- 1Graduate College in Animal Science, São Paulo Western University, Presidente Prudente, Brazil
- 2Laboratory of Veterinary Parasitology, Veterinary Teaching Hospital, São Paulo Western University, Presidente Prudente, Brazil
- 3Laboratory of Pisciculture, Zootechny Teaching Aquaculture, São Paulo Western University, Presidente Prudente, Brazil
- 4Graduate College of Molecular Biology, Federal University of Paraná, Curitiba, Brazil
- 5Department of Comparative Pathobiology, College of Veterinary Medicine, Purdue University, West Lafayette, IN, United States
- 6Department of Veterinary Medicine, Federal University of Paraná, Curitiba, Brazil
The present study aimed to experimentally assess Nile tilapia as potential paratenic host of Toxocara spp. A total of 15 Nile tilapia (Oreochromis niloticus) were fed with 300 embryonated Toxocara canis eggs by oral gavage, while five others of the control group received distilled water. The fish were individually analyzed at 16, 24, 48, 72, and 240 h after inoculation. Water contamination was assessed, and tissue migration by liver, gastrointestinal tract (GIT), eyes, and central nervous system. A murine model was used as the paratenic host for egg infectivity assessment. Eggs and larvae were found in plastic tank water and fish GIT, ranging from 23 to 86% per fish. Eggs and larvae were recovered from the tank water (76.3%) and fish GIT (23.7%). The counting of eggs and larvae observed was negatively correlated with number of eggs and larvae in the water tank (rho = −0.698, p = 0.003). Shedding of embryonated eggs was first detected at 16 and up to 240 h, with significant egg and larvae yield decrease on water-shedding (p = 0.001) and in the GIT (p = 0.007). Although no T. canis larva was recovered in fish tissues, egg infectivity after fish GIT transit was experimentally confirmed by mice assessment. In conclusion, despite shedding viable embryonated eggs through the gastrointestinal tract, tilapias may not play a role as a suitable paratenic hosts for Toxocara spp., posing low risk of zoonotic transmission by fish meat consumption.
Introduction
The consumption of raw or inadequately cooked fish has been increasingly popular throughout the world (1, 2). Several parasitic zoonotic agents may be related to such fish and seafood consumption, including Anisakis spp. (3, 4), Gnathostoma spp. (5), and Toxoplasma gondii (6, 7). In addition, contamination of fish-based dishes during handling may also contribute to spreading zoonotic diseases (8).
Toxocarosis has been considered one of the most prevalent parasitic zoonoses, particularly in vulnerable populations (9–11). Despite the widely used term “toxocariasis,” toxocarosis has been the standardized nomenclature of this animal parasitic disease (10, 11). Toxocarosis is among the six most important neglected parasitic infections in the USA, along with Chagas disease, cyclosporiasis, cysticercosis, toxoplasmosis, and trichomoniasis, due to its high prevalence, chronic and disabling characteristics, and a strong link with poverty (12).
Although most human infections have been asymptomatic (13), systemic larval migration through organs may cause liver damage (14), respiratory symptoms and other disorders such as asthma (15, 16). Ocular toxocarosis may lead to vision impairment, strabismus, leukocoria and retinal granulomatous lesion (17, 18). Toxocara larvae can cross the blood-brain barrier, invading the central nervous system (neurotoxocarosis), leading to meningitis, encephalitis, myelitis and cerebral vasculitis (19, 20).
Toxocarosis has been primarily associated with the ingestion of Toxocara spp. eggs from the soil (21). The definitive hosts of Toxocara canis and Toxocara cati, respectively, dogs and cats, play an important role in the oral–fecal transmission cycle, by excreting eggs directly into the anthropic environment, including recreational, public and urban green areas (22–24). As shown in a recent meta-analysis study, a fifth of public areas worldwide has been contaminated with Toxocara spp. eggs, indicating that soil may be a major source of toxocarosis and public health concern (25), which has been associated to presence of stray dogs with a higher number of positive fecal samples for intestinal nematode eggs (26). Prevalence of anti-T. canis antibodies has been extensively studied in dogs throughout the world, including 188/7,409 (2.54%) owned dogs of North America (27); 7/200 (3.5%) owned and sheltered dogs in Greece (28); 11/239 (4.6%) owned dogs of Belgium and Netherlands (29) and 157/296 (53.04%) dogs of Egypt (30). Meta-analysis studies have estimated a global 11.1% prevalence in dogs (31) and of 17.0% in cats (32), whereas the global human seroprevalence was estimated in 19.0% (33).
History of intaking raw meat of paratenic hosts such as sheep (34), rabbits (35), cattle (36), domestic pigs (37), chickens (38, 39), and ostriches (40), have also been considered a risk factor for toxocarosis (33, 41, 42). Humans are considered accidental hosts of Toxocara spp. and are most commonly infected by ingesting embryonated eggs from soil or larvae from paratenic host tissues (9, 21, 43). In this species, the larvae may migrate to the small intestine and other organs, but the parasite is unable to complete its cycle (37, 38, 44, 45).
The assessment of T. canis in livestock animals helps prevent disease transmission (46), however, the role of fish in the epizootiological chain of Toxocara spp. remains unclear. In addition, companion animals such as dogs and cats are often maintained close to lakes, rivers, and ponds, including fish farms, resulting in water contamination and exposure of the fish to dog and cat feces (47). Consequently, infected fish later be consumed by dogs and human beings (48).
The Nile tilapia (Oreochromis niloticus) has been considered one of the most common freshwater-bred fish species worldwide (49, 50), because of their fast growth, hardiness, omnivore diet, resistance to low oxygen concentrations, easy farm management, and pleasant flavor with fewer bones (51). Toxocara eggs in open water from infected dogs and cats may also embryonate without fish presence and may develop into infective stages. Moreover, the fish gastrointestinal tract is mostly removed before human consumption, and when left it may be killed or inactivated by cooking or microwave, as previously shown (52). However, the role of raw fish meat as foodborne toxocarosis source remains to be fully established. Accordingly, this study aimed to experimentally assess Nile tilapia as potential paratenic host of Toxocara spp.
Method
Fish Selection and Maintenance
This study has been approved by the Ethics Committee of Animal Use of the São Paulo Western University (UNOESTE) (Protocol Number 4,299). Nile tilapias ranging from 7 to 10 cm in size, weighing between 20 and 31 g, and ~3 months old were randomly obtained in the fish farming section of the Zootechnical Center at the São Paulo Western University (UNOESTE). The fish was first transferred to a depuration tank with a water recirculation system and air compressor aeration for 7 days before the experiment, as previously recommended (50).
During the adjustment period, a fecal examination of each tilapia using flotation and centrifugal sedimentation (53) was performed to ensure the absence of coccidia and helminths.
After inoculation with T. canis eggs, each fish was individually housed in a 5-liter polyethylene tank throughout the post-inoculation period. Fish tanks were maintained in a controlled environment with 12-h light-dark cycles at 25 ± 5°C, constant water tank oxygenation by an air compressor, and fed twice a day until apparent satiation with commercially available fish food (Acqua 32 Matsuda®, São Paulo, Brazil).
Experimental Design
Egg shedding into tank water and fish larvae migration were assessed at 16, 24, 48, 72, and 240 h post-inoculation. The inoculated group (IG) consisted of 15 fish inoculated with 300 T. canis embryonated eggs, and the control group (CG) consisted of five fish inoculated with distilled water (Table 1). Three inoculated and one control fish were euthanized and examined for larvae migration in each post-inoculation time.
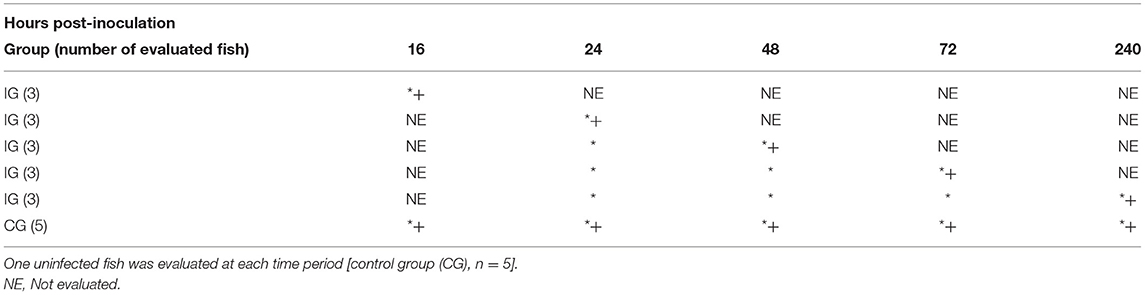
Table 1. Assessment of T. canis eggs of the aquatic environment (*) and larvae in fish tissues (+), after Nile tilapia experimental inoculation [inoculated group (IG), n = 15] with embryonated eggs.
A period of 16 h was set as the first fish assessment due to the minimal amount of time for food to pass into the intestine in Nile tilapia, as previously observed (54). Only one subgroup of (n = 3) fish was assessed at 16 h to avoid sampling stress on transfer to another tank in such a short interval.
Recovery of Toxocara canis Eggs
Toxocara canis eggs were recovered according to a previously described protocol (55), with minor modifications. In short, adult T. canis females were recovered from feces shed by naturally infected puppies. Adult female parasites were washed with saline solution and hysterectomized. Eggs were incubated in 2% formalin solution for at least 30 days at 25 ± 2°C.
After incubation and confirmed embryonation, the eggs were washed with saline solution and centrifuged at 697 g for 3 min, the embryonated eggs were then placed on histological slides, and 300 units counted, as previously established (56). Eggs were transferred to plastic tubes containing 20 μL of distilled water, later used for fish inoculation.
Fish Inoculation
Fish were sedated by immersion in an anesthetic solution of 50 mg of benzocaine diluted in ethanol until loss of equilibrium, as previously established (57). A total of 300 T. canis embryonated eggs were administered by oral gavage with a needle designed for mice (55). An additional 20 μL of distilled water was then administered to ensure successful egg ingestion. After inoculation, fish were individually monitored until equilibrium and external stimuli recovery and stabilization and then housed into a 5-L polyethylene tank throughout the post-inoculation period. Fish in the CG were orally given the same volume of 40 μL of distilled water.
Egg Shedding Assessment
Tank water was filtered through 212- and 38-μm metal sieves to collect T. canis eggs. The filtered material was collected using a plastic pipette, transferred to a conical bottom tube (15 mL), and centrifuged at 697 g for 3 min to concentrate sediments. After centrifugation, the supernatant was discarded, and sediment was observed under an optical microscope at 100× and 400× magnifications to quantify recovered eggs.
After each egg shedding observation, the fish were individually transferred to new tanks with clean water, while old tanks were flushed with abundant water and filtered to obtain eggs potentially adhered to tank plastic walls.
Assessment of T. canis Larvae in Fish Tissues
As already described, three inoculated and one control fish were euthanized and examined at 16, 24, 48, 72, and 240 post-inoculation hours to assess larval tissue migration (Table 1). Fish were immersed in an anesthetic solution of 50 mg of benzocaine diluted in ethanol, following previously described protocol (57). After observing no opercular movement and permanent stasis at the plastic tank bottom, fish were euthanized by spinal cord section (58).
Fish were dissected using forceps and scalpel blade; liver, stomach, intestines, gills, eyes, central nervous system, and lateral portion of the fish's musculature were extracted. Each organ was individually grounded in Petri dishes and subjected to acid digestion with 5 g pepsin and 10 mL HCl 37% in distilled water for 6 h at 37°C under agitation, as recommended (59). After digestion, tissue material was filtered through a 300 μm sieve and centrifuged at 697 g for 3 min.
Egg and larvae assessment in the GIT included stomach and intestines. Larvae were assessed thoroughly observing the final material with a light microscope at 100× and 400× magnifications.
Assessment of T. canis Egg Infectivity in Mice
Two 5–7 weeks old male Swiss mice (Mus musculus), weighing ~50 g were maintained in a controlled environment at the Experimental Laboratory at UNOESTE, with 12-h light-dark cycles at 22 ± 2°C, and provided with commercial food and water ad libitum.
Assessment of T. canis egg infectivity after passing throughout the fish GIT was based on bioassay, as previously described (35). One tilapia was inoculated with embryonated (n = 300) and one with unembryonated (n = 300) T. canis eggs, following the same procedure described previously, except that eggs were retrieved from the tank water after 48 post-inoculation.
Following retrieval, 50 eggs shed by the tilapia inoculated with embryonated eggs were used for one mice inoculation. Recovered unembryonated eggs were used to inoculate the other mice but first maintained in 2% formalin solution in a temperature-controlled environment (27 ± 3°C) for embryonation and larval development. Then, the material was washed three times with saline solution by centrifugation at 679 g for 3 min, and 50 eggs were counted for inoculation.
The inoculation was achieved by oral gavage with 100 μL of buffered saline solution containing 50 eggs in each mouse, following a protocol previously described (60). The two mice were euthanized in a CO2 chamber 48 h after inoculation and necropsied for liver extraction. Larvae recovery was achieved using 1 g of the liver samples subjected to the previously described Baermann technique (41).
Statistical Analysis
The dispersion was assessed, assuming that the concentration of eggs and larvae in the fish and water tank could be described by a Poisson distribution or negative binomial distribution (61, 62). Thus, a generalized linear model was proposed, in which the dependent variable was the count of eggs and larvae and the time in hours was the independent variable. The counts of eggs and larvae were super-dispersed, so a negative binomial distribution was used to describe the data (63).
Spearman non-parametric correlation analysis was used to describe the relationship between the counts of eggs and larvae in fish and water tank, accumulated for all evaluated time points. A significance of p < 0.05 was used. The statistical analysis was performed using R (64).
Results
The recovery rate of egg and larvae shedding and retained in fish GIT ranged from 23 to 86% (mean = 48.4%) per fish. Approximately three-quarters of eggs and larvae were recovered from the water tank (76.3%), and one-quarter of eggs and larvae from the GIT (23.7%). The shedding of embryonated eggs was first detected 16 h post-inoculation and was observed until the end of the experiment at 240 h.
The counting of eggs and larvae observed in the fish GIT was negatively correlated with the number of eggs and larvae identified in the water tank (rho = −0.698, p = 0.003). Regression models have shown a statistically significant decrease over time in egg shedding into the water tank (p = 0.001) and the presence of T. canis eggs in the fish GIT (p = 0.007; Table 2 and Figure 1).
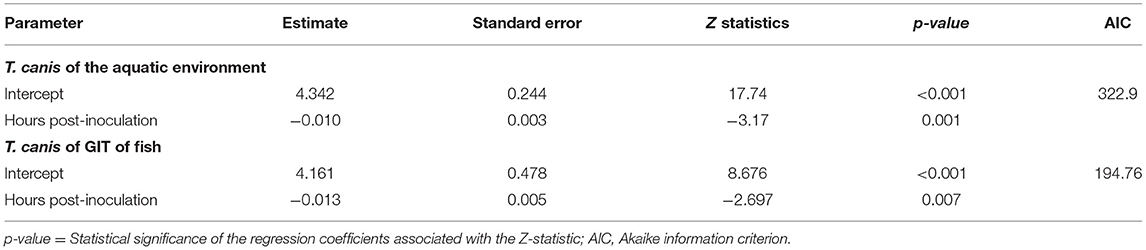
Table 2. Negative binomial regression model to assessment T. canis eggs and larvae recovered of the aquatic environment and in the gastrointestinal tracts (GIT) of Nile tilapia experimentally inoculated with T. canis embryonated eggs, overtime.
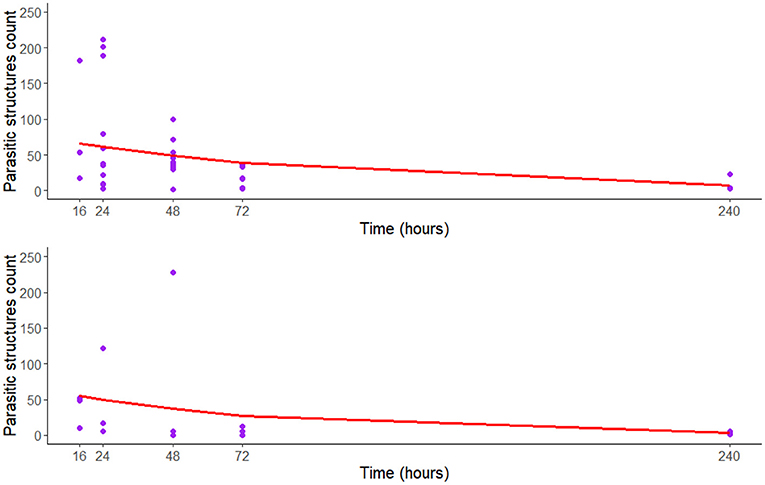
Figure 1. Regression curve of the negative binomial model to assessment T. canis eggs and larvae recovered of aquatic environment (upper) and in the gastrointestinal tracts (GIT) of Nile tilapia (lower) experimentally inoculated with T. canis embryonated eggs, overtime (n = 15).
Although some larvae were found in the water tank and fish GIT, no larvae were recovered from fish tissues. However, the bioassay has shown larvae in the digested liver of both mice, recovered at 48-h post-inoculation, with 5/50 (10.0%) and 9/50 (18.0%) larvae, for inoculation with unembryonated and embryonated eggs, respectively.
No fish died or presented any behavioral changes in either fish group. No other eggs and larvae were identified in the water, GIT, or in fish tissues. After abundantly washing the metallic meshes with water, no egg was retained in the sieves used to filter the plastic water tanks' material.
Discussion
The presence of T. canis eggs into the water tanks herein has confirmed the capacity of egg shedding and dispersion into the aquatic environment by the Nile tilapia, as previously observed in other paratenic hosts and mechanical carriers experimentally infected with T. canis embryonated eggs, such as chickens (60), and cockroaches (65, 66). Not surprisingly, toxocarosis can be transmitted to human beings by ingestion of invertebrate hosts such as snails (67, 68) and earthworms (69), which may play a role as paratenic, mechanical, or biological hosts.
The egg recovery rate was 48.4% on average. Eggs were observed in tanks after 16 h post-inoculation, and fewer eggs were observed over time. The higher shedding of eggs occurred at 24 and 48 h post-inoculation, as previously observed in cockroaches inoculated with T. canis eggs (66). As expected, egg shedding was inversely proportional to the larvae presence in the tilapia GIT, consistent with observations in chicken (60) and cockroaches (65). The wide variation herein in egg and larval recovery per fish (23 to 86%) shows the absence of an egg dispersal pattern as observed in chickens (60).
In this study, we applied standard metallic sieves of different mesh sizes, which have been used to recover Toxocara spp. eggs from dog or cat fur (70, 71) and soil samples (72, 73). However, no egg was retained in the meshes employed for filtering the organic material from the plastic water tanks.
Toxocara spp. eggs, especially those of T. canis, tend to adhere to different materials, especially plastic (74). It is possible that the plastic water tanks may have favored egg adhesion and influenced egg recovery, despite the abundant washing during the filtering process.
As previously established, some nematodes may be transmitted by ingestion of raw/undercooked fish, after dogs/cats shed unembryonated eggs in water where they become embryonated, and larvae develop in the tissue of the intermediate fish host, as observed in Gnathostoma spp. (75). Despite the presence of eggs in the tank water and the GIT of the fish, no evidence of T. canis larvae migration into fish tissue was observed herein, as already observed in cockroaches and dog puppy tissues that were experimentally infected with feces of cockroaches containing embryonated eggs and larvae of T. canis (65). The authors hypothesize that the absence of migration may be due to the fish's poikilothermic characteristics, with GITs providing poor quality conditions for T. canis larvae hatching and tissue migration. On the other hand, homeothermic species such as dogs and cats have been confirmed as definitive hosts of Toxocara spp., and other homeothermic animals effectively play a role as intermediate hosts (76).
In this study, a few undamaged motile larvae were retrieved from tanks and the GITs of infected tilapia, which may be likely due to the rupture of eggs during sample processing, since eggshells mainly were observed near the larvae. Both embryonated and unembryonated T. canis eggs have the potential of infecting mice in experimental conditions after passing throughout the tilapia GIT, as observed in chicken (60). Although no larvae were found in Nile tilapia tissues herein, T. canis eggs and larvae were present in the tilapia GIT, and shedding eggs and larvae sustained infectivity for mice bioassay. Thus, Nile tilapia and other commercial fish may play a dispersion role of viable T. canis eggs.
Nile tilapia's ability to disperse T. canis eggs into the water tank should be considered in the epidemiological chain of toxocarosis. Non-dewormed dogs and cats infected by Toxocara spp. may live near fish breeding farms, and their feces can contaminate ponds and tanks used for fish farming (77). Dogs often enter the water to cool off, particularly on hot days (78), which may contaminate water resources and maintain the life cycle of parasites that have fish and other aquatic animals as intermediate or paratenic hosts (77). The presence of non-dewormed companion animals should be a public health concern in commercial fish farms. Restricting pet access to fish farms, feeding care, and pet regular anthelmintic treatment can reduce water resources contamination by parasite eggs (48, 79).
Despite Toxocara eggs in open water from infected dogs and cats may also embryonate without the presence of tilapia fish and develop into infective stages, the study herein has shown no evidence of larvae in the tilapia tissues. Thus, as tilapia act not as paratenic host, intake of raw fish meat may present no alimentary zoonotic infection risk. The fish, like other animals (e.g., dogs with coprophagy that eat cat feces) and human beings will just (partially or fully) pass developed eggs trough the gastrointestinal tract after ingestion, which may be removed from fish before human consumption. Although fish contamination with (embryonated) eggs may be possible with water contamination, as previously observed in irrigated vegetables (80), the most important conclusion from the present study has been that tilapia is not a suitable paratenic host for Toxocara spp.
One limitation of our study is the low number of animals included in the experimental design. Further studies should be conducted considering a larger number of animals and different dilution of embryonated eggs, to assess the sensibility of the fish to became infected. Nonetheless, to the authors knowledge, this is the first experimental study in which Nile tilapia has been tested, as previously experimentally performed in several other host species, infected with T. canis embryonated eggs to assess its role of infection carrier and potential risk of foodborne transmission.
Finally, as cross-contamination and human infection by parasitic agents may also occur during the handling and preparation of fish-based dishes (8), appropriate management practices, including training of fish handlers and workers in the production fish chain may minimize the impacts of fish-transmitted zoonoses.
The study herein has been the first experimental T. canis infection in Nile tilapia to assess its role as carrier. In conclusion, despite shedding viable embryonated eggs through the gastrointestinal tract, tilapias may not play a role as a suitable paratenic hosts for Toxocara spp., posing low risk of zoonotic transmission by fish meat consumption.
Data Availability Statement
The original contributions presented in the study are included in the article/supplementary material, further inquiries can be directed to the corresponding author.
Ethics Statement
This animal study was approved by the Ethics Committee of Animal Use of the São Paulo Western University—Brazil (protocol number 4299).
Author Contributions
EO, YM, IF, IG, AP, RS, and VS performed experimental study and analysis. EO, RG, and VS wrote the first draft of the manuscript. EO, YM, IF, IG, AP, RS, LK, AS, AB, RG, and VS wrote sections of the manuscript. All authors contributed to data collection, data analysis, manuscript revision, read, and approved the submitted version.
Funding
This study was financed in part by the Coordenação de Aperfeiçoamento de Pessoal de Nível Superior e Brasil (Capes)—Finance Code 001, provided for YM.
Conflict of Interest
The authors declare that the research was conducted in the absence of any commercial or financial relationships that could be construed as a potential conflict of interest.
References
1. Macpherson CNL. Human behaviour and the epidemiology of parasitic zoonoses. Int J Parasitol. (2005) 35:1319–31. doi: 10.1016/j.ijpara.2005.06.004
2. Mehrdana F, Buchmann K. Excretory/secretory products of anisakid nematodes: biological and pathological roles. Acta Vet Scand. (2017) 59:42. doi: 10.1186/s13028-017-0310-3
3. Carbotta G, Laforgia R, Milella M, Sederino MG, Minafra M, Fortarezza F, et al. Small bowel obstruction caused by Anisakis and Meckel's diverticulum: a rare case. G Chir. (2016) 37:281–3. doi: 10.11138/gchir/2016.37.6.281
4. Bao M, Pierce GJ, Pascual S, González-Muñoz M, Mattiucci S, Mladineo I, et al. Assessing the risk of an emerging zoonosis of worldwide concern: anisakiasis. Sci Rep. (2017) 7:43699. doi: 10.1038/srep43699
5. Cornaglia J, Jean M, Bertrand K, Aumaître H, Roy M, Nickel B. Gnathostomiasis in Brazil: an emerging disease with a challenging diagnosis. J Travel Med. (2016) 24:taw074. doi: 10.1093/jtm/taw074
6. Zhang M, Yang Z, Wang S, Tao L, Xu L, Yan R, et al. Detection of Toxoplasma gondii in shellfish and fish in parts of China. Vet Parasitol. (2014) 200:85–9. doi: 10.1016/j.vetpar.2013.10.022
7. Sanders JL, Zhou Y, Moulton HM, Moulton ZX, McLeod R, Dubey JP, et al. The zebrafish, Danio rerio, as a model for Toxoplasma gondii: an initial description of infection in fish. J Fish Dis. (2015) 38:675–9. doi: 10.1111/jfd.12393
8. Tran TKC, Murrell KD, Madsen H, Nguyen VK, Dalsgaard A. Fishborne zoonotic trematodes in raw fish dishes served in restaurants in Nam Dinh Province and Hanoi, Vietnam. J Food Prot. (2009) 72:2394–9. doi: 10.4315/0362-028X-72.11.2394
9. Ma G, Holland CV, Wang T, Hofmann A, Fan C-K, Maizels RM, et al. Human toxocariasis. Lancet Infect Dis. (2018) 18:e14–24. doi: 10.1016/S1473-3099(17)30331-6
10. Kassai T. Nomenclature for parasitic diseases: cohabitation with inconsistency for how long and why? Vet Parasitol. (2006) 138:169–78. doi: 10.1016/j.vetpar.2006.02.019
11. Kassai T, Cordero del Campillo M, Euzeby J, Gaafar S, Hiepe T, Himonas CA. Standardized nomenclature of animal parasitic diseases (SNOAPAD). Vet Parasitol. (1988) 29:299–326. doi: 10.1016/0304-4017(88)90148-3
12. CDC—Parasites—Neglected Parasitic Infections (NPIs) in the United States. Available online at: https://www.cdc.gov/parasites/npi/ (accessed May 11, 2021).
13. Rubinsky-Elefant G, Hirata CE, Yamamoto JH, Ferreira MU. Human toxocariasis: diagnosis, worldwide seroprevalences and clinical expression of the systemic and ocular forms. Ann Trop Med Parasitol. (2010) 104:3–23. doi: 10.1179/136485910X12607012373957
14. Gakosso LGC, Baadi F, Abakka FZ, Basraoui D, Jalal H. The visceral larva migrans caused by Toxocara canis: a case report. Pan Afr Med J. (2020) 36:150. doi: 10.11604/pamj.2020.36.150.24176
15. Aghaei S, Riahi SM, Rostami A, Mohammadzadeh I, Javanian M, Tohidi E, et al. Toxocara spp. infection and risk of childhood asthma: a systematic review and meta-analysis. Acta Trop. (2018) 182:298–304. doi: 10.1016/j.actatropica.2018.03.022
16. Lu CY, Lai SC, Lee HH, Chien HT, Lan KP, Chen K-M. Matrix metalloproteinases activation in Toxocara canis induced pulmonary pathogenesis. J Microbiol Immunol Infect. (2020). doi: 10.1016/j.jmii.2020.07.022. [Epub ahead of print].
17. Fonseca C, Silva AM, Freire S, Proença R. Ocular toxocariasis: atypical clinical course. BMJ Case Rep. (2019) 12:e228717. doi: 10.1136/bcr-2018-228717
18. Martinez J, Ivankovich-Escoto G, Wu L. Pediatric ocular toxocariasis in costa rica: 1998-2018 experience. Ocul Immunol Inflamm. (2020). doi: 10.1080/09273948.2020.1792513. [Epub ahead of print].
19. Nicoletti A. Neurotoxocariasis. Adv Parasitol. (2020) 109:219–31. doi: 10.1016/bs.apar.2020.01.007
20. Docu Axelerad A, Stroe AZ, Gogu AE, Pusztai A, Jianu DC, Daniel D, et al. Clinical spectrum of symptoms in cerebral Toxocariasis (Review). Exp Ther Med. (2021) 21:521. doi: 10.3892/etm.2021.9953
21. Magnaval JF, Glickman LT, Dorchies P, Morassin B. Highlights of human toxocariasis. Korean J Parasitol. (2001) 39:1–11. doi: 10.3347/kjp.2001.39.1.1
22. Overgaauw PAM, van Knapen F. Veterinary and public health aspects of Toxocara spp. Vet Parasitol. (2013) 193:398–403. doi: 10.1016/j.vetpar.2012.12.035
23. Simonato G, Cassini R, Morelli S, Di Cesare A, La Torre F, Marcer F, et al. Contamination of Italian parks with canine helminth eggs and health risk perception of the public. Prev Vet Med. (2019) 172:104788. doi: 10.1016/j.prevetmed.2019.104788
24. Gillespie S, Bradbury RS. A survey of intestinal parasites of domestic dogs in central Queensland. Trop Med Infect Dis. (2017) 2:60. doi: 10.3390/tropicalmed2040060
25. Fakhri Y, Gasser RB, Rostami A, Fan CK, Ghasemi SM, Javanian M, et al. Toxocara eggs in public places worldwide—a systematic review and meta-analysis. Environ Pollut. (2018) 242:1467–75. doi: 10.1016/j.envpol.2018.07.087
26. Medina-Pinto RA, Rodríguez-Vivas RI, Bolio-González ME. Zoonotic intestinal nematodes in dogs from public parks in Yucatán, México. Biomedica. (2018) 38:105–10. doi: 10.7705/biomedica.v38i0.3595
27. Nagamori Y, Payton ME, Looper E, Apple H, Johnson EM. Retrospective survey of endoparasitism identified in feces of client-owned dogs in North America from 2007 through 2018. Vet Parasitol. (2020) 282:109137. doi: 10.1016/j.vetpar.2020.109137
28. Diakou A, Di Cesare A, Morelli S, Colombo M, Halos L, Simonato G, et al. Endoparasites and vector-borne pathogens in dogs from Greek islands: pathogen distribution and zoonotic implications. PLoS Negl Trop Dis. (2019) 13:e0007003. doi: 10.1371/journal.pntd.0007003
29. Lempereur L, Nijsse R, Losson B, Marechal F, De Volder A, Schoormans A, et al. Coprological survey of endoparasite infections in owned dogs and owners' perceptions of endoparasite control in Belgium and the Netherlands. Vet Parasitol Reg Stud Rep. (2020) 22:100450. doi: 10.1016/j.vprsr.2020.100450
30. Abdel Aziz AR, Hassan AA, Elmahallawy EK, Elshahawy IS, Almuzaini AM. Prevalence and associated risk factors of Toxocara infection in dogs in northern and southern Egypt. Vet Parasitol Reg Stud Rep. (2019) 17:100305. doi: 10.1016/j.vprsr.2019.100305
31. Rostami A, Riahi SM, Hofmann A, Ma G, Wang T, Behniafar H, et al. Global prevalence of Toxocara infection in dogs. Adv Parasitol. (2020) 109:561–83. doi: 10.1016/bs.apar.2020.01.017
32. Rostami A, Sepidarkish M, Ma G, Wang T, Ebrahimi M, Fakhri Y, et al. Global prevalence of Toxocara infection in cats. Adv Parasitol. (2020) 109:615–39. doi: 10.1016/bs.apar.2020.01.025
33. Rostami A, Riahi SM, Holland CV, Taghipour A, Khalili-Fomeshiid M, Fakhri Y, et al. Seroprevalence estimates for toxocariasis in people worldwide: a systematic review and meta-analysis. PLoS Negl Trop Dis. (2019) 13:e0007809. doi: 10.1371/journal.pntd.0007809
34. Salem G, Schantz P. Toxocaral visceral larva migrans after ingestion of raw lamb liver. Clin Infect Dis. (1992) 15:743–4. doi: 10.1093/clind/15.4.743
35. Stürchler D, Weiss N, Gassner M. Transmission of toxocariasis. J Infect Dis. (1990) 162:571. doi: 10.1093/infdis/162.2.571
36. Yoshikawa M, Nishiofuku M, Moriya K, Ouji Y, Ishizaka S, Kasahara K, et al. A familial case of visceral toxocariasis due to consumption of raw bovine liver. Parasitol Int. (2008) 57:525–9. doi: 10.1016/j.parint.2008.08.002
37. Davidson RK, Mermer A, Øines Ø. Toxocara cati larva migrans in domestic pigs—detected at slaughterhouse control in Norway. Acta Vet Scand. (2012) 54:66. doi: 10.1186/1751-0147-54-66
38. de Oliveira AC, Rubinsky-Elefant G, Merigueti YFFB, Batista A da S, Santarém VA. Frequency of anti-Toxocara antibodies in broiler chickens in southern Brazil. Rev Bras Parasitol Vet. (2018) 27:141–5. doi: 10.1590/s1984-296120180025
39. Morimatsu Y, Akao N, Akiyoshi H, Kawazu T, Okabe Y, Aizawa H. A familial case of visceral larva migrans after ingestion of raw chicken livers: appearance of specific antibody in bronchoalveolar lavage fluid of the patients. Am J Trop Med Hyg. (2006) 75:303–6. doi: 10.4269/ajtmh.2006.75.303
40. Noh Y, Hong S-T, Yun JY, Park H-K, Oh J-H, Kim YE, et al. Meningitis by Toxocara canis after ingestion of raw ostrich liver. J Korean Med Sci. (2012) 27:1105–8. doi: 10.3346/jkms.2012.27.9.1105
41. Kong L, Peng H-J. Chapter twenty-one—current epidemic situation of human toxocariasis in China. In: Bowman DDBT-A, editor. Toxocara and Toxocariasis. Cambridge, MA: Academic Press (2020). p. 433–48.
42. Song HB, Lee D, Jin Y, Kang J, Cho S-H, Park MS, et al. Prevalence of toxocariasis and its risk factors in patients with Eosinophilia in Korea. Korean J Parasitol. (2020) 58:413–9. doi: 10.3347/kjp.2020.58.4.413
43. Strube C, Heuer L, Janecek E. Toxocara spp. infections in paratenic hosts. Vet Parasitol. (2013) 193:375–89. doi: 10.1016/j.vetpar.2012.12.033
44. Kyei G, Ayi I, Boampong JN, Turkson PK. Sero-epidemiology of Toxocara canis infection in children attending four selected health facilities in the central region of Ghana. Ghana Med J. (2015) 49:77–83. doi: 10.4314/gmj.v49i2.3
45. Krücken J, Blümke J, Maaz D, Demeler J, Ramünke S, Antolová D, et al. Small rodents as paratenic or intermediate hosts of carnivore parasites in Berlin, Germany. PLoS ONE. (2017) 12:e0172829. doi: 10.1371/journal.pone.0172829
46. Taira K, Permin A, Kapel CMO. Establishment and migration pattern of Toxocara canis larvae in chickens. Parasitol Res. (2003) 90:521–3. doi: 10.1007/s00436-003-0894-6
47. Thu ND, Dalsgaard A, Loan LTT, Murrell KD. Survey for zoonotic liver and intestinal trematode metacercariae in cultured and wild fish in An Giang Province, Vietnam. Korean J Parasitol. (2007) 45:45–54. doi: 10.3347/kjp.2007.45.1.45
48. Nissen S, Nguyen LAT, Thamsborg SM, Dalsgaard A, Johansen MV. Reinfection of dogs with fish-borne zoonotic trematodes in northern Vietnam following a single treatment with praziquantel. PLoS Negl Trop Dis. (2014) 8:e2625. doi: 10.1371/journal.pntd.0002625
49. Melo RMC, Ribeiro YM, Luz RK, Bazzoli N, Rizzo E. Influence of low temperature on structure and dynamics of spermatogenesis during culture of Oreochromis niloticus. Anim Reprod Sci. (2016) 172:148–56. doi: 10.1016/j.anireprosci.2016.07.013
50. El-Leithy AAA, Hemeda SA, El Naby WSHA, El Nahas AF, Hassan SAH, Awad ST, et al. Optimum salinity for Nile tilapia (Oreochromis niloticus) growth and mRNA transcripts of ion-regulation, inflammatory, stress- and immune-related genes. Fish Physiol Biochem. (2019) 45:1217–32. doi: 10.1007/s10695-019-00640-7
51. FAO Fisheries & Aquaculture—Cultured Aquatic Species Information Programme—Oreochromis niloticus (Linnaeus 1758). Available online at: http://www.fao.org/fishery/culturedspecies/Oreochromis_niloticus/en (accessed March 3, 2021)
52. Çetinkaya H, Gargili A, Alta SK. Effects of microwave cooking on the infectivity of Toxocara canis (Werner, 1782) larvae in the liver of paratenic host mice. Turkish J Vet Anim Sci. (2006) 30:533–8. Available online at: https://dergipark.org.tr/tr/download/article-file/132551 (accessed June 2, 2021).
53. Taylor M, Coop RL, Wall R. Veterinary Parasitology Fourth edition, Chichester; West Sussex; Ames, IA: John Wiley and Sons (2016).
54. Carvalho JSO, Ramos APS, Azevedo RV, Braga LGT. Trânsito gastrintestinal de dieta seca para tilápia de 200 e 300 g (2016). Available online at: http://www.uesc.br/laboratorios/aquanut/conbr.pdf (accessed April 12, 2019).
55. Pecinali NR, Gomes RN, Amendoeira FC, Bastos ACMP, Martins MJQA, Pegado CS, et al. Influence of murine Toxocara canis infection on plasma and bronchoalveolar lavage fluid eosinophil numbers and its correlation with cytokine levels. Vet Parasitol. (2005) 134:121–30. doi: 10.1016/j.vetpar.2005.06.022
56. Santos SV, Dos Santos FHY, Lescano SAZ, Santos DM, Dos Tiago É da S, Fonseca GRE, et al. Migration pattern of Toxocara canis larvae in experimentally infected male and female Rattus norvegicus. Rev Soc Bras Med Trop. (2017) 50:698–700. doi: 10.1590/0037-8682-0076-2017
57. Neiffer DL, Stamper MA. Fish sedation, analgesia, anesthesia, and euthanasia: considerations, methods, and types of drugs. ILAR J. (2009) 50:343–60. doi: 10.1093/ilar.50.4.343
58. Pedrazzani AAS, Molento CCFM, Carneiro PCF, Fernandes M. Senciência e bem-estar de peixes: uma visão de futuro do mercado consumidor. Panor da Aqüicultura. (2007) 102:24–9. Available online at: https://prp.ufla.br/wp-content/uploads/2011/08/bem-estar-em-peixes.pdf (accessed June 2, 2021).
59. Azizi S, Oryan A, Sadjjadi SM, Zibaei M. Histopathologic changes and larval recovery of Toxocara cati in experimentally infected chickens. Parasitol Res. (2007) 102:47–52. doi: 10.1007/s00436-007-0722-5
60. Merigueti YFFB, da Silva Raposo R, Zampieri BP, de Lima Cerazo LM, Pereira L, Santarém VA. Dispersion and infectivity of Toxocara canis eggs after passage through chicken intestine. Parasitol Res. (2018) 117:3481–6. doi: 10.1007/s00436-018-6045-x
61. Wilson K, Grenfell BT. Generalized linear modelling for parasitologists. Parasitol Today. (1997) 13:33–8. doi: 10.1016/S0169-4758(96)40009-6
62. Alexander N. Review: analysis of parasite and other skewed counts. Trop Med Int Heal. (2012) 17:684–93. doi: 10.1111/j.1365-3156.2012.02987.x
63. Liboschik T, Fried R, Fokianos K, Probst P. tscount: Analysis of Count Time Series. R package version 1.4.1. (2017). Available online at: https://CRAN.R-project.org/package=tscount
64. R Core Team. R: A Language and Environment for Statistical Computing. R Foundation for Statistical Computing. Vienna (2019). Available online at: https://www.R-project.org/
65. Sasmal NK, Pahari TK, Laha R. Experimental infection of the cockroach Periplaneta americana with Toxocara canis and the establishment of patent infections in pups. J Helminthol. (2008) 82:97–100. doi: 10.1017/S0022149X07875936
66. González-García T, Muñoz-Guzmán MA, Sánchez-Arroyo H, Prado-Ochoa MG, Cuéllar-Ordaz JA, Alba-Hurtado F. Experimental transmission of Toxocara canis from Blattella germanica and Periplaneta americana cockroaches to a paratenic host. Vet Parasitol. (2017) 246:5–10. doi: 10.1016/j.vetpar.2017.08.025
67. Caldera F, Burlone ME, Genchi C, Pirisi M, Bartoli E. Toxocara encephalitis presenting with autonomous nervous system involvement. Infection. (2013) 41:691–4. doi: 10.1007/s15010-012-0342-6
68. Cardillo N, Prous CG, Krivokapich S, Pittaro M, Ercole M, Perez M, et al. Primer reporte de Toxocara cati en el caracol doméstico Rumina decollata. Rev Argent Microbiol. 2016;48(3):206–9. doi: 10.1016/j.ram.2016.04.004
69. Cianferoni A, Schneider L, Schantz PM, Brown D, Fox LM. Visceral larva migrans associated with earthworm ingestion: clinical evolution in an adolescent patient. Pediatrics. (2006) 117:e336LP−9. doi: 10.1542/peds.2005-1596
70. Anh NTL, Thuy DTT, Hoan DH, Hop NT, Dung DT. Levels of Toxocara infections in dogs and cats from urban Vietnam together with associated risk factors for transmission. J Helminthol. (2016) 90:508–10. doi: 10.1017/S0022149X15000619
71. Merigueti YFFB, Santarém VA, Ramires LM, da Silveira Batista A, da Costa Beserra LV, Nuci AL, et al. Protective and risk factors associated with the presence of Toxocara spp. eggs in dog hair. Vet Parasitol. (2017) 244:39–43. doi: 10.1016/j.vetpar.2017.07.020
72. Ferreira A, Alho AM, Otero D, Gomes L, Nijsse R, Overgaauw PAM, et al. Urban dog parks as sources of canine parasites: contamination rates and pet owner behaviours in Lisbon, Portugal. Buckley B, editor. J Environ Public Health. (2017) 2017:5984086. doi: 10.1155/2017/5984086
73. Choobineh M, Mikaeili F, Sadjjadi SM, Ebrahimi S, Iranmanesh S. Molecular characterization of Toxocara spp. eggs isolated from public parks and playgrounds in Shiraz, Iran. J Helminthol. (2019) 93:306–12. doi: 10.1017/S0022149X18000354
74. Kleine A, Janecek E, Waindok P, Strube C. Flotation and adherence characteristics of Toxocara canis and T. cati and a reliablemethod for recovering Toxocara eggs from soil. Vet Parasitol. (2016) 227:35–41. doi: 10.1016/j.vetpar.2016.07.023
75. Eiras JC, Pavanelli GC, Takemoto RM, Nawa Y. Fish-borne nematodiases in South America: neglected emerging diseases. J Helminthol. (2018) 92:649–54. doi: 10.1017/S0022149X17001006
76. Jacobs DE, Pegg EF, Stevenson P. Helminths of British dogs: Toxocara canis–a veterinary perspective. J Small Anim Pract. (1977) 18:79–92. doi: 10.1111/j.1748-5827.1977.tb05859.x
77. Lima dos Santos CAM, Howgate P. Fishborne zoonotic parasites and aquaculture: a review. Aquaculture. (2011) 318:253–61. doi: 10.1016/j.aquaculture.2011.05.046
78. Otake Sato M, Sato M, Yoonuan T, Pongvongsa T, Sanguankiat S, Kounnavong S, et al. The role of domestic dogs in the transmission of zoonotic helminthes in a rural area of Mekong river basin. Acta Parasitol. (2017) 62:393–400. doi: 10.1515/ap-2017-0047
79. Awadallah MAI, Salem LMA. Zoonotic enteric parasites transmitted from dogs in Egypt with special concern to Toxocara canis infection. Vet World. (2015) 8:946–57. doi: 10.14202/vetworld.2015.946-957
Keywords: environmental contamination, fish, toxocariasis, transmission, zoonosis
Citation: de Oliveira EA, Merigueti YFFB, Ferreira IB, Garcia IS, Pereira AS, Santos RdS, Kmetiuk LB, Santos APd, Biondo AW, Giuffrida R and Santarém VA (2021) The Role of Nile Tilapia (Oreochromis niloticus) in the Life Cycle of Toxocara spp. Front. Vet. Sci. 8:685911. doi: 10.3389/fvets.2021.685911
Received: 26 March 2021; Accepted: 21 May 2021;
Published: 17 June 2021.
Edited by:
Donato Traversa, University of Teramo, ItalyReviewed by:
Paul Overgaauw, Utrecht University, NetherlandsFabrizia Veronesi, University of Perugia, Italy
Copyright © 2021 de Oliveira, Merigueti, Ferreira, Garcia, Pereira, Santos, Kmetiuk, Santos, Biondo, Giuffrida and Santarém. This is an open-access article distributed under the terms of the Creative Commons Attribution License (CC BY). The use, distribution or reproduction in other forums is permitted, provided the original author(s) and the copyright owner(s) are credited and that the original publication in this journal is cited, in accordance with accepted academic practice. No use, distribution or reproduction is permitted which does not comply with these terms.
*Correspondence: Vamilton Alvares Santarém, dmFtaWx0b24mI3gwMDA0MDt1bm9lc3RlLmJy