- 1Department of Veterinary and Biomedical Sciences, College of Veterinary Medicine, University of Minnesota, Saint Paul, MN, United States
- 2Department of Veterinary Population Medicine, College of Veterinary Medicine, University of Minnesota, Saint Paul, MN, United States
- 3Veterinary Diagnostic Laboratory, College of Veterinary Medicine, University of Minnesota, Saint Paul, MN, United States
Infection with Campylobacter species is one of the leading causes of bacterial diarrhea in humans in the US. Chickens, which become colonized on the farm, are important reservoirs of this bacterium. Campylobacter can establish itself in the broiler house via a variety of sources, can survive in the litter of the house, and possibly persist over successive flock cycles. However, the role of the broiler litter microbiome on Campylobacter persistence is not clear. A matched case-control study was conducted to determine whether the broiler litter microbiome composition was associated with Campylobacter isolation within the broiler house. Flocks were classified as cases when either Campylobacter jejuni or Campylobacter coli was isolated in boot sock samples, or as controls otherwise. Case and control flocks were matched at the broiler house level. Composite broiler litter samples were collected and used for DNA extraction and 16S rRNA gene V4 region sequencing. Reads were processed using the DADA2 pipeline to obtain a table of amplicon sequence variants. Alpha diversity and differential bacterial relative abundance were used as predictors of Campylobacter isolation status in conditional logistic regression models adjusting for flock age and sampling season. Beta diversity distances were used as regressors in stratified PERMANOVA with Campylobacter isolation status as predictor, and broiler house as stratum. When Campylobacter was isolated in boot socks, broiler litter microbiome richness and evenness were lower and higher, respectively, without reaching statistical significance. Campylobacter isolation status significantly explained a small proportion of the beta diversity (genus-level Aitchison dissimilarity distance). Clostridium and Anaerostipes were positively associated with Campylobacter isolation status, whereas Bifidobacterium, Anaerosporobacter, and Stenotrophomonas were negatively associated. Our results suggest the presence of bacterial interactions between Campylobacter and the broiler litter microbiome. The negative association of Campylobacter with Bifidobacterium, Anaerosporobacter, and Stenotrophomonas in litter could be potentially exploited as a pre-harvest control strategy.
Introduction
Campylobacter are Gram-negative non-spore forming bacteria with ~31 species and 13 subspecies (1). Several species of Campylobacter, primarily Campylobacter jejuni and Campylobacter coli, are important human pathogens, causing an estimated 1.3 million cases of diarrhea in the US every year (2). The incidence of Campylobacter infections in humans seems to be increasing in the US (3). Furthermore, irritable bowel syndrome, reactive arthritis, and Guillain-Barré syndrome have been associated with previous episodes of campylobacteriosis (4). Sources of Campylobacter for humans include chicken meat, beef, raw milk, and water (5, 6).
Chickens become colonized with Campylobacter on the farm. Although many broiler chicken farms can be negative for Campylobacter, once the bacterium is established in the broiler house, the prevalence of colonization in chickens can reach 100% (7, 8). Campylobacter predominantly colonizes the chicken cecum, in which it can multiply up to 109 CFU/g of cecal contents (9) without inducing clinical signs. In addition, Campylobacter colonization has been shown to increase the translocation of Escherichia coli to extraintestinal sites in chickens (10, 11), potentially leading to systemic disease.
Within the broiler house, bedding material, often referred to as litter, is comprised of a substrate (e.g., wood shavings) and chicken excreta (12). Broiler litter is frequently reused across subsequent flocks after windrowing (akin to composting). Windrowing is performed to control poultry pathogens present in the broiler litter (13). However, there is evidence that Campylobacter can persist in reused broiler litter (14–16). Therefore, survival of Campylobacter in the broiler litter may play an important role in the ecology and colonization of this bacterium in broiler chickens. Campylobacter can also enter the broiler house via rodents, flies, darkling beetles, wild birds, cockroaches, or water (17–22).
Broiler litter harbors a microbiome comprised of a collection of bacterial, viral, fungal, and protozoan microorganisms. The bacterial component of the broiler litter microbiome has been shown to commonly include genera associated with the gut and/or the environment. For instance, Lactobacillus, Escherichia, Bacteroides, and Brevibacterium have been documented in broiler litter and are common members of the gut microbiome, while Salinicoccus, Arthrobacter, and Brachybacterium are present in both, broiler litter and soil. Furthermore, bacteria such as Clostridium and Corynebacterium, members of the gut and soil microbiomes, have also been detected in broiler litter (12, 23–27).
The interactions between Campylobacter and the bacterial members of the broiler litter microbiome remain unexplored. The aim of this study was to examine the relationship between broiler litter microbiome composition and the detection of Campylobacter in the litter of commercial broiler houses. Identifying microorganisms that are either positively or negatively associated with Campylobacter detection could lead to potential interventions to prevent or hinder the successful colonization of the broiler house with Campylobacter.
Materials and Methods
Study Design
A matched case-control study was carried out to evaluate the study objective. The sampling frame consisted of broiler houses enrolled from six large poultry production companies in the US. Enrolled broiler houses were sampled once during the production cycle, between 3 weeks of age and the end of the grow-out period. For this study, samples that were collected from flocks reared consecutively in the same house were used. Two types of samples were obtained: boot socks and composite broiler litter samples. Two boot socks per sampling were collected following previously published procedures (28) and were used for Campylobacter isolation. One composite broiler litter sample representative of the house litter was collected per sampling (28) and was used for the microbiome analysis. Briefly, the composite litter samples were collected while walking the house. The composite litter sample included litter from different areas of the house, such as near water lines, feed lines, and along the walls. The primary substrate of the litter was wood shavings; no information regarding litter management was obtained from the participating companies. Flock age and season at the time of sampling were recorded and used in data analysis.
Flocks were classified as cases when either C. jejuni or C. coli was isolated in boot sock samples or as controls if no Campylobacter was isolated. Fifty-one case flocks were individually matched to 139 control flocks by broiler house in a variable case-control ratio (Table 1) for a total of 190 flocks. The case-control assignment was employed to categorize each flock as colonized by Campylobacter near the age of slaughter, which has been shown to be correlated with chicken carcass contamination with this bacterium in the processing plant (28). For simplicity, the case-control assignment will be referred to as Campylobacter isolation status of the flock.
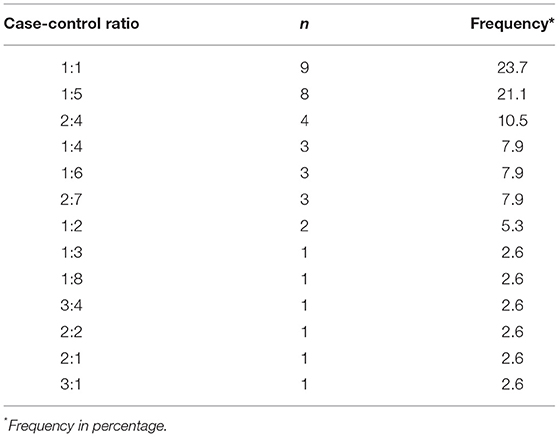
Table 1. Distribution of the ratios used to individually match flocks with isolation of Campylobacter (cases) to flocks with no Campylobacter isolation (controls).
Isolation of Campylobacter
Boot sock samples were collected and transported overnight to the laboratory at 4°C. Upon arrival, 20 mL Bolton broth (Oxoid, UK) was added to each boot sock and then homogenized. Supernatants were recovered and placed in polyurethane tubes with loose caps (Corning Science, Mexico). Samples were incubated at 42°C for 24 h under microaerophilic conditions (5% O2, 10% CO2, and 85% N2). Afterwards, samples were streaked onto CampyCefex plates (Becton, Dickinson, and Company, USA) supplemented with Preston (Oxoid, UK) and incubated at 42°C for 48 h under microaerophilic conditions. Campylobacter colonies were presumptively identified through the observation of typical morphological characteristics (29). Five colonies per plate were selected and streaked for a second passage onto CampyCefex plates supplemented with Preston as performed in the initial step. After a further passage on 5% sheep blood agar (Becton, Dickinson, and Company, USA) to ensure purity, isolates were speciated through PCR.
Molecular Identification of Campylobacter Isolates
DNA from isolates was extracted using proteinase K treatment (0.1 mg/mL) and incubation at 55°C and 80°C for 10 min each in a water bath. Afterwards, 80 μL of DNAse-free water were added to the samples, which were centrifuged for 2 min at 4,500 g. Supernatants (with genomic DNA) were harvested and stored at 4°C until use.
Genomic DNA was used for a multiplex PCR employing two primers specific to the Campylobacter genus and three primers for discrimination between C. jejuni and C. coli (30–32). Two microliters of gDNA from presumptive Campylobacter isolates were added to 23 μL of PCR reaction mix, which contained 10.25 μL of nuclease-free water, 5 μL of 5X Green GoTaq Flexi buffer (Promega, USA), 3 μL of 25 mM MgCl2, 1 μL of 10 mM dNTPs, 0.5 μL of 10 μM lpxA C. coli forward primer (5′-AGACAAATAAGAGAGAATCAG-3′), 0.5 μL of 10 μM lpxA C. jejuni forward primer (5′-ACAACTTGGTGACGATGTTGTA-3′), 0.5 μL of 30 μM lpxARKK2m reverse primer (5′-CAATCATGDGCDATATGASAATAHGCCAT-3′), 1 μL of 10 μM C412 forward primer (5′-GGATGACACTTTTCGGAGC-3′), 1 μL of 10 μM C1228 reverse primer (5′-CATTGTAGCACGTGTGTC-3′), and 0.25 μL of 5 U/μL GoTaq DNA polymerase (Promega, USA). The following PCR program was used: 5 min at 95°C initially, 30 cycles comprising 30 s of denaturation at 94°C, 30 s of annealing at 50°C, and 1 min of extension at 72°C. The last cycle was followed by 7 min of final extension at 72°C. Amplicons were visualized using UV light after 1.5% agarose gel electrophoresis, with ethidium bromide as DNA stain and 100 bp DNA ladder as standard. The size of the expected products was 816 bp for Campylobacter genus, 331 bp for C. jejuni and 391 bp for C. coli.
DNA Extraction and 16S rRNA Gene Sequencing
Composite broiler litter samples were prepared by adding PBS (1:1 v/w) and then vortexed for 1 min. Particles present in the solution were allowed to sediment for 5 min, and the supernatant was collected. Supernatant was used for DNA extraction with the PowerSoil kit (Qiagen, Germany) following manufacturer's instructions. The V4 region from 16S rRNA gene was amplified and dual indexed during library preparation (33). Sequencing of the amplicons was performed using MiSeq 2x300 bp platform at the University of Minnesota Genomic Center (UMGC).
Bioinformatics Analysis
All bioinformatics analyses were performed using R version 4.0.3 (34). The quality profiles of the reads for each sample were visually inspected separately for forward and reverse reads. Primers and adapters were trimmed from all reads (Trim Galore version 0.6.4_dev for adapter removal; URL: http://www.bioinformatics.babraham.ac.uk/projects/trim_galore/). The last 30 bases of forward reads and the last 50 bases of reverse reads were also trimmed. Denoising of reads, merging of paired reads, and removal of chimera were performed using the DADA2 package version 1.18.0 (35). The DADA2-formated training fasta file derived from the Silva Project's version 138 release (36) was used to assign taxonomy to the amplicon sequence variants (ASVs) obtained from the previous steps via a naïve Bayesian classifier (37). Furthermore, exact matching was performed to assign species to ASVs (38). Contaminant ASVs were inferred using the frequency approach of the decontam package version 1.10.0 (39) and pruned from the data set. Amplicon sequence variants from Eukarya, chloroplasts, and mitochondria were removed, as were those present in <1% of the samples. Chao1, Abundance-based Coverage Estimator of species richness (ACE), Fisher, Shannon, and Inverse Simpson alpha diversity indices were computed and used in conditional logistic regression models. Aitchison, Bray-Curtis, and Jaccard beta diversity dissimilarity distances were computed at the ASV-level and used in NMDS and PCoA plots. Additionally, beta diversity dissimilarity distances, calculated at the ASV and genus-level, were used in stratified PERMANOVA (40).
Statistical Analyses
The association between Campylobacter isolation status and alpha diversity was assessed using conditional logistic regression in Stata 16.1 (41). Campylobacter isolation status was regressed on each of the alpha diversity indices, adjusting for flock age and sampling season, with broiler house as stratum, and robust standard errors. Multiple imputation to handle missing data on the variable flock age was performed. The association between Campylobacter isolation status and beta diversity was evaluated using PERMANOVA stratified by broiler house, with Campylobacter isolation status as predictor, and each beta diversity dissimilarity distance as regressor.
Differentially abundant bacteria by Campylobacter isolation status were detected with and without aggregation of read counts at the genus level. Read counts were transformed into centered log ratio (42). The feature (genus or ASV, respectively) with the highest centered log ratio was dropped from the data set to avoid collinearity (43). The number of features was reduced using LASSO conditional logistic regression from clogitL1 package version 1.5 in R (44). The regularization parameter (λ) was selected via leave-one-out cross-validation. LASSO selected features were used as predictors in a conditional logistic regression model with Campylobacter isolation status as regressor. Adjustment for potential confounders and handling of missing data were performed as indicated for the evaluation of alpha diversity. The significance level was set a priori at 0.05.
Results
The total number of ASVs detected in the composite broiler litter samples was 2,246. Seventy-three percent of ASVs (1,629) were assigned to 395 genera by the naïve Bayesian classifier. The top five most abundant bacterial genera were Lactobacillus (median relative abundance: 11.8%), Corynebacterium (9.8%), Brachybacterium (9.6%), Brevibacterium (6.9%), and Salinicoccus (5.2%). The 20 most abundant bacterial genera did not change by Campylobacter isolation status of the flock; however, their ranks differed (Table 2 and Figure 1).
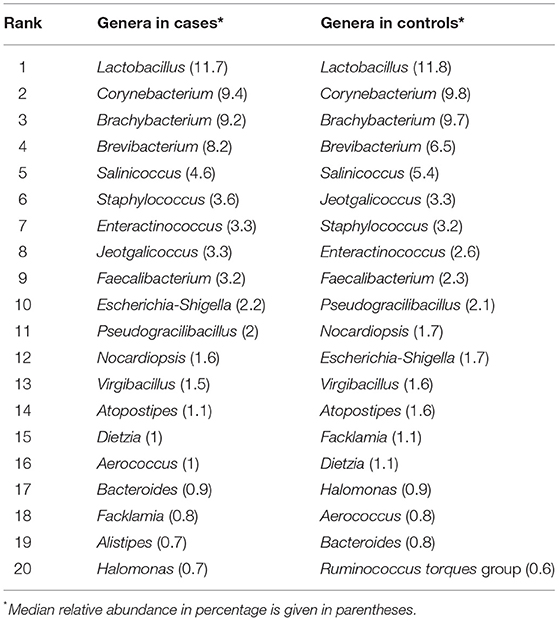
Table 2. Top 20 most abundant bacterial genera in composite broiler litter segregated according to Campylobacter isolation status of flocks.
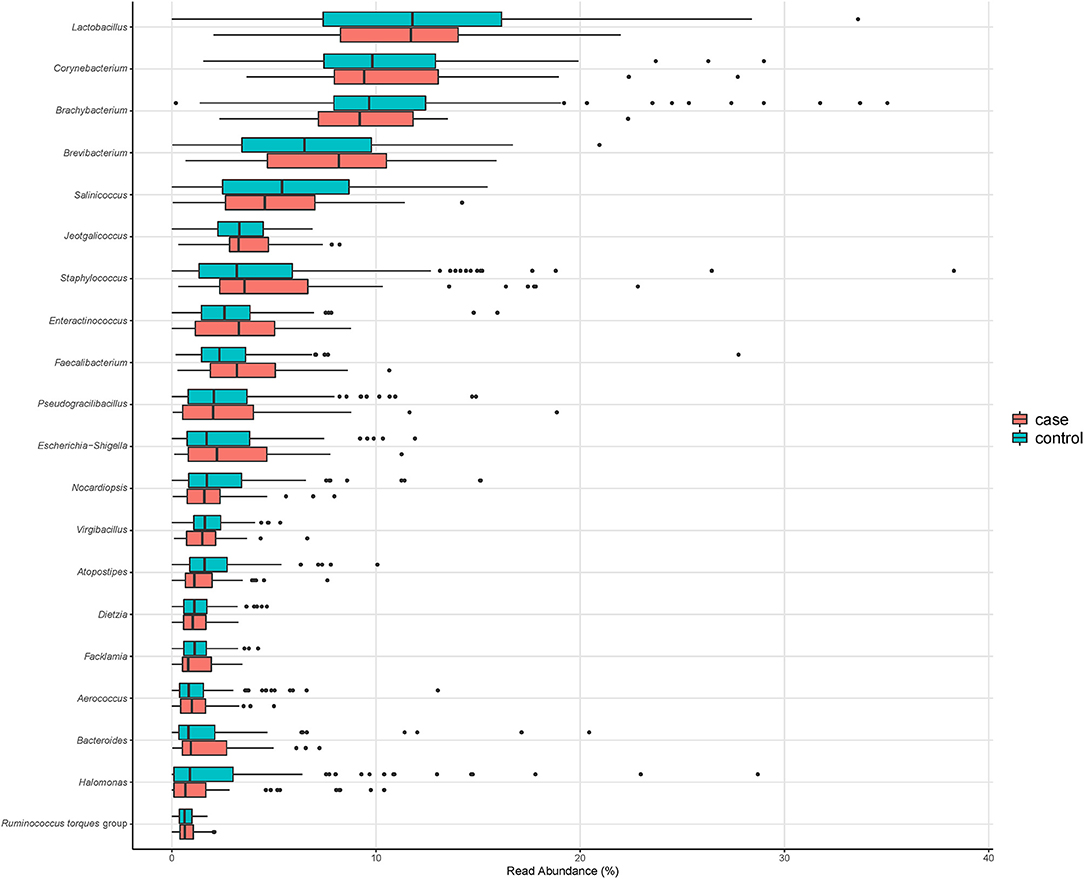
Figure 1. Relative abundance of the 20 most abundant genera in composite broiler litter samples. Samples are separated by the Campylobacter isolation status of the flock. Flocks were classified as cases when either C. jejuni or C. coli was isolated in boot sock samples, or as controls otherwise.
Alpha Diversity
Using a conditional logistic regression model, it was observed that alpha diversity indices measuring species richness (Chao1, ACE, and Fisher) of composite broiler litter were negatively associated with the odds of Campylobacter isolation in boot socks. By contrast, alpha diversity indices estimating species richness and evenness (Inverse Simpson and Shannon) were positively associated with the odds of Campylobacter isolation. In other words, fewer bacterial species were detected, on average, in the litter of flocks with Campylobacter isolation. The distribution of the species was more homogeneous when compared with litter of flocks without isolation of Campylobacter (Figure 2). The associations did not reach statistical significance after adjustment for flock age and sampling season (Table 3).
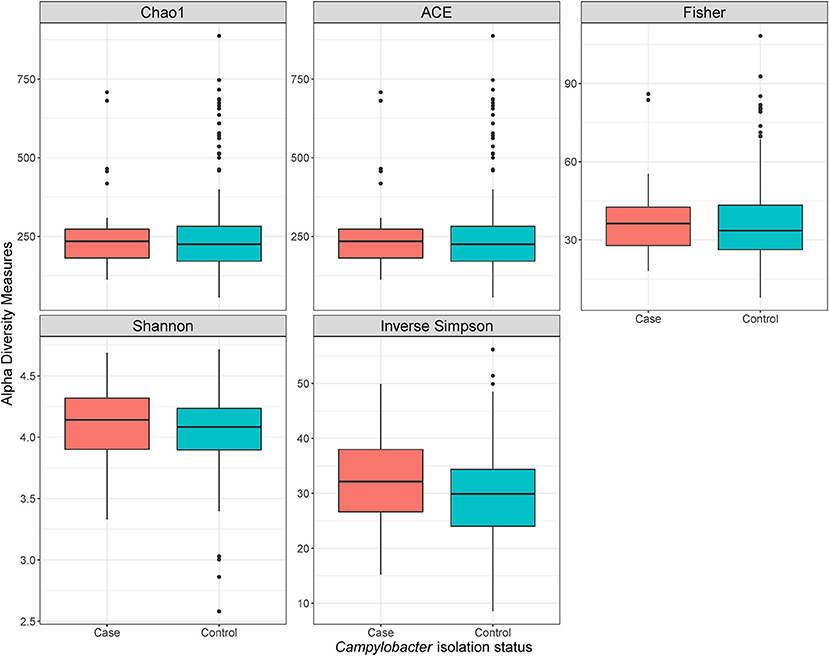
Figure 2. Alpha diversity plots of composite broiler litter samples. Samples are separated by the Campylobacter isolation status of the flock. Flocks were classified as cases when either C. jejuni or C. coli was isolated in boot sock samples, or as controls otherwise.
Beta Diversity
No evidence of distinct clustering of samples according to Campylobacter isolation status was observed in any of the evaluated beta diversity dissimilarity distances (Figure 3). Moreover, no statistically significant associations between Campylobacter isolation status and beta diversity dissimilarity distances calculated at the ASV level were detected (P-value = 0.131, 0.116, and 0.081, for Aitchison, Bray-Curtis, and Jaccard distances, respectively) after stratifying on broiler house to account for the matching. When beta diversity dissimilarity distances were calculated at the genus level, Campylobacter isolation status was shown to explain approximately 1% of the variance in Aitchison dissimilarity distance (stratified PERMANOVA: R2 = 0.007; P-value = 0.027). No significant association between Campylobacter isolation status and genus-level Bray-Curtis or Jaccard distances (P-values = 0.092 and 0.079, respectively) was detected.
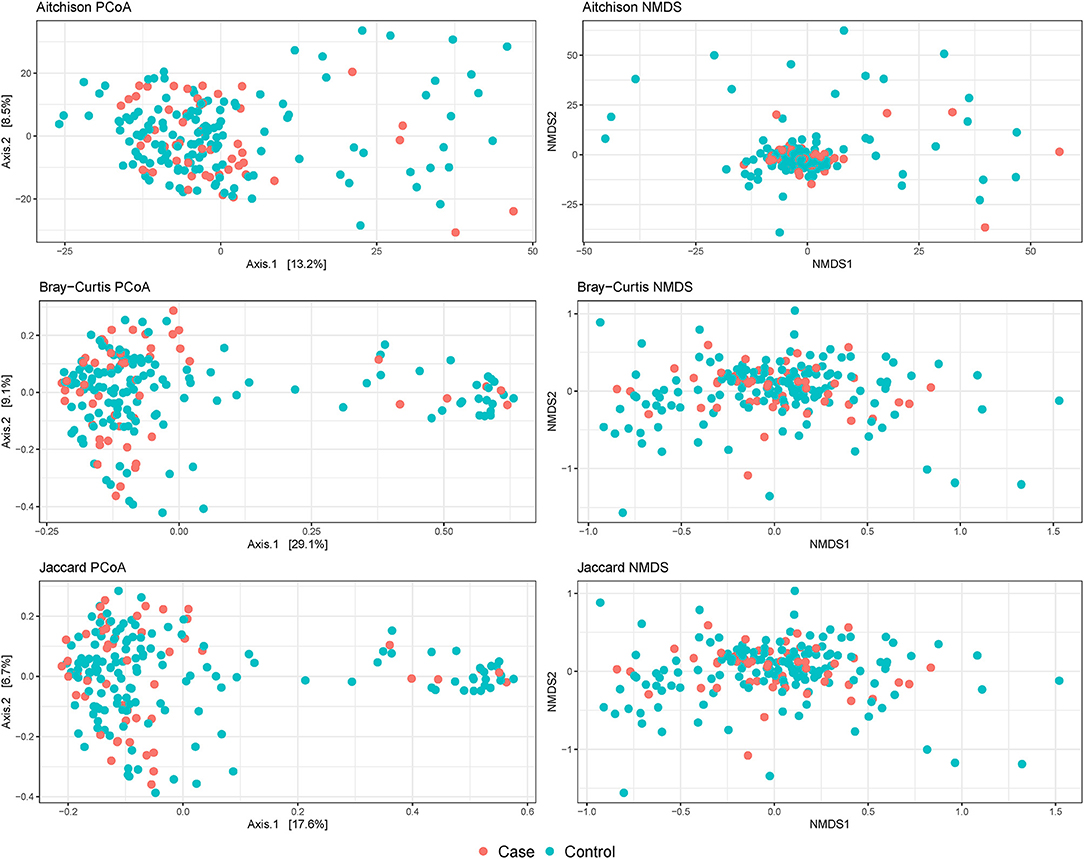
Figure 3. Beta diversity plots of composite broiler litter samples. Samples are colored by the Campylobacter isolation status of the flock. Cases (orange) were defined as flocks with isolation of C. jejuni or C. coli in boot sock samples, and controls (tile) were defined as flocks without isolation of C. jejuni or C. coli in boot sock samples.
Differentially Abundant Bacteria
When ASV counts were aggregated at the genus level, 16 genera were selected by the LASSO conditional logistic regression algorithm. Eight out of 16 genera, Biophila, Anaerostipes, Globicatella, Gracilibacillus, Anaerosporobacter, Salinimicrobium, Bifidobacterium, and Stenotrophomonas, were significantly associated with Campylobacter isolation status after adjusting for flock age and sampling season. When these nine genera were evaluated together in a conditional logistic regression model to estimate their independent association with Campylobacter isolation status, the relative abundance (transformed as centered log ratio) of Biophila and Anaerostipes was shown to be positively associated, while the relative abundance of the other bacterial genera was negatively associated. The adjusted ORs (aORs) for Anaerosporobacter, Bifidobacterium, and Stenotrophomonas reached statistical significance (Table 4 and Figure 4).
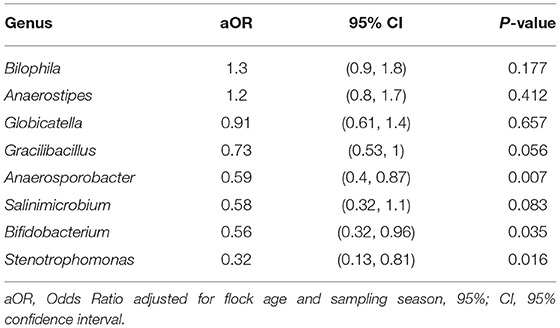
Table 4. Association of differentially abundant bacterial genera with the isolation of Campylobacter.
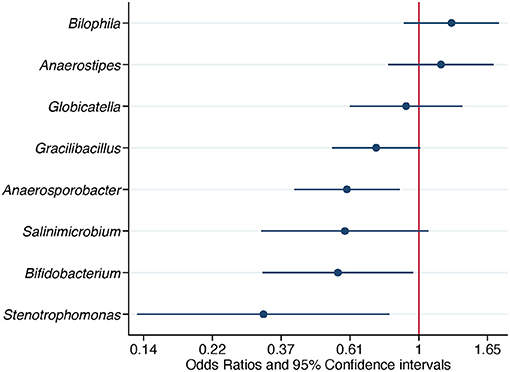
Figure 4. Association of differentially abundant bacterial genera with the isolation of Campylobacter. Odds ratios and 95% confidence intervals were obtained from a conditional logistic regression model and adjusted for flock age and sampling season. Differentially abundant bacterial genera were detected in composite litter samples. Log scale was used in the abscissa.
When ASV counts were not aggregated at the genus level, 15 ASVs were selected. Seven out of 15 ASVs were further evaluated after detecting a significant association with Campylobacter isolation status, adjusting for flock age and sampling season. Three ASVs, belonging to the genera Clostridium, Anaerostipes, and Rikenella, were positively associated with the odds of Campylobacter isolation. The aORs for Clostridium and Anaerostipes were statistically significant (Table 5 and Figure 5).
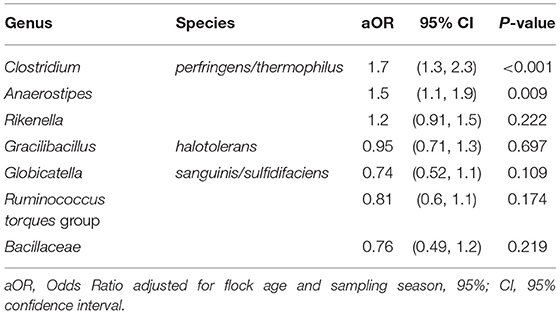
Table 5. Association of differentially abundant amplicon sequence variants with the isolation of Campylobacter.
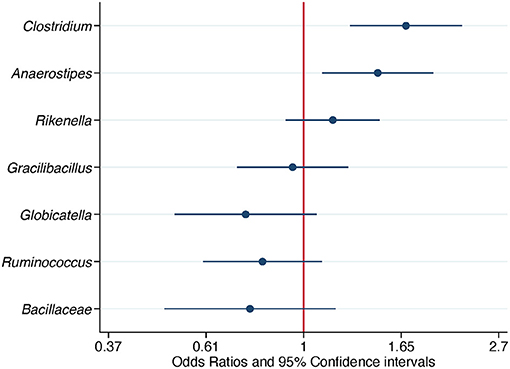
Figure 5. Association of differentially abundant amplicon sequence variants (ASVs) with the isolation of Campylobacter. Odds ratios and 95% confidence intervals were obtained from a conditional logistic regression model and adjusted for flock age and sampling season. Differentially abundant ASVs were detected in composite litter samples. The classification at the genus level or at the family level if the former was not obtained, is shown for each ASV. Log scale was used in the abscissa.
Two Campylobacter ASVs were detected, one assigned to C. coli/jejuni and another to C. coli/helveticus/jejuni/upsaliensis. Campylobacter relative abundance, both with and without aggregating at the genus level, was significantly associated with Campylobacter isolation status after adjusting for flock age and sampling season (genus-level aOR = 2.6, 95% CI = 1.8–3.7, P-value < 0.001; ASV-level aOR = 2.5, 95% CI = 1.8–3.5, P-value < 0.001). Campylobacter relative abundance was considered to be the mediator of the effects of the other members of the litter microbiome on Campylobacter isolation status of the flock; thus, it was not included in the final conditional logistic regression models.
Discussion
This study explored the association of broiler litter microbiome composition and the Campylobacter isolation status of the flock. The results of this study showed that the characteristics of the microbiome in broiler litter differed when categorized by the presence or absence of culturable Campylobacter in boot socks. Differences in the overall microbiome composition, captured by genus-level Aitchison distance, were significantly associated with the isolation of Campylobacter. Moreover, the relative abundance of several bacteria differed depending on the Campylobacter isolation status of the flock. Clostridium and Anaerostipes were significantly increased in abundance whereas Bifidobacterium, Anaerosporobacter, and Stenotrophomonas were significantly decreased when culturable Campylobacter was detected.
Three of the 20 most abundant genera identified in flocks with and without isolation of Campylobacter have not been previously reported in broiler litter. However, these three genera have been commonly detected either in the environment, such as soil and plant roots (Dietzia and Pseudogracilibacillus, respectively) (45, 46) or in the gut of broilers (Alistipes) (47), which is not surprising due to the dual nature of broiler litter as a mixture of bedding and chicken feces.
A nuanced association between alpha diversity of the broiler litter microbiome and the isolation of Campylobacter was observed, without reaching statistical significance. Alpha diversity captures the richness and evenness of a microbial population, which can be phrased as how many bacterial species coexist and how even their numbers are, respectively. In flocks with Campylobacter isolation, broiler litter microbiome richness was lower and evenness was higher than in flocks with no Campylobacter isolation. These data suggest that bacterial populations with few species and with a homogeneous composition may provide better support for the growth and viability of Campylobacter in broiler litter. Interestingly, our results contrast with those obtained by Bucher et al. (27), who observed that both richness and evenness were increased when litter microcosms were inoculated with Salmonella Heidelberg preconditioned in poultry litter extract.
Broiler litter has an important bacterial component originating in the chicken gut (48). Therefore, it is natural to compare the results of this study with those from research focusing on the interplay between Campylobacter and the gut microbiome, specifically, the cecal microbiome. Statistically non-significant associations between C. jejuni and alpha diversity indices have been documented in ceca from broiler chickens (49–51), with the exception of higher richness in the cecal content of 28-day-old chickens, 14 days after an experimental infection with C. jejuni (50).
A significant association was detected between beta diversity of the broiler litter microbiome and the isolation of Campylobacter, specifically when genus-level Aitchison dissimilarity distance was used as a beta diversity estimator. Changes in cecum beta diversity have been shown to occur after experimental inoculation of broiler chickens with C. jejuni (49–51). Beta diversity summarizes the overall differences between two microbial populations. Specific bacterial species, called differentially abundant bacteria, are responsible for the beta diversity observed between two microbial populations. Therefore, these results suggest that the broiler litter microbiome harbored bacterial species that increased or decreased when Campylobacter was isolated in boot socks. In fact, differentially abundant bacterial genera were detected. Besides the expected higher relative abundance of the genus Campylobacter in broiler litter when Campylobacter was isolated in boot socks, Clostridium, Anaerostipes, Bifidobacterium, Anaerosporobacter, and Stenotrophomonas relative abundance also significantly differed according to the Campylobacter isolation status of the flock.
A positive association was observed between Campylobacter isolation status and an ASV matching the 16S rRNA gene V4 region of Clostridium perfringens and Clostridium thermophilus. The genus Clostridium comprises obligate anaerobic endospore-forming Gram-positive bacteria, some of which are major pathogens for humans and domestic animals. The genus is commonly present in broiler feces, litter, and carcasses (52). Clostridium perfringens is the causative agent of necrotic enteritis in poultry and has been documented to alter the small intestine microbiome after experimental infection of broilers (53). Skånseng et al. (54) reported a positive correlation of the relative abundance of C. perfringens and that of C. jejuni in ceca from broiler chickens at slaughter. Similarly, Clostridium was shown to contribute to ~9% of the similarity in microbiome composition among C. jejuni positive cecal samples (55). However, lack of association between Clostridium and Campylobacter has also been documented. Froebel et al. (56) reported that Campylobacter counts in ceca significantly decreased whereas no significant effect on C. perfringens in ileum was observed in broilers after 42 days of prebiotic use. Furthermore, higher C. perfringens isolation rates were observed with no significant change in Campylobacter prevalence in broiler feces under a drug-free program (57).
An ASV belonging to the genus Anaerostipes was positively associated with Campylobacter isolation status of the flock. Anaerostipes is a genus of endospore-forming bacteria belonging to the family Lachnospiraceae. One species, Anaerostipes butyraticus, has been shown to be present in broiler chicken ceca and produce short-chain fatty acids (SCFA), specifically butyric acid, which promotes the normal functions of the gut (58). The relative abundance of Anaerostipes in the ceca has been shown to increase after the administration of xylo-oligosaccharides (59) and Bacillus subtilis (60) to broiler chickens. Connerton et al. (51) documented a negative association between Campylobacter and Anaerostipes in ceca after experimental infection of broiler chickens at 6 days of age, which contrasts with the positive association observed in broiler litter in this study. A potential explanation for this relationship is that Campylobacter might benefit from SCFA produced by Anaerostipes, as the utilization of such carbon source has been documented in the chicken gut (10).
The genus Anaerosporobacter is another member of the family Lachnospiraceae. It was first isolated in soil (61), but is also present in the gut of broiler chickens and pigs (62–64). Anaerosporobacter abundance was documented to increase in ceca as a result of the incorporation of enramycin to the feed of broiler chickens throughout a 43-day trial (64) and in feces of broiler chickens with high feed conversion ratio (63). In our study, the genus Anaerosporobacter relative abundance was negatively associated with Campylobacter isolation, which complements previous findings of the enhancement of the efficacy of a vaccine against Campylobacter in chickens by the co-administration of Anaerosporobacter mobilis as a probiotic (65).
The abundance of the genus Stenotrophomonas decreased when Campylobacter was isolated in boot socks. Stenotrophomonas are Gram-negative bacteria that are opportunistic pathogens in humans (66), are the predominant bacteria aerosolized from swine feces (67), and have been documented in the poultry environment (68), including litter (12) and poultry meat (69). Li et al. (70) reported that Stenotrophomonas was enriched in the jejunum of chickens reared at 33.5°C and was associated with multiple lipid metabolic pathways.
A significant negative association between the genus Bifidobacterium and Campylobacter isolation status was detected in our study. Bifidobacterium are Gram-positive anaerobic bacteria with an extensive arsenal of saccharolytic enzymes and are present in a variety of hosts and environments, including mammals, birds, and insects (71). Several species of Bifidobacterium (especially B. bifidum, B. animalis, and B. longum) are part of commercial probiotic and symbiotic formulations for domestic animals, including poultry (72). Bifidobacterium has been shown to competitively exclude Campylobacter in broilers. For instance, a one-log10 reduction of C. jejuni concentration in feces of broilers was observed after a 15-day treatment with B. longum in feed (73). Similar results were obtained when combining B. longum with the prebiotics galactooligosaccharide (74) and xylooligosaccharide (75).
It is important to highlight that the design of this research was a matched case-control study. Thus, confounders, variables affecting both the isolation of Campylobacter in boot socks and the composition of the broiler litter microbiome, need to be adjusted for. Matching on the broiler house accounted for the confounders that vary between broiler houses, for instance, use of probiotics/prebiotics, biosecurity measures, use of organic acids, and other litter treatments (76–79). Flock age and sampling season were adjusted for at the analysis stage as potential confounders. Despite all efforts, confounding might still be present after adjustment (residual confounding), potentially biasing results. In addition, the outcome of this study relied on bacterial isolation, a method with a moderate sensitivity for Campylobacter detection that might lead to misclassification of controls. As an example, if Campylobacter cells entered a viable not culturable state (16, 80), there is the possibility that flocks were assigned as controls due to lack of Campylobacter isolation in boot socks even though Campylobacter was present in the broiler house. However, the significant positive association between the relative abundance of Campylobacter ASVs in litter and the isolation of Campylobacter in boot socks supports the validity of the bacterial isolation approach.
Microbiome studies pose significant challenges, especially during the analysis of differentially abundant bacteria. Observational studies present additional challenges, such as the need for statistical methods that can properly handle highly dimensional compositional data while simultaneously controlling for confounding. In spite of efforts to set guidelines for the statistical analysis of microbiome data (81–84), no clear consensus has been reached in the field. In this study, we selected the centered log relative abundance of the ASVs in a LASSO conditional logistic regression to address the compositionality and the hyperdimensionality of the microbiome data, respectively. The LASSO selected features were included in conditional logistic regression models to estimate their association with the isolation of Campylobacter in boot socks. It is important to recognize that this penalized approach might not detect subtle associations with important biological implications. Fortunately, this is an active area of research, and suitable approaches will hopefully be developed and become widely adopted in the near future.
Our results suggest the existence of bacterial interactions between Campylobacter and members of the broiler litter microbiome, which may be a reflection of bacterial interactions occurring in the chicken gut. Broiler litter can be considered an environmental extension of the chicken gut since feces are a major component of that litter and chickens display a coprophagic behavior (85). Therefore, it is possible that interventions targeting the broiler litter may have an impact on the chicken gut microbiome. Likewise, it might be the case that probiotics administered to broiler chickens in order to modulate the gut microbiome also have indirect effects when passed on to the broiler litter microbiome through feces. In particular, the negative association of Campylobacter with Bifidobacterium, Anaerosporobacter, and Stenotrophomonas could be potentially exploited using probiotics/prebiotics to reduce the spread of Campylobacter in the flock.
This study showed that bacterial interactions related to Campylobacter in broiler litter are complex. In order to unravel this complexity and the intricate interrelation between the microbiome of the chicken gut and the broiler litter, future research should assess both microbial communities simultaneously while following flocks under commercial settings. In conclusion, the role of the broiler litter microbiome in the ecology of Campylobacter colonization and persistence on-farm needs to be understood to identify and establish effective pre-harvest interventions to control these bacteria. Reducing the risk of campylobacteriosis to consumers due to the application of interventions on the full spectrum of the food system, from farm to fork, will translate into a decrease of the burden that this foodborne disease lays upon the health, welfare, and economy of communities.
Data Availability Statement
The datasets presented in this study can be found in online repositories. The names of the repository/repositories and accession number(s) can be found below: https://www.ncbi.nlm.nih.gov/, PRJNA686691.
Author Contributions
RV-C and RS: conceptualization and investigation. RS: funding acquisition. RV-C, HH, and RS: methodology. RV-C, MP, TJ, and RS: formal analysis. RV-C, MP, and RS: visualization. MP and RS: supervision. RV-C and MP: writing—original draft preparation. RV-C, MP, HH, TJ, and RS: writing—review and editing. All authors have read and agreed to the published version of the manuscript.
Funding
This work was partially supported by Grants Number: 2015-68003-22972 and 2019-67017-29582 from the USDA National Institute of Food and Agriculture.
Conflict of Interest
The authors declare that the research was conducted in the absence of any commercial or financial relationships that could be construed as a potential conflict of interest.
Acknowledgments
The authors thank the broiler companies that voluntarily participated in the study.
References
1. Silva MF, Pereira G, Carneiro C, Hemphill A, Mateus L, Lopes-da-Costa L., et al. Campylobacter portucalensis sp. nov., a new species of Campylobacter isolated from the preputial mucosa of bulls. PLoS ONE. (2020) 15:e0227500. doi: 10.1371/journal.pone.0227500
2. Scallan E, Hoekstra RM, Angulo FJ, Tauxe RV, Widdowson MA, Roy SL, et al. Foodborne illness acquired in the United States–major pathogens. Emerg Infect Dis. (2011) 17:7–15. doi: 10.3201/eid1701.P11101
3. Tack DM, Marder EP, Griffin PM, Cieslak PR, Dunn J, Hurd S, et al. Preliminary incidence and trends of infections with pathogens transmitted commonly through food - Foodborne Diseases Active Surveillance Network, 10 US sites, 2015-2018. MMWR Morb Mortal Wkly Rep. (2019) 68:369–73. doi: 10.15585/mmwr.mm6816a2
4. Backert S, Tegtmeyer N, Cróinín TÓ, Boehm M, Heimesaat MM. Chapter 1 - Human campylobacteriosis. In: Klein G. editor. Campylobacter. Boston, MA: Academic Press (2017). p. 1–25. doi: 10.1016/B978-0-12-803623-5.00001-0
5. Cody AJ, Maiden MC, Strachan NJ, McCarthy ND. A systematic review of source attribution of human campylobacteriosis using multilocus sequence typing. Euro Surveill. (2019) 24:1800696. doi: 10.2807/1560-7917.ES.2019.24.43.1800696
6. Mughini-Gras L, Pijnacker R, Coipan C, Mulder AC, Fernandes Veludo A, de Rijk S, et al. Source and transmission routes of campylobacteriosis: a combined analysis of genome and exposure data. J Infect. (2021) 82:216–26. doi: 10.1016/j.jinf.2020.09.039
7. van Gerwe T, Miflin JK, Templeton JM, Bouma A, Wagenaar JA, Jacobs-Reitsma WF, et al. Quantifying transmission of Campylobacter jejuni in commercial broiler flocks. Appl Environ Microbiol. (2009) 75:625–8. doi: 10.1128/AEM.01912-08
8. Goddard AD, Arnold ME, Allen VM, Snary EL. Estimating the time at which commercial broiler flocks in Great Britain become infected with Campylobacter: a Bayesian approach. Epidemiol Infect. (2014) 142:1884–92. doi: 10.1017/S0950268813002926
9. Sahin O, Kassem II, Shen Z, Lin J, Rajashekara G, Zhang Q. Campylobacter in poultry: ecology and potential interventions. Avian Dis. (2015) 59:185–200. doi: 10.1637/11072-032315-Review
10. Awad WA, Dublecz F, Hess C, Dublecz K, Khayal B, Aschenbach JR, et al. Campylobacter jejuni colonization promotes the translocation of Escherichia coli to extra-intestinal organs and disturbs the short-chain fatty acids profiles in the chicken gut. Poult Sci. (2016) 95:2259–65. doi: 10.3382/ps/pew151
11. Awad WA, Hess C, Hess M. Re-thinking the chicken-Campylobacter jejuni interaction: a review. Avian Pathol. (2018) 47:352–63. doi: 10.1080/03079457.2018.1475724
12. Lu J, Sanchez S, Hofacre C, Maurer JJ, Harmon BG, Lee MD. Evaluation of broiler litter with reference to the microbial composition as assessed by using 16S rRNA and functional gene markers. Appl. Environ Microbiol. (2003) 69:901–8. doi: 10.1128/AEM.69.2.901-908.2003
13. Rauber Wurfel SdF, Voss-Rech D, Dos Santos Pozza J, Coldebella A, Santiago Silva V, et al. Population dynamics of thermotolerant campylobacter in broilers reared on reused litter. Foodborne Pathog Dis. (2019) 16:738–43. doi: 10.1089/fpd.2019.2645
14. Kassem II, Sanad Y, Gangaiah D, Lilburn M, Lejeune J, Rajashekara G. Use of bioluminescence imaging to monitor Campylobacter survival in chicken litter. J Appl Microbiol. (2010) 109:1988–97. doi: 10.1111/j.1365-2672.2010.04828.x
15. Smith S, Meade J, Gibbons J, McGill K, Bolton D, Whyte P. The impact of environmental conditions on Campylobacter jejuni survival in broiler faeces and litter. Infect. Ecol. Epidemiol. (2016) 6:31685. doi: 10.3402/iee.v6.31685
16. Kassem II, Helmy YA, Kathayat D, Candelero-Rueda RA, Kumar A, Deblais L., et al. Nonculturability might underestimate the occurrence of campylobacter in broiler litter. Foodborne Pathog Dis. (2017) 14:472–7. doi: 10.1089/fpd.2017.2279
17. Fernie DS, Park RWA. The isolation and nature of Campylobacters (microaerophilic vibrios) from laboratory and wild rodents. J. Med, Microbiol. (1977) 10:325–9. doi: 10.1099/00222615-10-3-325
18. Montrose MS, Shane SM, Harrington KS. Role of litter in the transmission of Campylobacter jejuni. Avian Dis. (1985) 29:392–9. doi: 10.2307/1590500
19. Jacobs-Reitsma WF, van de Giessen NM, Bolder NM, Mulder RWAW. Epidemiology of Campylobacter spp. at two Dutch broiler farms. Epidemiol Infect. (1995) 114:413–21. doi: 10.1017/S0950268800052122
20. Gregory E, Barnhart H, Dreesen DW, Stern NJ, Corn JL. Epidemiological study of Campylobacter spp. in broilers: source, time of colonization, and prevalence. Avian Dis. (1997) 41:890–8. doi: 10.2307/1592343
21. Hiett KL, Stern NJ, Fedorka-Cray P, Cox NA, Musgrove MT, Ladely S. Molecular Subtype Analyses of Campylobacter spp. from Arkansas and California Poultry Operations. Appl Environ Microbiol. (2002) 68:6220–36. doi: 10.1128/AEM.68.12.6220-6236.2002
22. Skov MN, Spencer AG, Hald B, Petersen L, Nauerby B, Carstensen B, et al. The role of litter beetles as potential reservoir for Salmonella enterica and thermophilic Campylobacter spp. between broiler flocks. Avian Dis. (2004) 48:9–18. doi: 10.1637/5698
23. Enticknap JJ, Nonogaki H, Place AR, Hill RT. Microbial diversity associated with odor modification for production of fertilizers from chicken litter. Appl Environ Microbiol. (2006) 72:4105–14. doi: 10.1128/AEM.02694-05
24. Lovanh N, Cook KL, Rothrock MJ, Miles DM, Sistani K. Spatial shift in microbial population structure within poultry litter associated with physicochemical properties. Poult Sci. (2007) 86:1840–9. doi: 10.1093/ps/86.9.1840
25. Cressman MD, Yu Z, Nelson MC, Moeller SJ, Lilburn MS, Zerby HN. Interrelations between the microbiotas in the litter and in the intestines of commercial broiler chickens. Appl Environ Microbiol. (2010) 76:6572–82. doi: 10.1128/AEM.00180-10
26. Dumas MD, Polson SW, Ritter D, Ravel J, Gelb J, et al. Impacts of poultry house environment on poultry litter bacterial community composition. PLoS ONE. (2011) 6:e24785. doi: 10.1371/journal.pone.0024785
27. Bucher MG, Zwirzitz B, Oladeinde A, Cook K, Plymel C, Zock G, et al. Reused poultry litter microbiome with competitive exclusion potential against Salmonella Heidelberg. J Environ Qual. (2020) 49:869–81. doi: 10.1002/jeq2.20081
28. Berghaus RD, Thayer SG, Law BF, Mild RM, Hofacre CL, Singer RS. Enumeration of Salmonella and Campylobacter spp. in environmental farm samples and processing plant carcass rinses from commercial broiler chicken flocks. Appl Environ Microbiol. (2013) 79:4106–14. doi: 10.1128/AEM.00836-13
29. Rodgers JD, Lawes JR, Vidal AB, Ellis-Iversen J, Ridley A, Pleydell EJ, et al. Characteristics and comparative performance of direct culture, direct PCR and enumeration methods for detection and quantification of Campylobacter spp. in broiler caeca. Vet Microbiol. (2012) 159:390–6. doi: 10.1016/j.vetmic.2012.04.011
30. Linton D, Owen RJ, Stanley J. Rapid identification by PCR of the genus Campylobacter and of five Campylobacter species enteropathogenic for man and animals. Res. Microbiol. (1996) 147:707–18. doi: 10.1016/S0923-2508(97)85118-2
31. Inglis GD, Kalischuk LD. Use of PCR for direct detection of Campylobacter species in bovine feces. Appl Environ Microbiol. (2003) 69:3435–47. doi: 10.1128/AEM.69.6.3435-3447.2003
32. Klena JD, Parker CT, Knibb K, Claire I, bbitt J, Devane PML, Horn ST, et al. Differentiation of Campylobacter coli, Campylobacter jejuni, Campylobacter lari, and Campylobacter upsaliensis by a multiplex PCR developed from the nucleotide sequence of the lipid A gene lpxA. J Clin Microbiol. (2004) 42:5549–57. doi: 10.1128/JCM.42.12.5549-5557.2004
33. Gohl DM, Vangay P, Garbe J, MacLean A, Hauge A, Becker A, et al. Systematic improvement of amplicon marker gene methods for increased accuracy in microbiome studies. Nat Biotechnol. (2016) 34:942–9. doi: 10.1038/nbt.3601
34. R Core Team. R: A Language and Environment for Statistical Computing. Vienna: R Foundation for Statistical Computing (2020).
35. Callahan BJ, McMurdie PJ, Rosen MJ, Han AW, Johnson AJ, Holmes SP. DADA2: high-resolution sample inference from Illumina amplicon data. Nat Methods. (2016) 13:581–3. doi: 10.1038/nmeth.3869
36. McLaren MR. Silva SSU taxonomic training data formatted for DADA2 (Silva version 138) [Dataset]. Zenodo, 2 (2020).
37. Wang Q, Garrity GM, Tiedje JM, Cole JR. Naive Bayesian classifier for rapid assignment of rRNA sequences into the new bacterial taxonomy. Appl Environ Microbiol. (2007) 73:5261–7. doi: 10.1128/AEM.00062-07
38. Edgar RC. Updating the 97% identity threshold for 16S ribosomal RNA OTUs. Bioinformatics. (2018) 34:2371–5. doi: 10.1093/bioinformatics/bty113
39. Davis NM, Proctor DM, Holmes SP, Relman DA, Callahan BJ. Simple statistical identification and removal of contaminant sequences in marker-gene and metagenomics data. Microbiome. (2018) 6:226. doi: 10.1186/s40168-018-0605-2
40. Oksanen J, Blanchet FG, Friendly M, Kindt R, Legendre P, McGlinn D, et al. Vegan: Community ecology package. R package version 2.5–6 (2019).
42. Quinn TP, Erb I, Richardson MF, Crowley TM. Understanding sequencing data as compositions: an outlook and review. Bioinformatics. (2018) 34:2870–8. doi: 10.1093/bioinformatics/bty175
43. Shankar J, Szpakowski S, Solis NV, Mounaud S, Liu H, Losada L, et al. A systematic evaluation of high-dimensional, ensemble-based regression for exploring large model spaces in microbiome analyses. BMC Bioinform. (2015) 16:31. doi: 10.1186/s12859-015-0467-6
44. Reid S, Tibshirani R. Regularization paths for conditional logistic regression: the clogitL1 package. J Stat Softw. (2014) 58:1–23. doi: 10.18637/jss.v058.i12
45. Procopio L, Alvarez VM, Jurelevicius DA, Hansen L, Sorensen SJ, Cardoso JS., et al. Insight from the draft genome of Dietzia cinnamea P4 reveals mechanisms of survival in complex tropical soil habitats and biotechnology potential. Antonie Van Leeuwenhoek. (2012) 101:289–302. doi: 10.1007/s10482-011-9633-7
46. Park J, Kim MK, Yun BR, Han JH, Kim SB. Pseudogracilibacillus endophyticus sp. nov., a moderately thermophilic and halophilic species isolated from plant root. Int J Syst Evol Microbiol. (2018) 68:165–9. doi: 10.1099/ijsem.0.002475
47. De Maesschalck C, Eeckhaut V, Maertens L, De Lange L, Marchal L, Daube G, et al. Amorphous cellulose feed supplement alters the broiler caecal microbiome. Poult Sci. (2019) 98:3811–7. doi: 10.3382/ps/pez090
48. Oakley BB, Morales CA, Line J, Berrang ME, Meinersmann RJ, Tillman GE, et al. The poultry-associated microbiome: network analysis and farm-to-fork characterizations. PLoS ONE. (2013) 8:e57190. doi: 10.1371/journal.pone.0057190
49. Thibodeau A, Fravalo P, Yergeau É, Arsenault J, Lahaye L, Letellier A. Chicken Caecal Microbiome modifications induced by Campylobacter jejuni colonization and by a non-antibiotic feed additive. PLoS ONE. (2015) 10:e0131978. doi: 10.1371/journal.pone.0131978
50. Awad WA, Mann E, Dzieciol M, Hess C, Schmitz-Esser S, Wagner M, et al. Age-Related differences in the luminal and mucosa-associated gut microbiome of broiler chickens and shifts associated with Campylobacter jejuni infection. Front Cell Infect Microbiol. (2016) 6:154. doi: 10.3389/fcimb.2016.00154
51. Connerton PL, Richards PJ, Lafontaine GM, O'Kane PM, Ghaffar N, Cummings NJ, et al. The effect of the timing of exposure to Campylobacter jejuni on the gut microbiome and inflammatory responses of broiler chickens. Microbiome. (2018) 6:88. doi: 10.1186/s40168-018-0477-5
52. Oakley BB, Lillehoj HS, Kogut MH, Kim WK, Maurer JJ, Pedroso A, et al. The chicken gastrointestinal microbiome. FEMS Microbiol Lett. (2014) 360:100–12. doi: 10.1111/1574-6968.12608
53. Fasina YO, Newman MM, Stough JM, Liles MR. Effect of Clostridium perfringens infection and antibiotic administration on microbiota in the small intestine of broiler chickens. Poult Sci. (2016) 95:247–60. doi: 10.3382/ps/pev329
54. Skånseng B, Kaldhusdal M, Rudi K. Comparison of chicken gut colonisation by the pathogens Campylobacter jejuni and Clostridium perfringens by real-time quantitative PCR. Mol Cell Probes. (2006) 20:269–79. doi: 10.1016/j.mcp.2006.02.001
55. Kaakoush NO, Sodhi N, Chenu JW, Cox JM, Riordan SM, Mitchell HM. The interplay between Campylobacter and Helicobacter species and other gastrointestinal microbiota of commercial broiler chickens. Gut Pathog. (2014) 6:18–18. doi: 10.1186/1757-4749-6-18
56. Froebel LK, Jalukar S, Lavergne TA, Lee JT, Duong T. Administration of dietary prebiotics improves growth performance and reduces pathogen colonization in broiler chickens. Poult Sci. (2019) 98:6668–76. doi: 10.3382/ps/pez537
57. Gaucher ML, Quessy S, Letellier A, Arsenault J, Boulianne M. Impact of a drug-free program on broiler chicken growth performances, gut health, Clostridium perfringens and Campylobacter jejuni occurrences at the farm level. Poult Sci. (2015) 94:1791–801. doi: 10.3382/ps/pev142
58. Eeckhaut V, Van I, mmerseel F, Pasmans F, De Brandt E, Haesebrouck F, Ducatelle R, et al. Anaerostipes butyraticus sp. nov., an anaerobic, butyrate-producing bacterium from Clostridium cluster XIVa isolated from broiler chicken caecal content, and emended description of the genus Anaerostipes. Int J Syst Evol Microbiol. (2010) 60:1108–12. doi: 10.1099/ijs.0.015289-0
59. De Maesschalck C, Eeckhaut V, Maertens L, De Lange L, Marchal L, Nezer C, et al. Effects of Xylo-Oligosaccharides on Broiler Chicken Performance and Microbiota. Appl Environ Microbiol. (2015) 81:5880–8. doi: 10.1128/AEM.01616-15
60. Jacquier V, Nelson A, Jlali M, Rhayat L, Brinch KS, Devillard E. Bacillus subtilis 29784 induces a shift in broiler gut microbiome toward butyrate-producing bacteria and improves intestinal histomorphology and animal performance. Poult Sci. (2019) 98:2548–54. doi: 10.3382/ps/pey602
61. Jeong H, Lim YW, Yi H, Sekiguchi Y, Kamagata Y, Chun J. Anaerosporobacter mobilis gen. nov., sp. nov., isolated from forest soil. Int J Syst Evol Microbiol. (2007) 57:1784–7. doi: 10.1099/ijs.0.63283-0
62. Kim HB, Borewicz K, White BA, Singer RS, Sreevatsan S, Tu ZJ, et al. Longitudinal investigation of the age-related bacterial diversity in the feces of commercial pigs. Vet Microbiol. (2011) 153:124–33. doi: 10.1016/j.vetmic.2011.05.021
63. Singh KM, Shah T, Deshpande S, Jakhesara SJ, Koringa PG, Rank DN, et al. High through put 16S rRNA gene-based pyrosequencing analysis of the fecal microbiota of high FCR and low FCR broiler growers. Mol Biol Rep. (2012) 39:10595–602. doi: 10.1007/s11033-012-1947-7
64. Costa MC, Bessegatto JA, Alfieri AA, Weese JS, Filho JA, Oba A. Different antibiotic growth promoters induce specific changes in the cecal microbiota membership of broiler chicken. PLoS ONE. (2017) 12:e0171642. doi: 10.1371/journal.pone.0171642
65. Nothaft H, Perez-Muñoz ME, Gouveia GJ, Duar RM, Wanford JJ, Lango-Scholey L, et al. Co-administration of the Campylobacter jejuni N-glycan based vaccine with probiotics improves vaccine performance in broiler chickens. Appl Environ Microbiol. (2017) 83:e01523-17. doi: 10.1128/AEM.01523-17
66. Brooke JS. Stenotrophomonas maltophilia: an emerging global opportunistic pathogen. Clin Microbiol Rev. (2012) 25:2–41. doi: 10.1128/CMR.00019-11
67. Chien YC, Chen CJ, Lin TH, Chen SH, Chien YC. Characteristics of microbial aerosols released from chicken and swine feces. J Air Waste Manag Assoc. (2011) 61:882–9. doi: 10.3155/1047-3289.61.8.882
68. Shah DH, Board MM, Crespo R, Guard J, Paul NC, Faux C. The occurrence of Salmonella, extended-spectrum beta-lactamase producing Escherichia coli and carbapenem resistant non-fermenting Gram-negative bacteria in a backyard poultry flock environment. Zoonoses Public Health. (2020) 67:742–53. doi: 10.1111/zph.12756
69. Yamamoto S, Nakayama T, Asakura H. Draft genome sequence of Stenotrophomonas maltophilia CRB139-1, isolated from poultry meat in Japan. Microbiol Resour Announc. (2020) 9:e00075-20. doi: 10.1128/MRA.00075-20
70. Li X, Cao Z, Yang Y, Chen L, Liu J, Lin Q, et al. Correlation between jejunal microbial diversity and muscle fatty acids deposition in broilers reared at different ambient temperatures. Sci Rep. (2019) 9:11022. doi: 10.1038/s41598-019-47323-0
71. Bottacini F, van Sinderen D, Ventura M. Omics of bifidobacteria: research and insights into their health-promoting activities. Biochem J. (2017) 474:4137–52. doi: 10.1042/BCJ20160756
72. Markowiak P, Slizewska K. The role of probiotics, prebiotics and synbiotics in animal nutrition. Gut Pathog. (2018) 10:21. doi: 10.1186/s13099-018-0250-0
73. Santini C, Baffoni L, Gaggia F, Granata M, Gasbarri R, Gioia D, et al. Characterization of probiotic strains: an application as feed additives in poultry against Campylobacter jejuni. Int J Food Microbiol. (2010) 141:S98–108. doi: 10.1016/j.ijfoodmicro.2010.03.039
74. Baffoni L, Gaggia F, Gioia Di D, Santini C, Mogna L, Biavati B. A Bifidobacterium-based synbiotic product to reducer the transmission of C. jejuni along the poultry food chain. Int J Food Microbiol. (2012) 157:156–61. doi: 10.1016/j.ijfoodmicro.2012.04.024
75. Baffoni L, Gaggia F, Garofolo G, Serafino Di G, Buglione E, Di Giannatale E, et al. Evidence of Campylobacter jejuni reduction in broilers with early synbiotic administration. Int J Food Microbiol. (2017) 251:41–7. doi: 10.1016/j.ijfoodmicro.2017.04.001
76. Line JE. Campylobacter and Salmonella populations associated with chickens raised on acidified litter. Poult Sci. (2002) 81:1473–7. doi: 10.1093/ps/81.10.1473
77. Willis WL, Reid L. Investigating the effects of dietary probiotic feeding regimens on broiler chicken production and Campylobacter jejuni presence. Poult Sci. (2008) 87:606–11. doi: 10.3382/ps.2006-00458
78. Kelly C, Gundogdu O, Pircalabioru G, Cean A, Scates P, Linton M, et al. The in vitro and in vivo effect of carvacrol in preventing campylobacter infection, colonization and in improving productivity of chicken broilers. Foodborne Pathog Dis. (2017) 14:341–9. doi: 10.1089/fpd.2016.2265
79. Smialek M, Burchardt S, Koncicki A. The influence of probiotic supplementation in broiler chickens on population and carcass contamination with Campylobacter spp - Field study. Res Vet Sci. (2018) 118:312–6. doi: 10.1016/j.rvsc.2018.03.009
80. Kassem I, Chandrashekhar K, Rajashekara G. Of energy and survival incognito: a relationship between viable but non-culturable cells formation and inorganic polyphosphate and formate metabolism in Campylobacter jejuni. Front Microbiol. (2013) 4:183. doi: 10.3389/fmicb.2013.00183
81. McMurdie PJ, Holmes S. Waste not, want not: why rarefying microbiome data is inadmissible. PLoS Comput Biol. (2014) 10:e1003531. doi: 10.1371/journal.pcbi.1003531
82. Callahan BJ, Sankaran K, Fukuyama JA, McMurdie PJ, Holmes SP. Bioconductor workflow for microbiome data analysis: from raw reads to community analyses. F1000Res. (2016) 5:1492. doi: 10.12688/f1000research.8986.2
83. Gloor GB, Macklaim JM, Pawlowsky-Glahn V, Egozcue JJ. Microbiome datasets are compositional: and this is not optional. Front Microbiol. (2017) 8:2224. doi: 10.3389/fmicb.2017.02224
84. Knight R, Vrbanac A, Taylor BC, Aksenov A, Callewaert C, Debelius J, et al. Best practices for analysing microbiomes. Nat Rev Microbiol. (2018) 16:410–22. doi: 10.1038/s41579-018-0029-9
Keywords: Campylobacter, broilers, litter, microbiome, case-control
Citation: Valeris-Chacin R, Pieters M, Hwang H, Johnson TJ and Singer RS (2021) Association of Broiler Litter Microbiome Composition and Campylobacter Isolation. Front. Vet. Sci. 8:654927. doi: 10.3389/fvets.2021.654927
Received: 08 March 2021; Accepted: 19 April 2021;
Published: 24 May 2021.
Edited by:
Min Yue, Zhejiang University, ChinaReviewed by:
Ali Raza Jahejo, Shanxi Agricultural University, ChinaGuangxu Ma, Zhejiang University, China
Alaa Sewid, The University of Tennessee, Knoxville, United States
Copyright © 2021 Valeris-Chacin, Pieters, Hwang, Johnson and Singer. This is an open-access article distributed under the terms of the Creative Commons Attribution License (CC BY). The use, distribution or reproduction in other forums is permitted, provided the original author(s) and the copyright owner(s) are credited and that the original publication in this journal is cited, in accordance with accepted academic practice. No use, distribution or reproduction is permitted which does not comply with these terms.
*Correspondence: Randall S. Singer, cnNpbmdlciYjeDAwMDQwO3Vtbi5lZHU=