassi S, Besbes L, Boukef R, Mohamed B, et al. Effects of norepinephrine on static and dynamic preload indicators in experimental hemorrhagic shock. Crit Care Med. (2005) 33:2339–43. doi: 10.1097/01.CCM.0000182801.48137.13
50. Muller L, Toumi M, Bousquet PJ, Riu-Poulenc B, Louart G, Candela D, et al. An increase in aortic blood flow after an infusion of 100 ml colloid over 1 minute can predict fluid responsiveness: the mini-fluid challenge study. Anesthesiology. (2011) 115:541–7. doi: 10.1097/ALN.0b013e318229a500
51. Wu Y, Zhou S, Zhou Z, Liu B. A 10-second fluid challenge guided by transthoracic echocardiography can predict fluid responsiveness. Crit Care. (2014) 18:R108. doi: 10.1186/cc13891
52. Biais M, de Courson H, Lanchon R, Pereira B, Bardonneau G, Griton M, et al. Mini-fluid challenge of 100 ml of crystalloid predicts fluid responsiveness in the operating room. Anesthesiology. (2017) 127:450–6. doi: 10.1097/ALN.0000000000001753
53. Mukhtar A, Awad M, Elayashy M, Hussein A, Obayah G, El Adawy A, et al. Validity of mini-fluid challenge for predicting fluid responsiveness following liver transplantation. BMC Anesthesiol. (2019) 19:56. doi: 10.1186/s12871-019-0728-4
54. Ali A, Dorman Y, Abdullah T, Yasa C, Orhan-Sungur M, Akinci IO, et al. Ability of mini-fluid challenge to predict fluid responsiveness in obese patients undergoing surgery in the prone position. Minerva Anestesiol. (2019) 85:981–8. doi: 10.23736/S0375-9393.19.13276-2
55. Lee CT, Lee TS, Chiu CT, Teng HC, Cheng HL, Wu CY. Mini-fluid challenge test predicts stroke volume and arterial pressure fluid responsiveness during spine surgery in prone position: a STARD-compliant diagnostic accuracy study. Medicine. (2020) 99:e19031. doi: 10.1097/MD.0000000000019031
56. Zorio V, Lebreton T, Desgranges FP, Bochaton T, Desebbe O, Chassard D, et al. Does a two-minute mini-fluid challenge predict fluid responsiveness in pediatric patients under general anesthesia? Paediatr Anaesth. (2020) 30:161–7. doi: 10.1111/pan.13793
57. Messina A, Dell'Anna A, Baggiani M, Torrini F, Maresca GM, Bennett V, et al. Functional hemodynamic tests: a systematic review and a metanalysis on the reliability of the end-expiratory occlusion test and of the mini-fluid challenge in predicting fluid responsiveness. Crit Care. (2019) 23:264. doi: 10.1186/s13054-019-2545-z
58. Geisen M, Ganter MT, Hartnack S, Dzemali O, Hofer CK, Zollinger A. Accuracy, precision, and trending of 4 pulse wave analysis techniques in the postoperative period. J Cardiothorac Vasc Anesth. (2018) 32:715–22. doi: 10.1053/j.jvca.2017.09.006
59. Cecconi M, Hofer C, Teboul JL, Pettila V, Wilkman E, Molnar Z, et al. Fluid challenges in intensive care: the FENICE study: a global inception cohort study. Intensive Care Med. (2015) 41:1529–37. doi: 10.1007/s00134-015-3850-x
60. Pierrakos C, Velissaris D, Scolletta S, Heenen S, De Backer D, Vincent JL. Can changes in arterial pressure be used to detect changes in cardiac index during fluid challenge in patients with septic shock? Intensive Care Med. (2012) 38:422–8. doi: 10.1007/s00134-011-2457-0
61. Silverstein DC, Kleiner J, Drobatz KJ. Effectiveness of intravenous fluid resuscitation in the emergency room for treatment of hypotension in dogs: 35 cases (2000-2010). J Vet Emerg Crit Care. (2012) 22:666–73. doi: 10.1111/j.1476-4431.2012.00822.x
62. Critchley LA, Critchley JA. A meta-analysis of studies using bias and precision statistics to compare cardiac output measurement techniques. J Clin Monit Comput. (1999) 15:85–91.
63. Critchley LA, Critchley LA, Yang XX, Lee A. Assessment of trending ability of cardiac output monitors by polar plot methodology. J Cardiothorac Vasc Anesth. (2011) 25:536–46. doi: 10.1053/j.jvca.2011.01.003
64. Geerts BF, Aarts LP, Jansen JR. Methods in pharmacology: measurement of cardiac output. Br J Clin Pharmacol. (2011) 71:316–30. doi: 10.1111/j.1365-2125.2010.03798.x
65. Reuter DA, Huang C, Edrich T, Shernan SK, Eltzschig HK. Cardiac output monitoring using indicator-dilution techniques: basics, limits, and perspectives. Anesth Analg. (2010) 110:799–811. doi: 10.1213/ANE.0b013e3181cc885a
66. Cannesson M, Le Manach Y, Hofer CK, Goarin JP, Lehot JJ, Vallet B, et al. Assessing the diagnostic accuracy of pulse pressure variations for the prediction of fluid responsiveness: a “gray zone” approach. Anesthesiology. (2011) 115:231–41. doi: 10.1097/ALN.0b013e318225b80a
67. Vincent JL. The pulmonary artery catheter. J Clin Monit Comput. (2012) 26:341–5. doi: 10.1007/s10877-012-9389-2
68. Marik PE. Obituary: pulmonary artery catheter 1970 to 2013. Ann Intensive Care. (2013) 28:38. doi: 10.1186/2110-5820-3-38
69. Sakka SG, Reuter DA, Perel A. The transpulmonary thermodilution technique. J Clin Monit Comput. (2012) 26:347–53. doi: 10.1007/s10877-012-9378-5
70. Lemson J, de Boode WP, Hopman JC, Singh SK, van der Hoeven JG. Validation of transpulmonary thermodilution cardiac output measurement in a pediatric animal model. Pediatr Crit Care Med. (2008) 9:313–9. doi: 10.1097/PCC.0b013e31816c6fa1
71. Kutter AP, Bektas RN, Hofer CK, Larenza Menzies MP, Bettschart-Wolfensberger R. Trending ability and limitations of transpulmonary thermodilution and pulse contour cardiac output measurement in cats as a model for pediatric patients. J Clin Monit Comput. (2015) 29:377–83. doi: 10.1007/s10877-014-9615-1
72. Kutter AP, Bettschart-Wolfensberger R, Romagnoli N, Bektas RN. Evaluation of agreement and trending ability between transpulmonary thermodilution and calibrated pulse contour and pulse power cardiac output monitoring methods against pulmonary artery thermodilution in anesthetized dogs. J Vet Emerg Crit Care. (2016) 26:531–40. doi: 10.1111/vec.12439
73. Garofalo NA, Teixeira-Neto FJ, Rodrigues JC, Cerejo SA, Aguiar AJ, Becerra-Velásquez DR. Comparison of transpulmonary thermodilution and calibrated pulse contour analysis with pulmonary artery thermodilution cardiac output measurements in anesthetized dogs. J Vet Intern Med. (2016) 30:941–50. doi: 10.1111/jvim.13984
74. Briganti A, Evangelista F, Centonze P, Rizzo A, Bentivegna F, Crovace A, et al. A preliminary study evaluating cardiac output measurement using Pressure Recording Analytical Method (PRAM) in anaesthetized dogs. BMC Vet Res. (2018) 14:72. doi: 10.1186/s12917-018-1392-5
75. Sasaki K, Mutoh T, Mutoh T, Kawashima R, Tsubone H. Electrical velocimetry for non-invasive cardiac output and stroke volume variation measurements in dogs undergoing cardiovascular surgery. Vet Anaesth Analg. (2017) 44:7–16. doi: 10.1111/vaa.12380
76. Sano H, Chambers JP. Ability of pulse wave transit time to detect changes in stroke volume and to estimate cardiac output compared to thermodilution technique in isoflurane-anaesthetised dogs. Vet Anaesth Analg. (2017) 44:1057–67. doi: 10.1016/j.vaa.2016.11.014
77. Monnet X, Anguel N, Naudin B, Jabot J, Richard C, Teboul JL. Arterial pressure-based cardiac output in septic patients: different accuracy of pulse contour and uncalibrated pressure waveform devices. Crit Care. (2010) 14:R109. doi: 10.1186/cc9058
78. Monnet X, Anguel N, Jozwiak M, Richard C, Teboul JL. Third-generation FloTrac/Vigileo does not reliably track changes in cardiac output induced by norepinephrine in critically ill patients. Br J Anaesth. (2012) 108:615–22. doi: 10.1093/bja/aer491
79. Fischer MO, Rebet O, Guinot PG, Lemétayer C, Saplacan V, Gérard JL, et al. Assessment of changes in cardiac index with calibrated pulse contour analysis in cardiac surgery: a prospective observational study. Anaesth Crit Care Pain Med. (2016) 35:261–7. doi: 10.1016/j.accpm.2015.12.010
80. Monnet X, Persichini R, Ktari M, Jozwiak M, Richard C, Teboul JL. Precision of the transpulmonary thermodilution measurements. Crit Care. (2011) 15:R204. doi: 10.1186/cc10421
81. Shih A, Maisenbacher HW, Bandt C, Ricco C, Bailey J, Rivera J, et al. Assessment of cardiac output measurement in dogs by transpulmonary pulse contour analysis. J Vet Emerg Crit Care. (2011) 21:321–7. doi: 10.1111/j.1476-4431.2011.00651.x
82. Kurita T, Morita K, Kato S, Kawasaki H, Kikura M, Kazama T, et al. Lithium dilution cardiac output measurements using a peripheral injection site comparison with central injection technique and thermodilution. J Clin Monit Comput. (1999) 15:279–85. doi: 10.1023/A:1009918904525
83. Mason DJ, O'Grady M, Woods JP, McDonell W. Comparison of a central and a peripheral (cephalic vein) injection site for the measurement of cardiac output using the lithium-dilution cardiac output technique in anesthetized dogs. Can J Vet Res. (2002) 66:207–10.
84. Linton R, Band D, O'Brien T, Jonas M, Leach R. Lithium dilution cardiac output measurement: a comparison with thermodilution. Crit Care Med. (1997) 25:1796–800. doi: 10.1097/00003246-199711000-00015
85. Mason DJ, O'Grady M, Woods JP, McDonell W. Assessment of lithium dilution cardiac output as a technique for measurement of cardiac output in dogs. Am J Vet Res. (2001) 62:1255–61. doi: 10.2460/ajvr.2001.62.1255
86. Mason DJ, O'Grady M, Woods JP, McDonell WN. Effect of background serum lithium concentrations on the accuracy of lithium dilution cardiac output determination in dogs. Am J Vet Res. (2002) 63:1048–52. doi: 10.2460/ajvr.2002.63.1048
87. Beaulieu KE, Kerr CL, McDonell WN. Evaluation of a lithium dilution cardiac output technique as a method for measurement of cardiac output in anesthetized cats. Am J Vet Res. (2005) 66:1639–45. doi: 10.2460/ajvr.2005.66.1639
88. Monnet X, Teboul JL. Minimally invasive monitoring. Crit Care Clin. (2015) 31:25–42. doi: 10.1016/j.ccc.2014.08.002
89. Yamashita K, Nishiyama T, Yokoyama T, Abe H, Manabe M. The effects of vasodilation on cardiac output measured by PiCCO. J Cardiothorac Vasc Anesth. (2008) 22:688–92. doi: 10.1053/j.jvca.2008.04.007
90. Paarmann H, Groesdonk HV, Sedemund-Adib B, Hanke H, Heinze H, Heringlake M, et al. Lack of agreement between pulmonary arterial thermodilution cardiac output and the pressure recording analytical method in postoperative cardiac surgery patients. Br J Anaesth. (2011) 106:475–81. doi: 10.1093/bja/aeq372
91. Urbano J, López J, González R, Fernández SN, Solana MJ, Toledo B, et al. Comparison between pressure-recording analytical method (PRAM) and femoral arterial thermodilution method (FATD) cardiac output monitoring in an infant animal model of cardiac arrest. Intensive Care Med Exp. (2016) 4:13. doi: 10.1186/s40635-016-0087-0
92. Felbinger TW, Reuter DA, Eltzschig HK, Bayerlein J, Goetz AE. Cardiac index measurements during rapid preload changes: a comparison of pulmonary artery thermodilution with arterial pulse contour analysis. J Clin Anesth. (2005) 17:241–8. doi: 10.1016/j.jclinane.2004.06.013
93. Feissel M, Aho LS, Georgiev S, Tapponnier R, Badie J, Bruyère R, et al. Pulse wave transit time measurements of cardiac output in septic shock patients: a comparison of the estimated continuous cardiac output system with transthoracic echocardiography. PloS ONE. (2015) 10:e0130489. doi: 10.1371/journal.pone.0130489
94. Hamzaoui O, Monnet X, Richard C, Osman D, Chemla D, Teboul JL. Effects of changes in vascular tone on the agreement between pulse contour and transpulmonary thermodilution cardiac output measurements within an up to 6-hour calibration-free period. Crit Care Med. (2008) 36:434–40. doi: 10.1097/01.CCM.OB013E318161FEC4
95. Biais M, Cottenceau V, Stecken L, Jean M, Ottolenghi L, Roullet S, et al. Evaluation of stroke volume variations obtained with the pressure recording analytic method. Crit Care Med. (2012) 40:1186–91. doi: 10.1097/CCM.0b013e31823bc632
96. Barthélémy R, Neuschwander A, Dramé F, Redouté M, Ditchi D, Stern J, et al. Monitoring haemodynamic response to fluid-challenge in ICU: comparison of pressure recording analytical method and oesophageal Doppler: a prospective observational study. Eur J Anaesthesiol. (2019) 36:135–43. doi: 10.1097/EJA.0000000000000924
97. Hattori K, Maeda T, Masubuchi T, Yoshikawa A, Ebuchi K, Morishima K, et al. Accuracy and trending ability of the fourth-generation FloTrac/Vigileo system in patients with low cardiac index. J Cardiothorac Vasc Anesth. (2017) 31:99–104. doi: 10.1053/j.jvca.2016.06.016
98. Maeda T, Hamaguchi E, Kubo N, Shimokawa A, Kanazawa H, Ohnishi Y. The accuracy and trending ability of cardiac index measured by the fourth-generation FloTrac/Vigileo system™ and the Fick method in cardiac surgery patients. J Clin Monit Comput. (2019) 33:767–76. doi: 10.1007/s10877-018-0217-1
99. Valverde A, Gianotti G, Rioja E, Hathway A. Comparison of cardiac output determined by arterial pulse pressure waveform analysis method (FloTrac/Vigileo) versus lithium dilution method in anesthetized dogs. J Vet Emerg Crit Care. (2011) 21:328–34. doi: 10.1111/j.1476-4431.2011.00650.x
100. Bektas RN, Kutter AP, Hartnack S, Jud RS, Schnyder M, Matos JM, et al. Evaluation of a minimally invasive non-calibrated pulse contour cardiac output monitor (FloTrac/Vigileo) in anaesthetized dogs. Vet Anaesth Analg. (2012) 39:464–71. doi: 10.1111/j.1467-2995.2012.00741.x
101. Scolletta S, Romano SM, Biagioli B, Capannini G, Giomarelli P. Pressure recording analytical method (PRAM) for measurement of cardiac output during various haemodynamic states. Br J Anaesth. (2005) 95:159–65. doi: 10.1093/bja/aei154
102. Blanco P, Aguiar FM, Blaivas M. Rapid Ultrasound in Shock (RUSH) velocity-time integral: a proposal to expand the RUSH protocol. J Ultrasound Med. (2015) 34:1691–700. doi: 10.7863/ultra.15.14.08059
103. Miller A, Mandeville J. Predicting and measuring fluid responsiveness with echocardiography. Echo Res Pract. (2016) 3:G1–12. doi: 10.1530/ERP-16-0008
104. Blanco P. Rationale for using the velocity-time integral and the minute distance for assessing the stroke volume and cardiac output in point-of-care settings. Ultrasound J. (2020) 12:21. doi: 10.1186/s13089-020-00170-x
105. Mercado P, Maizel J, Beyls C, Titeca-Beauport D, Joris M, Kontar L, et al. Transthoracic echocardiography: an accurate and precise method for estimating cardiac output in the critically ill patient. Crit Care. (2017) 21:136. doi: 10.1186/s13054-017-1737-7
106. Riesen SC, Doherr MG, Lombard CW. Comparison of Doppler-derived aortic velocities obtained from various transducer sites in healthy dogs and cats. Vet Radiol Ultrasound. (2007) 48:570–3. doi: 10.1111/j.1740-8261.2007.00299.x
107. Villavicencio C, Leache J, Marin J, Oliva I, Rodriguez A, Bodí M, et al. Basic critical care echocardiography training of intensivists allows reproducible and reliable measurements of cardiac output. Ultrasound J. (2019) 11:5. doi: 10.1186/s13089-019-0120-0
108. Jozwiak M, Mercado P, Teboul JL, Benmalek A, Gimenez J, Dépret F, et al. What is the lowest change in cardiac output that transthoracic echocardiography can detect? Crit Care. (2019) 23:116. doi: 10.1186/s13054-019-2413-x
109. Mantovani MM, Fantoni DT, Gimenes AM, de Castro JR, Flor PB, Ida KK, et al. Clinical monitoring of cardiac output assessed by transoesophageal echocardiography in anaesthetised dogs: a comparison with the thermodilution technique. BMC Vet Res. (2017) 13:325. doi: 10.1186/s12917-017-1227-9
110. Brun C, Zieleskiewicz L, Textoris J, Muller L, Bellefleur JP, Antonini F, et al. Prediction of fluid responsiveness in severe preeclamptic patients with oliguria. Intensive Care Med. (2013) 39:593–600. doi: 10.1007/s00134-012-2770-2
111. Roger C, Zieleskiewicz L, Demattei C, Lakhal K, Piton G, Louart B, et al. Time course of fluid responsiveness in sepsis: the fluid challenge revisiting (FCREV) study. Crit Care. (2019) 23:179. doi: 10.1186/s13054-019-2448-z
112. Bunting KV, Steeds RP, Slater LT, Rogers JK, Gkoutos GV, Kotecha D. A practical guide to assess the reproducibility of echocardiographic measurements. J Am Soc Echocardiogr. (2019) 32:1505–15. doi: 10.1016/j.echo.2019.08.015
113. Hapfelmeier A, Cecconi M, Saugel B. Cardiac output method comparison studies: the relation of the precision of agreement and the precision of method. J Clin Monit Comput. (2016) 30:149–55. doi: 10.1007/s10877-015-9711-x
114. Knirsch W, Kretschmar O, Tomaske M, Stutz K, Nagdyman N, Balmer C, et al. Comparison of cardiac output measurement using the CardioQP oesophageal Doppler with cardiac output measurement using thermodilution technique in children during heart catheterisation. Anaesthesia. (2008) 63:851–5. doi: 10.1111/j.1365-2044.2008.05495.x
115. Møller-Sørensen H, Cordtz J, Østergaard M, Nilsson JC, Hansen KL. Transesophageal Doppler reliably tracks changes in cardiac output in comparison with intermittent pulmonary artery thermodilution in cardiac surgery patients. J Clin Monit Comput. (2017) 31:135–42. doi: 10.1007/s10877-015-9806-4
116. Abbas SM, Hill AG. Systematic review of the literature for the use of oesophageal Doppler monitor for fluid replacement in major abdominal surgery. Anaesthesia. (2008) 63:44–51. doi: 10.1111/j.1365-2044.2007.05233.x
117. Canfrán S, Cediel R, Sández I, Caro-Vadillo A, Gómez de Segura IA. Evaluation of an oesophageal Doppler device for monitoring cardiac output in anaesthetised healthy normotensive dogs. J Small Anim Pract. (2015) 56:450–5. doi: 10.1111/jsap.12362
118. Chohan A, Pascoe P. Evaluation of transesophageal Doppler in Beagle dogs as an aid to provide goal directed fluid therapy (abstract). Vet Anaesth Analg. (2019) 46:831.e4. doi: 10.1016/j.vaa.2019.08.014
119. Schmidt C, Theilmeier G, Van Aken H, Korsmeier P, Wirtz SP, Berendes E, et al. Comparison of electrical velocimetry and transoesophageal Doppler echocardiography for measuring stroke volume and cardiac output. Br J Anaesth. (2005) 95:603–10. doi: 10.1093/bja/aei224
120. Zoremba N, Bickenbach J, Krauss B, Rossaint R, Kuhlen R, Schälte G. Comparison of electrical velocimetry and thermodilution techniques for the measurement of cardiac output. Acta Anaesthesiol Scand. (2007) 51:1314–9. doi: 10.1111/j.1399-6576.2007.01445.x
121. Gujjar AR, Muralidhar K, Banakal S, Gupta R, Sathyaprabha TN, Jairaj PS. Non-invasive cardiac output by transthoracic electrical bioimpedence in post-cardiac surgery patients: comparison with thermodilution method. J Clin Monit Comput. (2008) 22:175–80. doi: 10.1007/s10877-008-9119-y
122. Raue W, Swierzy M, Koplin G, Schwenk W. Comparison of electrical velocimetry and transthoracic thermodilution technique for cardiac output assessment in critically ill patients. Eur J Anaesthesiol. (2009) 26:1067–71. doi: 10.1097/EJA.0b013e32832bfd94
123. Blohm ME, Obrecht D, Hartwich J, Mueller GC, Kersten JF, Weil J, et al. Impedance cardiography (electrical velocimetry) and transthoracic echocardiography for non-invasive cardiac output monitoring in pediatric intensive care patients: a prospective single-center observational study. Crit Care. (2014) 18:603. doi: 10.1186/s13054-014-0603-0
124. Cox PBW, den Ouden AM, Theunissen M, Montenij LJ, Kessels AGH, Lancé MD, et al. Accuracy, precision, and trending ability of electrical cardiometry cardiac index versus continuous pulmonary artery thermodilution method: a prospective, observational study. Biomed Res Int. (2017) 2017:2635151. doi: 10.1155/2017/2635151
125. Feng SM, Liu J. Electrical velocimetry has limited accuracy and precision and moderate trending ability compared with transthoracic echocardiography for cardiac output measurement during cesarean delivery: a prospective observational study. Medicine. (2020) 99:e21914. doi: 10.1097/MD.0000000000021914
126. Sanders M, Servaas S, Slagt C. Accuracy and precision of non-invasive cardiac output monitoring by electrical cardiometry: a systematic review and meta-analysis. J Clin Monit Comput. (2020) 34:433–60. doi: 10.1007/s10877-019-00330-y
127. Magliocca A, Rezoagli E, Anderson TA, Burns SM, Ichinose F, Chitilian HV. Cardiac output measurements based on the pulse wave transit time and thoracic impedance exhibit limited agreement with thermodilution method during orthotopic liver transplantation. Anesth Analg. (2018) 126:85–92. doi: 10.1213/ANE.0000000000002171
128. Ehlers U, Erlebach R, Brandi G, Stretti F, Valek R, Klinzing S, et al. Continuous estimation of cardiac output in critical care: a noninvasive method based on pulse wave transit time compared with transpulmonary thermodilution. Crit Care Res Pract. (2020) 2020:8956372. doi: 10.1155/2020/8956372
129. Richard JC, Bayle F, Bourdin G, Leray V, Debord S, Delannoy B, et al. Preload dependence indices to titrate volume expansion during septic shock: a randomized controlled trial. Crit Care. (2015) 19:5. doi: 10.1186/s13054-014-0734-3
130. Uchimido R, Schmidt EP, Shapiro NI. The glycocalyx: a novel diagnostic and therapeutic target in sepsis. Crit Care. (2019) 23:16. doi: 10.1186/s13054-018-2292-6
131. Omar S, Ali A, Atiya Y, Mathivha RL, Dulhunty JM. Changes in B-type natriuretic peptide and related hemodynamic parameters following a fluid challenge in critically ill patients with severe sepsis or septic shock. Indian J Crit Care Med. (2017) 21:117–21. doi: 10.4103/ijccm.IJCCM_318_16
132. Chappell D, Bruegger D, Potzel J, Jacob M, Brettner F, Vogeser M, et al. Hypervolemia increases release of atrial natriuretic peptide and shedding of the endothelial glycocalyx. Crit Care. (2014) 18:538. doi: 10.1186/s13054-014-0538-5
133. Jozwiak M, Teboul JL, Monnet X. Extravascular lung water in critical care: recent advances and clinical applications. Ann Intensive Care. (2015) 5:38. doi: 10.1186/s13613-015-0081-9
134. Tagami T, Ong MEH. Extravascular lung water measurements in acute respiratory distress syndrome: why, how, and when? Curr Opin Crit Care. (2018) 24:209–15. doi: 10.1097/MCC.0000000000000503
135. Marik PE, Lemson J. Fluid responsiveness: an evolution of our understanding. Br J Anaesth. (2014) 112:617–20. doi: 10.1093/bja/aet590
136. Diniz MS, Teixeira-Neto FJ, Celeita-Rodríguez N, Girotto CH, Fonseca MW, Oliveira-Garcia AC, et al. Effects of 6% tetrastarch and lactated ringer's solution on extravascular lung water and markers of acute renal injury in hemorrhaged, isoflurane-anesthetized healthy dogs. J Vet Intern Med. (2018) 32:712–21. doi: 10.1111/jvim.14853
137. Nagueh SF, Smiseth OA, Appleton CP, Byrd BF III, Dokainish H, Edvardsen T, et al. Recommendations for the evaluation of left ventricular diastolic function by echocardiography: an update from the American Society of Echocardiography and the European Association of Cardiovascular Imaging. J Am Soc Echocardiogr. (2016) 29:277–314. doi: 10.1016/j.echo.2016.01.011
138. Schober KE, Hart TM, Stern JA, Li X, Samii VF, Zekas LJ, et al. Detection of congestive heart failure in dogs by Doppler echocardiography. J Vet Intern Med. (2010) 24:1358–68. doi: 10.1111/j.1939-1676.2010.0592.x
139. Schober KE, Chetboul V. Echocardiographic evaluation of left ventricular diastolic function in cats: hemodynamic determinants and pattern recognition. J Vet Cardiol. (2015) 17(Suppl. 1):S102–33. doi: 10.1016/j.jvc.2015.02.002
140. Schober KE, Bonagura JD, Scansen BA, Stern JA, Ponzio NM. Estimation of left ventricular filling pressure by use of Doppler echocardiography in healthy anesthetized dogs subjected to acute volume loading. Am J Vet Res. (2008) 69:1034–49. doi: 10.2460/ajvr.69.8.1034
141. de Madron E. Echocardiographic evaluation of the left ventricle filling pressure. In: de Madron E, Chetboul V, Bussadori C, editors. Clinical Echocardiographic of the Dog and Cat. 1st ed. St. Louis, MO: Elsevier Masson (2016). p. 139–50. doi: 10.1016/B978-0-323-31650-7.00009-0
142. Sanfilippo F, Corredor C, Fletcher N, Landesberg G, Benedetto U, Foex P, et al. Diastolic dysfunction and mortality in septic patients: a systematic review and meta-analysis. Intensive Care Med. (2015) 41:1004–13. doi: 10.1007/s00134-015-3748-7
143. Sanfilippo F, Corredor C, Arcadipane A, Landesberg G, Vieillard-Baron A, Cecconi M, et al. Tissue Doppler assessment of diastolic function and relationship with mortality in critically ill septic patients: a systematic review and meta-analysis. Br J Anaesth. (2017) 119:583–94. doi: 10.1093/bja/aex254
144. Aya HD, Rhodes A, Chis Ster I, Fletcher N, Grounds RM, Cecconi M. Hemodynamic effect of different doses of fluids for a fluid challenge: a quasi-randomized controlled study. Crit Care Med. (2017) 45:e161–8. doi: 10.1097/CCM.0000000000002067
145. Toscani L, Aya HD, Antonakaki D, Bastoni D, Watson X, Arulkumaran N, et al. What is the impact of the fluid challenge technique on diagnosis of fluid responsiveness? A systematic review and meta-analysis. Crit Care. (2017) 21:207. doi: 10.1186/s13054-017-1796-9
Keywords: fluid challenge, fluid responsiveness, goal-directed fluid therapy, shock, cardiac output
Citation: Teixeira-Neto FJ and Valverde A (2021) Clinical Application of the Fluid Challenge Approach in Goal-Directed Fluid Therapy: What Can We Learn From Human Studies? Front. Vet. Sci. 8:701377. doi: 10.3389/fvets.2021.701377
Received: 29 April 2021; Accepted: 06 July 2021;
Published: 03 August 2021.
Edited by:
Charlotte Sandersen, University of Liège, BelgiumReviewed by:
Francesco Staffieri, University of Bari Aldo Moro, ItalySónia Campos, University of Liège, Belgium
Copyright © 2021 Teixeira-Neto and Valverde. This is an open-access article distributed under the terms of the Creative Commons Attribution License (CC BY). The use, distribution or reproduction in other forums is permitted, provided the original author(s) and the copyright owner(s) are credited and that the original publication in this journal is cited, in accordance with accepted academic practice. No use, distribution or reproduction is permitted which does not comply with these terms.
*Correspondence: Francisco José Teixeira-Neto, ZnJhbmNpc2NvLnRlaXhlaXJhJiN4MDAwNDA7dW5lc3AuYnI=