- 1State Engineering Technology Institute for Karst Desertfication Control, School of Karst Science, Guizhou Normal University, Guiyang, China
- 2State Key Laboratory of Grassland Agro-Ecosystems, International Centre for Tibetan Plateau Ecosystem Management, Engineering Research Center of Arid Agriculture and Ecological Remediation of Ministry of Education, School of Life Sciences, Lanzhou University, Lanzhou, China
Phosphorus is one of the essential mineral elements of animals that plays an important role in animal growth and development, bone formation, energy metabolism, nucleic acid synthesis, cell signal transduction, and blood acid–base balance. It has been established that the Type IIb sodium-dependent phosphate cotransporters (NaPi-IIb) protein is the major sodium-dependent phosphate (Pi) transporter, which plays an important role in Pi uptake across the apical membrane of epithelial cells in the small intestine. Previous studies have demonstrated that epidermal growth factor (EGF) is involved in regulating intestinal Pi absorption. Here we summarize the effects of EGF on active Pi transport of NaPi-IIb under different conditions. Under normal conditions, EGF inhibits the active transport of Pi by inhibiting the expression of NaPi-IIb, while, under intestinal injury condition, EGF promotes the active absorption of Pi through upregulating the expression of NaPi-IIb. This review provides a reference for information about EGF-regulatory functions in Pi absorption in the animal intestine.
Introduction
Phosphorus is one of the most abundant elements in mammals involved in a variety of physiologic processes in the form of inorganic phosphates (Pi), including cellular signaling, energy metabolism, and nucleotide biosynthesis, and is an important component of cell membranes and bones (1–4). As an important site for Pi absorption, the small intestine plays a crucial role in Pi homeostasis, which accounts for more than 70% of the Pi absorption (5). It is well-known that intestinal Pi absorption by the paracellular route, a non-hormonally-dependent process that occurs mainly through the tight junctions by passive diffusion and the transcellular pathway, occurs through sodium-dependent phosphate co-transporters present in the cell membrane (6–8). Previous studies have demonstrated that active absorption of Pi is mediated by the sodium-dependent transport, SLC34 families, and type II sodium dependent phosphate cotransporters (NaPi-II) (9, 10). NaPi-IIb-mediated Pi transport across the epithelial apical membrane is the main form of Pi uptake in the small intestine (5, 11). NaPi-IIb was first found in mice by Hilfiker in 1998 and confirmed that NaPi-IIb was mainly expressed in the brush border membranes (BBMs) of intestinal epithelial cells (12). Subsequently, researchers have cloned NaPi-IIb in human (13), rat (14), goat (15), chicken (16), and pig (5) and conducted comprehensive studies on the factors affecting its expression in the small intestine.
Epidermal growth factor (EGF), a small mitogenic polypeptide comprising 53 amino acid residues, has been established as a trophic factor for the epithelial cell homeostasis (17, 18) and nutrient transport in the small intestine (19–23). Previous studies have reported that EGF inhibited the expression of NaPi-IIb (24–27), which implied that EGF inhibited the active absorption of Pi. However, EGF is known to induce repair of oxidative damage of pig small intestinal epithelial cells stimulated by lipopolysaccharide (LPS) (18). In theory, the process of the intestinal barrier repair is accompanied by increased DNA and RNA syntheses, which leads to increased phosphorus absorption in intestinal epithelial cells since phosphorus is the main element in nucleic acid synthesis. Our previous study has confirmed that EGF can promote the expression of NaPi-IIb in LPS-induced injured porcine intestinal epithelial cells (IPEC-J2) and LPS-induced injured intestine of piglets (27). It indicated that EGF could promote the active absorption of Pi under stress condition. In this review, we mainly reviewed the effect of EGF on Pi absorption and its possible mechanism, to provide a theoretical basis for the application of EGF in animal production.
The SLC34 Family
Pi homeostasis is regulated by the coordinated interplay of the intestine, kidneys, and bones (28, 29). The intestine absorbs Pi from the diet, kidneys reabsorb Pi from the primary urine filtrate, and the bones serve as a Pi pool, where it can be deposited as hydroxyapatite or released in case Pi supply is low (30). There are two genetically distinct families of sodium-coupled co-transporters that mediate transport of Pi in mammals, namely, the SLC20 family comprises SLC20A1 (PiT-1) and SLC20A2 (PiT-2), and the SLC34 family comprises SLC34A1 (NaPi-IIa), SLC34A2 (NaPi-IIb), and SLC34A3 (NaPi-IIc) (3, 12, 31, 32) (Figure 1). PiT-1 is widely expressed in soft tissue, small intestine, and bone. PiT-2 is widely expressed in soft tissue, small intestine, bone, and kidney. NaPi-IIa and NaPi-IIc are mainly expressed in the kidney, and NaPi-IIb is mainly expressed in the small intestine (32). However, only the physiological roles of SLC34 proteins have been extensively investigated and characterized.
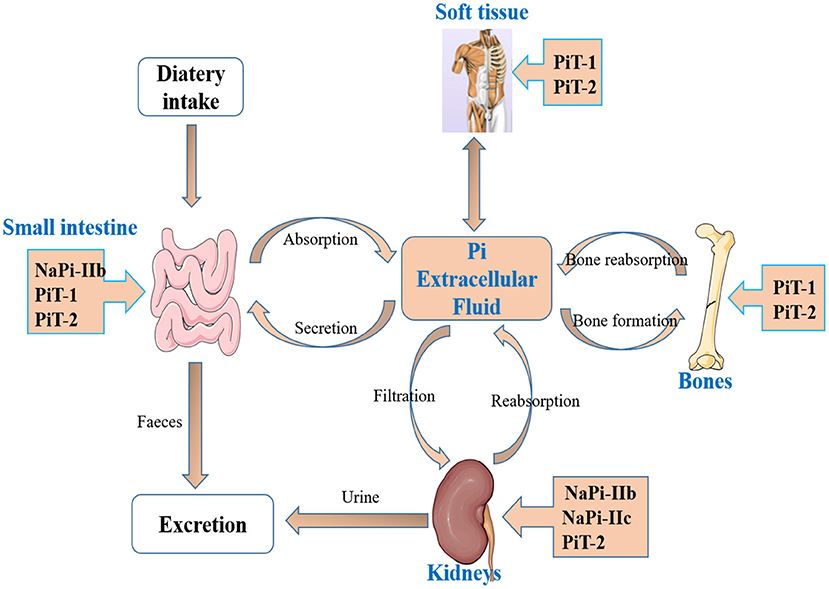
Figure 1. The main organs and transporters involved in inorganic phosphate (Pi) homeostasis. Pi homeostasis is regulated by the coordinated interplay of intestine, kidneys, and bones, and two families of sodium-coupled cotransporters, the SLC20 family (PiT-1, PiT-2) and the SLC34 family (NaPi-IIa, NaPi-IIb, and NaPi-IIc), involved in Pi absorption.
SLC34 family comprises three subtypes of phosphate transporters (Table 1). NaPi-IIa is encoded by the SLC34A1 gene, mainly expressed in the BBM of renal proximal tubular epithelial cells and is regulated by dietary Pi level, parathyroid hormone (PTH) and fibroblast growth factor (FGF23) (31–34). NaPi-IIc is encoded by the SLC34A3 gene, which is expressed exclusively in the kidney (32, 34). The expression of NaPi-IIc is related to age, with the highest level at weaning stage, and then gradually decreases with age (35). The expression of NaPi-IIc is regulated by dietary Pi level, PTH, and FGF23 too (31–34). Previous studies have shown that NaPi-IIa and NaPi-IIc are responsible for the renal reabsorption of Pi (31–35). Beck et al. (36) found that the NaPi-IIa gene knockout (NaPi-IIa−/−) mouse would lead to a reduced sodium-dependent phosphorus reabsorption by about 70% in the kidney. NaPi-IIa−/− mice lead to increased NaPi-IIc expression, which mediates about 30% phosphorus uptake (35, 37). However, the mechanism of renal Pi reabsorption regulated by NaPi-IIa and NaPi-IIc is different. NaPi-IIa is electrically charged and has a Na+:Pi ratio of 3:1, while NaPi-IIc is electrically neutral and has a Na+:Pi ratio of 3:1 (3, 31–35).
NaPi-IIb is encoded by the SLC34A2 gene, which widely expressed in lung, testicles, mammary glands, liver, salivary glands, thyroid, and small intestine, and the small intestine is the major expression site (3, 5). Like to NaPi-IIa, NAPI-IIb is also electrically charged and has a Na+:Pi ratio of 3:1 (3, 31–35). NaPi-IIb protein is thought to be the major sodium-dependent Pi transporter protein, since its ablation in mice abolishes Na+-dependent uptake of Pi (38, 39). NaPi-IIb accounts for 90% of transcellular sodium-dependent transport (38, 40, 41), which plays an important role in the intracellular Pi accumulation and Pi homeostasis. The NaPi-IIb expression in vivo is regulated by many physiological factors, including dietary Pi level (1, 42), calcitonin (43), 1.25(OH)2VD3 (44, 45), corticosterone (46), estrogen (47), B-RAF (48), EGF (24–27), and so on. Inhibition of intestinal NaPi-IIb expression would lead to an increased fecal phosphorus excretion, resulting in a waste of resources (30). Thus, investigating the regulatory factors of NaPi-IIb deeply is critically important for improving intestinal phosphorus utilization, decreasing manure phosphorus excretion, and reducing environmental pollution (43).
EGF and PI Absorption
Biological Function of EGF
Dr. Stanley Cohen first discovered EGF more than half a century ago (49). It is a small mitogenic polypeptide comprising 53 amino acid residues and three intramolecular disulfide bridges and widely exists in saliva, milk, amniotic fluid, urine, plasma, and intestinal fluid (17, 50). EGF is heat and acid stable and resistant to proteases digestion due to its special chemical structure (51), which allows its delivery to the gastrointestinal tract to exert trophic effects and makes it possible to be used in animal feed. The biological functions of EGF are mediated through binding to its receptor, EGF receptor (EGFR), a transmembrane glycoprotein, abundantly located on the apical and basolateral aspect of villus enterocytes (17, 52, 53). EGFR belongs to the transmembrane receptor tyrosine kinase of the ErbB family, with a molecular weight of 170 kDa consisting of a single polypeptide chain (54). The binding of EGF at the enterocytes surface induces dimerization of EGFR, which results in the activation of receptor tyrosine kinase (RTK) and RTK auto-phosphorylation, and subsequent activation of various signal transduction pathways, including mitogen-activated protein kinase (MAPK) (55), phosphoinositol 3 kinase (PI3K) (56), nuclear factor erythroid 2-related factor 2/ Kelch-like ECH-associated protein 1 (Nrf2/Keap1) (18), and mammalian target of rapamycin protein (mTOR) (57). Previous studies have demonstrated that EGF has many biological functions, including promoting intestinal repair (18) and nutrient absorption (23, 58, 59).
EGF Inhibits Active Transport of Pi Under Normal Conditions
Phosphorus is an essential element for the growth and development of animals. An important physiological regulator of Pi absorption is EGF, which acts through modulation of NaPi cotransporter activity (24–27, 60, 61). Early studies in rats (60) and opossum kidney cells (61) showed that EGF inhibited renal Pi uptake by modulating NaPi-IIa cotransporter protein and mRNA levels. In intestine, previous studies have confirmed that EGF also was an important physiological regulator of Pi absorption (24–27). The study in rat and human CACO2 cells from Xu et al. (24) showed that EGF significantly inhibited the expression of NaPi-IIb gene. Consistent with Xu et al. (24), our previous study also found that EGF downregulated NaPi-IIb expression in IPEC-J2 cells (26), indicating the loss of active transcellular transport of Pi in the small intestine. This suggested that, under normal conditions, EGF inhibited the active transport of Pi. However, the inhibition of NaPi-IIb expression would not affect the Pi homeostasis, because the intestinal Pi absorption is the consequence of transcellular transport plus paracellular absorption (62, 63). Passive absorption through the paracellular pathway may contribute to being an alternative transport pathway to supply enough Pi for the body when a sufficient gradient of Pi is established across the epithelium (30, 63). Additionally, the compensatory mechanism of increased renal reabsorption can also result in a normal plasma Pi (30). The phenomenon of EGF promotes cell proliferation (18, 64–67) and to some extent can also demonstrate that EGF can promote phosphorus uptake. This because, in theory, during cell proliferation, more phosphorus is needed to meet the demand of DNA and RNA syntheses, but through a paracellular pathway or activation of renal compensatory mechanisms, rather than through the active transcellular transport of Pi mediated by NaPi-IIb.
The Mechanism of EGF on NaPi-IIb Expression Regulation
EGF, as a growth hormone, plays an important role in modulating intestinal Pi absorption. Xu et al. (24) reported that EGF affected NaPi-IIb gene expression by inhibiting transcriptional activation in CACO2 cells. Further study indicated that EGF downregulated NaPi-IIb gene expression is through regulating the binding of transcription factor c-myb and NaPi-IIb gene promoter. The EGF response region was located in the promoter between −784 and −729 base pair (bp) of the promoter of human, and the downregulation of promoter function is mediated by EGF-activated protein kinase C/protein kinase A PKC/PKA and MAPK pathways (25). Previous work in our laboratory showed that the EGF response region was located in the −1,092 to −1,085 bp region (5′-TCCAGTTG-3′) in porcine intestinal epithelial cells, IPEC-J2 (26). Further studies showed that EGF downregulated the expression of NaPi-IIb in IPEC-J2 cells by activating signaling molecules such as EGFR, PKA, PKC, P38, extracellular regulated protein kinases (ERK), and c-Jun N-terminal kinase (JNK) (68). Although previous studies had proved that EGF-activated MAPK, PKC, and PKA pathways are all involved in the regulation of NaPi-IIb in intestinal epithelial cells (25, 68), how their downstream signaling molecules ultimately regulate the expression level of NaPi-IIb remains unknown.
EGF Promotes Active Transport of Pi Under Intestinal Injury Condition
The intestinal tract is not only the main part of animal nutrition digestion and absorption but also acts as a physical and immunological protective barrier against foreign antigens and pathogens (17, 69–71). The integrity of intestinal is the foundation of nutrition absorption for animals (72). However, the intestinal epithelium homeostasis of animals is usually affected by bacterial infection, endotoxin challenge, weaning stress, and oxidative stress, which can lead to intestinal damage and intestinal barrier function dysfunction (73–76). EGF has been established as a trophic factor for epithelial cell homeostasis (17, 18) and nutrient transport in the small intestine (22, 58, 66, 77, 78). Previous researches have demonstrated that EGF was able to attenuate the intestinal mucosal epithelial cells injury as well as promotes the repair of damaged mucosa epithelium (18, 79–82). In theory, during the process of injured intestine repairing, more phosphorus is needed to meet the demand of DNA and RNA syntheses. Previous studies had shown that in some disease states, such as hyperphosphatemia induced by intestinal ischemia/injury, serum Pi levels and EGF levels were increased (83, 84), which indicated that EGF might play a role in regulation of Pi homeostasis in response to intestinal injury. However, it is not clear whether EGF mediates the active transport of Pi by regulating the expression of NaPi-Iib, since the regulation of Pi is a complex network, which is achieved by the combined action of intestine, kidneys, and bones (10, 85–87). Our previous study showed that EGF could promote the expression of NaPi-IIb expression in LPS-induced IPEC-J2 cells and the jejunum and ileum of LPS-induced piglets (27). It indicated that under intestinal injury condition, EGF could release the inhibition of NaPi-IIb and regulate the active absorption of Pi mediated by NaPi-IIb to meet the body's need for phosphorus and accelerate the process of intestinal repair. However, there is still a lack of researches on EGF regulation of intestinal Pi uptake under other injury conditions, like intestinal ischemia/injury, inflammatory bowel diseases, and necrotizing enterocolitis. In addition, the mechanism of EGF on NaPi-IIb-mediated Pi uptake under intestinal injury condition remains unclear, which needs to be further research.
Conclusions
In summary, EGF is involved in regulating intestinal Pi absorption, and the role of EGF in modulating intestinal Pi absorption depends on the physiological status of the animal. Under normal conditions, EGF inhibited the active transport of Pi through activating MAPK, PKC, and PKA pathways to inhibit the expression of NaPi-IIb. While, under intestinal injury condition, EGF could promote the active absorption of Pi through upregulating the expression of NaPi-IIb (Figure 2). Further studies could focus on how EGF regulates the expression of NaPi-IIb under intestinal injury condition, thereby promoting the active transport of intestinal Pi.
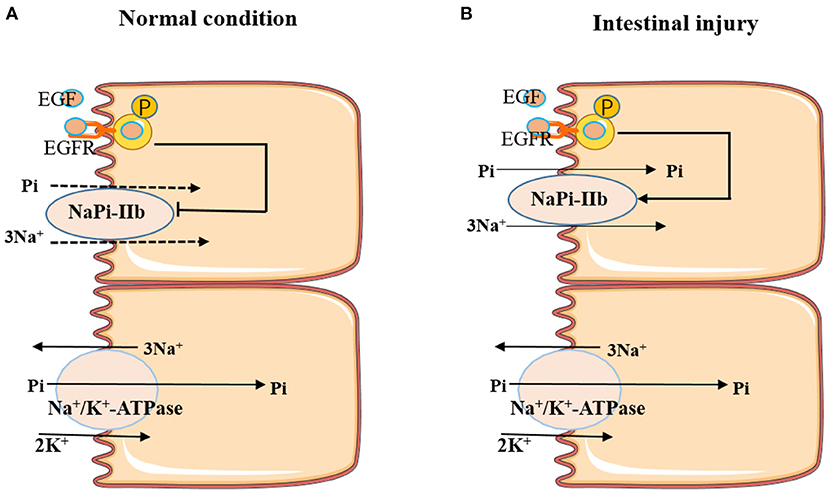
Figure 2. Effects of EGF on active Pi-transport-mediated NaPi-IIb under different conditions. (A) EGF inhibited the active transport of Pi by inhibiting the expression of NaPi-IIb under normal conditions. (B) EGF promoted the active absorption of Pi through upregulating the expression of NaPi-IIb under intestinal injury condition.
Author Contributions
XT: conceptualization and writing—original draft preparation. XT and XL: methodology and supervision. XT, HL, and XL: formal analysis and data curation. XT and HL: resources, writing—review and editing, and project administration. All authors have read and agreed to the published version of the manuscript.
Funding
This research was supported by grants from the Key Project of Science and Technology Program of Guizhou Province (No. 5411 2017 Qiankehe Pingtai Rencai); the World Top Discipline Program of Guizhou Province (No. 125 2019 Qianjiao Keyan Fa); the Natural Science Research Project of Education Department of Guizhou Province (Qianjiaohe KY Zi [2021] 294); and the Doctoral Launched Scientific Research Program of Guizhou Normal University (GZNUD [2018]26).
Conflict of Interest
The authors declare that the research was conducted in the absence of any commercial or financial relationships that could be construed as a potential conflict of interest.
Acknowledgments
We thank Prof. Kangning Xiong for his financial support and kindly revising the paper.
References
1. Fang RJ, Xiang ZF, Cao MH, He J. Different phosphate transport in the duodenum and jejunum of chicken response to dietary phosphate adaptation. Asian-Aust J Anim Sci. (2012) 25:1457–65. doi: 10.5713/ajas.2012.12187
2. Giral H, Cranston D, Lanzano L, Caldas Y, Sutherland E, Rachelson J, et al. NHE3 regulatory factor 1 (NHERF1) modulates intestinal sodium-dependent phosphate transporter (NaPi-2b) expression in apical microvilli. J Biol Chem. (2012) 287:35047–56. doi: 10.1074/jbc.M112.392415
3. Wagner CA, Hernando N, Forster IC, Biber J. The SLC34 family of sodium-dependent phosphate transporters. Pflugers Arch. (2014) 466:139–53. doi: 10.1007/s00424-013-1418-6
4. Erem S, Razzaque MS. Dietary phosphate toxicity: an emerging global health concern. Histochem Cell Biol. (2018) 150:711–9. doi: 10.1007/s00418-018-1711-8
5. Xiang ZF, Fang RJ, Hu LC, Su WQ. Molecular cloning and functional characterization of swine sodium dependent phosphate cotransporter type IIb (NaPi-IIb) gene. Mol Biol Rep. (2012) 39:10557–64. doi: 10.1007/s11033-012-1941-0
6. Schiavi SC, Tang W, Bracken C, O'Brien SP, Song W, Boulanger J, et al. Npt2b deletion attenuates hyperphosphatemia associated with CKD. J Am Soc Nephrol. (2012) 23:1691–1700. doi: 10.1681/ASN.2011121213
7. Penido MG, Alon US. Phosphate homeostasis and its role in bone health. Pediatr Nephrol. (2012) 27:2039–48. doi: 10.1007/s00467-012-2175-z
8. Fang RJ, Xiang ZF, Hu LC, Su WQ, Tang XP, Wang XR. Effects of mechanistic target of rapamycin signaling pathway on the estrogen-mediated NaPi-IIb protein expression in pig small intestinal epithelial cells. J Anim Sci. (2016) 94:303–6. doi: 10.2527/jas.2015-9866
9. Segawa H, Kaneko I, Yamanaka S, Ito M, Kuwahata M, Inoue Y, et al. Intestinal Na-Pi cotransporter adaptation to dietary Pi content in vitamin D receptor null mice. Am J Physiol Renal Physiol. (2004) 287:F39–47. doi: 10.1152/ajprenal.00375.2003
10. Hernando N, Wagner CA. Mechanisms and regulation of intestinal phosphate absorption. Compr Physiol. (2018) 8:1065–90. doi: 10.1002/cphy.c170024
11. Wong SH, Gao A, Ward S, Henley C, Lee PH. Development of a label-free assay for sodium-dependent phosphate transporter NaPi-IIb. J Biomol Screen. (2012) 17:829–34. doi: 10.1177/1087057112442961
12. Hilfiker H, Hattenhauer O, Traebert M, Forster I, Murer H, Biber J. Characterization of a murine type II sodium-phosphate cotransporter expressed in mammalian small intestine. Proc Natl Acad Sci. (1998) 95:14564–9. doi: 10.1073/pnas.95.24.14564
13. Field JA, Zhang L, Brum KA, Brooks DP, Edwards RM. Cloning and functional characterization of a sodium-dependent phosphate transporter expressed in human lungand small intestine. Biochem Biophys Res Commun. (1999) 258:578–82. doi: 10.1006/bbrc.1999.0666
14. Hashimoto M, Wang DY, Kamo T, Zhu Y, Tsujiuchi T, Konishi Y, et al. Isolation and localization of type IIb Na/Pi cotransporter in the developing rat lung. Am J Pathol. (2000) 157:21–7. doi: 10.1016/S0002-9440(10)64512-9
15. Huber K, Walter C, Schröder B, Breves G. Phosphate transport in the duodenum and jejunum of goats and its adaptation by dietary phosphate and calcium. Am J Physiol Regul Integr Comp Physiol. (2002) 283:R296–302. doi: 10.1152/ajpregu.00760.2001
16. Yan F, Angel R, Ashwell CM. Characterization of the chicken small intestine type IIb sodium phosphate cotransporter. Poult Sci. (2007) 86:67–76. doi: 10.1093/ps/86.1.67
17. Tang XP, Liu H, Yang SF, Li ZH, Zhong JF, Fang RJ. Epidermal growth factor and intestinal barrier function. Mediators Inflamm. (2016) 2016:1927348. doi: 10.1155/2016/1927348
18. Tang XP, Liu B, Wang XR, Yu QF, Fang RJ. Epidermal growth factor, through alleviating oxidative stress, protect IPEC-J2 cells from lipopolysaccharides-induced apoptosis. Int J Mol Sci. (2018) 19:848. doi: 10.3390/ijms19030848
19. Huang Q, Li N, Zhu WM, Li QR, Li JS. Glutamine transporter ASCT2 was down-regulated in ischemic injured human intestinal epithelial cells and reversed by epidermal growth factor. J Parenter Enteral Nutr. (2007) 31:86–93. doi: 10.1177/014860710703100286
20. Trapani V, Arduini D, Luongo F, Wolf FI. EGF stimulates Mg2+ influx in mammary epithelial cells. Biochem Biophys Res Commun. (2014) 454:572–5. doi: 10.1016/j.bbrc.2014.10.125
21. Wang L, Zhu F, Yang H, Zhong J, Li Y, Ding X, et al. Effects of dietary supplementation with epidermal growth factor on nutrient digestibility, intestinal development and expression of nutrient transporters in early-weaned piglets. J Anim Physiol Anim Nutr. (2019) 103:618–25. doi: 10.1111/jpn.13059
22. Tang XP, Fang RJ, Pan GC, Xiong KN. Acute effect of epidermal growth factor on phosphate diffusion across intestinal mucosa of hens using the ussing chamber system. Pakistan J Zool. (2019) 51:2209–16. doi: 10.17582/journal.pjz/2019.51.6.2209.2216
23. Wang LX, Zhu F, Li JZ, Li YL, Ding XQ, Xiong X, et al. Epidermal growth factor promotes intestinal secretory cell differentiation in weaning piglets via Wnt/β-catenin signalling. Animal. (2020) 14:790–8. doi: 10.1017/S1751731119002581
24. Xu H, Collins JF, BAI L, Kiela PR, Ghishan FK. Regulation of the human sodium-phosphate cotransporter NaPi-IIb gene promoter by epidermal growth factor. Am J Physiol Cell Physiol. (2001) 280:C628–36. doi: 10.1152/ajpcell.2001.280.3.C628
25. Xu H, Inouye M, Hines ER, Collins JF, Ghishan FK. Transcriptional regulation of the human NaPi-IIb cotransporter by EGF in Caco-2 cells involves c-myb. Am J Physiol Cell Physiol. (2003) 28:C1262–71. doi: 10.1152/ajpcell.00456.2002
26. Xing TJ, Tan X, Yu Q, Yang T, Fang R. Identifying the location of epidermal growth factor-responsive element involved in the regulation of type IIb sodium-phosphate cotransporter expression in porcine intestinal epithelial cells. J Anim Physiol Anim Nutr. (2017) 101:1249–58. doi: 10.1111/jpn.12645
27. Tang XP, Xu R, Li CL, Peng P, Yu QF, Fang RJ. Effects of epidermal growth factor on intestinal type IIb sodium-phosphate cotransporter expression of weaned piglets challenged by lipopolysaccharide. Chin J Anim Nutr. (2018) 30:4020−7. doi: 10.3969/j.issn.1006-267x.2018.10.027
28. Blau JE, Collins MT. The PTH-vitamin D-FGF23 axis. Rev Endocr Metab Disord. (2015) 16:165–74. doi: 10.1007/s11154-015-9318-z
29. Marks J. The role of SLC34A2 in intestinal phosphate absorption and phosphate homeostasis. Pflugers Arch. (2019) 471:165–73. doi: 10.1007/s00424-018-2221-1
30. Knöpfel T, Pastor-Arroyo EM, Schnitzbauer U, Kratschmar DV, Odermatt A, Pellegrini G, et al. The intestinal phosphate transporter NaPi-IIb (Slc34a2) is required to protect bone during dietary phosphate restriction. Sci Rep. (2017) 7:11018. doi: 10.1038/s41598-017-10390-2
31. Biber J, Hernando N, Forster I. Phosphate transporters and their function. Annu Rev Physiol. (2013) 75:535–50. doi: 10.1146/annurev-physiol-030212-183748
32. Forster I, Hernando N, Biber J, Murer H. Phosphate transporters of the SLC20 and SLC34 families. Mol Aspects Med. (2013) 34:386–95. doi: 10.1016/j.mam.2012.07.007
33. Biber J, Hernando N, Forste I, Murer H. Regulation of phosphate transport in proximal tubules. Pflugers Archiv. (2009) 458:39–52. doi: 10.1007/s00424-008-0580-8
34. Forster I, Hernando N, Sorribas V, Werner A. Phosphate transporters in renal, gastrointestinal, and other tissues. Adv Chronic Kidney Dis. (2011) 18:63–76. doi: 10.1053/j.ackd.2011.01.006
35. Marks J, Debnam ES, Unwin RJ. Phosphate homeostasis and the renal-gastrointestinal axis. Am J Physiol Renal Physiol. (2010) 299:F285–96. doi: 10.1152/ajprenal.00508.2009
36. Beck L, Karaplis AC, Amizuka N, Hewson AS, Ozawa H, Tenenhouse HS. Targeted inactivation of Npt2 in mice leads to severe renal phosphate wasting, hypercalcuria, and skeletal abnormalities. Proc Natl Acad Sci USA. (1998) 95:5372–7. doi: 10.1073/pnas.95.9.5372
37. Tenenhouse HS, Martel J, Gauthier C, Segawa H, Miyamoto K. Differential effects of Npt2a gene ablation and X-linked Hyp mutation on renal expression of Npt2c. Am J Physiol Renal Physiol. (2003) 285:F1271–8. doi: 10.1152/ajprenal.00252.2003
38. Sabbagh Y, O'Brien SP, Song W, Boulanger JH, Stockmann A, Arbeeny C, et al. Intestinal npt2b plays a major role in phosphate absorption and homeostasis. J Am Soc Nephrol. (2009) 20:2348–58. doi: 10.1681/ASN.2009050559
39. Hernando N, Myakala K, Simona F, Knöpfel T, Thomas L, Murer H, et al. Intestinal depletion of NaPi-IIb/Slc34a2 in mice: renal and hormonal adaptation. J Bone Miner Res. (2015) 30:1925–37. doi: 10.1002/jbmr.2523
40. Murer H, Forster I, Biber J. The sodium phosphate cotransporter family SLC34. Pflugers Arch. (2004) 447:763–7. doi: 10.1007/s00424-003-1072-5
41. Forster IC, Hernando N, Biber J, Murer H. Phosphate transport kinetics and structure-function relationships of SLC34 and SLC20 Proteins. Curr Top Membr. (2012) 70:313–56. doi: 10.1016/B978-0-12-394316-3.00010-7
42. Li P, Wang RM, Jiao HC, Wang XJ, Zhao JP, Lin H. Effects of dietary phosphorus level on the expression of calcium and phosphorus transporters in laying hens. Front Physiol. (2018) 9:627. doi: 10.3389/fphys.2018.00627
43. Tang XP, Su WQ, Fang RJ. Effects of calcitonin on porcine intestinal epithelial cells proliferation, phosphorus absorption, and NaPi-IIb expression. Pakistan J Zool. (2019) 51:2167–74. doi: 10.17582/journal.pjz/2019.51.6.2167.2174
44. Capuano P, Radanovic T, Wagner CA, Bacic D, Kato S, Uchiyama Y, et al. Intestinal and renal adaptation to a low-Pi diet of type II NaPi cotransporters in vitamin D receptor- and 1alphaOHase-deficient mice. Am J Physiol Cell Physiol. (2005) 288:C429–34. doi: 10.1152/ajpcell.00331.2004
45. Liao XD, Suo HQ, Lu L, Hu YX, Zhang LY, Luo XG. Effects of sodium, 1, 25-dihydroxyvitamin D3 and parathyroid hormone fragment on inorganic P absorption and Type IIb sodium-phosphate cotransporter expression in ligated duodenal loops of broilers. Poult Sci. (2017) 96:2344–50. doi: 10.3382/ps/pex033
46. Arima K, Hines ER, Kiela PR, Drees JB, Collins JF, Ghishan FK. Glucocorticoid regulation and glycosylation of mouse intestinal type IIb Na-Pi cotransporter during ontogeny. Am J Physiol Gastrointest Liver Physiol. (2002) 283:G426–34. doi: 10.1152/ajpgi.00319.2001
47. Xu H, Uno KJ, Inouye M, Xu LP. Regulation of intestinal NaPi-IIb cotransporter gene expression by estrogen. Am J Physiol Gastroimest Liver Physiol. (2003) 285:G1317–24. doi: 10.1152/ajpgi.00172.2003
48. Pakladok T, Hosseinzadeh Z, Lebedeva A, Alesutan I, Lang F. Upregulation of the Na+-Coupled phosphate cotransporters NaPi-IIa and NaPi-IIb by B-RAF. J Membrance Biol. (2014) 247:137–45. doi: 10.1007/s00232-013-9616-x
49. Cohen S. Isolation of a mouse submaxillary gland protein accelerating incisor eruption and eyelid opening in the new-born animal. J Biol Chem. (1962) 237:1555–62. doi: 10.1016/S0021-9258(19)83739-0
50. Zeng HF, Harris RC. Epidermal growth factor, from gene organization to bedside. Semin. Cell Dev Biol. (2014) 28:2–11. doi: 10.1016/j.semcdb.2014.01.011
51. Clark JA, Gan H, Samocha AJ, Fox AC, Buchman TG, Coopersmith CM. Enterocyte-specific epidermal growth factor prevents barrier dysfunction and improves mortality in murine peritonitis. Am J Physiol Gastrointest Liver Physiol. (2009) 297:G471–9. doi: 10.1152/ajpgi.00012.2009
52. Avissar N, Wang H, Miller J, Iannoli P, Sax HC. Epidermal growth factor receptor is increased in rabbit intestinal brush border membrane after small bowel resection. Dig Dis Sci. (2000) 45:1145–52. doi: 10.1023/A:1005597918552
53. Li D, Shatos MA, Hodges RR, Dartt DA. Role of PKCα activation of Src, PI-3K/AKT, and ERK in EGF-stimulated proliferation of rat and human conjunctival goblet cells. Invest Ophthalmol Vis Sci. (2013) 54:5661–74. doi: 10.1167/iovs.13-12473
54. Wee P, Shi H, Jiang J, Wang Y, Wang Z. EGF stimulates the activation of EGF receptors and the selective activation of major signaling pathways during mitosis. Cell Signal. (2015) 27:638–51. doi: 10.1016/j.cellsig.2014.11.030
55. Wijayagunawardane MP, Hambruch N, Haeger JD, Pfarrer C. Effect of epidermal growth factor (EGF) on the phosphorylation of mitogen-activated protein kinase (MAPK) in the bovine oviduct in vitro: alteration by heat stress. J Reprod Dev. (2015) 61:383–9. doi: 10.1262/jrd.2014-061
56. Hu ZX, Chen CL, Yang JS, Zhou ZL, Song ZM, Wang ZY. PI3K-mediated glioprotective effect of epidermal growth factor under oxidative stress conditions. Int J Ophthalmol. (2014) 7:413–20. doi: 10.3980/j.issn.2222-3959.2014.03.05
57. Wang L, Zhu F, Yang H, Li J, Li Y, Ding X, et al. Epidermal growth factor improves intestinal morphology by stimulating proliferation and differentiation of enterocytes and mTOR signaling pathway in weaning piglets. Sci China Life Sci. (2020) 63:259–68. doi: 10.1007/s11427-018-9519-6
58. Tang XP, Xiong KN. Effects of epidermal growth factor on glutamine and glucose absorption by IPEC-J2 cells challenged by lipopolysaccharide using the Ussing chamber system. Pakistan J Zool. (2021) 53:417–22. doi: 10.17582/journal.pjz/20200117080156
59. Wang CW, Chang WL, Huang IC, Chou FC, Chan FN, Su SC, et al. An essential role of cAMP response element-binding protein in epidermal growth factor-mediated induction of sodium/glucose cotransporter1 gene expression and intestinal glucose uptake. Int J Biochem Cell Biol. (2015) 64:239–51. doi: 10.1016/j.biocel.2015.04.006
60. Arar M, Zajicek HK, Elshihabi I, Levi M. Epidermal growth factor inhibits Na-Pi cotransport in weaned and suckling rats. Am J Physiol. (1999) 276:F72–8. doi: 10.1152/ajprenal.1999.276.1.F72
61. Arar M, Baum M, Biber J, Murer H, Levi M. Epidermal growth factor inhibits Na-Pi cotransport and mRNA in OK cells. Am J Physiol. (1995) 268:F309–14. doi: 10.1152/ajprenal.1995.268.2.F309
62. Marks J, Lee GJ, Nadaraja SP, Debnam ES, Unwin RJ. Experimental and regional variations in Na-dependent and Na-independent phosphate transport along the rat small intestine and colon. Physiol Rep. (2015) 3:e12281. doi: 10.14814/phy2.12281
63. Candeal E, Caldas YA, Guillén N, Levi M, Sorribas V. Intestinal phosphate absorption is mediated by multiple transport systems in rats. Am J Physiol Gastrointest Liver Physiol. (2017) 312:G355–66. doi: 10.1152/ajpgi.00244.2016
64. Kang P, Toms DY, Cheung Q, Gong J, De LK, Li J. Epidermal growth factor-expressing Lactococcus lactis enhances intestinal development of earlyweaned pigs. J Nutr. (2010) 140:806–11. doi: 10.3945/jn.109.114173
65. Jeong W, Kim J, Bazer FW, Song G. Epidermal growth factor stimulates proliferation and migration of porcine trophectoderm cells through protooncogenic protein kinase 1 and extracellular-signal-regulated kinases 1/2 mitogen-activated protein kinase signal transduction cascades during early pregnancy. Mol Cell Endocrinol. (2013) 381:302–11. doi: 10.1016/j.mce.2013.08.024
66. Bedford A, Chen T, Huynh E, Zhu C, Medeiros S, Wey D, et al. Epidermal growth factor containing culture supernatant enhances intestine development of early-weaned pigs in vivo: potential mechanisms. J Biotechnol. (2015) 196–197:9–19. doi: 10.1016/j.jbiotec.2015.01.007
67. Alexander PB, Yuan L, Yang P, Sun T, Chen R, Xiang H, et al. EGF promotes mammalian cell growth by suppressing cellular senescence. Cell Res. (2015) 25:135–8. doi: 10.1038/cr.2014.141
68. Xing TJ, Tang XP, Cao MH, Fang RJ. Identifying cell signaling pathway that mediates epidermal growth factor regulating sodium dependent phosphate cotransporters Type IIb expression in porcine intestinal epithelial cells. Chin J Anim Nutri. (2017) 29:1988–95. doi: 10.3969/j.issn.1006-267x.2017.06.020
69. Arrieta MC, Bistritz L, Meddings JB. Alterations in intestinal permeability. Gut. (2006) 55:1512–20. doi: 10.1136/gut.2005.085373
70. Yang HS, Xiong X, Wang XC, Tan BE, Li TJ, Yin YL. Effects of weaning on intestinal upper villus epithelial cells of piglets. PLoS ONE. (2016) 11:e0150216. doi: 10.1371/journal.pone.0150216
71. Peng P, Deng D, Chen SJ, Li CL, Luo J, Romeo A, et al. The effects of dietary porous zinc oxide supplementation on growth performance, inflammatory cytokines and tight junction's gene expression in early-weaned piglets. J Nutr Sci Vitaminol. (2020) 66:311–8. doi: 10.3177/jnsv.66.311
72. García-Hernández V, Flores-Maldonado C, Rincon-Heredia R, Verdejo-Torres O, Bonilla-Delgado J, Meneses-Morales I, et al. EGF Regulates Claudin-2 and-4 expression through Src and STAT3 in MDCK cells. J Cell Physiol. (2015) 230:105–15. doi: 10.1002/jcp.24687
73. Liu Y, Chen F, Odle J, Lin X, Jacobi SK, Zhu H, et al. Fish oil enhances intestinal integrity and inhibits TLR4 and NOD2 signaling pathways in weaned pigs after LPS challenge. J Nutr. (2012) 142:2017–24. doi: 10.3945/jn.112.164947
74. Campbell JM, Crenshaw JD, Polo J. The biological stress of early weaned piglets. Anim Sci Biotechnol. (2013) 4:19. doi: 10.1186/2049-1891-4-19
75. Yin J, Wu MM, Xiao H, Ren WK, Duan JL, Yang G, et al. Development of an antioxidant system after early weaning in piglets. J Anim Sci. (2014) 92:612–9. doi: 10.2527/jas.2013-6986
76. Zhu HL, Wang HB, Wang SH, Tu ZX, Zhang L, Wang XY, et al. Flaxseed oil attenuates intestinal damage and inflammation by regulating necroptosis and TLR4/NOD signaling pathways following lipopolysaccharide challenge in a piglet model. Mol Nutr Food Res. (2018) 62:e1700814. doi: 10.1002/mnfr.201700814
77. Cellini C, Xu J, Buchmiller-Crair T. Effect of epidermal growth factor on small intestinal sodium/glucose cotransporter-1 expression in a rabbit model of intrauterine growth retardation. J Pediatr Surg. (2005) 40:1892–7. doi: 10.1016/j.jpedsurg.2005.08.049
78. Xu S, Wang D, Zheng P, Lin Y, Fang ZF, Che LQ, et al. Oral administration of Lactococcus lactis-expressed recombinant porcine epidermal growth factor (rpEGF) stimulates the development and promotes the health of small intestines in early-weaned piglets. J Appl Microbiol. (2015) 119:225–35. doi: 10.1111/jam.12833
79. Clark JA, Lane RH, Maclennan NK, Holubec H, Dvorakova K, Halpern MD, et al. Epidermal growth factor reduces intestinal apoptosis in an experimental model of necrotizing enterocolitis. Am J Physiol Gastrointest Liver Physiol. (2005) 288:G755–62. doi: 10.1152/ajpgi.00172.2004
80. Maynard AA, Dvorak K, Khailova L, Dobrenen H, Arganbright KM, Halpern MD, et al. Epidermal growth factor reduces autophagy in intestinal epithelium and in the rat model of necrotizing enterocolitis. Am J Physiol Gastrointest Liver Physiol. (2010) 299:G614–22. doi: 10.1152/ajpgi.00076.2010
81. Arda-Pirincci P, Bolkent S. The role of epidermal growth factor in prevention of oxidative injury and apoptosis induced by intestinal ischemia/reperfusion in rats. Acta Histochem. (2014)116:167–75. doi: 10.1016/j.acthis.2013.07.005
82. Tang X, Liu X, Fang R. Role of epidermal growth factor in regulation of intestinal health of weaned piglets. Chin J Anim Nutri. (2021) 33:614–21. doi: 10.3969/j.issn.1006-267x.2021.02.002
83. Feretis CB, Koborozos BA, Vyssoulis GP, Manouras AJ, Apostolidis NS, Golematis BC. Serum phosphate levels in acute bowel ischemia. an aid to early diagnosis. Am Surg. (1985) 51:242–4.
84. Thompson JS. Epidermal growth factor and the short bowel syndrome. JPEN J Parenter Enteral Nutr. (1999) 23:S113–6. doi: 10.1177/014860719902300528
85. Christov M, Jüppner H. Phosphate homeostasis disorders. Best Pract Res Clin Endocrinol Metab. (2018) 32:685–706. doi: 10.1016/j.beem.2018.06.004
86. Lacerda-Abreu MA, Russo-Abrahão T, Meyer-Fernandes JR. The roles of sodium-independent inorganic phosphate transporters in inorganic phosphate homeostasis and in cancer and other diseases. Int J Mol Sci. (2020) 21:9298. doi: 10.3390/ijms21239298
Keywords: epidermal growth factor, NaPi-IIb, phosphate uptake, phosphorus, small intestine
Citation: Tang X, Liu X and Liu H (2021) Mechanisms of Epidermal Growth Factor Effect on Animal Intestinal Phosphate Absorption: A Review. Front. Vet. Sci. 8:670140. doi: 10.3389/fvets.2021.670140
Received: 20 February 2021; Accepted: 03 May 2021;
Published: 14 June 2021.
Edited by:
Xiangfeng Kong, Chinese Academy of Sciences, ChinaReviewed by:
Qingwei Meng, Northeast Agricultural University, ChinaKolapo Ajuwon, Purdue University, United States
Copyright © 2021 Tang, Liu and Liu. This is an open-access article distributed under the terms of the Creative Commons Attribution License (CC BY). The use, distribution or reproduction in other forums is permitted, provided the original author(s) and the copyright owner(s) are credited and that the original publication in this journal is cited, in accordance with accepted academic practice. No use, distribution or reproduction is permitted which does not comply with these terms.
*Correspondence: Xiaopeng Tang, dGFuZ3hpYW9wZW5nMTEwJiN4MDAwNDA7MTI2LmNvbQ==; Hu Liu, bGl1aDIwMTgmI3gwMDA0MDtsenUuZWR1LmNu