- 1Molecular Morphology and Development Laboratory, Department of Veterinary Medicine, College of Animal Sciences and Food Engineering, University of São Paulo, Pirassununga, Brazil
- 2Graduate Department, Faculty of Veterinary Medicine and Animal Science, National University of Colombia, Bogotá, Colombia
- 3Molecular Endocrinology Physiology Laboratory, Department of Animal Reproduction, School of Veterinary Medicine and Animal Science, University of São Paulo, São Paulo, Brazil
Early embryonic development occurs in the oviduct, where an ideal microenvironment is provided by the epithelial cells and by the oviductal fluid produced by these cells. The oviductal fluid contains small extracellular vesicles (sEVs), which through their contents, including microRNAs (miRNAs), can ensure proper cell communication between the mother and the embryo. However, little is known about the modulation of miRNAs within oviductal epithelial cells (OECs) and sEVs from the oviductal fluid in pregnant cows. In this study, we evaluate the miRNAs profile in sEVs from the oviductal flushing (OF-sEVs) and OECs from pregnant cows compared to non-pregnant, at 120 h after ovulation induction. In OF-sEVs, eight miRNAs (bta-miR-126-5p, bta-miR-129, bta-miR-140, bta-miR-188, bta-miR-219, bta-miR-345-3p, bta-miR-4523, and bta-miR-760-3p) were up-regulated in pregnant and one miRNA (bta-miR-331-5p) was up-regulated in non-pregnant cows. In OECs, six miRNAs (bta-miR-133b, bta-miR-205, bta-miR-584, bta-miR-551a, bta-miR-1193, and bta-miR-1225-3p) were up-regulated in non-pregnant and none was up-regulated in pregnant cows. Our results suggest that embryonic maternal communication mediated by sEVs initiates in the oviduct, and the passage of gametes and the embryo presence modulate miRNAs contents of sEVs and OECs. Furthermore, we demonstrated the transcriptional levels modulation of selected genes in OECs in pregnant cows. Therefore, the embryonic-maternal crosstalk potentially begins during early embryonic development in the oviduct through the modulation of miRNAs in OECs and sEVs in pregnant cows.
Introduction
The oviduct provides the ideal microenvironment for early embryonic development (1, 2). The bovine embryo develops in the oviduct for 4 days, staying in contact with oviductal epithelial cells (OECs) as well as its secretions, the oviductal fluid (3). The oviductal epithelium is composed of ciliated cells and secretory cells that produce the oviductal fluid (4). The oviductal fluid is composed of simple and complex carbohydrates, proteins, ions, inorganic salts, lipids, phospholipids, small metabolites, and vesicles (4, 5). Changes in this fluid and the oviductal cells are regulated by different factors, such as the oviduct region (ampulla or isthmus), the ipsilateral or contralateral side to the corpus luteum (6–8), and the concentration of steroid hormones (9, 10).
The oviductal fluid and OECs are also affected by the presence of gametes and embryos. Different studies have demonstrated changes in gene expression patterns of bovine OECs co-cultured with embryos (11, 12). In vivo, despite no differences in OECs gene expression were found using microarray analysis in the presence of a single embryo in artificially inseminated heifers compared to cyclic heifers by Maillo et al. (13) or using target genes analysis by Rodríguez-Alonso et al. (14), the same group identified differentially expressed genes by RNA-seq when multiples embryos were transferred (13). In the oviductal fluid, different proteins were described indicating that the fluid composition could be influenced by the presence of gametes (15). Recently, Rodríguez-Alonso et al. (16) demonstrated that the oviductal fluid presents a different composition of carbohydrates, proteins, and amino acids in pregnant cows (artificially inseminated) when compared to cyclic cows (non-breed). Thus, it is possible that other components within the oviductal environment, such as extracellular vesicles present in the oviductal fluid, can also be modulated in pregnant animals.
EVs are nanoparticles secreted by cells in the extracellular environment (17) and contain bioactive materials, such as proteins (18), lipids (19), mRNAs, and non-coding RNAs, including microRNAs (miRNAs) (20, 21). EVs are classified as exosomes, microvesicles, and apoptotic bodies according to their size, biogenesis, and secretion (22). However, due to the difficulty to establish the specific origin of these EVs, they can be classified according to their size as small EVs (sEVs; <200 nm) or large EVs (lEVs; >200 nm) (23). In the oviduct, EVs have been identified in the oviductal fluid and are potentially related to the maternal crosstalk with gametes and embryos (24–28). Functionally, both sEVs originating from the oviductal fluid and bovine OECs conditioned culture medium are internalized by bovine embryos and improve in vitro embryo development and quality (29–31). However, the protein profile of sEVs that originated from OECs in vivo is different from the sEVs produced by OECs in vitro (31). Lopera-Vasquez et al. (29) demonstrated that sEVs from the isthmus have a better effect on in vitro embryo quality than sEVs from the ampulla. Although they have not studied the content of these sEVs, it can be inferred that the contents from the isthmus are somehow different and better for the embryo. The molecular contents of oviductal sEVs (RNA, protein, and small ncRNA, including miRNAs) are also regulated by steroid hormones across the bovine estrous cycle (32). To our knowledge, there are no studies on how the sEVs miRNA contents are modulated in pregnant cows.
miRNAs are small non-coding molecules (~22 nucleotides) that act in post-transcriptional regulation (33, 34). miRNAs are transcribed as primary miRNAs (pri-miRNAs), which are processed in precursor miRNA (pre-miRNA) and then transferred to the cytoplasm for further processing into mature miRNAs sequences (35). In mammals, the partial complementary base pairing miRNA-mRNA can induce transcription inhibition, thus regulating protein levels (36). miRNAs are involved in different reproductive events, such as spermatogenesis (37), follicular development (38, 39), oocyte maturation (40, 41), and embryo development (42). To our knowledge, there are no studies on miRNAs regulation and function in OECs from pregnant cows. miRNAs are also involved in cell-to-cell communication through sEVs (43). miRNAs within sEVs may play a role in modulating embryo-maternal communication during early pregnancy (44, 45). EVs can mediate the delivery of its miRNAs contents into embryos, a phenomenon already implicated in embryo development and maternal-embryonic communication (45–48). However, there is no evidence of the effects on miRNA contents of the oviductal sEVs from pregnant cows during the first embryo cleavages at the oviduct.
Therefore, the modulation and function of miRNAs within oviductal epithelial cells and EVs from the oviductal fluid in early pregnant cows is still elusive. Thus, we hypothesize that the miRNAs contents in small extracellular vesicles from the oviductal flushing (OF-sEVs) and in oviductal epithelial cells (OECs) undergo differential modulations in pregnant cows. The present study evaluated the profile of 383 miRNAs in OF-sEVs and OECs in artificially inseminated (pregnant group) and in sham-inseminated (non-pregnant group) cows. Additionally, we access in OECs genes known to influence the immune system, inflammation, and early pregnancy development. To our knowledge, this is the first study to characterize the changes in miRNA profile from both OF-sEVs and OECs obtained from pregnant and non-pregnant cows. Additionally, functional enrichment analysis was performed to evaluate the predicted pathways related to differentially expressed miRNAs between pregnant and non-pregnant cows.
Materials and Methods
All the reagents were purchased from Sigma–Aldrich Chemical Company (St. Louis, MO) unless otherwise stated. The present study was approved by the University of São Paulo Research Ethics Committee (protocol number: 4909010817).
Animal Model
This study was conducted at the University of São Paulo (campus of Pirassununga, SP, Brazil) with Nelore (Bos indicus) multiparous cows with a body condition score 4 (0-emaciated; 5-obese) according to Ayres et al. (49). Cows were fed with corn silage, supplemented with mineral salt, and free access to potable water. In order to obtain the oviducts exposed or not exposed to embryos, 25 cows were submitted to estrous synchronization protocol. As described by Gonella-Diaza (50) with minor modifications: on the first day, all the cows received 2 mg of estradiol benzoate (2 ml, i.m., Sincrodiol, Ourofino Saúde Animal, Brazil), 0.53 mg of sodium cloprostenol (2 ml, i.m., Sincrocio, Ourofino Saúde Animal, Brazil) and an intravaginal P4-releasing device (1 g; Sincrogest®, Ourofino Saúde Animal, Brazil). On day 7, the P4 device was removed, and cows received 0.53 mg of sodium cloprostenol (2 ml, i.m., Sincrocio, Ourofino Saúde Animal, Brazil). On day 9, cows received 0.01 mg of gonadotrophin releasing hormone (GnRH) analog (2.5 ml, i.m., Sincroforte, Ourofino Saúde Animal, Brazil). Twelve hours after GnRH analog application, cows were randomly assigned into two groups: fixed-time artificial inseminated (FTAI, n = 14) or sham-inseminated (control, n = 11). In the group FTAI, cows were artificially inseminated with frozen-thawed semen from the same bull of proved fertility. In the control group, cows were sham-inseminated with deposition of depleted semen extender in the uterine tract. For sperm depletion, straws from the same bull used in the FTAI group were thawed, centrifuged at 4,000 × g for 15 min, and the supernatant was filled into a new straw. The ovulation time was controlled by ultrasound (MyLab Delta, Esaote, Italy) and only animals that had an ovulated follicle between 24 and 36 h after GnRH analog application were kept in this study. All cows were slaughtered about 120 h after ovulation induction with GnRH at the slaughterhouse at University of São Paulo (campus of Pirassununga/SP, Brazil).
Sample Collection and Groups Formation
Reproductive tracts were processed within 30 min after slaughter. Oviducts ipsilateral to the corpus luteum (CL) were dissected and separated from the utero-tubal junction. Next, oviducts were divided in ampulla and isthmus through the ampullary–isthmic junction, identified as the place where the oviductal diameter starts to decrease, as described by Maillo et al. (51) and Gonella-Diaza et al. (52). The isthmus was individually flushed with 1 ml of calcium and magnesium-free PBS with 0.1% of polyvinylpyrrolidone (PVP). The presence or absence of the embryo within the isthmus portion was used for group formation and confirmed in the oviductal flushing using a stereo microscope. After washing, epithelial cells of each isthmus were obtained by squeezing the tissue using a sterile glass slide and frozen in liquid nitrogen until further use.
In addition to the criterion of the presence or absence of the embryo, we also analyzed pre-ovulatory follicle (POF) diameter, weight, and diameter of the CL and serum concentration of progesterone (P4) and estrogen (E2). The POF diameter was measured by ultrasound (MyLab Delta, Esaote, Italy) on day 9 of the estrous synchronization protocol. For CL weight and size, CLs were dissected from the ovary, weighed, and their diameter was calculated as the average between two perpendicular axes measured. For serum concentration of P4 and E2, blood samples were collected from the jugular vein in serum blood collection tubes (BD, São Paulo/SP, Brazil) at the time of slaughter. The serum was separated by centrifugation at 1,500 × g for 30 min, stored at −20°C, and the measurement of E2 and P4 was performed using chemiluminescence immunoassay (ADVIA Centaur®-Siemens) at Pasin Laboratory (Santa Maria/RS, Brazil).
Isolation of OF-sEVs
OF-sEVs were obtained from the isthmus of ipsilateral oviducts from pregnant and non-pregnant groups according to the isolation protocol previously used by da Silveira et al. (53), Alminaña et al. (32), and Ávila et al. (54). Briefly, the oviductal flushing was centrifuged at 300 × g for 10 min to remove live cells, 2,000 × g for 10 min to remove cellular debris, 16,500 × g for 30 min to remove large vesicles, and the remaining supernatant was placed at −80°C until further use. The supernatant was filtered through a 0.20 μm sterile syringe filter (PES membrane; Corning) in order to remove any remaining large EVs. For sEVs isolation, filtered oviductal flushing was centrifuged twice at 119,700 × g for 70 min at 4°C (Optima XE-90 Ultracentrifuge; rotor 70 Ti; Beckman Coulter). After this procedure, the supernatant was discarded, and the sEVs pellets were resuspended in 20 μL of phosphate-buffered saline (1 × Ca2+/Mg2+ free PBS; 137 mM NaCl, 2.7 mM KCl, 10 mM Na2HPO4, 2 mM KH2PO4) and used for further analysis. Due to the small volume of oviductal flushing obtained from a single animal, the protocol to isolate OF-sEVs was validated using female reproductive tracts obtained from slaughterhouses, as described in Supplementary Data 1.
Nanoparticle Tracking Analysis
The OF-sEVs isolated from pregnant and non-pregnant groups were analyzed based on particle mode size and concentration by nanoparticle tracking analysis (NTA). OF-sEVs isolated from 200 μL of each oviductal flushing were individually centrifuged, and their pellets were resuspended in 20 μL of 1 × Ca2+/Mg2+ free PBS. As described by Ávila et al. (54), with minimal modifications, particle size, and concentration were measured by nanoparticle tracking analysis using Nanosight (NS300; Malvern). Briefly, for this analysis, the dilution factor used was 1: 100 in 1 × Ca2+/Mg2+ free PBS. Five videos of 30 s were taken for each sample, captured by a scientific complementary metal–oxide–semiconductor (sCMOS) camera at camera level 13 and under a controlled temperature of 38.5°C. Considering the threshold level of 5, these captured images were tracked by the NanoSight NTA 3.4 Analytical Software (NTA 3.4 Build 3.4.003; Malvern) that measures and provides information about particle size and concentrations of each sample.
Total RNA Extraction, Reverse Transcription, and Real-Time PCR
Total RNA, including miRNAs, from OF-sEVs and OECs was extracted according to the TRIzol reagent (Thermo Fisher Scientific) manufacturer's instruction and with the addition of 1.33 μL the coprecipitator GlycoBlue (Thermo Fisher Scientific) to the aqueous phase before RNA precipitation, as previously described by Da Silveira et al. (55) and Gonella-Diaza et al. (56) with minimal modifications. Total RNA concentrations were analyzed using spectrometry (NanoDrop 2000, Thermo Fisher Scientific). The RNA was treated with DNaseI (Invitrogen; Carlsbad, CA) according to the manufacturer's instructions.
miRNA analysis of OF-sEVs and OECs were performed as described in Da Silveira et al. (55). Briefly, for cDNA synthesis, reverse transcription was performed with the commercial miSCRIPT II RT kit (QIAGEN), using the MiScript HiFlex Buffer and 200 ng of total RNA. For quantitative RT-PCR, the miScript SYBR® Green PCR Kit (QIAGEN) was used according to the manufacturer's instructions. The relative levels of 383 miRNAs were evaluated for each sample. Three hundred eighty four-well plates were assembled using a multichannel electronic pipetting system and analyzed by RT-PCR with QuantStudio 6 Flex (Applied Biosystems). miRNAs were considered present when cycle threshold (CT) was <37 in at least three out of six biological repetitions with an adequate melting curve. For both OF-sEVs and OECs, the CT values were normalized using the geometric mean CT of the endogenous genes Hm/Ms/Rt U1 snRNA and bta-miR-99b (57). The relative expression values were calculated using the ΔCt method, and the normalized data were transformed by 2−ΔCt for graphical representation of the relative transcript levels (58).
Regarding mRNAs analysis of OECs, we evaluated the relative expression of 14 mRNAs selected from key pathways known to influence oviductal function and two endogenous controls (Table 1) for each sample. Briefly, reverse transcription reaction was performed with 30 ng of total RNA per gene using High-Capacity cDNA Reverse Transcription Kit (Thermo Fisher Scientific), according to the manufacturer's protocol. For quantitative RT-PCR, the GoTaq® qPCR Master Mix (Promega) was used according to the manufacturer's instructions and using the QuantStudio 6 Flex (Applied Biosystems) with the following PCR cycle conditions: 95°C for 10 min, 45 cycles of 95°C for 15 s, and 60°C for 60 s. The amplification of single cDNA products was confirmed by melt curve analysis. CT values were normalized using the geometric mean CT of the endogenous genes PPIA and GAPDH, as previously described for similar tissues (50). The relative expression values were calculated using the ΔCt method, and the normalized data were transformed by 2−ΔCt for graphical representation of the relative transcript levels (58).
Statistical and Bioinformatics Analysis
The data obtained were tested for outliers' presence, normality by Shapiro–Wilk test, and the normalized RT-PCR data were analyzed by the Student's t-test. For all analyzes, p < 0.05 was considered significant unless otherwise stated. Statistical analyzes were performed with JMP 14 (SAS Institute Inc.). For bioinformatics analysis and identification of biological pathways, the miRNAs differently detected within the groups were evaluated by miRWalk 2.0 bovine database (65). MiRWalk is an online database that, through their TarPmiR algorithm, allowed the searching of validated and predicted interactions between miRNAs and gene sequences. Then, the biological pathways predicted as modulated by these miRNAs of interest were identified through the gene set enrichment analysis (GSEA) function within the MiRWalk platform, assessing the geneset “KEGG Pathways.” Only pathways with a p-value lower than 0.05 were considered as significant.
Results
Sample Collection and Groups Formation
Embryos were found in six out of the 14 cows in FTAI group, and these samples formed the Pregnant group (n = 6). Six out of the 11 sham-inseminated cows that followed the analyzed variables described in Table 2 formed the Non-Pregnant group (n = 6). No differences were found on diameter POF size, weight, and diameter of the CL as well as serum concentration of P4 and E2 between the two groups, ensuring that these variables did not interfere with our results.
Isolation of OF-sEVs
Initially, the efficiency of the sEVs isolation protocol was confirmed using OF-sEVs obtained as described in the Supplementary Data 1. TEM was used to analyze the morphology and size of the isolated OF-sEVs. Throughout the images, we were able to identify cup-shaped particles with characteristic sizes resembling sEVs (Supplementary Figure 1). Therefore, the isolation protocol was adequate for the isolation of OF-sEVs.
Nanoparticle Tracking Analysis
In order to analyze particle concentration and mode size of sEVs, we performed NTA using OF-sEVs isolated from non-pregnant and pregnant cows. No differences were identified in mean particle concentration (OF-sEV/Non-pregnant: 4.3 × 109 ± 2.3 × 109 particles/mL; OF-sEV/Pregnant: 3.5 × 109 ± 1.2 × 109 particles/mL; Figure 1A) or mean mode size (OF-sEV/Non-pregnant: 140.7 ± 10.1 nm; OF-sEV/Pregnant: 135.4 ± 11.6 nm; Figure 1B) between the groups. From this result, we concluded that the pregnancy does not affect concentration and size of sEVs in the oviductal flushing.
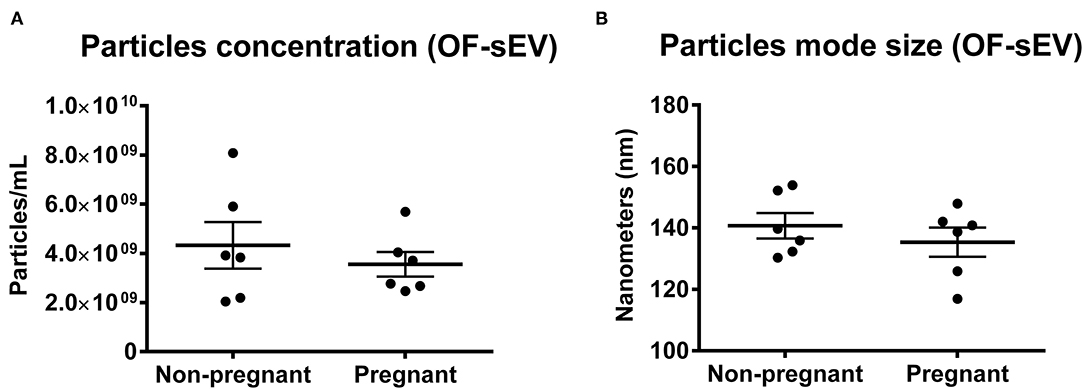
Figure 1. Characterization of OF-sEVs by nanoparticle analysis. Nanoparticle tracking analysis showing no difference in particles concentration (A) and mode size (B) between the groups OF-sEV/Non-pregnant (n = 6) and OF-sEV/Pregnant (n = 6). Error bars represents standard errors of the means.
miRNA Profile of OF-sEVs and Bioinformatics Analyses
To analyze the miRNAs profile, the relative expression levels of 383 miRNAs were investigated in OF-sEVs from six oviducts from pregnant and six oviducts non-pregnant cows. The presence of 248 and 205 miRNAs were identified in OF-sEVs isolated from the non-pregnant and pregnant group, respectively. Of these, 192 were commonly detected between the two groups, 56 were exclusive to OF-sEV/Non-pregnant, and 13 were exclusive to OF-sEV/Pregnant (for CT levels, see Supplementary Table 1). Among the 192 miRNAs detected in both groups, eight miRNAs (bta-miR-126-5p, bta-miR-129, bta-miR-140, bta-miR-188, bta-miR-219, bta-miR-345-3p, bta-miR-4523, and bta-miR-760-3p) were up-regulated in OF-sEV/Pregnant and one miRNA (bta-miR-331-5p) was up-regulated in OF-sEV/Non-pregnant (p < 0.05; Figure 2).
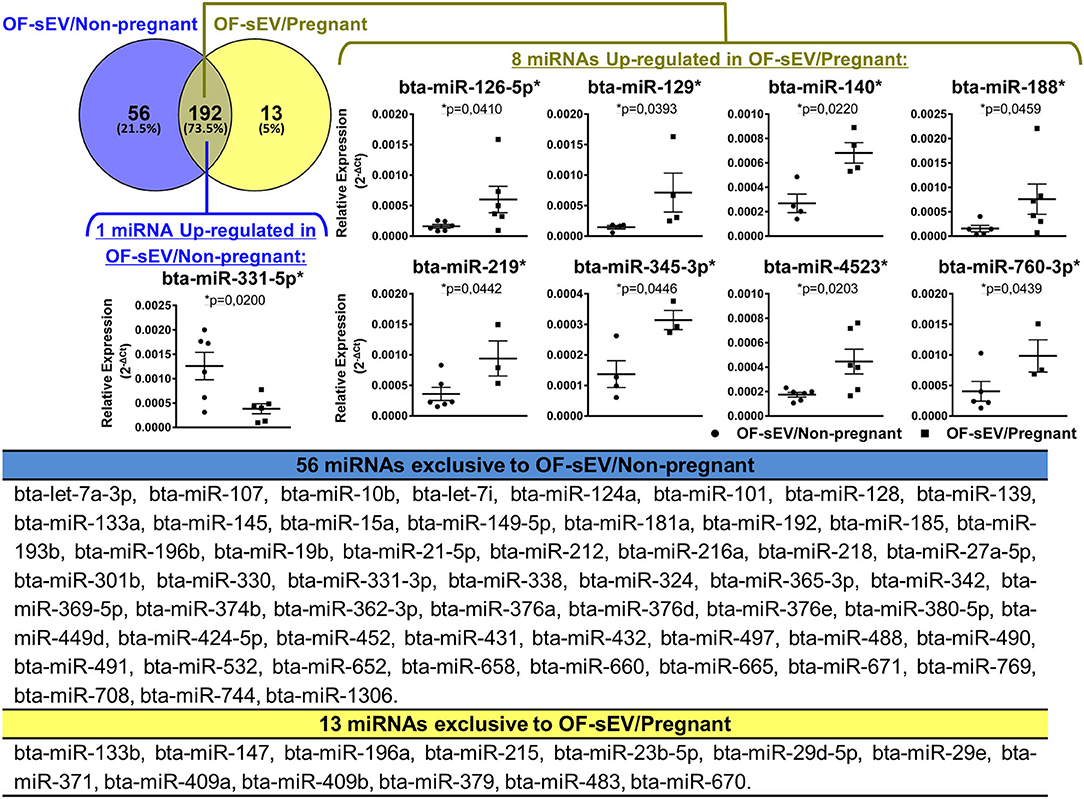
Figure 2. miRNAs profile of OF-sEVs isolated from non-pregnant and pregnant cows. Venn diagram representing the 261 miRNAs detected: 192 in common between the two groups, 56 exclusive to OF-sEV/Non-pregnant and 13 exclusive to OF-sEV/Pregnant. Among the 192 miRNAs detected in both groups, eight miRNAs (bta-miR-126-5p, bta-miR-129, bta-miR-140, bta-miR-188, bta-miR-219, bta-miR-345-3p, bta-miR-4523, and bta-miR-760-3p) were up-regulated in OF-sEV/Pregnant and one miRNA (bta-miR-331-5p) was up-regulated in OF-sEV/Non-pregnant. The asterisk (*) indicates miRNAs with relative expression significantly different (p < 0.05) within the groups. Samples size were n = 6 for both groups. Error bars represent SEM.
To investigate the biological functions of miRNAs differently expressed in OF-sEVs, we performed bioinformatics analysis. All the analyses were performed with up-regulated miRNAs. Based on their potential relevance for the oviductal milieu, 21 pathways were predicted to be modulated by the miRNA up-regulated in OF-sEVs from the non-pregnant group (Figure 3A), and 41 pathways predicted to be modulated by the miRNAs up-regulated in OF-sEVs from the pregnant group (Figure 3B) were selected. Our results demonstrated that differentially expressed OF-sEVs miRNAs modulate different pathways, such as PI3K-Akt signaling pathway, MAPK signaling pathway, and mTOR signaling pathway. For all identified pathways (p < 0.05), see Supplementary Tables 2, 3.
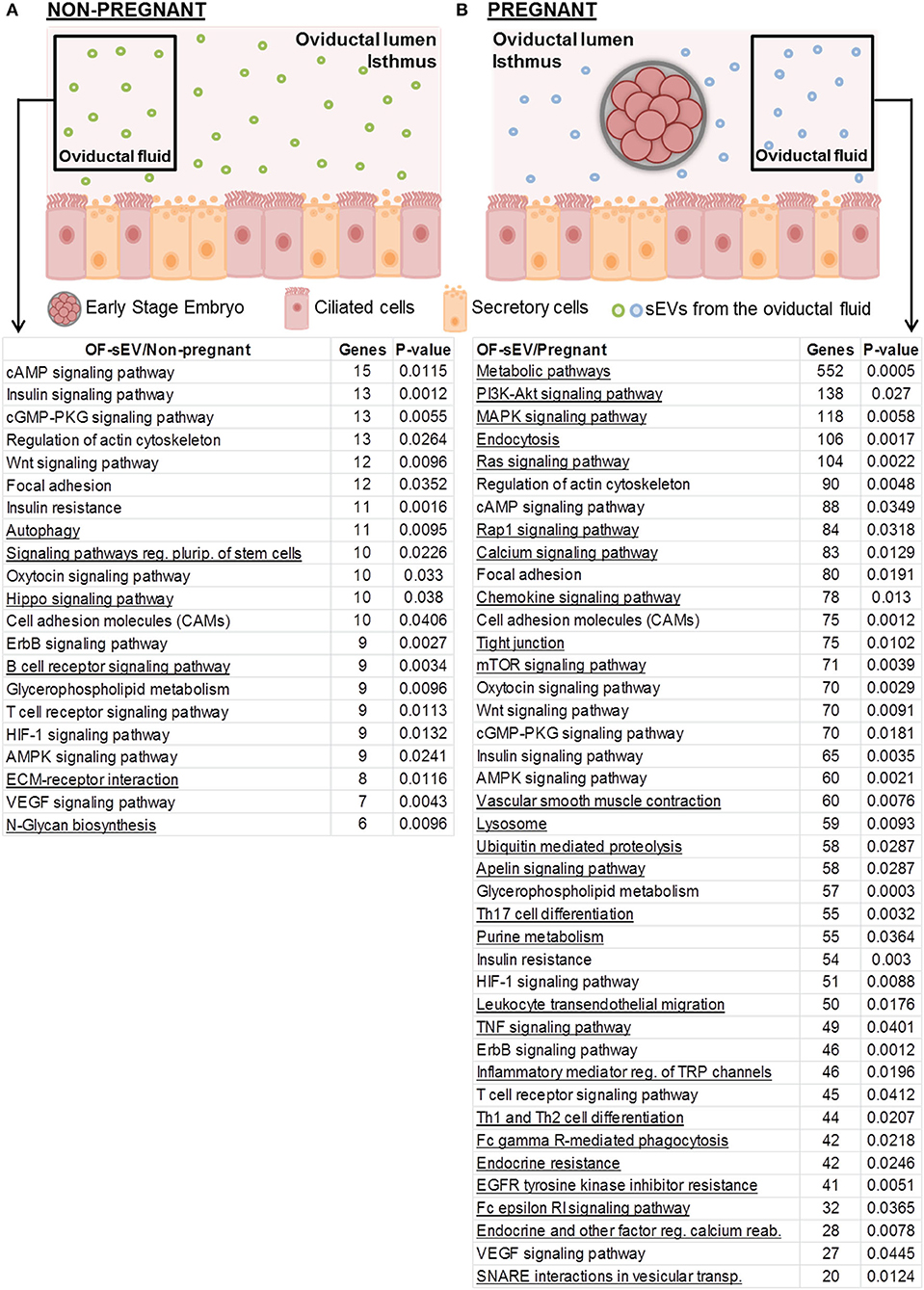
Figure 3. Selected biological pathways predicted to be regulated by miRNAs with increased expression in OF-sEVs. (A) Pathways predicted as regulated by the miRNA (bta-miR-331-5p) up-regulated in OF-sEV/Non-pregnant. (B) Pathways predicted as regulated by the eight miRNAs (bta-miR-126-5p, bta-miR-129, bta-miR-140, bta-miR-188, bta-miR-219, bta-miR-345-3p, bta-miR-4523, and bta-miR-760-3p) up-regulated in OF-sEV/Pregnant. Pathways were selected based on the potential relevance for oviductal functions and p < 0.05. Underlined pathways are modulated only in the group that they are present.
miRNA Contents of OECs and Bioinformatics Analyses
Next, we evaluated the levels of 383 miRNAs in OECs from the non-pregnant group (OEC/Non-pregnant) and the pregnant group (OEC/Pregnant). We identified a total of 355 and 353 miRNAs in OEC/Non-pregnant and OEC/Pregnant, respectively (for CT levels, see Supplementary Table 4). Of these, 345 miRNAs were commonly detected between the two groups, 10 were exclusive to OEC/Non-pregnant, and eight were exclusive to OEC/Pregnant. Among the 345 miRNAs detected in both groups, six miRNAs (bta-miR-133b, bta-miR-205, bta-miR-584, bta-miR-551a, bta-miR-1193, and bta-miR-1225-3p) were up-regulated in OEC/Non-pregnant, and none was up-regulated in OEC/Pregnant group and (p < 0.05; Figure 4).
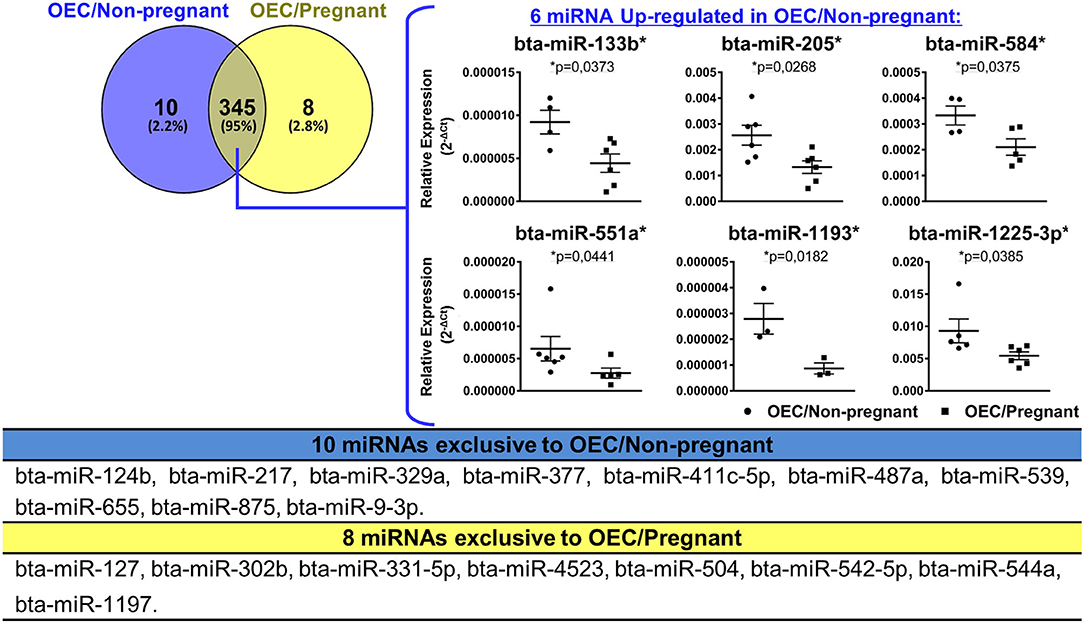
Figure 4. miRNAs profile of OECs from non-pregnant and pregnant cows. Venn diagram representing the detection of 363 miRNAs: 345 common between the two groups, 10 exclusive to OEC/Non-pregnant and eight exclusive to OEC/Pregnant. Among the 345 miRNAs detected in both groups, six miRNAs (bta-miR-133b, bta-miR-205, bta-miR-584, bta-miR-551a, bta-miR-1193, and bta-miR-1225-3p) were up-regulated in OEC/Non-pregnant. The asterisk (*) indicates miRNAs with relative expression significantly different (p < 0.05) within the groups. Samples size were n = 6 for both groups. Error bars represent SEM.
The prediction of biological functions modulated by miRNAs differently detected in OECs demonstrated 37 selected pathways predicted as regulated by six miRNAs (bta-miR-133b, bta-miR-205, bta-miR-584, bta-miR-551a, bta-miR-1193, and bta-miR-1225-3p) up-regulated in OECs from non-pregnant compared with pregnant cows (Figure 5). Our results suggest that differentially expressed OECs miRNAs modulate pathways, such as chemokine, oxytocin, and TNF signaling pathways. For all identified pathways (p < 0.05), see Supplementary Table 5.
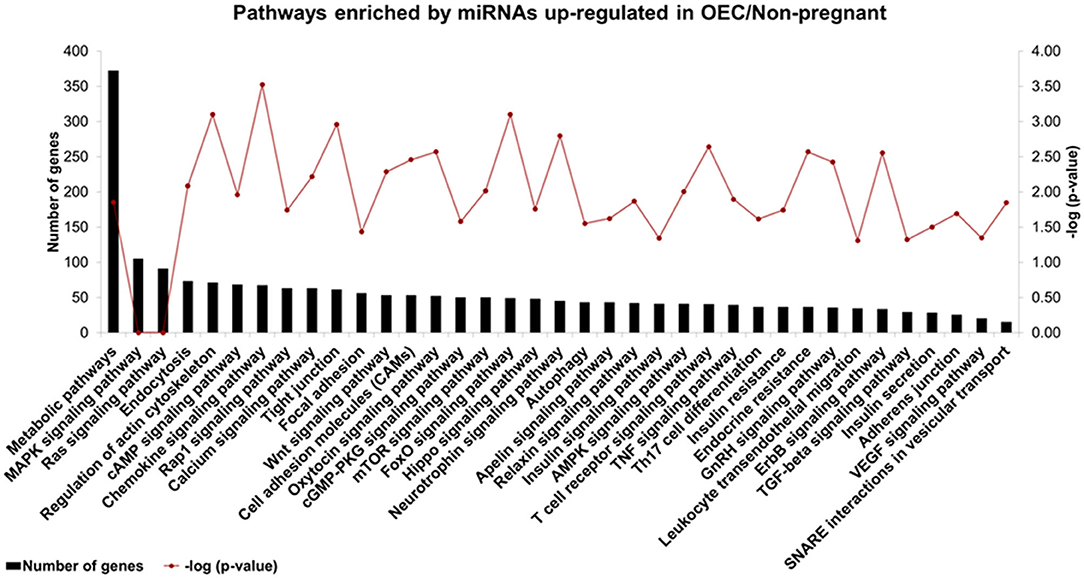
Figure 5. Biological pathways predicted using up-regulated miRNAs in OECs. Pathways predicted as regulated by six miRNAs (bta-miR-133b, bta-miR-205, bta-miR-584, bta-miR-551a, bta-miR-1193, and bta-miR-1225-3p) up-regulated in OECs from non-pregnant compared with OECs from pregnant cows. The left Y-axis values represent the number of genes predicted as modulated by the miRNAs for the respective pathways. The right Y-axis represents the enrichment score (–log10 of the p-value) for each pathway.
Relative Transcripts Levels for Selected Genes
To evaluate genes present in predicted pathways regulated by miRNAs differently expressed in OECs, we analyzed the relative levels of 14 mRNAs selected from inflammatory, immune response, and pregnancy recognition pathways, known to influence oviductal function during early pregnancy. Considering a significant difference of p < 0.1, the genes TGFB1 (p = 0.029), TNFR1 (p = 0.096), and PTGES2 (p = 0.068) were up-regulated in OECs from the pregnant group (Figure 6). Thus, our results demonstrate that the presence of one single embryo can affect the gene expression of those pathways in OECs.
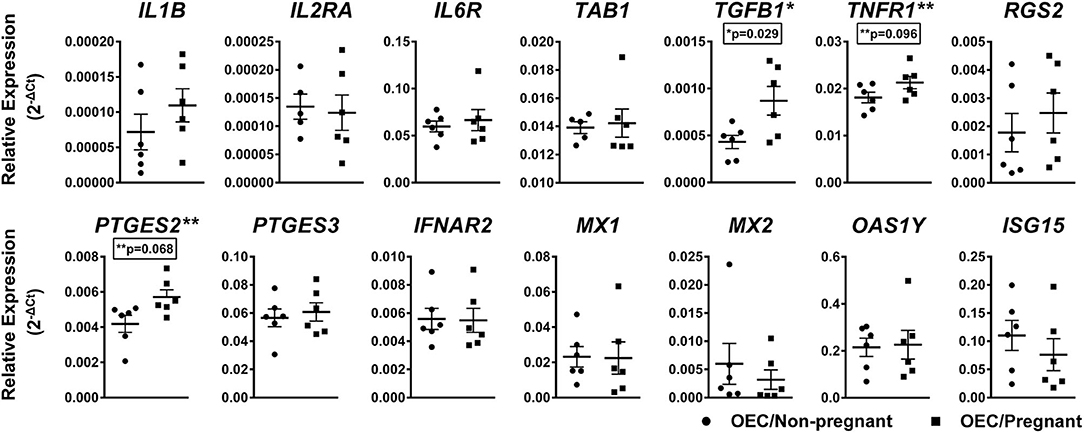
Figure 6. Relative mRNA expression of selected genes in OECs from non-pregnant compared with pregnant cows. Relative expression of up and downregulated mRNAs are shown as mean of 2−ΔCt ± SEM. One asterisk (*) indicates genes with p < 0.05 and two asterisks (**) indicates genes with p < 0.1 within the groups. Samples size were n = 6 for both groups.
Discussion
Here we identified the miRNA contents of OF-sEVs and OECs as well as selected genes in OECs from pregnant and non-pregnant cows. This study indicates that communication within the pregnant animals, mediated by sEVs, is potentially initiated in the oviduct and, although the passage of spermatozoa and presence of a single embryo does not interfere with the OF-sEVs concentration, it modulates the miRNAs' contents in both OF-sEVs and OECs, as well as the transcript levels of the selected genes TGFB1, TNFR1, and PTGES2 in OECs.
Oviductal flushing samples from the isthmus of ipsilateral oviducts were collected and used for sEVs isolation. The validation of the OF-sEVs isolation was based on the Minimal information for studies of extracellular vesicles 2018 [MISEV 2018; (28)]. No differences were identified in mode size or mean particle concentration between the groups; additionally, our results showed a population of vesicles with the expected size for sEVs, <200 nm (23). For instance, the passage of the spermatozoa and embryo presence do not induce changes in the oviductal secretion of sEVs, and the amount of sEVs secreted by the embryo were not enough to cause a detectable increase in the total amount of sEVs. Although there is no influence on the concentration of sEVs in pregnant cows, we were able to identify miRNAs with distinct levels between the pregnant and non-pregnant groups in both OF-sEVs and OECs.
In OF-sEVs, we identified eight miRNAs up-regulated in pregnant and one miRNA up-regulated in non-pregnant cows. The presence of EVs in the bovine oviductal fluid and their functional effects on the embryo are already recognized (30, 31, 66). miRNAs from oviductal EVs have been studied in bovines and murine across the estrous cycle, and in both models, it was showed a hormonal effect on the miRNAs in cyclic animals (32, 67). We showed that the passage of the spermatozoa and embryo presence could induce differential secretion of miRNAs in OF-sEVs. Among the up-regulated miRNAs in the pregnant group, the bta-miR-140 was also detected across the bovine estrous cycle, without differences among the stages (32). In the same study, the miRNAs bta-miR-129, bta-miR-188, bta-miR-219, bta-miR-345-3p, bta-miR-4523, bta-miR-760-3p identified in our study as up-regulated in pregnant cows were not detected across the estrous cycle. Similarly, the bta-miR-331-5p, up-regulated in our study in the non-pregnant group, was not detected across the estrus cycle (32). Thus, these differences in miRNA contents could be due to differences in the isolation protocol and mainly in the technique used for miRNAs analysis and not necessarily that these miRNAs originated from the embryo itself since they are also present in low levels in the non-pregnant cows.
Differentially expressed miRNAs in OF-sEVs modulate pathways, such as PI3K/AKT, mTOR, and MAPK, which are predicted to be modulated by up-regulated miRNAs from OF-sEVs from the pregnant group. These pathways are related to transcription, translation, proliferation, growth, control of the cytoskeletal organization, metabolism, and survival (68–72). Previous studies have demonstrated that PI3K/mTOR signaling pathways are related to angiogenesis, a crucial process in the female's reproductive system and essential for pregnancy establishment (73, 74). Additionally, PI3K/AKT pathway can mediate cell growth and survival in pre-implantation embryos. In early mouse embryos, PI3K-Akt subunits are expressed from zygote to blastocyst stage and are involved with blastomere proliferation (75, 76). In bovine pre-implantation embryos, the activation PI3K signaling is also required for apoptosis control (71). Together, these studies demonstrate that the presence and function of the PI3K/AKT/mTOR pathway are essential for embryo development. We suggest that these miRNAs within sEVs could influence embryo development in the oviduct, mediating maternal-embryonic communication during early embryo development. In order to test that, in future studies we aim to utilize oviductal sEVs collected from oviductal fluid from the pregnant group as a tool to modulate the embryo environment in vitro, as well as to study the effects of those sEVs on embryo quality and development.
In OECs, six miRNAs were up-regulated in the non-pregnant group (bta-miR-133b, bta-miR-205, bta-miR-584, bta-miR-551a, bta-miR-1193, and bta-miR-1225-3p) and none in the pregnant group. It is well-known that sex-steroid hormones, E2 and P4, coordinate changes in the oviductal transcriptome and secretory function across the estrous cycle (6, 77–79). Also, considering day 4 of the estrous cycle, the POF and CL size potentially affect the oviductal environment (50). In our results, no differences were found in POF and CL size as well as no differences in E2 and P4 between our groups, ensuring that they did not interfere with our results. The miRNAs bta-miR-133b, bta-miR-205, bta-miR-584, bta-miR-551a, identified in our study as up-regulated in non-pregnant cows, were already detected on bovine OECs exposed to low and high E2/P4 levels without the presence of the embryo, but with no differences in transcript levels (56). Thus, these miRNAs are not modulated by steroid hormones, supporting our data indicating that the passage of the spermatozoa and embryo presence can induce changes in miRNAs within oviductal cells in the same stage of the estrous cycle. Taken together, our results suggest that the passage of the spermatozoa and presence of an embryo within the oviduct can modulate the oviductal microenvironment, specifically the contents of miRNAs in OF-sEVs and OECs.
Based on bioinformatics analysis, we decided to evaluate the modulation of key-genes transcripts involved in the immune system, inflammation, and pregnancy recognition on OECs. Our study suggests that pregnancy stimulates changes in TGFB1, PTGES2, and TNFR1 levels. TGFB1 is an anti-inflammatory cytokine present in OECs throughout the estrous cycle and crucial for tolerance to non-self-cells (80). Increased expression level of TGFB1, as found in our pregnant group, is also present in bovine OECs cultured with embryos in vitro (81). However, it cannot be disregarded that the increase in TGFB1 levels is also related to sperm binding and induction of sperm-tolerance (80, 82). Synergistically with TGFB1, the PTGES2, up-regulated in our pregnant group, participates in the eicosanoid metabolic process that will drive the production of PGE2 (83), promoting immune tolerance in the bovine oviduct (81, 84). Talukder et al. (81) and Maillo et al. (13) suggested that the embryo generates an anti-inflammatory response by suppressing key factors, such as NFkB2 and NFkBIA. The activation of NFkB transcriptional responses can be triggered by TNFR1 (85). In our results, TNFR1 was increased in OECs from the pregnant cows. The TNFR1 is the receptor for the pro-inflammatory cytokines TNF-α. However, this cytokine also stimulates the productions of contractive substances, such as prostaglandins, regulating gametes transport, and ensuring the embryo migration into the uterus (86). Thus, it is imperative the adequate modulation of TNFR1, and among the modulators of the inflammatory responses of mammals are miRNAs (87). We identified a classic relationship between miR-584 and its predicted target TNFR1 in OECs. The bta-miR-584 was down-regulated in OECs from the pregnant group, while TNFR1 is increased in this group, while the opposite was observed in the non-pregnant group. Our results suggest that miRNAs may be decisive for the modulation of pro- and anti-inflammatory cytokines, leading to a proper immune response. Regarding genes involved in pregnancy recognition, no differences were identified between OECs groups. These results can be explained as the OECs from the pregnant group were exposed to embryos between 4 and 16 cells stage, and not morulas our blastocysts. Thereby, the interferon tau mechanism might not be initiated during this stage. Similar results were also found in vitro, where the presence of day-04 embryo did not influence the expression of interferon-stimulated genes (such as ISG15, OAS1, MX2) and IFNAR2 on bovine OECs (81). Taken together, pregnancy can dynamically modulate the immune system and inflammation response as early as day 4, affecting the oviductal environment and possibly embryo survival and development.
In this study, we identify the modulation of miRNAs and mRNAs in the oviduct. However, some limitations should be noticed since our experimental model cannot determine if differently expressed miRNAs are a direct or indirect effect of the spermatozoa or the embryo. Nevertheless, previous studies have shown that the embryo has a local effect on both, the oviduct and the endometrium (13, 62, 88). Additionally, the miRNAs up-regulated in OF-sEVs from the pregnant group are potentially secreted by the embryo, by the oviduct as a result of the embryonic stimulus, or from both. Although the ideal model to isolate the embryo effect is to transfer embryos directly into the oviduct (13), we must not disregard that the physiological environment is dynamic and depends on a set of synchronous factors and signals that together will guarantee the success of the pregnancy (89). Therefore, we chose not to remove these factors or to amplify the embryo's signal by transferring a large number of embryos as it would not represent the physiological response of the oviductal milieu. Additionally, future approaches should consider increase the number of animals and also small RNA sequencing analysis for a more comprehensive understanding of the expression and regulation of miRNAs associated with pregnancy in OECs and sEVs.
In conclusion, our results demonstrate that pregnancy can modulate the miRNA contents of sEVs as well as the miRNAs and mRNAs levels in OECs in cows. The functional enrichment of miRNAs highlights pathways involved in physiological processes, such as inflammation, cell proliferation, and immune response, crucial for both the reproductive tract and embryo development. Furthermore, our results confirm in vivo that the embryo-maternal crosstalk starts within the oviduct, enhancing oviductal receptivity, allowing proper fertilization and early embryo development, thus leading to a successful pregnancy.
Data Availability Statement
The original contributions presented in the study are included in the article/Supplementary Material, further inquiries can be directed to the corresponding author/s.
Ethics Statement
The animal study was reviewed and approved by University of São Paulo Research Ethics Committee (Protocol Number 4909010817).
Author Contributions
RM and JS designed the study. RM, NB, AB, MC, GA, and CP collected the samples and performed the experiments. RM, FP, and JS analyzed the data and wrote the manuscript. JP and GP performed the ultrasonography and artificial insemination. LS provided the conditions for cattle housing and management. FM and GP contributed the expertise and reagents. All authors discussed, reviewed, and agreed to the final version of the manuscript.
Funding
This research was funded by São Paulo Research Foundation (FAPESP), grants number 2014/22887-0, 2015/21829-9 (JS) and 2019/04981-2 (RM), by National Council for Scientific and Technological Development (CNPq), grants number 420152/2018-0 (JS) and 306349/2017-5 (FP), and by Coordination for the Improvement of Higher Education Personnel—Brazil (CAPES—Finance code 001).
Conflict of Interest
The authors declare that the research was conducted in the absence of any commercial or financial relationships that could be construed as a potential conflict of interest.
Acknowledgments
The authors would like to thank staff and students from the Molecular Morphology and Development Laboratory (LMMD-FZEA/USP), the Molecular Endocrinology Physiology Laboratory (LFEM-FMVZ/USP), and the Multiuser Laboratory of Electron Microscopy (LMME-FMRP/USP).
Supplementary Material
The Supplementary Material for this article can be found online at: https://www.frontiersin.org/articles/10.3389/fvets.2021.639752/full#supplementary-material
References
1. Besenfelder U, Havlicek V, Brem G. Role of the oviduct in early embryo development. Reprod Domest Anim. (2012) 47:156–63. doi: 10.1111/j.1439-0531.2012.02070.x
2. Avilés M, Coy P, Rizos D. The oviduct: a key organ for the success of early reproductive events. Anim Front. (2015) 5:25–31. doi: 10.2527/af.2015-0005
3. Kölle S, Dubielzig S, Reese S, Wehrend A, König P, Kummer W. Ciliary transport, gamete interaction, and effects of the early embryo in the oviduct: ex vivo analyses using a new digital videomicroscopic system in the cow. Biol Reprod. (2009) 81:267–74. doi: 10.1095/biolreprod.108.073874
4. Leese HJ, Hugentobler SA, Gray SM, Morris DG, Sturmey RG, Whitear SL, et al. Female reproductive tract fluids: composition, mechanism of formation and potential role in the developmental origins of health and disease. Reprod Fertil Dev. (2008) 20:1–8. doi: 10.1071/RD07153
5. Avilés M, Gutiérrez-Adán A, Coy P. Oviductal secretions: will they be key factors for the future ARTs? Mol Hum Reprod. (2010) 16:896–906. doi: 10.1093/molehr/gaq056
6. Bauersachs S, Blum H, Mallok S, Wenigerkind H, Rief S, Prelle K, et al. Regulation of ipsilateral and contralateral bovine oviduct epithelial cell function in the postovulation period: a transcriptomics approach. Biol Reprod. (2003) 68:1170–7. doi: 10.1095/biolreprod.102.010660
7. Ulbrich SE, Kettler A, Einspanier R. Expression and localization of estrogen receptor α, estrogen receptor β and progesterone receptor in the bovine oviduct in vivo and in vitro. J Steroid Biochem Mol Biol. (2003) 84:279–89. doi: 10.1016/S0960-0760(03)00039-6
8. Rodríguez-Alonso B, Maillo V, Acuña OS, López-úbeda R, Torrecillas A, Simintiras CA, et al. Spatial and pregnancy-related changes in the protein, amino acid, and carbohydrate composition of bovine oviduct fluid. Int J Mol Sci. (2020) 21:1681. doi: 10.3390/ijms21051681
9. Buhi WC. Characterization and biological roles of oviduct-specific, oestrogen-dependent glycoprotein. Reproduction. (2002) 123:355–62. doi: 10.1530/rep.0.1230355
10. Binelli M, Gonella-Diaza A, Mesquita F, Membrive C. Sex steroid-mediated control of oviductal function in cattle. Biology (Basel). (2018) 7:15. doi: 10.3390/biology7010015
11. Schmaltz-Panneau B, Cordova A, Dhorne-Pollet S, Hennequet-Antier C, Uzbekova S, Martinot E, et al. Early bovine embryos regulate oviduct epithelial cell gene expression during in vitro co-culture. Anim Reprod Sci. (2014) 149:103–16. doi: 10.1016/j.anireprosci.2014.06.022
12. Maillo V, Sánchez-Calabuig MJ, Lopera-Vasquez R, Hamdi M, Gutierrez-Adan A, Lonergan P, et al. Oviductal response to gametes and early embryos in mammals. Reproduction. (2016) 152:R127–41. doi: 10.1530/REP-16-0120
13. Maillo V, Gaora PÓ, Forde N, Besenfelder U, Havlicek V, Burns GW, et al. Oviduct-embryo interactions in cattle: two-way traffic or a one-way street?. Biol Reprod. (2015) 92:1–8. doi: 10.1095/biolreprod.115.127969
14. Rodríguez-Alonso B, Hamdi M, Sánchez JM, Maillo V, Gutierrez-Adan A, Lonergan P, et al. An approach to study the local embryo effect on gene expression in the bovine oviduct epithelium in vivo. Reprod Domest Anim. (2019) 54:1516–23. doi: 10.1111/rda.13558
15. Georgiou AS, Sostaric E, Wong CH, Snijders APL, Wright PC, Moore HD, et al. Gametes alter the oviductal secretory proteome. Mol Cell Proteomics. (2005) 4:1785–96. doi: 10.1074/mcp.M500119-MCP200
16. Rodríguez-Alonso B, Sánchez JM, González E, Lonergan P, Rizos D. Challenges in studying preimplantation embryo-maternal interaction in cattle. Theriogenology. (2020) 150:139–49. doi: 10.1016/j.theriogenology.2020.01.019
17. Raposo G, Stoorvogel W. Extracellular vesicles: exosomes, microvesicles, and friends. J Cell Biol. (2013) 200:373–83. doi: 10.1083/jcb.201211138
18. Simpson RJ, Jensen SS, Lim JWE. Proteomic profiling of exosomes: current perspectives. Proteomics. (2008) 8:4083–99. doi: 10.1002/pmic.200800109
19. Subra C, Laulagnier K, Perret B, Record M. Exosome lipidomics unravels lipid sorting at the level of multivesicular bodies. Biochimie. (2007) 89:205–12. doi: 10.1016/j.biochi.2006.10.014
20. Jeppesen DK, Fenix AM, Franklin JL, Higginbotham JN, Zhang Q, Zimmerman LJ, et al. Reassessment of exosome composition. Cell. (2019) 177:428–45.e18. doi: 10.1016/j.cell.2019.02.029
21. Valadi H, Ekström K, Bossios A, Sjöstrand M, Lee JJ, Lötvall JO. Exosome-mediated transfer of mRNAs and microRNAs is a novel mechanism of genetic exchange between cells. Nat Cell Biol. (2007) 9:654–9. doi: 10.1038/ncb1596
22. György B, Szabó TG, Pásztói M, Pál Z, Misják P, Aradi B, et al. Membrane vesicles, current state-of-the-art: emerging role of extracellular vesicles. Cell Mol Life Sci. (2011) 68:2667–88. doi: 10.1007/s00018-011-0689-3
23. Théry C, Witwer KW, Aikawa E, Alcaraz MJ, Anderson JD, Andriantsitohaina R, et al. Minimal information for studies of extracellular vesicles 2018 (MISEV2018): a position statement of the International Society for Extracellular Vesicles and update of the MISEV2014 guidelines. J Extracell Vesicles. (2018) 7:1535750. doi: 10.1080/20013078.2018.1535750
24. Almiñana C, Bauersachs S. Extracellular vesicles in the oviduct: progress, challenges and implications for the reproductive success. Bioengineering. (2019) 6:32. doi: 10.3390/bioengineering6020032
25. Saadeldin, Islam M, Hyun Ju Oh BCL. Embryonic–maternal cross-talk via exosomes: potential implications. Stem Cells Cloning Adv Appl. (2015) 8:103–7. doi: 10.2147/SCCAA.S84991
26. Al-Dossary AA, Martin-Deleon PA. Role of exosomes in the reproductive tract Oviductosomes mediate interactions of oviductal secretion with gametes/early embryo. Front Biosci (Landmark Ed). (2016) 21:1278–85. doi: 10.2741/4456
27. Pavani KC, Alminana C, Wydooghe E, Catteeuw M, Ramírez MA, Mermillod P, et al. Emerging role of extracellular vesicles in communication of preimplantation embryos in vitro. Reprod Fertil Dev. (2017) 29:66–83. doi: 10.1071/RD16318
28. Qamar AY, Mahiddine FY, Bang S, Fang X, Shin ST, Kim MJ, et al. Extracellular vesicle mediated crosstalk between the gametes, conceptus, and female reproductive tract. Front Vet Sci. (2020) 7:589117. doi: 10.3389/fvets.2020.589117
29. Lopera-Vasquez R, Hamdi M, Maillo V, Lloreda V, Coy P, Gutierrez-Adan A, et al. Effect of bovine oviductal fluid on development and quality of bovine embryos produced in vitro. Reprod Fertil Dev. (2017) 29:621–9. doi: 10.1071/RD15238
30. Lopera-Vasquez R, Hamdi M, Fernandez-Fuertes B, Maillo V, Beltran-Brena P, Calle A, et al. Extracellular vesicles from BOEC in in vitro embryo development and quality. PLoS ONE. (2016) 11:e0148083. doi: 10.1371/journal.pone.0148083
31. Almiñana C, Corbin E, Tsikis G, Alcântara-Neto AS, Labas V, Reynaud K, et al. Oviduct extracellular vesicles protein content and their role during oviduct–embryo cross-talk. Reproduction. (2017) 154:253–68. doi: 10.1530/REP-17-0054
32. Almiñana C, Tsikis G, Labas V, Uzbekov R, da Silveira JC, Bauersachs S, et al. Deciphering the oviductal extracellular vesicles content across the estrous cycle: Implications for the gametes-oviduct interactions and the environment of the potential embryo. BMC Genomics. (2018) 19:1–27. doi: 10.1186/s12864-018-4982-5
33. Ha M, Kim VN. Regulation of microRNA biogenesis. Nat Rev Mol Cell Biol. (2014) 15:509–24. doi: 10.1038/nrm3838
34. Lau NC. An abundant class of tiny RNAs with probable regulatory roles in Caenorhabditis elegans. Science. (2001) 294:858–62. doi: 10.1126/science.1065062
35. Lee Y. MicroRNA maturation: stepwise processing and subcellular localization. EMBO J. (2002) 21:4663–70. doi: 10.1093/emboj/cdf476
36. Guo H, Ingolia NT, Weissman JS, Bartel DP. Mammalian microRNAs predominantly act to decrease target mRNA levels. Nature. (2010) 466:835–40. doi: 10.1038/nature09267
37. Kotaja N. MicroRNAs and spermatogenesis. Fertil Steril. (2014) 101:1552–62. doi: 10.1016/j.fertnstert.2014.04.025
38. Tesfaye D, Gebremedhn S, Salilew-Wondim D, Hailay T, Hoelker M, Grosse-Brinkhaus C, et al. MicroRNAs: tiny molecules with a significant role in mammalian follicular and oocyte development. Reproduction. (2018) 155:R121–35. doi: 10.1530/REP-17-0428
39. Da Silveira JC, Winger QA, Bouma GJ, Carnevale EM. Effects of age on follicular fluid exosomal microRNAs and granulosa cell transforming growth factor-β signalling during follicle development in the mare. Reprod Fertil Dev. (2015) 27:897–905. doi: 10.1071/RD14452
40. Tesfaye D, Worku D, Rings F, Phatsara C, Tholen E, Schellander K, et al. Identification and expression profiling of microRNAs during bovine oocyte maturation using heterologous approach. Mol Reprod Dev. (2009) 76:665–77. doi: 10.1002/mrd.21005
41. Andrade GM, Da Silveira JC, Perrini C, Del Collado M, Gebremedhn S, Tesfaye D, et al. The role of the PI3K-Akt signaling pathway in the developmental competence of bovine oocytes. PLoS ONE. (2017) 12:e0185045. doi: 10.1371/journal.pone.0185045
42. Mondou E, Dufort I, Gohin M, Fournier E, Sirard MA. Analysis of micrornas and their precursors in bovine early embryonic development. Mol Hum Reprod. (2012) 18:425–34. doi: 10.1093/molehr/gas015
43. Kosaka N, Yoshioka Y, Hagiwara K, Tominaga N, Katsuda T, Ochiya T. Trash or treasure: extracellular microRNAs and cell-to-cell communication. Front Genet. (2013) 4:173. doi: 10.3389/fgene.2013.00173
44. Hwang H-W, Mendell JT. MicroRNAs in cell proliferation, cell death, and tumorigenesis. Br J Cancer. (2006) 94:776–80. doi: 10.1038/sj.bjc.6603023
45. Tesfaye D, Salilew-Wondim D, Gebremedhn S, Sohel MMH, Pandey HO, Hoelker M, et al. Potential role of microRNAs in mammalian female fertility. Reprod Fertil Dev. (2017) 29:8–23. doi: 10.1071/RD16266
46. Salilew-Wondim D, Gebremedhn S, Hoelker M, Tholen E, Hailay T, Tesfaye D. The role of MicroRNAs in mammalian fertility: from gametogenesis to embryo implantation. Int J Mol Sci. (2020) 21:585. doi: 10.3390/ijms21020585
47. Hossain MM, Salilew-Wondim D, Schellander K, Tesfaye D. The role of microRNAs in mammalian oocytes and embryos. Anim Reprod Sci. (2012) 134:36–44. doi: 10.1016/j.anireprosci.2012.08.009
48. Barkalina N, Jones C, Wood MJA, Coward K. Extracellular vesicle-mediated delivery of molecular compounds into gametes and embryos: learning from nature. Hum Reprod Update. (2015) 21:627–39. doi: 10.1093/humupd/dmv027
49. Ayres H, Ferreira RM, de Souza Torres-Júnior JR, Demétrio CGB, de Lima CG, Baruselli PS. Validation of body condition score as a predictor of subcutaneous fat in Nelore (Bos indicus) cows. Livest Sci. (2009) 123:175–9. doi: 10.1016/j.livsci.2008.11.004
50. Gonella-Diaza AM, da Silva Andrade SC, Sponchiado M, Pugliesi G, Mesquita FS, Van Hoeck V, et al. Size of the ovulatory follicle dictates spatial differences in the oviductal transcriptome in cattle. PLoS ONE. (2015) 10:e0145321. doi: 10.1371/journal.pone.0145321
51. Maillo V, De Frutos C, O'Gaora P, Forde N, Burns GW, Spencer TE, et al. Spatial differences in gene expression in the bovine oviduct. Reproduction. (2016) 152:37–46. doi: 10.1530/REP-16-0074
52. Gonella-Diaza AM, da Silva Andrade SC, Sponchiado M, Pugliesi G, Mesquita FS, Van Hoeck V, et al. Oviductal transcriptional profiling of a bovine fertility model by next-generation sequencing. Genomics Data. (2017) 13:27–9. doi: 10.1016/j.gdata.2017.06.004
53. da Silveira JC, Andrade GM, Collado M del, Sampaio RV, Sangalli JR, Silva LA, et al. Supplementation with small-extracellular vesicles from ovarian follicular fluid during in vitro production modulates bovine embryo development. PLoS ONE. (2017) 12:e0179451. doi: 10.1371/journal.pone.0179451
54. de Ávila ACFCM, Bridi A, Andrade GM, del Collado M, Sangalli JR, Nociti RP, et al. Estrous cycle impacts microRNA content in extracellular vesicles that modulate bovine cumulus cell transcripts during in vitro maturation. Biol Reprod. (2020) 102:362–75. doi: 10.1093/biolre/ioz177
55. Da Silveira J, Andrade GM, Perecin F, Meireles FV, Winger QA, Bouma GJ. Isolation and analysis of exosomal microRNAs from ovarian follicular fluid. Methods Mol Biol. (2018) 1733:53–63. doi: 10.1007/978-1-4939-7601-0_4
56. Gonella-Diaza AM, Lopes E, Ribeiro da Silva K, Perecin Nociti R, Mamede Andrade G, Atuesta-Bustos JE, et al. Steroidal regulation of oviductal microRNAs Is associated with microRNA-processing in beef cows. Int J Mol Sci. (2021) 22:953. doi: 10.3390/ijms22020953
57. Andrade GM, Bomfim MM, del Collado M, Meirelles FV, Perecin F, da Silveira JC. Oxygen tension modulates extracellular vesicles and its miRNA contents in bovine embryo culture medium. Mol Reprod Dev. (2019) 86:1067–80. doi: 10.1002/mrd.23223
58. Schmittgen TD, Livak KJ. Analyzing real-time PCR data by the comparative CT method. Nat Protoc. (2008) 3:1101–8. doi: 10.1038/nprot.2008.73
59. Lopes E. Effect of the periovulatory endocrine milieu on microRNAs expression and IL1/TLR systems in bovine endometrium (Dissertation thesis), University of São Paulo, Pirassununga, Brazil (2016).
60. Scolari SC, Pugliesi G, Strefezzi RDF, Andrade SCDS, Coutinho LL, Binelli M. Dynamic remodeling of endometrial extracellular matrix regulates embryo receptivity in cattle. Reproduction. (2017) 153:49–61. doi: 10.1530/REP-16-0237
61. Oliveira ML, D'Alexandri FL, Pugliesi G, Van Hoeck V, Mesquita FS, Membrive CMB, et al. Peri-ovulatory endocrine regulation of the prostanoid pathways in the bovine uterus at early dioestrus. Reprod Fertil Dev. (2017) 29:544–56. doi: 10.1071/RD15269
62. Sponchiado M, Gomes NS, Fontes PK, Martins T, del Collado M, Pastore ADA, et al. Pre-hatching embryo-dependent and -independent programming of endometrial function in cattle. PLoS ONE. (2017) 12:e0175954. doi: 10.1371/journal.pone.0175954
63. Gifford CA, Racicot K, Clark DS, Austin KJ, Hansen TR, Lucy MC, et al. Regulation of interferon-stimulated genes in peripheral blood leukocytes in pregnant and bred, nonpregnant dairy cows. J Dairy Sci. (2007) 90:274–80. doi: 10.3168/jds.S0022-0302(07)72628-0
64. Araújo ER, Sponchiado M, Pugliesi G, Van Hoeck V, Mesquita FS, Membrive CMB, et al. Spatio-specific regulation of endocrine-responsive gene transcription by periovulatory endocrine profiles in the bovine reproductive tract. Reprod Fertil Dev. (2016) 28:1533–44. doi: 10.1071/RD14178
65. Sticht C, De La Torre C, Parveen A, Gretz N. miRWalk: an online resource for prediction of microRNA binding sites. PLoS ONE. (2018) 13:e0206239. doi: 10.1371/journal.pone.0206239
66. Lopera-Vasquez R, Hamdi M, Maillo V, Gutierrez-Adan A, Bermejo-Alvarez P, Angel Ramirez M, et al. Effect of bovine oviductal extracellular vesicles on embryo development and quality in vitro. Reproduction. (2017) 153:461–70. doi: 10.1530/REP-16-0384
67. Fereshteh Z, Schmidt SA, Al-Dossary AA, Accerbi M, Arighi C, Cowart J, et al. Murine oviductosomes (OVS) microRNA profiling during the estrous cycle: delivery of OVS-borne microRNAs to sperm where miR-34c-5p localizes at the centrosome. Sci Rep. (2018) 8:16094. doi: 10.1038/s41598-018-34409-4
68. Yu JSL, Cui W. Proliferation, survival and metabolism: the role of PI3K/AKT/mTOR signalling in pluripotency and cell fate determination. Development. (2016) 143:3050–60. doi: 10.1242/dev.137075
69. Engelman JA, Luo J, Cantley LC. The evolution of phosphatidylinositol 3-kinases as regulators of growth and metabolism. Nat Rev Genet. (2006) 7:606–19. doi: 10.1038/nrg1879
70. Ersahin T, Tuncbag N, Cetin-Atalay R. The PI3K/AKT/mTOR interactive pathway. Mol Biosyst. (2015) 11:1946–54. doi: 10.1039/C5MB00101C
71. Jousan FD, Hansen PJ. Insulin-like growth factor-I promotes resistance of bovine preimplantation embryos to heat shock through actions independent of its anti-apoptotic actions requiring PI3K signaling. Mol Reprod Dev. (2007) 74:189–96. doi: 10.1002/mrd.20527
72. Hansen PJ, Denicol AC, Dobbs KB. Maternal embryokines that regulate development of the bovine preimplantation embryo. Turkish J Vet Anim Sci. (2014) 38:589–98. doi: 10.3906/vet-1405-96
73. Rizov M, Andreeva P, Dimova I. Molecular regulation and role of angiogenesis in reproduction. Taiwan J Obstet Gynecol. (2017) 56:127–32. doi: 10.1016/j.tjog.2016.06.019
74. Karar J, Maity A. PI3K/AKT/mTOR pathway in angiogenesis. Front Mol Neurosci. (2011) 4:51. doi: 10.3389/fnmol.2011.00051
75. Fiorenza MT, Russo G, Narducci MG, Bresin A, Mangia F, Bevilacqua A. Protein kinase Akt2/PKBβ is involved in blastomere proliferation of preimplantation mouse embryos. J Cell Physiol. (2020) 235:3393–401. doi: 10.1002/jcp.29229
76. Lu DP, Chandrakanthan V, Cahana A, Ishii S, O'Neill C. Trophic signals acting via phosphatidylinositol-3 kinase are required for normal pre-implantation mouse embryo development. J Cell Sci. (2004) 117:1567–76. doi: 10.1242/jcs.00991
77. Buhi WC, Alvarez IM, Kouba AJ. Secreted proteins of the oviduct. Cells Tissues Organs. (2000) 166:165–79. doi: 10.1159/000016731
78. Bauersachs S, Rehfeld S, Ulbrich S, Mallok S, Prelle K, Wenigerkind H, et al. Monitoring gene expression changes in bovine oviduct epithelial cells during the oestrous cycle. J Mol Endocrinol. (2004) 32:449–66. doi: 10.1677/jme.0.0320449
79. Lamy J, Labas V, Harichaux G, Tsikis G, Mermillod P, Saint-Dizier M. Regulation of the bovine oviductal fluid proteome. Reproduction. (2016) 152:629–44. doi: 10.1530/REP-16-0397
80. Yousef MS, Marey MA, Hambruch N, Hayakawa H, Shimizu T, Hussien HA, et al. Sperm binding to oviduct epithelial cells enhances TGFB1 and IL10 expressions in epithelial cells aswell as neutrophils in vitro: prostaglandin E2 as a main regulator of anti-inflammatory response in the bovine oviduct. PLoS ONE. (2016) 11:e0162309. doi: 10.1371/journal.pone.0162309
81. Talukder AK, Rashid MB, Yousef MS, Kusama K, Shimizu T, Shimada M, et al. Oviduct epithelium induces interferon-tau in bovine day-4 embryos, which generates an anti-inflammatory response in immune cells. Sci Rep. (2018) 8:7850. doi: 10.1038/s41598-018-26224-8
82. Morillo VA, Akthar I, Fiorenza MF, Takahashi K, Sasaki M, Marey MA, et al. Toll-like receptor 2 mediates the immune response of the bovine oviductal ampulla to sperm binding. Mol Reprod Dev. (2020) 87:1059–69. doi: 10.1002/mrd.23422
83. Wang H, Dey SK. Lipid signaling in embryo implantation. Prostaglandins Other Lipid Mediat. (2005) 77:84–102. doi: 10.1016/j.prostaglandins.2004.09.013
84. Baratelli F, Lin Y, Zhu L, Yang SC, Heuzé-Vourc'h N, Zeng G, et al. Prostaglandin E2 induces FOXP3 gene expression and T regulatory cell function in human CD4+ T cells. J Immunol. (2005) 175:1483–90. doi: 10.4049/jimmunol.175.3.1483
85. Ting AT, Bertrand MJM. More to life than NF-κB in TNFR1 signaling. Trends Immunol. (2016) 37:535–45. doi: 10.1016/j.it.2016.06.002
86. Wijayagunawardane MPB, Miyamoto A. Tumor necrosis factor α system in the bovine oviduct: a possible mechanism for embryo transport. J Reprod Dev. (2004) 50:57–62. doi: 10.1262/jrd.50.57
87. Ibrahim S, Salilew-Wondim D, Rings F, Hoelker M, Neuhoff C, Tholen E, et al. Expression pattern of inflammatory response genes and their regulatory MicroRNAs in bovine oviductal cells in response to lipopolysaccharide: implication for early embryonic development. PLoS ONE. (2015) 10:e0119388. doi: 10.1371/journal.pone.0119388
88. Sponchiado M, Marei WFA, Beemster GTS, Bols PEJ, Binelli M, Leroy JLMR. Molecular interactions at the bovine embryo-endometrial epithelium interface. Reproduction. (2020) 160:887–903. doi: 10.1530/REP-20-0344
Keywords: small extracellular vesicles, miRNAs, oviductal cells, oviductal fluid, embryo maternal-communication, reproduction, bovine
Citation: Mazzarella R, Bastos NM, Bridi A, del Collado M, Andrade GM, Pinzon J, Prado CM, Silva LA, Meirelles FV, Pugliesi G, Perecin F and da Silveira JC (2021) Changes in Oviductal Cells and Small Extracellular Vesicles miRNAs in Pregnant Cows. Front. Vet. Sci. 8:639752. doi: 10.3389/fvets.2021.639752
Received: 09 December 2020; Accepted: 02 February 2021;
Published: 04 March 2021.
Edited by:
Islam M. Saadeldin, King Saud University, Saudi ArabiaReviewed by:
Waleed Fawzy Marei, University of Antwerp, BelgiumMohamed Samir, Zagazig University, Egypt
Copyright © 2021 Mazzarella, Bastos, Bridi, del Collado, Andrade, Pinzon, Prado, Silva, Meirelles, Pugliesi, Perecin and da Silveira. This is an open-access article distributed under the terms of the Creative Commons Attribution License (CC BY). The use, distribution or reproduction in other forums is permitted, provided the original author(s) and the copyright owner(s) are credited and that the original publication in this journal is cited, in accordance with accepted academic practice. No use, distribution or reproduction is permitted which does not comply with these terms.
*Correspondence: Juliano Coelho da Silveira, anVsaWFub2Rhc2lsdmVpcmEmI3gwMDA0MDt1c3AuYnI=