- 1Institute of Animal Breeding and Husbandry, Christian-Albrechts-University, Kiel, Germany
- 2Gesellschaft für Marine Aquakultur mbH (GMA), Büsum, Germany
- 3Department of Aquaculture and Sea-Ranching, Faculty of Agricultural and Environmental Science, University of Rostock, Rostock, Germany
- 4Institute of Genome Biology, Leibniz Institute for Farm Animal Biology (FBN), Dummerstorf, Germany
Blood analyses provide substantial information about the physiological aspects of animal welfare assessment, including the activation status of the neuroendocrine and immune system, acute and long-term impacts due to adverse husbandry conditions, potential diseases, and genetic predispositions. However, fish blood is still not routinely analyzed in research or aquaculture for the assessment of health and/or welfare. Over the years, the investigative techniques have evolved from antibody-based or PCR-based single-parameter analyses to now include transcriptomic, metabolomic, and proteomic approaches and from hematological observations to fluorescence-activated blood cell sorting in high-throughput modes. The range of testing techniques established for blood is now broader than for any other biogenic test material. Evaluation of the particular characteristics of fish blood, such as its cell composition, the nucleation of distinct blood cells, or the multiple isoforms of certain immune factors, requires adapted protocols and careful attention to the experimental designs and interpretation of the data. Analyses of fish blood can provide an integrated picture of the endocrine, immunological, reproductive, and genetic functions under defined environmental conditions and treatments. Therefore, the scarcity of high-throughput approaches using fish blood as a test material for fish physiology studies is surprising. This review summarizes the wide range of techniques that allow monitoring of informative fish blood parameters that are modulated by different stressors, conditions, and/or treatments. We provide a compact overview of several simple plasma tests and of multiparametric analyses of fish blood, and we discuss their potential use in the assessment of fish welfare and pathologies.
Introduction
A significant segment of the constantly growing aquaculture sector is represented by intensive farming practices (1) aimed at meeting the increasing demand of a growing world population. However, at the same time, this emphasis raises many concerns due to the competition for resources and the negative impact of intensive aquaculture on the environment and fish welfare (2). Consequently, the visionary concepts for future fish farming include welfare-friendly systems that can monitor animal-based parameters and quickly regulate disruptive environmental variables when necessary (3, 4). Certified farming procedures should be based on a comprehensive, science-based understanding of how teleost fish respond to anthropogenic environmental disruptions and challenging aquaculture-related conditions. This requires multidisciplinary investigations to define and evaluate optimal species-specific husbandry conditions, especially for newly introduced aquaculture species (5). Consequently, research groups and large scientific consortia are currently studying the influences of a variety of factors, ranging from global climate change, ocean acidification, and eutrophic habitats to introduced pathogens and pollution by environmental toxins or microplastics, on fish breeding and wild fish stocks. The use of more extensive research approaches to screen the effects of environmental conditions can increase the probability of detecting disturbances and even dangerous confounding factors, thereby allowing the discovery of relevant diagnostic biomarkers for fish health.
One favorable option for rapid and non-lethal sampling of large numbers of fish individuals is blood analysis. Blood is a complex mixture of heterogeneous cell populations (6–8) that include erythrocytes (red blood cells), leukocytes (white blood cells), and thrombocytes (analogs of mammalian platelets) (9). These fish blood cells are broadly similar in function to their mammalian counterparts and are found in all other tissues and organs throughout the body. Blood transports an immense variety of substances (gases, water, minerals, nutrients, hormones, immune effectors, toxins, microbial structures, or waste products), so its analysis can provide a wealth of information about fish physiology and health status. Alterations in informative blood-based indicators like metabolite concentrations, hormonal profiles, and transcript abundances can reflect systemic reactions to changes or disturbances in homeostasis that can alert scientists and veterinarians (as well as fish farmers), who monitor the physiological status of an individual fish or an entire population.
Blood tests on fish have been carried out for decades (10) in laboratory and field settings to assess endocrine, reproductive, and immune functions; maturation; nutrition and health status or to perform genetic studies (11). More analysis techniques have been established for blood as a test material (Figure 1) than for any other tissue or fluid sample. Nevertheless, interpreting the obtained data to extract meaningful information on the individual condition remains difficult. On the one hand, a few excellent reviews have recently updated the history of selected hematological techniques (12, 13); however, remarkably, blood analyses using systems biological approaches or even PCR-based techniques are still underrepresented in fish physiology (14). On the other hand, the omics-based hypotheses put forward by fish geneticists and molecular biologists are correlated only on a small scale with non-transcript parameters extracted from fish blood.
A growing part of the research on farmed fish focuses on “animal welfare” involving optimization of husbandry conditions, stress avoidance, and improvement of fish quality of life (15, 16). The focus is largely on the ability of domesticated fish populations to cope with environmental and/or anthropogenic challenges (17). In general, animal-welfare programs aim to ensure freedom from (i) thirst, hunger, and malnutrition; (ii) discomfort; (iii) pain, injury, and disease; (iv) restriction of normal behaviors; and (v) fear and suffering (18). These basic aspects also apply to some extent to the welfare of fish in aquaculture. However, fish require different treatment than terrestrial animals in many ways due to their aquatic nature and differences in their anatomy and physiology, as well as in their required husbandry conditions. According to Huntingford et al. (19), fish welfare can be expressed by (i) a feelings-based definition, focusing on a reduction in pain and negative emotions, and/or increased access to positive experiences; (ii) a nature-based definition assuming that every fish species must express its inherent biological nature; or (iii) a function-based definition targeting the ability of fish to adapt to environmental demands.
The well-being of fish in aquaculture is impaired by acute environmental changes, coupled with husbandry practices, such as sorting, grading, and transport that can induce stress responses. Most research studies are based on the third concept (iii), which involves fish health and the adequate functioning of fish biological systems, especially those involved in managing a compromised homeostasis imposed by aquaculture conditions. The physiological state of a fish with disturbed homeostasis is usually captured by observing and recording indicative signs and measurable characteristics of the response to environmental challenges or stressors. Restoration of homeostasis usually requires the invocation of complex behavioral and physiological adaptive responses (20).
Primary stress responses are characterized by the release of catecholamines and corticosteroids (21). The subsequent secondary stress responses have a multitude of actions in many tissues, including blood, and can range from accelerated mobilization of energy via glucose, altered hydromineral balance, and increased lactate levels to decreased blood pH, hematocrit, and sodium levels and lower liver glycogen levels (20, 21). Increased cardiovascular activity and breath rate that enhance the uptake and transport capacity for oxygen are accompanied by a redistribution or suppression of immune functions (21, 22). Tertiary responses are reflected by behavioral adaptions, inhibited growth, decreased reproduction, and a compromised capacity to endure any additional stressors. The repeated exposure to a low-intensity stressor triggers the development of an adaptive response over time and attenuates acute stress reactions, whereas the exposure to harmful, persistent stressors is likely to intensify the physiological response (23). When stressors co-occur with a high pathogenic pressure, they provoke serious diseases (24) and strongly impair fish welfare (25).
Many previous and current studies on fish welfare have measured the main components of the primary physiological stress response, largely plasma cortisol and glucose (26–28). Both components are informative stress markers, but they have limitations (29). The current scientific consensus is that the assessment of fish welfare is a complex task (30), in part because the absence of a physiological stress response does not necessarily imply adequate welfare (31). Each fish species has distinct “ecological and behavioral demands” (15); therefore, the responses to adverse conditions vary across taxa (32, 33). In recent years, the scientific evaluation of fish physiology has shifted from the conventional, limited biomarker approach to comprehensive and rather holistic approaches (34, 35). The spectrum of the parameters now recorded has expanded and is accompanied by an increasingly well-equipped fish-specific toolbox (Figure 1). These advances now facilitate the generation of weighted welfare scores (36, 37) that can integrate operational and laboratory-based parameters.
Laboratory data, such as gene expression studies (30, 38, 39), are based on RNA specimens that are mostly obtained from the organs of previously killed fish. By contrast, fish blood collection can be conducted non-lethally (11); therefore, blood represents an alternative and preferable matrix for ethical reasons. Though relatively non-invasive, blood sampling is generally stressful for fish, but repeated blood sampling from the same individual provides the possibility of tracking the time course of various processes after the treatment or determining fish welfare during the developmental stages. Other operational parameters, such as the monitoring of exploratory and swimming behavior (40, 41) or the rate of gill ventilation (42), can readily be recorded on commercial farms, even those that lack the well-equipped wet laboratories.
This review compiles the most commonly reported techniques for obtaining chemico-physical, cellular, metabolic, and transcriptional information from fish blood. It also provides interpretations of the various methods in terms of their importance in assessing the impact of changing environmental conditions or experimental treatments and the intensity and duration of the response of fish.
Anesthesia and Blood Sampling Procedures
For gentle handling and for safety reasons, fish should be sedated prior to and during blood sampling to minimize pain or discomfort and to prevent defensive or flight reactions and subsequent injuries. An analgesic alone may mask the sensation of pain but will not prevent the perception of subsequent treatments. A loss of consciousness, therefore, ensures the welfare of the fish during blood collection. The most commonly used anesthetic is MS-222 (also known as Tricaine-S or 3-ethoxycarbonylanilinium methanesulfonate) (43), although clove oil (containing eugenol, 4-allyl-2-methoxyphenol), quinaldine (2-methylquinoline), 2-phenoxyethanol, and benzocaine (ethyl 4-aminobenzoate) are also commonly used to stun fish (44). The legal provisions of a given country determine the circumstances that justify the use of particular fish anesthetics. Anesthetics are usually administered as an immersion bath, but the capture of the fish and its transfer to the anesthetic bath, with the brief exposure to air, are likely to evoke a stress reaction (45, 46). Therefore, these steps should be carried out quickly. The anesthetic per se can also act as a stressor on the individual fish being treated.
MS-222 blocks the sodium channels and action potentials of neurons, but concentrations of up to 300 mg MS-222 per liter were shown to have no significant impact on the plasma cortisol levels of South American silver catfish (Rhamdia quelen) (47). By contrast, gilthead sea bream (Sparus aurata) showed significantly increased cortisol levels following the exposure to even 25 mg/L MS-222 or 0.075 mg/L 2-phenoxyethanol (48). In zebrafish, 15 mg/L of buffered MS-222 altered various hematological characters, including hematocrit, coagulation, and the amount of blood collected (49). The influence of a given anesthetic on the biochemical profile of a sample obviously depends on the dose and on the treated species. These considerations should therefore be taken into account during the evaluation of fish blood parameters. Nevertheless, the anesthesia certainly facilitates the blood collection and prevents a more pronounced stress response, so these advantages may outweigh the potential difficulties related to stress diagnostics.
Optimal blood collection depends on the size and anatomical characteristics of the investigated individual (11). The least traumatic and most widely used blood sampling procedure is to withdraw blood from the caudal vessels—laterally or ventrally—using a cannula and syringe filled with an anticoagulant (sodium citrate, heparin, or potassium ethylenediaminetetraacetic acid) (Figure 2). Some analyses require quite large amounts of blood, which creates difficulties when the blood is collected from small teleosts with an estimated whole-blood volume of 3–7 ml per 100 g (50–52). Fish smaller than 8 cm in length are difficult to bleed, but one option is to sever the fin of an anesthetized small fish and then centrifuge the fish at low force (14). Larger teleosts may also be bled by puncture of the cardiac ventricle or other vessels, such as gill-blood vessels (Figure 2) (53).
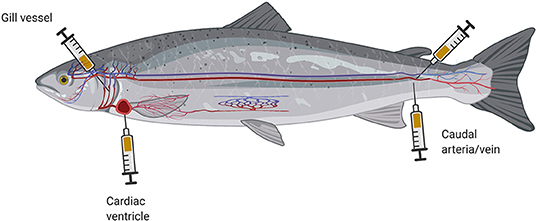
Figure 2. Schematic illustration of different cannula placements for blood collection from fish (created with BioRender.com).
These blood sampling techniques are reserved for studies aimed at answering distinct research questions (54), as they bear a high risk of late complications. In this context, a point worth noting is that the site of blood collection often influences the obtained biomarker profile (55). More detailed information on blood collection techniques has been provided in recent review articles (45, 56). After blood sampling, the fish should be transferred to an aerated recovery tank to allow it to regain perception, awareness, and ability to react. Immediate return of a fish to its usual environment poses a risk that it may be attacked by its tank mates if it remains in a state of non-reactivity.
The liquid part of the blood—the plasma fraction—transports diverse metabolites of interest, including hormones and catabolic products, such as proteins, amino acids, glucose, lipids, and organic acids. For this reason, many researchers separate the plasma via centrifugation with an anticoagulant or obtain the serum after blood clotting and subsequent centrifugation (57). Hence, plasma contains natural coagulation factors, whereas serum contains all plasma components except the coagulation factors. Thus, the choice of using plasma or serum depends largely on the research question and its related need for coagulation factors.
Immunoassays and Clinical Test Kits
Many studies that have evaluated fish welfare in aquaculture have revealed that aversive husbandry conditions alter the stress-hormone levels in the farm fish. Stressors and adverse conditions can trigger the chromaffin cells of the head kidney to rapidly release catecholamines, such as noradrenaline/norepinephrine and adrenaline/epinephrine. These reactions may also occur in direct response to the catching process, the subsequent anesthesia, and/or the blood sampling, so they complicate the evaluation of experimentally induced challenges. However, the hypothalamus generates a parallel production of corticotropin-releasing hormone (CRH), which then stimulates the pituitary gland to release adrenocorticotropic hormone (ACTH) (19, 58, 59). This is followed by the synthesis and secretion of cortisol by the interrenal tissue in the head kidney. Many scientists prefer to measure cortisol or ACTH as blood-based indicators of acute stress responses due to the slow increase (within minutes) of these compounds.
Cortisol is probably the most commonly measured molecule for determining the effects of stress in fish (16, 35). Cortisol and ACTH are commonly measured using radioimmunoassays (RIAs). Typically, RIA incorporates a radioactive iodine isotope due to its decay properties. However, the higher technical effort required in an isotope laboratory has led to an increasing preference for ELISAs (60) as an alternative non-radioactive method for the cortisol and ACTH measurements. Both RIAs and ELISAs are antibody-based detection methods, but ELISAs use specific antibodies that bind to the substance (antigen) and rely on an enzymatic color reaction for antigen detection. Electrochemical immunoassays (61), gas or liquid chromatography (62), mass spectrometry (63), and fluorescence-based methods (64) are alternatives to RIA and ELISA for determining blood cortisol levels.
Increased cortisol levels stimulate a number of metabolic processes, such as glucogenolysis and gluconeogenesis, to mobilize and allocate energy reserves (e.g., glucose release into the bloodstream) (21). Increased, as well as decreased, glucose levels are considered signs of stress (Table 1) (88), as stress-induced alterations in muscle activity accelerate the conversion of glucose to lactate (or alternatively to pyruvate) by anaerobic glycolysis. The resulting plasma levels of lactate and glucose are often used in conjunction with cortisol levels to establish the physiological state and to assess the severity of a stress response (9, 87, 99–101). Some studies have demonstrated that even the removal of an individual S. aurata fish evoked an acute stress reaction in the remaining fish in the tank. The plasma cortisol levels in the tank fish returned to the initial level after 8 h, whereas the plasma glucose and lactate levels and other hematological parameters required 24 h for recovery (102). The plasma triglycerides or cholesterol levels can also reflect the metabolic changes and serve as physiological indices (86), as these lipids can be used as alternate sources of energy by the fish (103–105). Blood glucose, lactate, and triglyceride levels can be determined easily and quickly using appropriate cuvette tests or test strips in portable devices designed for clinical test kits (also known as point-of-care tests) (76). The advantages of point-of-care tests are their time-saving and simple operation, with their reliable and easily readable results.
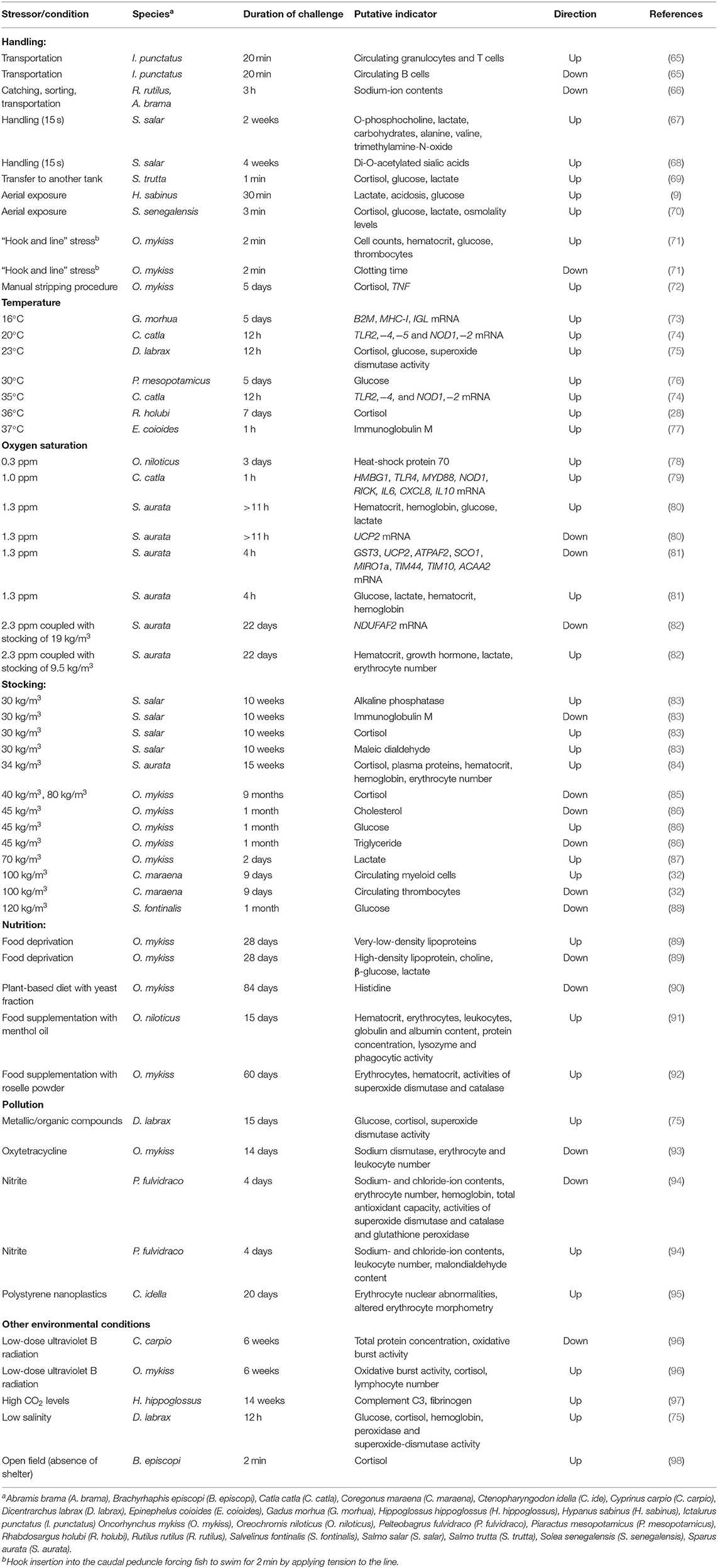
Table 1. Overview of the selected physiological parameters that change in blood, plasma, or serum in response to stressful conditions in various fish species.
Changes in cortisol levels indicate the magnitude of a primary stress response, but usually do not provide sufficient information to gauge the ability of an animal to cope with a challenging condition (106). Notably, cortisol levels can vary widely among individuals in response to diurnal or seasonal fluctuations and the ambient temperature (107–109) or according to gender and maturity (58, 106, 110, 111). The cortisol level depends on many factors that can have diverse interactions (29). In optimal cases, the cortisol level rises in correlation with the intensity of a stressor and returns to its baseline level if the stressor does not persist. Multiple stressors occurring simultaneously or persistent chronic stress conditions can complicate the interpretation of a measured cortisol level, as cortisol release might be suppressed by feedback interactions through the activated stress axis. In particular, differences in treatments can complicate comparisons between experiments (112); therefore, we recommend measuring other parameters of the secondary and tertiary stress response to establish the ability of the animal to cope with stressors and to assess its well-being.
Hematological Profiling and Blood Cell Sorting
The original technique for obtaining a differential blood count is simple and relatively inexpensive, but time-consuming and tedious (113). Hematological evaluations are usually based on blood smears and require a sound knowledge of blood cell morphology.
The vast majority of blood cells are erythrocytes, which ensure a sufficient supply of oxygen in the various body tissues. Metabolic alterations associated with physical work, excitement, and stress responses increase the tissue oxygen requirements, so large numbers of erythrocytes are additionally recruited and mobilized from depots in the spleen (114). For instance, rainbow trout (Oncorhynchus mykiss) responded to a 2-month food supplementation with roselle (Hibiscus sabdariffa) meal by significantly increasing the number of erythrocytes in the blood and simultaneously lowering the blood cortisol and glucose levels (92). By contrast, Indian major carp (Labeo rohita) exposed to water 6°C warmer than usual showed significantly increased glucose levels and a reduction in erythrocyte counts (115). A recent examination of the erythrocytes from grass carp (Ctenopharyngodon idella) did not reveal any significant alteration in numbers after 20 days of exposure to polystyrene nanoplastics, but numerous abnormalities were observed regarding the shape and size of the cells and their nuclei (95).
Adverse environmental conditions can affect the numbers and shapes of circulating erythrocytes, but they can also change the composition of leukocytes in circulation (58, 116, 117). Leukocytes are generally subdivided into monocytes, lymphocytes, and granulocytes (118). Granulocyte staining with Romanowsky/Wright or May–Grünwald–Giemsa stains can differentiate the eosinophilic, basophilic, and neutrophilic types (119, 120) that occur in tetrapods. Some fish species possess heterophilic granulocytes that are characterized by the presence of additional eosinophilic granules (121, 122).
As reported in other vertebrates (123), the ratios of certain leukocyte populations in fish blood can provide insights into the response to defined treatments or environmental variables. Stress hormones inhibit the proliferation of lymphocytes (58), the apoptosis of granulocytes (124), and the emigration of monocytes and neutrophilic granulocytes from the hematopoietic tissue of the head kidney into the peripheral blood (125). A high number of circulating leukocytes (leukocytosis) can therefore reflect an increased number of monocytes (monocytosis) and neutrophilic/heterophilic granulocytes (neutrophilia) and a concomitant decrease in the number of lymphocytes (lymphopenia) (126). The resulting dysregulation of the immune system can lead to a persistent inflammatory state in fish (127).
Although the neutrophil/heterophil-to-lymphocyte ratio is considered as an important indicator of distress across vertebrate species, it does not necessarily correlate with stress-hormone levels (128). The delayed mobilization of immune cells following the cortisol peak has been regarded as a mechanism that enables the immune system to respond once the primary threat has been overcome (129). The response of the neutrophil/heterophil-to-lymphocyte ratio to long-term environmental stressors is detectable over relatively long periods of time, in contrast to the temporary increase in hormone levels (130). Infections also have a decisive influence on the proliferation and trafficking of leukocytes (131), and the coincidence of stress and immune responses can have an antagonistic effect on the ultimate number of peripheral leukocytes (132).
Differential blood counts have been used to assess the effects of acute and chronic stress events, such as heavy metal exposure in common carp (Cyprinus carpio) (133), organochloride herbicide exposure in African catfish (Clarias gairepinus) (134), or crowding in Atlantic salmon (Salmo salar) (135). Nevertheless, conducting a differential blood count in fish is not very common in clinical laboratory diagnostics, often because reference values are missing.
Flow cytometry is an alternative method for studying the blood cell composition and has the advantage of high sample throughput. In the flow cytometer, individual blood cells successively pass by a laser beam, and the light they scatter is characteristic for a specific blood cell population, allowing their separation. The use of specific antibodies facilitates a more precise determination of the proportions of specific immune cell subpopulations in the blood. Fish cell sorting has depended more on cell characteristics, such as size and granularity, because of a general lack of fish-specific antibodies except for a few model fish species (7, 136).
Similar alterations in blood cell composition have been studied in different fish species exposed to various types of stress (137). For instance, in channel catfish (Ictalurus punctatus), the number of circulating neutrophil granulocytes increased after transportation stress (138), while the overall number of leukocytes (including lymphocytes) decreased. Another study on I. punctatus confirmed the occurrence of the previously observed neutrophilia simultaneously with decreasing numbers of peripheral B-lymphocytes in response to handling and transportation (Table 1) (65). In Gulf killifish (Fundulus grandis) and sea trout (Cynoscion nebulosus), the response to crude-oil pollution was characterized by a significantly decreased number of circulating lymphocytes and an increased number of monocytes and eosinophilic granulocytes, respectively (139). Maraena whitefish (Coregonus maraena) exposed to high stocking densities (100 kg/m3) showed strong increases in the numbers of myeloid cells (granulocytes, monocytes, myeloid dendritic cells, and mast cells), whereas the number of thrombocytes was significantly reduced (Table 1) (32). Overall, different types of stress apparently trigger an increased mobilization of myeloid cells and a reduction in the levels of lymphocytes in the circulation of the affected fish.
Hematocrit Measurements
As outlined in the previous section, the stress-related secretion of cortisol might provoke contractions of the fish spleen to induce the release of stored erythrocytes into the peripheral blood (114). This would then elevate the volume percentage of erythrocytes, also referred to as the hematocrit value. Along with the hemoglobin (Hb) content and the leukocyte count, the hematocrit is regarded as a key indicator of the secondary stress response. The hematocrit measurement is easy and relatively inexpensive, as the collected whole blood is simply centrifuged in heparinized micro-hematocrit capillaries that are then read off a measuring template (140). A substantially greater effort is required to establish leukocyte profiles; consequently, hematocrit measurements are about 50 times more common in fish studies, as evident from our recent literature search in the Web of Science.
One important aspect for fish physiologists is the association between the hematocrit and the blood viscosity (141, 142). Wells and Weber measured the blood viscosity in O. mykiss kept under stressful conditions and found that a 30% hematocrit indicates an optimal relative oxygen transport capacity in the presence of a blood viscosity with a variable hematocrit but a constant Hb concentration (142). The hematocrit in S. salar ranges between 44 and 49% (143); however, the levels depend on the temperature (115, 144), fish strain (145), diet (91), and body weight. Large, active fish generally have a high muscular oxygen demand, which can lead to a stimulation of erythropoiesis in the head kidney (146, 147). Accordingly, the physiological hematocrit differs between fast-swimming pelagic fish and fish living sedentarily or in benthic habits (148).
Anesthesia can increase the hematocrit (149), while malnutrition, infection, or environmental toxins can reduce hematocrit and Hb values in fish (150). Non-physiologically low hematocrit values are considered hallmarks of anemia, a specific pathophysiological stress response. Anemia in fish can be easily diagnosed by examining the gills, although more detailed blood analyses help to identify the cause of anemia (53). A significantly reduced hematocrit and an increased erythropoietin production, for instance, can be observed with experimental hemolysis induced by the hemolytic compound phenylhydrazine in S. salar (151).
The hematocrit and Hb values associated with erythrocyte count can also depend on the electrolyte–water balance of the fish blood (152). Stressed freshwater fish undergo a drop in their plasma sodium concentrations, and this drop activates counter-transporting ion channels on the erythrocyte membranes (66). The rising ion concentration then induces an inflow of water, causing the erythrocytes to swell and increase their binding capacity for oxygen. These responses are accompanied by the release of additional erythrocytes from splenic stores to compensate for the increased oxygen demands.
Measurements of Osmolality and Ion Contents
The electrolyte–water balance in the body is termed its osmolality. The plasma osmolality and osmotic regulatory capacity are measured with an osmometer, whereas a spectrophotometer can identify the contributing ions; these are predominantly sodium, chloride, calcium, magnesium, potassium, and phosphate (46).
Freshwater fish species are hyperosmotic in relation to their habitat, whereas marine fishes are hypo-osmotic to the surrounding sea. The resulting differential osmotic pressures force teleost fishes to undergo continuous osmoregulation. Water and ions are exchanged primarily via the skin, gills, intestines, and kidneys (52, 153, 154), though freshwater and marine fishes have developed different strategies to maintain their internal blood osmolality within narrow limits. Osmoregulation is a persistently energy-intensive process, even in the absence of additional stress. However, since stress hormones control both the hydromineral balance and energy metabolism in fish, variations in the osmolality of the blood plasma, including changes in the ion composition, are part of the secondary stress response (155, 156). In general, aversive conditions decrease the osmolality in freshwater fish and increase osmolality in marine fish (Table 1) (59, 69, 70, 145, 157, 158). The exposure to high nitrite concentrations, for example, caused a significant reduction in sodium and chloride ion contents in the blood of the freshwater species yellow catfish (Pelteobagrus fulvidraco) (94). Catching and a subsequent 3-h-long transportation of freshwater roach (Rutilus rutilus) and common bream (Abramis brama) reduced the level of plasma sodium by one-third (66). By contrast, the osmolality significantly increased in the plasma of the marine Japanese flounder (Paralichthys olivaceus) after the acute exposure to air (159).
Adverse environmental conditions (e.g., hypoxia, which is often associated with high ambient temperature) require an increased branchial activity to enhance the uptake of oxygen. In (hypotonic) freshwater, this hyperventilation accelerates the loss of osmolytes. The conflicting demands of osmoregulation and respiratory gas exchange cause an increased oxygen uptake and loss of ions from the plasma in freshwater fishes. This concept of the “osmorespiratory compromise” has been well-researched in salmonids (160, 161) and hypoxia-tolerant and euryhaline fishes (162). The salinity level (162, 163), the species-specific cellular gill architecture (164), and various extreme environmental variables (165) influence the osmoregulatory ability of fishes under stressful conditions and can maintain physiological osmolality (166, 167) and ion levels (168) in response to diverse challenges in freshwater and saltwater species in their native osmotic environments. For example, ion concentrations were unaffected in Pacific hagfish (Eptatretus stoutii) exposed to hypoxia (169) or in Gulf toadfish (Opsanus beta) stimulated with spill oil (170).
Some fishes compensate relatively quickly for increased ion flux rates within a certain range; therefore, ion concentrations and osmolality can serve as suitable indicators of acute environmental stressors (140). On the other hand, both parameters change less rapidly compared to the dynamics of stress-hormone levels, making this difference advantageous for recording post-stress responses.
Assessment of the Humoral Immune Capacity
The response to distinct external signals involves the neuroendocrine system and the immune system (171). For decades, researchers have extracted various immune-relevant parameters from fish blood and evaluated their potential as indicators for compromised homeostasis (172). The central question addressed by these studies is the extent that stress and related adaptive responses influence immunocompetence in fish.
An initial test for assessing immunocompetence is to record the bacterial growth rate in blood plasma from treated vs. control fish (173–176). For example, the growth of the bacterium Aeromonas salmonicida after a 24-h incubation was significantly enhanced in plasma from O. mykiss with impaired immune capacity due to the exposure to high temperature coupled with crowding (177). By contrast, the non-stressed fish clearly showed potent bactericidal mechanisms that depended mainly on the concentration of a range of immunocompetent macromolecules known as humoral factors. These humoral factors consist of antimicrobial peptides, antibodies, and complement components (178) that circulate in the body fluids. Many fish physiologists have therefore examined the activity of these specific humoral factors rather than the general bactericidal activity of the plasma.
The bactericidal enzyme lysozyme and the microbe-clearing complement components are important humoral molecules of the teleostean innate immune defense (179, 180) and are frequently used as non-specific immune markers (181). In fish, lysozyme has a broader activity than its mammalian counterpart, and several complement components are present as multiple isoforms in bony fishes (182). Lysozyme and complement components are mainly synthesized by leukocytes and the liver, respectively (183–185), but they are secreted into the blood to eliminate invasive pathogens and harmful agents. However, the actual concentrations of the multiple immune factors that are synthesized and secreted into the blood of various fishes at different stages of an infection process or during a challenging situation are not known. Therefore, the measured values for supposedly one factor could actually represent multiple variants and should be interpreted with caution.
The activity of lysozyme and the complement system are measured using turbidimetric assays (186), lysoplate assays (187), lysorocket electrophoresis (188), or microplate assays (189). These assays have demonstrated, for example, that acute handling increased the activity of lysozyme in O. mykiss (181) and goldfish (Carassius auratus) (190). By contrast, lysozyme activity decreased in Siberian sturgeon (Acipenser baerii) after heat stress (189), in sheatfish (Silurus glanis) after exposure to intense halogen light (191), in Nile tilapia (Oreochromis niloticus) after exposure to the pesticide chlorpyrifos (91), and in O. mykiss after transport and exposure to chemicals (192, 193) or low stocking densities (85). Subordinate O. niloticus individuals had lower lysozyme activity levels than their dominant conspecifics (194). Overall, stress seems to dampen the lysozyme activity in fish (193).
The influence of stress on the complement system is ambiguous. For example, heat-stressed A. baerii (189) and acutely stressed S. aurata (195) showed increased complement activity, whereas this activity decreased in European bass (Dicentrarchus labrax), red porgy (Pagrus pagrus), and S. aurata exposed to crowding (84, 196–198). The complement gene families are expanded in several fishes (180, 185), and this has often been associated with newly acquired or partitioned functions of the original complement factors. Consequently, complement-involving events are likely to be more complex than indicated by the snapshot provided by a complement test result.
Other parameters have also proven significant for the assessment of fish welfare. These include the coagulation capacity of the blood (71), the antibody titer (83, 96), the phagocytic activity (32, 91), or the oxidative burst (199, 200), including the activities of the myeloperoxidase (201), superoxide dismutase (75, 202), glutathione peroxidase (93, 94), or glutathione reductase (152). Together with the bactericidal activity, these parameters provide valuable downstream information about the effects of stress hormone release on the immune performance under challenging conditions. Hormones are also likely to induce rather subtle changes in the activity of these immune parameters, either daily or seasonally, and between the sexes (203–205). Most of these analyses are carried out ex vivo within a limited time after the previous collection. The oxidative burst assay or phagocytic tests can also be performed in vitro on cultured cells (206).
In vitro Tests on Primary Blood Cell Cultures
The development of in vitro systems has allowed testing of the effect of certain stressors or challenging conditions delivered in defined doses, intensities, time frames, and/or time points. The dominant model systems for primary fish cell cultures are still derived from the head kidney and spleen (207–209); however, many protocols for blood cell cultures from different fish species have been established (210–213). These models are mainly used to investigate the influence of microbial structures/vaccines and viruses on particular immune cascades (212, 213). In addition, blood cells from fish are useful for investigating the influence of drugs or environmental toxins on the cellular homeostasis. For example, low concentrations of a halogenated hydrocarbon (once used as the insecticide lindane) were shown to increase intracellular calcium levels in peripheral blood leukocytes of O. mykiss, whereas high concentrations reduced the synthesis of vital cytokines and induced cell death (214). The polycyclic aromatic hydrocarbon 3-methylcholantrene stimulated the proliferation of blood leukocytes from C. carpio, but inhibited the lymphocyte proliferation in response to immunostimulants (215). Similarly, the toxin microcystin-LR (produced by cyanobacteria) and bisphenol A (the monomer component of polycarbonate plastics) modulated the proliferation of lymphocytes isolated from the blood of O. mykiss (216) and C. auratus (217), respectively. Leukocytes from C. carpio subjected to the “alkaline comet assay” have been used to assess the genotoxic potential of organic sediment by determining the DNA damage (218).
Apart from these toxicological studies, blood cell cultures are actually not suitable for modeling aquaculture-relevant problems, such as malnutrition or stocking density stress. For this reason, generalizing the data obtained from in vitro systems to an entire organism or a population is controversial, as the response to environmental stress is usually systemic and involves complex cellular networks and tissue systems that communicate with each other via stress-inducible mediators, such as steroids and amines. Only a few studies have investigated how stress hormones affect events like the in vitro proliferation of blood cells (219). The further development of three-dimensional cultures from fish cells (220) will bring in vitro data one step closer to their practical use as fish model systems.
Expression Profiling of Selected Genes in Blood Cells
Quantitative PCRs (qPCRs) detect the smallest alterations in the expression of genes (221) that are subject to modulation by environmental challenges or stressors. Importantly, gene expression profiles rarely allow absolute statements about the functioning of biological systems, for a number of reasons. One is that many genes are not completely switched on or off in response to a specific treatment or under certain environmental conditions. Instead, most treatments induce a stronger (upregulated) or a reduced (downregulated) expression of a distinct set of genes, and these expression changes only become evident when treated cells are compared with an untreated matching control. One case in point is the expression of potential thermal indicator genes that correlate with the well-studied response of many fish to suboptimal water temperatures (189). Heat stress is well-known to induce the expression of certain heat-shock protein (HSP) genes, such as HSP70 (HSP1A1) and HSP90 (HSP90AA1) (189, 222, 223), whereas hypothermia also induces the copy number of HSP90 in the blood of C. carpio (224). The HSP-encoding transcripts have also been proposed as indicators of the potentially destructive effects of environmental toxins and pollutants. For example, the level of HSP90 copies dropped almost by half in the blood cells of C. carpio exposed to cadmium for 24 h (224). By contrast, the abundance of HSPA8 copies increased, together with the HSP70 level, in silver sea bream (Sparus sarba) exposed to sublethal concentrations of cadmium for only 2 h (225).
Several investigations have also demonstrated that thermal stress modulates the expression of immune genes in the blood cells of different fish species. In particular, cytokine-encoding transcripts appear to mirror the immune status during stress (226). The classic cytokines, such as interleukins (IL), tumor necrosis factor α (TNF), interferon (IFN), and transforming growth factor (TGF), are relevant in this context (72, 226, 227). The head kidney, spleen, and liver are the usual tissue choices for quantifying immune-relevant transcripts in stimulated or stressed fish, whereas the skin, gills, and blood are used to detect impaired homeostasis. For instance, a temperature study exposed Atlantic cod (Gadus morhua) to water temperatures rising from 10°C to 16°C or 19°C and reported slightly increased plasma glucose and cortisol levels (73). In parallel, upregulated expression was observed for the genes encoding interleukin-1β (IL1B), β2-microglobulin (B2M), major histocompatibility complex class I (MHC-I), and immunoglobulin M light chain (IGL) in leukocytes of the thermally challenged G. morhua (Table 1). These findings were partly confirmed by a report of increased IgM-transcript levels in blood cells of orange-spotted grouper (Epinephelus coioides) after heat shock (77). The Indian major carp (Catla catla) exposed to temperatures above and below the optimum temperature of 25°C showed a significant increase in blood expression levels of immune genes coding for toll-like receptors (TLR2,−4,−5) and nucleotide binding oligomerization domain containing proteins (NOD1,−2) (Table 1) (74).
An expanded set of immune genes was profiled in C. catla exposed to an oxygen saturation below 3 ppm for 1 h (79). The increased expression of the genes coding for the transcriptional regulator high-mobility-group-box-1 protein HMBG1, the receptors TLR4 and NOD1, and their associated adapter proteins myeloid differentiation primary-response protein 88 (MYD88) and receptor interacting serine/threonine kinase 2 (RIPK2), as well as the cytokines IL6, CXCL8, and IL10 suggested an activation of early innate immune mechanisms by hypoxia (Table 1). Other hypoxia studies reported that considerably fewer genes were regulated in fish blood cells, and most were downregulated. For example, a 1-h exposure of S. aurata to hypoxic conditions with an oxygen saturation of 1.3 ppm increased hematocrit, Hb content, glucose and lactate levels, but the level of uncoupling protein 2 (UCP2) transcripts in the blood cells was strongly decreased (Table 1) (80). Another research group also investigated S. aurata under similarly acute hypoxic conditions but with an extended set of qPCR assays (81). They reported a significant downregulation of UCP2 along with reduced levels of transcripts coding for antioxidant enzymes (GST3), outer and inner membrane translocases (TIM44 and TIM10), respiratory enzyme subunits (SCO1 and NDUFAF2), and also markers of mitochondrial dynamics (MIRO1a) and fatty acid β-oxidation (ACAA2) (Table 1). A subsequent experiment by the same group exposed S. aurata to a lowered oxygen saturation of 2.3 ppm combined with crowding (82). The slightly higher oxygen concentration (compared with the previous hypoxia experiment) or an antagonistic effect of hypoxia and density stress was proposed as reasons for the unexpected lack of modulation of either UCP2 or 42 other profiled genes (Table 1). The exception was NDUFAF2 (Table 1), which codes for an assembly factor of the Nicotinamide Adenine Dinucleotide - Hydrogen (NADH): ubiquinone oxidoreductase complex and had been included in the list of differentially regulated genes in their previous hypoxia experiment.
Gene profiling can significantly extend the list of stress response parameters beyond hematological, immunological, and metabolic types by identifying negative biomarkers whose values drop below the control levels (39, 80). A key advantage of gene profiling over the detection of stress hormones is that the stressful events that occur immediately prior to sampling (e.g., due to the capture, stunning, and killing of the animal) are usually not reflected in altered transcript levels, while the levels of ACTH or cortisol increase promptly (see section Immunoassays and Clinical Test Kits). Stress hormones are stored as preformed molecules that can be released within seconds, whereas several minutes are required for activation of the appropriate signaling pathways that culminate in the activation and nuclear transfer of the respective transcription factors that initiate the gene transcription process (228). Nonetheless, stress is mechanistically defined by a hormonal response (229), and this response cannot be adequately demonstrated at the transcript level. Without accompanying data on stress hormone levels, alterations in gene expression might only reflect the adaptive changes in the pathways that reestablish homeostasis.
A shortcoming of transcript-specific assays is that the selection of supposedly suitable parameters is left to the skill and knowledge of the experimenters. Studying the complete set of transcripts facilitates the identification of novel indicators that may not previously have been recognized as relevant. However, in truth, exploratory omics approaches are less effective in elucidating mechanistic insights than they are in generating hypotheses on how subsequent experiments can validate a selection of meaningful biomarkers.
Blood Transcriptomics
High-throughput transcriptomic approaches, such as microarray or RNA-sequencing (RNA-seq) analyses, allow monitoring of the transcriptional changes in a comprehensive panel of potential indicators for a defined research setting (230, 231). In this way, transcriptomic approaches help to arrange traditional and novel parameters into virtual pathways and/or gene networks. RNA-seq is certainly the transcriptomic method of choice over microarrays, which have only been available for a few fish species for decades (230, 232, 233). This shortage is unlikely to be overcome in the future since the availability of reference genomes from a constantly increasing number of fish species and the reduced costs of deep sequencing have now made RNA-seq analyses highly attractive. Another benefit is that RNA-seq analysis distinguishes between individual transcript variants and ohnologous genes, whereas standard PCRs and microarrays typically do not.
The blood cells of most fish species are nucleated (234), whereas the mature erythrocytes and thrombocytes of mammals lack nuclei. This fact alone makes teleostean erythrocytes and thrombocytes highly interesting for (comparative) transcriptomic analyses to record the constitutively expressed transcripts in either cell type (8, 231, 235). Moreover, erythrocytes have been proven to actively supplement allostatic reactions by the induced expression of certain genes. Most investigations have focused so far on the immune responses of erythrocytes after stimulation with pathogen-associated microbial patterns (236), bacteria (237), or viruses (238, 239).
Beyond this, the impact of only a few other stressors has been investigated on the transcriptional response of blood cells. Following an acute exposure of O. mykiss to a 25°C water temperature, the erythrocytes showed altered (at least 2-fold) expression of 26 genes at 4 h and 33 genes at 24 h (240). The panel of upregulated genes comprised a cluster of molecular chaperones, including the genes coding for HSP70 (constitutive and inducible forms), HSP90, and the heat-shock factor-binding protein HSBP1. The genes HSP90, HSP70, and zinc-finger AN1-type-containing protein 2B (ZFAND2B) were later confirmed by literature-mining approaches to represent robust biomarkers for temperature stress in different tissues of salmonid fish (241, 242). Heat stress also increased the transcription of additional stress-related genes, such as stress-induced-phosphoprotein (STIP1) and JUN, and also immune-relevant features, including NF-κB inhibitor α (NFKBIA) and interferon regulatory factor 1 (IRF1) in erythrocytes from O. mykiss, whereas immunoglobulin-encoding genes were downregulated. This example once again points to the close interdigitation of immune and stress pathways in teleost fishes (171, 243, 244).
High water temperatures are often associated with oxygen depletion as a co-occurring stressor. The schizothoracine fish (Gymnocypris eckloni) is an established model for the study of adaptation (245) and hypoxia tolerance (246). A comparison of two G. eckloni cohorts exposed to water containing ~8 mg oxygen per liter (normoxia) or ~3 mg oxygen per liter (hypoxia) for 3 days revealed differential expression of about 70 genes in the blood (q-value <0.05) of the stressed fish (246). Insulin-like-growth-factor-binding protein 1 (IGFBP1) was among the upregulated genes and had previously been identified in the liver of hypoxia-stressed goby fish (Gillichthys mirabilis) (247). The differentially expressed genes were assigned to nine Kyoto Encyclopedia of Genes and Genomes (KEGG) pathways, including hypoxia-inducible factor 1-alpha (HIF1α) signaling and fructose/mannose metabolism. Both pathways are expected to be regulated in the context of oxygen depletion in particular and stress in general.
The transcriptome of blood samples from C. auratus was analyzed to identify a suitable anesthesia method for routine use in aquaculture (see section Anesthesia and Blood Sampling Procedures) (248). This study revealed that most genes were differentially regulated after percussive stunning (877 at least 2-fold regulated features, q-value <0.05), compared with two chemical anesthetics, MS-222 (487 genes) and eugenol (208 genes). Handling of C. auratus triggered the upregulation of a large cluster of genes involved in general stress responses (including heat and cold shock, oxidative stress, and endoplasmic reticulum stress), whereas the anesthetized groups showed comparably fewer differentially regulated stress genes. In addition, all three anesthetics effectively maintained the serum cortisol at low levels (<100 ng/ml).
Transcriptomic profiles are critical for understanding relevant functional pathways and networks, but they also have limitations. One serious problem regarding transcriptome analyses of blood samples (and in other samples from whole tissues and organs as well) is that blood is generally a very heterogeneous mixture of cells, so the transcripts represent an average over a broad range of populations. Single-cell RNA sequencing (scRNA-seq) is one step beyond whole-transcriptome analysis, as it identifies the entirety of the transcriptional changes at the level of an individual cell. The use of an scRNA-seq approach in zebrafish provided novel insights into the unique expression patterns of rare immune cell subsets in the teleostean spleen (249) and documented that scRNA-seq created multifold possibilities for recording the tailored response of distinct blood cells to a defined stressor.
Blood Proteomics
The debate regarding how well-transcript and protein levels correlate (250, 251) is fueled by continuously published confirmatory and contradictory results. Therefore, the safest policy is to consider transcriptome and proteome datasets as complementary. Before qPCR analyses became a standard method in research, antibodies were exploited to record specific biomarkers in blood. The parameters chosen for examination were mostly the already established markers. An additional limitation was that the antibodies, which had generally been produced for mammalian antigens, needed to be cross-reactive (i.e., be able to recognize well-conserved epitope sequences). For instance, the elevated levels of HSP70 in the blood of O. niloticus (Table 1) were reported to indicate acute hypoxia (78), whereas the elevated levels of ubiquitin in the erythrocytes of blue maomao (Scorpis violaceus) suggested confinement stress (252). The protein analysis conducted by 2D- or differential polyacrylamide-gel electrophoresis or by liquid chromatography coupled to tandem mass spectrometry and using matrix-assisted laser desorption/ionization and time-of-flight-mass analysis, now provides more comprehensive insights into the dynamic allostatic events occurring at the protein level (253).
The effects of handling stress in S. salar were examined by Liu et al. (68), who profiled the O-acetylation of sialic acids in the serum. They found that the levels of di-O-acetylated sialic acids increased (Table 1), whereas the levels of mono-O-acetylated sialic acids decreased significantly in stressed fish (68). The exposure of Atlantic halibut (Hippoglossus hippoglossus) to an optimal temperature of 12°C and a suboptimal temperature of 18°C in combination with high-CO2 water (1,000 matm) for 14 weeks resulted in increased levels of the complement component C3 and fibrinogen γ chain (FGB) in the plasma of both high CO2-exposed groups (Table 1) (97). A synthesis of these two factors is triggered very early after injury and pathogen invasion. The plasma of salinity-stressed Mozambique tilapia (Oreochromis mossambicus) also showed high concentrations of C3, together with NADH dehydrogenase, Mg2+-dependent neutral sphingomyelinase, semaphorin, and caspase-3 (254). The phagocytic activity of leukocytes from the head kidney and spleen also decreased in parallel, suggesting that both aspects might be causally connected.
Blood Plasma Metabolomics
Metabolome research is increasingly finding its way into aquaculture research, but it still lags behind the metabolomic-based research in mammalian models (46). Most metabolite structures are identical across species, in contrast to gene and protein sequences; therefore, the analytical assays do not need to be customized for a particular investigated species (34). The metabolites found in the blood plasma include various intermediates from a wide range of biochemical pathways. For this reason, metabolomic analyses of blood serum can be used to understand nutritional (89, 90), developmental (255), or pathophysiological (256) aspects of fish physiology and are increasingly being used for disease diagnostics (257). The most commonly used analytical techniques for studying endogenous metabolite profiles are nuclear magnetic resonance spectroscopy in combination with mass spectrometry or vibrational spectroscopy (258). Most metabolomic studies that have been conducted on plasma samples from fish have dealt with toxicological questions (259–262). Application of a five-percent-by-weight concentration of heavy oil has been reported to increase the levels of several plasma metabolites, including amino acids, butyrate derivatives, creatinine, glycerol, and glucose, in C. carpio (259). These findings suggested a perturbed tricarboxylic acid cycle of energy metabolism. A similar conclusion was drawn following the analysis of plasma samples from zebrafish exposed to the herbicide acetamiprid (260). The insecticide chlorpyrifos was found to enhance gluconeogenesis (glucose and glycerol), fatty acid metabolism (3-D-hydroxybutyrates and acetoacetate), energy metabolism (creatine), and glutamate generation (glutamine and proline) in C. carpio (261). O. mykiss exposed to the synthetic contraceptive estrogen ethinylestradiol revealed increased vitellogenin levels, concomitant with significant changes in the plasma lipid profiles that, in turn, were attributed to the high lipid content of vitellogenin (262).
Metabolomic approaches for blood plasma analysis have also been utilized to address aquaculture-related issues. Food deprivation in juvenile O. mykiss increased the level of very-low-density lipoproteins while reducing the concentrations of high-density lipoproteins, choline, β-glucose, and lactate, in fasted fish (Table 1) (89). The daily netting of juvenile S. salar for 2 weeks disturbed the plasma metabolic balance, as reflected by altered levels of lipoproteins, lipids, lactate, carbohydrates, and specific amino acids (Table 1) (67).
The concentration of a particular enzyme does not necessarily increase or decrease (coupled to an up- or downregulated gene expression) due to varying environmental conditions, though the efficiency in converting certain metabolites may vary. Therefore, metabolic profiling can provide an alternative list of highly sensitive potential biomarkers (34) that can complement the findings of PCR-based techniques and transcriptomics or antibody-based techniques and proteomics. This type of holistic approach can help to coordinate the differentially regulated features in blood and plasma/serum samples in cases where elevated concentrations of a certain metabolite co-occur with increased levels of the associated catalyzing enzyme and with upregulated expression of the enzyme-encoding gene. However, these holistic high-level approaches (cf. Figure 1) remain to be performed in fish.
Conclusions
Blood contains easily accessible information about the individual physiological state of a fish. Nonetheless, blood is not the appropriate matrix for every research question; for instance, not all aspects of “welfare” can be detected in the blood. Several studies have reported the isolation of steroids from matrices other than blood (i.e., mucus, scales, feces, or water) (263–266); however, the data obtained directly from blood are still far more accurate, as the risk of rapid cortisol degradation and contamination from external cortisol sources are evidently lower (16). Most blood sampling techniques are considered minimally invasive for fish above a given size, though sampling activates primary stress responses within minutes. During the experimental manipulations, the researcher should remain aware that the sampling itself might conceal the hallmarks of a (stress) response to previous treatments, thereby biasing the interpretation of the extracted data. In general, the interpretation of blood-derived parameters requires caution, since particular physiological perturbations do not necessarily depend on a given experimental protocol. The metabolic changes, for instance, might also result from persistent chronic disturbances and/or causally independent events (e.g., circadian rhythms, seasonality, feeding times, conspecific aggressions, water quality, etc.) or substandard sampling and laboratory-specific procedures. The influence of sex and body weight/size of the individual fish should also not be underestimated. Multiple parameters should be recorded simultaneously, preferably from different analysis techniques, to disclose unsuitable husbandry conditions and to identify less obvious or previously unnoticed environmental stressors that exceed the adaptive capacity of fish. This approach supports identification of the comprehensive signature of a distinct stressor, thereby allowing valid conclusions to be drawn regarding fish welfare aspects. Unfortunately, the question of which method(s) should be used to detect the signature of a distinct stressor cannot be answered given the current state of knowledge.
This manuscript reviews different methods for recording welfare-related physiological processes in fish blood. Over the past few decades, a broad repertoire of fish-specific tools and methods has been established that enables the quantification of the concentrations of numerous hormones, metabolites, immune factors, and relevant transcripts that now supplement the panel of traditional biomarkers in blood. In the future, high-throughput -omics technologies (particularly transcriptomics, proteomics, and metabolomics) are expected to provide holistic snapshots of the physiological state of an individual. Assembling the ever-growing number of -omics puzzle pieces will ultimately provide a comprehensive picture of the metabolic, transcriptional, and immunological activities in the blood (and other tissues) of fish. Recent technological innovations, such as scRNA-seq and spheroid cell cultures, will further boost the identification of transcriptional signatures in blood cells of farmed and model fish species.
Author Contributions
HS wrote sections anesthesia and blood sampling procedures, hematological profiling and blood cell sorting, hematocrit measurements, measurements of osmolality and ion contents, and assessment of the humoral immune capacity. BB wrote sections anesthesia and blood sampling procedures and immunoassays and clinical test kits. AR wrote sections hematological profiling and blood cell sorting, assessment of the humoral immune capacity, in vitro tests on primary blood cell cultures, expression profiling of selected genes in blood cells, blood transcriptomics, blood proteomics, and blood plasma metabolomics. All authors wrote the introduction, conclusion, and edited the entire manuscript.
Funding
The German Federal Environmental Foundation funded this work (project FKZ 2813NA002). The publication of this review article was funded by the Open-Access Fund of the FBN.
Conflict of Interest
The authors declare that the research was conducted in the absence of any commercial or financial relationships that could be construed as a potential conflict of interest.
Acknowledgments
The authors acknowledge Carsten Schulz (CAU/GMA) for his inspiring ideas and his enthusiastic discussion of the draft manuscript. We thank, especially, the two reviewers of this article, who carefully conducted the revision process in a very constructive and helpful way. Joan Martorell-Ribera, Solvig Görs, and Raphael Koll (all FBN) are acknowledged for providing their results images. The jigsaw-puzzle template for Figure 1 is available at https://www.presentationmagazine.com.
Abbreviations
ACTH, adrenocorticotropic hormone; CRH, corticotropin-releasing hormone; Hb, hemoglobin; HSP, heat-shock protein; IL, interleukin; KEGG, Kyoto Encyclopedia of Genes and Genomes; MHC, major histocompatibility complex; NOD, nucleotide binding oligomerization domain containing protein; NADH, Nicotinamide Adenine Dinucleotide - Hydrogen; RIA, radioimmunoassay; RNA-seq, RNA sequencing; scRNA-seq, single-cell RNA sequencing; TLR, toll-like receptor.
References
1. Leal JF, Neves MGPMS, Santos EBH, Esteves VI. Use of formalin in intensive aquaculture: properties, application and effects on fish and water quality. Rev Aquac. (2018) 10:281–95. doi: 10.1111/raq.12160
2. Leith P, Ogier E, Haward M. Science and social license: defining environmental sustainability of Atlantic Salmon Aquaculture in South-Eastern Tasmania, Australia. Soc Epistemol. (2014) 28:277–96. doi: 10.1080/02691728.2014.922641
3. Dupont C, Cousin P, Dupont S. IoT for Aquaculture 4.0 Smart and easyto- deploy real-time water monitoring with IoT. In: 2018 Global Internet of Things Summit (GIoTS) (Bilbao: IEEE). p. 1–5. doi: 10.1109/GIOTS.2018.8534581
4. Antonucci F, Costa C. Precision aquaculture: a short review on engineering innovations. Aquac Int. (2020) 28:41–57. doi: 10.1007/s10499-019-00443-w
5. Mylonas CC, Robles R, Tacken G, Banovic M, Krystallis A, Guerrero L, et al. New species for EU aquaculture. Food Sci Technol. (2019) 33:22–6. doi: 10.1002/fsat.3302_6.x
6. Fazio F, Saoca C, Costa G, Zumbo A, Piccione G, Parrino V. Flow cytometry and automatic blood cell analysis in striped bass Morone saxatilis (Walbaum, 1792): a new hematological approach. Aquaculture. (2019) 513:734398. doi: 10.1016/j.aquaculture.2019.734398
7. Korytár T, Dang Thi H, Takizawa F, Köllner B. A multicolour flow cytometry identifying defined leukocyte subsets ofrainbow trout (Oncorhynchus mykiss). Fish Shellfish Immunol. (2013) 35:2017–9. doi: 10.1016/j.fsi.2013.09.025
8. Shen Y, Wang D, Zhao J, Chen X. Fish red blood cells express immune genes and responses. Aquac Fish. (2018) 3:14–21. doi: 10.1016/j.aaf.2018.01.001
9. Lambert FN, Treberg JR, Anderson WG, Brandt C, Evans AN. The physiological stress response of the Atlantic stingray (Hypanus sabinus) to aerial exposure. Comp Biochem Physiol A Mol Integr Physiol. (2018) 219–220:38–43. doi: 10.1016/j.cbpa.2018.02.009
10. Field JB, Elvehjem CA, Juday C. A study of the blood constituents of carp and trout. J Biol Chem. (1943) 148:261–9. doi: 10.1016/S0021-9258(18)72280-1
11. Cooke SJ, Lawrence MJ, Raby GD, Teffer AK, Jeffries KM, Danylchuk AJ, et al. Comment: practices for drawing blood samples from teleost fish. N Am J Aquac. (2019) 81:424–6. doi: 10.1002/naaq.10115
12. Fazio F. Fish hematology analysis as an important tool of aquaculture: a review. Aquaculture. (2019) 500:237–42. doi: 10.1016/j.aquaculture.2018.10.030
13. Burgos-Aceves MA, Lionetti L, Faggio C. Multidisciplinary haematology as prognostic device in environmental and xenobiotic stress-induced response in fish. Sci Total Environ. (2019) 670:1170–83. doi: 10.1016/j.scitotenv.2019.03.275
14. Babaei F, Ramalingam R, Tavendale A, Liang Y, Yan LSK, Ajuh P, et al. Novel blood collection method allows plasma proteome analysis from Single Zebrafish. J Proteome Res. (2013) 12:1580–90. doi: 10.1021/pr3009226
15. Lugert V, Steinhagen D, Reiser S. Lack of knowledge does not justify a lack of action: the case for animal welfare in farmed fish. J Sustainable Organic Agric Syst. (2020) 70:31–4. doi: 10.3220/LBF1592499937000
16. Sadoul B, Geffroy B. Measuring cortisol, the major stress hormone in fishes. J Fish Biol. (2019) 94:540–55. doi: 10.1111/jfb.13904
17. Broom DM. Indicators of poor welfare. Br Vet J. (1986) 142:524–6. doi: 10.1016/0007-1935(86)90109-0
18. Webster J. Animal Welfare. A Cool Eye Towards Eden. Blackwell Science (1995). Available online at: https://www.wiley.com/en-us/Animal+Welfare%3A+A+Cool+Eye+Towards+Eden-p-9780632039289 (accessed October 12, 2020).
19. Huntingford FA, Adams C, Braithwaite VA, Kadri S, Pottinger TG, Sandoe P, et al. Current issues in fish welfare. J Fish Biol. (2006) 68:332–72. doi: 10.1111/j.0022-1112.2006.001046.x
20. Chrousos GP. Stress and disorders of the stress system. Nat Rev Endocrinol. (2009) 5:374–81. doi: 10.1038/nrendo.2009.106
21. Wendelaar Bonga SE. The stress response in fish. Physiol Rev. (1997) 77:591–625. doi: 10.1152/physrev.1997.77.3.591
22. Schreck CB, Tort L, Farrell AP, Brauner CB. Biology of stress in fish. In: Schreck CB, Tort L, Farrell AP, Brauner C, editors. Biology of Stress in Fish. Cambridge, MA: Academic Press (2016). p. iii.
23. von Borell E. Stress and coping in farm animals. Arch Fur Tierzucht-Archives Anim Breed. (2000) 43:144–52. doi: 10.5194/aab-43-441-2000
24. Segner H, Sundh H, Buchmann K, Douxfils J, Sundell KS, Mathieu C, et al. Health of farmed fish: its relation to fish welfare and its utility as welfare indicator. Fish Physiol Biochem. (2012) 38:85–105. doi: 10.1007/s10695-011-9517-9
25. Broom DM. Behaviour and welfare in relation to pathology. Appl Anim Behav Sci. (2006) 97:73–83. doi: 10.1016/j.applanim.2005.11.019
26. Silbergeld EK. Blood glucose: a sensitive indicator of environmental stress in fish. Bull Environ Contam Toxicol. (1974) 11:20–5. doi: 10.1007/BF01685023
27. Sumpter JP, Dye HM, Benfey TJ. The effects of stress on plasma ACTH, α-MSH, and cortisol levels in salmonid fishes. Gen Comp Endocrinol. (1986) 62:377–85. doi: 10.1016/0016-6480(86)90047-X
28. van der Vyver JSF, Kaiser H, Potts WM, James N. Using blood plasma cortisol concentration and fish behaviour to determine temperature avoidance in the estuarine-dependent fish species Rhabdosargus holubi (Steindachner, 1881) (Sparidae). J Appl Ichthyol. (2013) 29:1275–8. doi: 10.1111/jai.12268
29. Martinez-Porchas M, Martinez-Cordova LT, Ramos-Enriquez R. Cortisol and glucose: reliable indicators of fish stress? J Aquat Sci. (2009) 4:158–78. Available online at: https://panamjas.org/pdf_artigos/PANAMJAS_4(2)_158-178.pdf
30. Noble C, Gismervik K, Iversen MH, Kolarevic J, Nilsson J, Stien LH, et al. (2018). Welfare Indicators for farmed Atlantic salmon: tools for assessing fish welfare An FHF-financed project, led by Nofima in partnership with. Available online at: www.nofima.no/fishwell/english (accessed October 12, 2020).
31. Moberg GP. Problems in defining stress and distress in animals. (1987). Available online at: https://www.semanticscholar.org/paper/Problems-in-defining-stress-and-distress-in-Moberg/fd97479bd5536a7842a3faf1135138aa8ff33a3d (accessed October 12, 2020).
32. Korytár T, Nipkow M, Altmann S, Goldammer T, Köllner B, Rebl A. Adverse husbandry of maraena whitefish directs the immune system to increase mobilization of myeloid cells and proinflammatory responses. Front Immunol. (2016) 7:631. doi: 10.3389/fimmu.2016.00631
33. Rebl A, Zebunke M, Borchel A, Bochert R, Verleih M, Goldammer T. Microarray-predicted marker genes and molecular pathways indicating crowding stress in rainbow trout (Oncorhynchus mykiss). Aquaculture. (2017) 473:355–65. doi: 10.1016/j.aquaculture.2017.03.003
34. Alfaro AC, Young T. Showcasing metabolomic applications in aquaculture: a review. Rev Aquac. (2018) 10:135–52. doi: 10.1111/raq.12152
35. Aluru N, Vijayan MM. Stress transcriptomics in fish: a role for genomic cortisol signaling. Gen Comp Endocrinol. (2009) 164:142–50. doi: 10.1016/j.ygcen.2009.03.020
36. Turnbull J, Bell A, Adams C. Stocking density and welfare of cage farmed Atlantic salmon: application of a multivariate analysis. Aquaculture. (2005) 243:121–32. doi: 10.1016/j.aquaculture.2004.09.022
37. Pettersen JM, Bracke MBM, Midtlyng PJ, Folkedal O, Stien LH, Steffenak H, et al. Salmon welfare index model 2.0: an extended model for overall welfare assessment of caged Atlantic salmon, based on a review of selected welfare indicators and intended for fish health professionals. Rev Aquac. (2014) 6:162–79. doi: 10.1111/raq.12039
38. Wiseman S, Osachoff H, Bassett E, Malhotra J, Bruno J, Vanaggelen G, et al. Gene expression pattern in the liver during recovery from an acute stressor in rainbow trout. Comp Biochem Physiol D Genom Proteomics. (2007) 2:234–44. doi: 10.1016/j.cbd.2007.04.005
39. Krasnov A, Afanasyev S, Nylund S, Rebl A. Multigene expression assay for assessment of the immune status of Atlantic Salmon. Genes. (2020) 11:111236. doi: 10.3390/genes11111236
40. DePasquale C, Wagner T, Archard GA, Ferguson B, Braithwaite VA. Learning rate and temperament in a high predation risk environment. Oecologia. (2014) 176:661–7. doi: 10.1007/s00442-014-3099-z
41. Baker MR, Goodman AC, Santo JB, Wong RY. Repeatability and reliability of exploratory behavior in proactive and reactive zebrafish, Danio rerio. Sci Rep. (2018) 8:12114. doi: 10.1038/s41598-018-30630-3
42. van de Nieuwegiessen PG, Olwo J, Khong S, Verreth JAJ, Schrama JW. Effects of age and stocking density on the welfare of African catfish, Clarias gariepinus Burchell. Aquaculture. (2009) 288:69–75. doi: 10.1016/j.aquaculture.2008.11.009
43. Topic Popovic N, Strunjak-Perovic I, Coz-Rakovac R, Barisic J, Jadan M, Berakovic AP, et al. Review Tricaine methane-sulfonate (MS-222) application in fish anaesthesia. J Appl Ichthyol. (2012) 28:553–64. doi: 10.1111/j.1439-0426.2012.01950.x
44. Uehara SA, Andrade DR, Takata R, Gomes Júnior AV, Vidal MV. The effectiveness of tricaine, benzocaine, clove oil, and menthol as anesthetics for lambari-bocarra Oligosarcus argenteus. Aquaculture. (2019) 502:326–31. doi: 10.1016/j.aquaculture.2018.12.054
45. Lawrence MJ, Raby GD, Teffer AK, Jeffries KM, Danylchuk AJ, Eliason EJ, et al. Best practices for non-lethal blood sampling of fish via the caudal vasculature. J Fish Biol. (2020) 97:4–15. doi: 10.1111/jfb.14339
46. Young T, Walker SP, Alfaro AC, Fletcher LM, Murray JS, Lulijwa R, et al. Impact of acute handling stress, anaesthesia, and euthanasia on fish plasma biochemistry: implications for veterinary screening and metabolomic sampling. Fish Physiol Biochem. (2019) 45:1485–94. doi: 10.1007/s10695-019-00669-8
47. Teixeira dos N S, Marques LS, Rodrigues RB, Gusso D, Fossati AAN, Streit DP. Effects of anesthetic tricaine on stress and reproductive aspects of South American silver catfish (Rhamdia quelen) male. bioRxiv. (2019) 759340. doi: 10.1101/759340
48. Molinero A, Gonzalez J. Comparative effects of MS 222 and 2-phenoxyethanol on gilthead sea bream (Sparus aurata L.) during confinement. Comp Biochem Physiol A Physiol. (1995) 111:405–14. doi: 10.1016/0300-9629(95)00037-8
49. Deebani A, Iyer N, Raman R, Jagadeeswaran P. Effect of MS222 on hemostasis in zebrafish. J Am Assoc Lab Anim Sci. (2019) 58:390–6. doi: 10.30802/AALAS-JAALAS-18-000069
50. Smith LS. Blood volumes of three salmonids. J Fish Res Board Canada. (1966) 23:1439–46. doi: 10.1139/f66-129
51. Tort L, González-Arch F, Torres P, Hidalgo J. On the blood volume of the Mediterranean dogfish, Scyliorhinus canicula. Fish Physiol Biochem. (1991) 9:173–7. doi: 10.1007/BF02265133
52. Olson KR. “Blood and extracellular fluid volume regulation: Role of the renin-angiotensin system, kallikrein-kinin system, and atrial natriuretic peptides,” in Fish Physiology (Elsevier Inc.), 135–254. doi: 10.1016/S1546-5098(08)60010-2
53. Noga EJ. “Major Cultured Species,” in Fish Disease (Ames, Iowa USA: Blackwell Publishing, Inc.), 1–8. doi: 10.1002/9781118786758.ch1
54. Chakraborty S, Rout SK, Anupama RR, Milli K, Sona RR, Behera L. Response of salinity (brine solution) induced stress on cortisol hormone in Indian major carp, Labeo rohita. J Exp Zool India. (2017) 20:1377–81. Available online at: http://www.connectjournals.com/toc.php?bookmark=CJ-033215&did=Supplement&volume=20&year=2017&issue_id=Supplement&issue_month=October
55. Bando K, Kawahara R, Kunimatsu T, Sakai J, Kimura J, Funabashi H, et al. Influences of biofluid sample collection and handling procedures on GC-MS based metabolomic studies. J Biosci Bioeng. (2010) 110:491–9. doi: 10.1016/j.jbiosc.2010.04.010
56. Duman M, Saticioglu IB, Suzer B, Altun S. Practices for drawing blood samples from teleost fish. N Am J Aquac. (2019) 81:119–25. doi: 10.1002/naaq.10077
57. Tuck MK, Chan DW, Chia D, Godwin AK, Grizzle WE, Krueger KE, et al. Standard operating procedures for serum and plasma collection: early detection research network consensus statement standard operating procedure integration working group. J Proteome Res. (2009) 8:113–7. doi: 10.1021/pr800545q
58. Barton BA, Iwama GK. Physiological changes in fish from stress in aquaculture with emphasis on the response and effects of corticosteroids. Annu Rev Fish Dis. (1991) 1:3–26. doi: 10.1016/0959-8030(91)90019-G
59. Barton BA. Stress in fishes: a diversity of responses with particular reference to changes in circulating corticosteroids. Integr Comp Biol. (2002) 42:517–25. doi: 10.1093/icb/42.3.517
60. Martos-Sitcha JA, Wunderink YS, Straatjes J, Skrzynska AK, Mancera JM, Martínez-Rodríguez G. Different stressors induce differential responses of the CRH-stress system in the gilthead sea bream (Sparus aurata). Comp Biochem Physiol A Mol Integr Physiol. (2014) 177:49–61. doi: 10.1016/j.cbpa.2014.07.021
61. Wu H, Ohnuki H, Hibi K, Ren H, Endo H. Development of a label-free immunosensor system for detecting plasma cortisol levels in fish. Fish Physiol Biochem. (2016) 42:19–27. doi: 10.1007/s10695-015-0113-2
62. Blahová J, Dobšíková R, Svobodová Z, Kaláb P. Simultaneous determination of plasma cortisol by high pressure liquid chromatography and radioimmunoassay methods in fish. Acta Vet Brno. (2007) 76:59–64. doi: 10.2754/avb200776010059
63. Raposo De Magalhães C, Schrama D, Farinha AP, Revets D, Kuehn A, et al. Protein changes as robust signatures of fish chronic stress: a proteomics approach to fish welfare research. BMC Genom. (2020) 21:309. doi: 10.1186/s12864-020-6728-4
64. Tschmelak J, Proll G, Gauglitz G. Verification of performance with the automated direct optical TIRF immunosensor (River Analyser) in single and multi-analyte assays with real water samples. Biosens Bioelectron. (2004) 20:743–52. doi: 10.1016/j.bios.2004.04.006
65. Ainsworth AJ, Dexiang C, Waterstrat PR. Changes in peripheral blood leukocyte percentages and function of neutrophils in stressed channel catfish. J Aquat Anim Health. (1991) 3:41–7. doi: 10.1577/1548-8667(1991)003<0041:CIPBLP>2.3.CO;2
66. Martemyanov VI. Patterns of changes in sodium content in plasma and erythrocytes of freshwater fish at stress. J Ichthyol. (2013) 53:220–4. doi: 10.1134/S0032945213020094
67. Karakach TK, Huenupi EC, Soo EC, Walter JA, Afonso LOB. 1H-NMR and mass spectrometric characterization of the metabolic response of juvenile Atlantic salmon (Salmo salar) to long-term handling stress. Metabolomics. (2009) 5:123–37. doi: 10.1007/s11306-008-0144-0
68. Liu X, Afonso L, Altman E, Johnson S, Brown L, Li J. O-acetylation of sialic acids in N-glycans of Atlantic salmon (Salmo salar) serum is altered by handling stress. Proteomics. (2008) 8:2849–57. doi: 10.1002/pmic.200701093
69. Pickering AD, Pottinger TG, Christie P. Recovery of the brown trout, Salmo trutta L., from acute handling stress: a time-course study. J Fish Biol. (1982) 20:229–44. doi: 10.1111/j.1095-8649.1982.tb03923.x
70. Costas B, Conceição LEC, Aragão C, Martos JA, Ruiz-Jarabo I, Mancera JM, et al. Physiological responses of Senegalese sole (Solea senegalensis Kaup, 1858) after stress challenge: effects on non-specific immune parameters, plasma free amino acids and energy metabolism. Aquaculture. (2011) 316:68–76. doi: 10.1016/j.aquaculture.2011.03.011
71. Casillas E, Smith LS. Effect of stress on blood coagulation and haematology in rainbow trout (Salmo gairdneri). J Fish Biol. (1977) 10:481–91. doi: 10.1111/j.1095-8649.1977.tb04081.x
72. Stone DAJ, Gaylord TG, Johansen KA, Overturf K, Sealey WM, Hardy RW. Evaluation of the effects of repeated fecal collection by manual stripping on the plasma cortisol levels, TNF-α gene expression, and digestibility and availability of nutrients from hydrolyzed poultry and egg meal by rainbow trout, Oncorhynchus mykiss (Walbaum). Aquaculture. (2008) 275:250–9. doi: 10.1016/j.aquaculture.2008.01.003
73. Pérez-Casanova JC, Rise ML, Dixon B, Afonso LOB, Hall JR, Johnson SC, et al. The immune and stress responses of Atlantic cod to long-term increases in water temperature. Fish Shellfish Immunol. (2008) 24:600–9. doi: 10.1016/j.fsi.2008.01.012
74. Basu M, Paichha M, Swain B, Lenka SS, Singh S, Chakrabarti R, et al. Modulation of TLR2, TLR4, TLR5, NOD1 and NOD2 receptor gene expressions and their downstream signaling molecules following thermal stress in the Indian major carp catla (Catla catla). Biotech. (2015) 5:1021–30. doi: 10.1007/s13205-015-0306-5
75. Roche H, Bogé G. Fish blood parameters as a potential tool for identification of stress caused by environmental factors and chemical intoxication. Mar Environ Res. (1996) 41:27–43. doi: 10.1016/0141-1136(95)00015-1
76. Pinto RD, Nascimento DS, Reis MIR, do Vale A, dos Santos NMS. Molecular characterization, 3D modelling and expression analysis of sea bass (Dicentrarchus labrax L.) interleukin-10. Mol Immunol. (2007) 44:2056–65. doi: 10.1016/j.molimm.2006.09.014
77. Cui M, Zhang Q, Yao Z, Zhang Z, Zhang H, Wang Y. Immunoglobulin M gene expression analysis of orange-spotted grouper, Epinephelus coioides, following heat shock and Vibrio alginolyticus challenge. Fish Shellfish Immunol. (2010) 29:1060–5. doi: 10.1016/j.fsi.2010.08.018
78. Delaney MA, Klesius PH. Hypoxic conditions induce Hsp70 production in blood, brain and head kidney of juvenile Nile tilapia Oreochromis niloticus (L.). Aquaculture. (2004) 236:633–44. doi: 10.1016/j.aquaculture.2004.02.025
79. Basu M, Paichha M, Lenka SS, Chakrabarty R, Samanta M. Hypoxic stress: impact on the modulation of TLR2, TLR4, NOD1 and NOD2 receptor and their down-stream signalling genes expression in catla (Catla catla). Mol Biol Rep. (2016) 43:1–9. doi: 10.1007/s11033-015-3932-4
80. Bermejo-Nogales A, Calduch-Giner JA, Pérez-Sánchez J. Tissue-specific gene expression and functional regulation of uncoupling protein 2 (UCP2) by hypoxia and nutrient availability in gilthead sea bream (Sparus aurata): implications on the physiological significance of UCP1-3 variants. Fish Physiol Biochem. (2014) 40:751–62. doi: 10.1007/s10695-013-9882-7
81. Martos-Sitcha JA, Bermejo-Nogales A, Calduch-Giner JA, Pérez-Sánchez J. Gene expression profiling of whole blood cells supports a more efficient mitochondrial respiration in hypoxia-challenged gilthead sea bream (Sparus aurata). Front Zool. (2017) 14:1–12. doi: 10.1186/s12983-017-0220-2
82. Martos-Sitcha JA, Simó-Mirabet P, de las Heras V, Calduch-Giner JÀ, Pérez-Sánchez J. Tissue- specific orchestration of gilthead sea bream resilience to hypoxia and high stocking density. Front Physiol. (2019) 10:1–18. doi: 10.3389/fphys.2019.00840
83. Liu B, Liu Y, Wang X. The effect of stocking density on growth and seven physiological parameters with assessment of their potential as stress response indicators for the Atlantic salmon (Salmo salar). Mar Freshw Behav Physiol. (2015) 48:177–92. doi: 10.1080/10236244.2015.1034956
84. Montero D, Tort L, Robaina L, Vergara JM, Izquierdo MS. Low vitamin E in diet reduces stress resistance of gilthead seabream (Sparus aurata) juveniles. Fish Shellfish Immunol. (2001) 11:473–90. doi: 10.1006/fsim.2000.0324
85. North B, Turnbull J, Ellis T. The impact of stocking density on the welfare of rainbow trout (Oncorhynchus mykiss). Aquaculture. (2006) 255:466–79. doi: 10.1016/j.aquaculture.2006.01.004
86. Yarahmadi P, Miandare HK, Hoseinifar SH, Gheysvandi N, Akbarzadeh A. The effects of stocking density on hemato-immunological and serum biochemical parameters of rainbow trout (Oncorhynchus mykiss). Aquac Int. (2014) 23:55–63. doi: 10.1007/s10499-014-9797-z
87. Conde-Sieira M, Aguilar AJ, López-Patiño MA, Míguez JM, Soengas JL. Stress alters food intake and glucosensing response in hypothalamus, hindbrain, liver, and Brockmann bodies of rainbow trout. Physiol Behav. (2010) 101:483–93. doi: 10.1016/j.physbeh.2010.07.016
88. Vijayan MM, Ballantyne JS, Leatherland JF. High stocking density alters the energy metabolism of brook charr, Salvelinus fontinalis. Aquaculture. (1990) 88:371–81. doi: 10.1016/0044-8486(90)90162-G
89. Kullgren A, Samuelsson LM, Larsson DGJ, Björnsson BT, Bergman EJ. A metabolomics approach to elucidate effects of food deprivation in juvenile rainbow trout (Oncorhynchus mykiss). Am J Physiol. (2010) 299:1440–8. doi: 10.1152/ajpregu.00281.2010
90. Roques S, Deborde C, Richard N, Marchand Y, Larroquet L, Prigent S, et al. Proton-NMR Metabolomics of rainbow trout fed a plant-based diet supplemented with graded levels of a protein-rich yeast fraction reveal several metabolic processes involved in growth. J Nutr. (2020) 150:2268–77. doi: 10.1093/jn/nxaa206
91. Dawood MAO, El-Salam Metwally A, Elkomy AH, Gewaily MS, Abdo SE, Abdel-Razek MAS, Soliman AA, Amer AA, Abdel-Razik NI, Abdel-Latif HMR, et al. The impact of menthol essential oil against inflammation, immunosuppression, and histopathological alterations induced by chlorpyrifos in Nile tilapia. Fish Shellfish Immunol (2020) 102:316–25. doi: 10.1016/j.fsi.2020.04.059
92. Hoseini SM, Hoseinifar SH, Van Doan H. Growth performance and hematological and antioxidant characteristics of rainbow trout, Oncorhynchus mykiss, fed diets supplemented with Roselle, Hibiscus sabdariffa. Aquaculture. (2021) 530:735827. doi: 10.1016/j.aquaculture.2020.735827
93. Enis Yonar M, Mişe Yonar S, Silici S. Protective effect of propolis against oxidative stress and immunosuppression induced by oxytetracycline in rainbow trout (Oncorhynchus mykiss, W.). Fish Shellfish Immunol. (2011) 31:318–25. doi: 10.1016/j.fsi.2011.05.019
94. Zhang M, Yin X, Li M, Wang R, Qian Y, Hong M. Effect of nitrite exposure on haematological status, oxidative stress, immune response and apoptosis in yellow catfish (Pelteobagrus fulvidraco). Comp Biochem Physiol C Toxicol Pharmacol. (2020) 238:108867. doi: 10.1016/j.cbpc.2020.108867
95. Guimarães ATB, Estrela FN, Pereira PS, de Andrade Vieira JE, de Lima Rodrigues AS, Silva FG, et al. Toxicity of polystyrene nanoplastics in Ctenopharyngodon idella juveniles: a genotoxic, mutagenic and cytotoxic perspective. Sci Total Environ. (2021) 752:141937. doi: 10.1016/j.scitotenv.2020.141937
96. Markkula E, Salo HM, Rikalainen K, Jokinen IE. Long-term UVB irradiation affects the immune functions of carp (Cyprinus carpio) and rainbow trout (Oncorhynchus mykiss). Photochem Photobiol. (2009) 85:347–52. doi: 10.1111/j.1751-1097.2008.00446.x
97. De Souza KB, Jutfelt F, Kling P, Förlin L, Sturve J. Effects of increased CO2 on fish gill and plasma proteome. PLoS ONE. (2014) 9:102901. doi: 10.1371/journal.pone.0102901
98. Archard GA, Braithwaite VA. Increased exposure to predators increases both exploration and activity level in Brachyrhaphis episcopi. J Fish Biol. (2011) 78:593–601. doi: 10.1111/j.1095-8649.2010.02880.x
99. Acerete L, Balasch J, Espinosa E, Josa A, Tort L. Physiological responses in Eurasian perch (Perca fluviatilis, L.) subjected to stress by transport and handling. Aquaculture. (2004) 237:167–78. doi: 10.1016/j.aquaculture.2004.03.018
100. Ashley PJ. Fish welfare: current issues in aquaculture. Appl Anim Behav Sci. (2007) 104:199–235. doi: 10.1016/j.applanim.2006.09.001
101. Arends RJ, Mancera JM, Muñoz JL, Wendelaar Bonga SE, Flik G. The stress response of the gilthead sea bream (Sparus aurata L.) to air exposure and confinement. J Endocrinol. (1999) 163:149–57. doi: 10.1677/joe.0.1630149
102. Molinero A, Gomez E, Balasch J, Tort L. Stress by fish removal in the Gilthead Sea Bream, Sparus aurata: a time course study on the remaining fish in the same tank. J Appl Aquac. (1997) 7:1–12. doi: 10.1300/J028v07n02_01
103. Di Marco P, Priori A, Finoia MG, Massari A, Mandich A, Marino G. Physiological responses of European sea bass Dicentrarchus labrax to different stocking densities and acute stress challenge. Aquaculture. (2008) 275:319–28. doi: 10.1016/j.aquaculture.2007.12.012
104. Sánchez-Muros MJ, Villacreces S, Miranda-de la Lama G, de Haro C, García-Barroso F. Effects of chemical and handling exposure on fatty acids, oxidative stress and morphological welfare indicators in gilt-head sea bream (Sparus aurata). Fish Physiol Biochem. (2013) 39:581–91. doi: 10.1007/s10695-012-9721-2
105. Assem H, Khalifa A, ELSalhia M. Physiological and microbiological indices as indicators of evaluating dietary fungi degraded date pits as a probiotic for cultured Nile tilapia Oreochromis niloticus fingerling and its effect on fish welfare. Egypt J Aquat Res. (2014) 40:435–41. doi: 10.1016/j.ejar.2014.10.004
106. Ellis T, Yildiz HY, López-Olmeda J, Spedicato MT, Tort L, Øverli Ø, et al. Cortisol and finfish welfare. Fish Physiol Biochem. (2012) 38:163–88. doi: 10.1007/s10695-011-9568-y
107. López-Patiño MA, Gesto M, Conde-Sieira M, Soengas JL, Míguez JM. Stress inhibition of melatonin synthesis in the pineal organ of rainbow trout (Oncorhynchus mykiss) is mediated by cortisol. J Exp Biol. (2014) 217:1407–16. doi: 10.1242/jeb.087916
108. Manuel R, Boerrigter JGJ, Cloosterman M, Gorissen M, Flik G, van den Bos R, et al. Effects of acute stress on aggression and the cortisol response in the African sharptooth catfish Clarias gariepinus: differences between day and night. J Fish Biol. (2016) 88:2175–87. doi: 10.1111/jfb.12989
109. Almazan-Rueda P, van Helmond ATM, Verreth JAJ, Schrama JW. Photoperiod affects growth, behaviour and stress variables in Clarias gariepinus. J Fish Biol. (2005) 67:1029–39. doi: 10.1111/j.0022-1112.2005.00806.x
110. Pottinger TG, Carrick TR. Modification of the plasma cortisol response to stress in rainbow trout by selective breeding. Gen Comp Endocrinol. (1999) 116:122–32. doi: 10.1006/gcen.1999.7355
111. Pottinger TG, Moran TA, Morgan JAW. Primary and secondary indices of stress in the progeny of rainbow trout (Oncorhynchus mykiss) selected for high and low responsiveness to stress. J Fish Biol. (1994) 44:149–63. doi: 10.1111/j.1095-8649.1994.tb01591.x
112. Ellis T, Berrill I, Lines J, Turnbull JF, Knowles TG. Mortality and fish welfare. Fish Physiol Biochem. (2012) 38:189–99. doi: 10.1007/s10695-011-9547-3
113. Blaxhall PC, Daisley KW. Routine haematological methods for use with fish blood. J Fish Biol. (1973) 5:771–81. doi: 10.1111/j.1095-8649.1973.tb04510.x
114. Pearson MP, Stevens ED. Size and hematological impact of the splenic erythrocyte reservoir in rainbow trout, Oncorhynchus mykiss. Fish Physiol Biochem. (1991) 9:39–50. doi: 10.1007/BF01987610
115. Ashaf-Ud-Doulah M, Mamun AAl, Rahman ML, Islam SMM, Jannat R, Hossain MAR, et al. High temperature acclimation alters upper thermal limits and growth performance of Indian major carp, rohu, Labeo rohita (Hamilton, 1822). J Therm Biol. (2020) 93:102738. doi: 10.1016/j.jtherbio.2020.102738
116. Wojtaszek J, Dziewulska-Szwajkowska D, Łozińska-Gabska M, Adamowicz A, Dzugaj A. hematological effects of high dose of cortisol on the carp (Cyprinus carpio L.): cortisol effect on the carp blood. Gen Comp Endocrinol. (2002) 125:176–83. doi: 10.1006/gcen.2001.7725
117. Pulsford AL, Lemaire-Gony S, Tomlinson M, Collingwood N, Glynn PJ. Effects of acute stress on the immune system of the dab, Limanda limanda. Comp Biochem Physiol C Pharmacol Toxicol Endocrinol. (1994) 109:129–39. doi: 10.1016/0742-8413(94)00053-D
118. Ainsworth AJ. Fish granulocytes: morphology, distribution, and function. Annu Rev Fish Dis. (1992) 2:123–48. doi: 10.1016/0959-8030(92)90060-B
119. Balla KM, Lugo-Villarino G, Spitsbergen JM, Stachura DL, Hu Y, Bañuelos K, et al. Eosinophils in the zebrafish: prospective isolation, characterization, and eosinophilia induction by helminth determinants. Blood. (2010) 116:3944–54. doi: 10.1182/blood-2010-03-267419
120. Nakada K, Fujisawa K, Horiuchi H, Furusawa S. Studies on morphology and cytochemistry in blood cells of ayu Plecoglossus altivelis altivelis. J Vet Med Sci. (2014) 76:693–704. doi: 10.1292/jvms.13-0584
121. Hine PM. The granulocytes of fish. Fish Shellfish Immunol. (1992) 2:79–98. doi: 10.1016/S1050-4648(05)80038-5
122. Dikić D, Lisičí D, Matić-Skoko S, Tutman P, Skaramuca D, Franić Z, et al. Comparative hematology of wild Anguilliformes (Muraena helena, L. 1758, Conger conger, L. 1758 and Anguilla anguilla L. 1758). Anim Biol. (2013) 63:77–92. doi: 10.1163/15707563-00002395
123. Silva MB da, Fraga RE, Nishiyama PB, Silva da ISS, Costa NLB, de Oliveira LAA, et al. Leukocyte profiles in Odontophrynus carvalhoi (Amphibia: Odontophrynidae) tadpoles exposed to organophosphate chlorpyrifos pesticides. Water Air Soil Pollut. (2020) 231:372. doi: 10.1007/s11270-020-04726-4
124. Weyts FA, Verburg-van Kemenade BM, Flik G. Characterisation of glucocorticoid receptors in peripheral blood leukocytes of Carp, Cyprinus carpio L. Gen Comp Endocrinol. (1998) 111:1–8. doi: 10.1006/gcen.1998.7080
125. Ortuño J, Esteban MA, Meseguer J. Effects of short-term crowding stress on the gilthead seabream (Sparus aurata L) innate immune response. Fish Shellfish Immunol. (2001) 11:187–97. doi: 10.1006/fsim.2000.0304
126. Pickering AD. Cortisol-induced lymphocytopenia in brown trout, Salmo trutta L. Gen Comp Endocrinol. (1984) 53:252–9. doi: 10.1016/0016-6480(84)90250-8
127. Weyts FAA, Cohen N, Flik G, Verburg-van Kemenade BML. Interactions between the immune system and the hypothalamo-pituitary-interrenal axis in fish. Fish Shellfish Immunol. (1999) 9:1–20. doi: 10.1006/fsim.1998.0170
128. Davis AK, Maney DL. The use of glucocorticoid hormones or leucocyte profiles to measure stress in vertebrates: what's the difference? Methods Ecol Evol. (2018) 9:1556–68. doi: 10.1111/2041-210X.13020
129. Frank MG, Watkins LR, Maier SF. Stress-induced glucocorticoids as a neuroendocrine alarm signal of danger. Brain Behav Immun. (2013) 33:1–6. doi: 10.1016/j.bbi.2013.02.004
130. Goessling JM, Kennedy H, Mendonça MT, Wilson AE. A meta-analysis of plasma corticosterone and heterophil: lymphocyte ratios - is there conservation of physiological stress responses over time? Funct Ecol. (2015) 29:1189–96. doi: 10.1111/1365-2435.12442
131. Katakura F, Nishiya K, Wentzel AS, Hino E, Miyamae J, Okano M, et al. Paralogs of common carp granulocyte colony-stimulating factor (G-CSF) have different functions regarding development, trafficking and activation of neutrophils. Front Immunol. (2019) 10:255. doi: 10.3389/fimmu.2019.00255
132. Kepka M, Verburg-van Kemenade BML, Chadzinska M. Neuroendocrine modulation of the inflammatory response in common carp: adrenaline regulates leukocyte profile and activity. Gen Comp Endocrinol. (2013) 188:102–9. doi: 10.1016/j.ygcen.2012.11.014
133. Vinodhini R, Narayanan M. The impact of toxic heavy metals on the hematological parameters in common carp (Cyprinus carpio l.). Iran J Environ Heal Sci Eng. (2009) 6:23–8. Available online at: https://www.semanticscholar.org/paper/THE-IMPACT-OF-TOXIC-HEAVY-METALS-ON-THE-PARAMETERS-Vinodhini-Narayanan/5390407c1f96e4ea1c3457c19485d12fe3546449
134. Oluah NS, Aguzie IO, Ekechukwu NE, Madu JC, Ngene CI, Oluah C. Hematological and immunological responses in the African catfish Clarias gairepinus exposed to sublethal concentrations of herbicide Ronstar®. Ecotoxicol Environ Saf. (2020) 201:110824. doi: 10.1016/j.ecoenv.2020.110824
135. Delfosse C, Pageat P, Lafont-Lecuelle C, Asproni P, Chabaud C, Cozzi A, et al. Effect of handling and crowding on the susceptibility of Atlantic salmon (Salmo salar L.) to Lepeophtheirus salmonis (Krøyer) copepodids. J Fish Dis. (2020) 6:jfd.13286. doi: 10.1111/jfd.13286
136. Gansner JM, Leung AD, Superdock M, Blair MC, Ammerman MB, Durand EM, et al. Sorting zebrafish thrombocyte lineage cells with a Cd41 monoclonal antibody enriches hematopoietic stem cell activity. Blood. (2017) 129:1394–7. doi: 10.1182/blood-2016-12-759993
137. Morgan JAW, Pottinger TG, Rippon P. Evaluation of flow cytometry as a method for quantification of circulating blood cell populations in salmonid fish. J Fish Biol. (1993) 42:131–41. doi: 10.1111/j.1095-8649.1993.tb00311.x
138. Ellsaesser CF, Clem LW. Haematological and immunological changes in channel catfish stressed by handling and transport. J Fish Biol. (1986) 28:511–21. doi: 10.1111/j.1095-8649.1986.tb05187.x
139. Ali AO, Hohn C, Allen PJ, Ford L, Dail MB, Pruett S, et al. The effects of oil exposure on peripheral blood leukocytes and splenic melano-macrophage centers of Gulf of Mexico fishes. Mar Pollut Bull. (2014) 79:87–93. doi: 10.1016/j.marpolbul.2013.12.036
140. Sopinka NM, Donaldson MR, O'Connor CM, Suski CD, Cooke SJ. Stress indicators in fish. In: Schreck CB, Tort L, Farrell AP, Brauner CJ, editors. Biology of Stress in Fish (Amsterdam: Elsevier Inc.). p. 405–62. doi: 10.1016/B978-0-12-802728-8.00011
141. Rand PW, Lacombe E, Hunt HE, Austin WH. Viscosity of normal human blood under normothermic and hypothermic conditions. J Appl Physiol. (1964) 19:117–22. doi: 10.1152/jappl.1964.19.1.117
142. Wells RMG, Weber RE. Is there an optimal haematocrit for rainbow trout, Oncorhynchus mykiss (Walbaum)? An interpretation of recent data based on blood viscosity measurements. J Fish Biol. (1991) 38:53–65. doi: 10.1111/j.1095-8649.1991.tb03090.x
143. Sandnes K, Lie O, Waagbo R. Normal ranges of some blood chemistry parameters in adult farmed Atlantic salmon, Salmo salar. J Fish Biol. (1988) 32:129–36. doi: 10.1111/j.1095-8649.1988.tb05341.x
144. Sambraus F, Olsen RE, Remen M, Hansen TJ, Torgersen T, Fjelldal PG. Water temperature and oxygen: the effect of triploidy on performance and metabolism in farmed Atlantic salmon (Salmo salar L.) post-smolts. Aquaculture. (2017) 473:1–12. doi: 10.1016/j.aquaculture.2017.01.024
145. Iversen M, Finstad B, Nilssen KJ. Recovery from loading and transport stress in Atlantic salmon (Salmo salar L.) smolts. Aquaculture. (1998) 168:387–94. doi: 10.1016/S0044-8486(98)00364-0
146. Putnam RW, Freel RW. Hematological parameters of five species of marine fishes. Comp Biochem Physiol Part A Physiol (1978) 61:585–8. doi: 10.1016/0300-9629(78)90132-9
147. Jawad LA, Al-Mukhtar MA, Ahmed HK. The relationship between haematocrit and some biological parameters of the Indian shad, Tenualosa ilisha (Family Clupeidae). Anim Biodivers Conserv. (2004) 27:47–52. Available online at: http://abc.museucienciesjournals.cat/volume-27-2-2004-abc/the-relationship-between-haematocrit-and-some-biological-parameters-of-the-indian-shad-tenualosa-ilisha-family-clupeidae-2/?lang=en
148. Wells RMG, Davie PS. Oxygen binding by the blood and hematological effects of capture stress in two big gamefish: Mako shark and striped marlin. Comp Biochem Physiol A Physiol. (1985) 81:643–6. doi: 10.1016/0300-9629(85)91041-2
149. Phuong LM, Damsgaard C, Huong DTT, Ishimatsu A, Wang T, Bayley M. Recovery of blood gases and haematological parameters upon anaesthesia with benzocaine, MS-222 or Aqui-S in the air-breathing catfish Pangasianodon hypophthalmus. Ichthyol Res. (2017) 64:84–92. doi: 10.1007/s10228-016-0545-4
150. Witeska M. Anemia in teleost fishes. Bull Eur Assoc Fish Pathol. (2015) 35:148–60. Available online at: https://eafp.org/download/2015-volume35/issue_4/35-4-148-witeska.pdf
151. Krasnov A, Timmerhaus G, Afanasyev S, Takle H, Jørgensen SM. Induced erythropoiesis during acute anemia in Atlantic salmon: a transcriptomic survey. Gen Comp Endocrinol. (2013) 192:181–90. doi: 10.1016/j.ygcen.2013.04.026
152. Islam MJ, Kunzmann A, Thiele R, Slater MJ. Effects of extreme ambient temperature in European seabass, Dicentrarchus labrax acclimated at different salinities: growth performance, metabolic and molecular stress responses. Sci Total Environ. (2020) 735:139371. doi: 10.1016/j.scitotenv.2020.139371
153. Varsamos S, Flik G, Pepin JF, Bonga SEW, Breuil G. Husbandry stress during early life stages affects the stress response and health status of juvenile sea bass, Dicentrarchus labrax. Fish Shellfish Immunol. (2006) 20:83–96. doi: 10.1016/j.fsi.2005.04.005
154. Whittamore JM. Osmoregulation and epithelial water transport: lessons from the intestine of marine teleost fish. J Comp Physiol B. (2012) 182:1–39. doi: 10.1007/s00360-011-0601-3
155. Veiseth E, Fjaera SO, Bjerkeng B, Skjervold PO. Accelerated recovery of Atlantic salmon (Salmo salar) from effects of crowding by swimming. Comp Biochem Physiol B Biochem Mol Biol. (2006) 144:351–8. doi: 10.1016/j.cbpb.2006.03.009
156. Robertson L, Thomas P, Arnold CR, Trant JM. Plasma cortisol and secondary stress responses of red drum to handling, transport, rearing density, and a disease outbreak. Progress Fish-Culturist. (1987) 49:1–12. doi: 10.1577/1548-8640(1987)49<1:PCASSR>2.0.CO;2
157. Liebert AM, Schreck CB. Effects of acute stress on osmoregulation, feed intake, IGF-1, and cortisol in yearling steelhead trout (Oncorhynchus mykiss) during seawater adaptation. Gen Comp Endocrinol. (2006) 148:195–202. doi: 10.1016/j.ygcen.2006.03.002
158. Cataldi E, Di Marco P, Mandich A, Cataudella S. Serum parameters of Adriatic sturgeon Acipenser naccarii (Pisces: Acipenseriformes): effects of temperature and stress. Comp Biochem Physiol A Mol Integr Physiol. (1998) 121:351–4. doi: 10.1016/S1095-6433(98)10134-4
159. Lim HK, Hur JW. Effects of acute and chronic air exposure on growth and stress response of juvenile olive flounder, Paralichthys olivaceus. Turkish J Fish Aquat Sci. (2018) 18:143–51. doi: 10.4194/1303-2712-v18_1_16
160. Randall DJ, Baumgarten D, Malyusz M. The relationship between gas and ion transfer across the gills of fishes. Comp Biochem Physiol A Physiol. (1972) 41:629–37. doi: 10.1016/0300-9629(72)90017-5
161. Onukwufor JO, Wood CM. The osmorespiratory compromise in rainbow trout (Oncorhynchus mykiss): the effects of fish size, hypoxia, temperature and strenuous exercise on gill diffusive water fluxes and sodium net loss rates. Comp Biochem Physiol A Mol Integr Physiol. (2018) 219–220:10–8. doi: 10.1016/j.cbpa.2018.02.002
162. Giacomin M, Bryant HJ, Val AL, Schulte PM, Wood CM. The osmorespiratory compromise: physiological responses and tolerance to hypoxia are affected by salinity acclimation in the euryhaline Atlantic killifish (Fundulus heteroclitus). J Exp Biol. (2019) 222:206599. doi: 10.1242/jeb.206599
163. Damsgaard C, McGrath M, Wood CM, Richards JG, Brauner CJ. Ion-regulation, acid/base-balance, kidney function, and effects of hypoxia in coho salmon, Oncorhynchus kisutch, after long-term acclimation to different salinities. Aquaculture. (2020) 528:735571. doi: 10.1016/j.aquaculture.2020.735571
164. Robertson LM, Val AL, Almeida-Val VF, Wood CM. Ionoregulatory aspects of the osmorespiratory compromise during acute environmental hypoxia in 12 tropical and temperate teleosts. Physiol Biochem Zool. (2015) 88:357–70. doi: 10.1086/681265
165. Fiess JC, Kunkel-Patterson A, Mathias L, Riley LG, Yancey PH, Hirano T, et al. Effects of environmental salinity and temperature on osmoregulatory ability, organic osmolytes, and plasma hormone profiles in the Mozambique tilapia (Oreochromis mossambicus). Comp Biochem Physiol A Mol Integr Physiol. (2007) 146:252–64. doi: 10.1016/j.cbpa.2006.10.027
166. Barton BA, Zitzow RE. Physiological responses of juvenile walleyes to handling stress with recovery in saline water. Progress Fish-Culturist. (1995) 57:267–76. doi: 10.1577/1548-8640(1995)057<0267:PROJWT>2.3.CO;2
167. Nakamura M, Watanabe S, Kaneko T, Masuda R, Tsukamoto K, Otake T. Limited adaptation to non-natal osmotic environments at high water temperature in euryhaline wanderer fishes. Environ Biol Fishes. (2020) 103:137–45. doi: 10.1007/s10641-019-00940-0
168. Barton BA, Ribas L, Acerete L, Tort L. Effects of chronic confinement on physiological responses of juvenile gilthead sea bream, Sparus aurata L., to acute handling. Aquac Res. (2005) 36:172–9. doi: 10.1111/j.1365-2109.2004.01202.x
169. Giacomin M, Dal Pont G, Eom J, Schulte PM, Wood CM. The effects of salinity and hypoxia exposure on oxygen consumption, ventilation, diffusive water exchange and ionoregulation in the Pacific hagfish (Eptatretus stoutii). Comp Biochem Physiol A Mol Integr Physiol. (2019) 232:47–59. doi: 10.1016/j.cbpa.2019.03.007
170. Alloy MM, Cartolano MC, Sundaram R, Plotnikova A, McDonald MD. Exposure and recovery of the gulf toadfish (Opsanus beta) to weathered Deepwater Horizon slick oil: impacts on liver and blood endpoints. Environ Toxicol Chem. (2020) etc.4966. doi: 10.1002/etc.4966. [Epub ahead of print].
171. Tort L. Stress and immune modulation in fish. Dev Comp Immunol. (2011) 35:1366–75. doi: 10.1016/j.dci.2011.07.002
172. Nakanishi T. Seasonal changes in the humoral immune response and the lymphoid tissues of the marine teleost, Sebastiscus marmoratus. Vet Immunol Immunopathol. (1986) 12:213–21. doi: 10.1016/0165-2427(86)90125-X
173. Trust TJ, Courtice ID, Khouri AG, Crosa JH, Schiewe MH. Serum resistance and hemagglutination ability of marine vibrios pathogenic for fish. Infect Immun. (1981) 34:702–7. doi: 10.1128/IAI.34.3.702-707.1981
174. Ourth DD, Wilson EA. Bactericidal serum response of the channel catfish against Gram-negative bacteria. Dev Comp Immunol. (1982) 6:579–83. doi: 10.1016/S0145-305X(82)80044-X
175. Nagai T, Nakai T. Growth of Flavobacterium psychrophilum in fish serum correlates with pathogenicity. J Fish Dis. (2011) 34:303–10. doi: 10.1111/j.1365-2761.2011.01245.x
176. Jiang X-F, Liu Z-F, Lin A-F, Xiang L-X, Shao J-Z. Coordination of bactericidal and iron regulatory functions of hepcidin in innate antimicrobial immunity in a zebrafish model. Sci Rep. (2017) 7:4265. doi: 10.1038/s41598-017-04069-x
177. Rebl A, Korytár T, Borchel A, Bochert R, Strzelczyk JE, Goldammer T, et al. The synergistic interaction of thermal stress coupled with overstocking strongly modulates the transcriptomic activity and immune capacity of rainbow trout (Oncorhynchus mykiss). Sci Rep. (2020) 10:1–15. doi: 10.1038/s41598-020-71852-8
178. Magnadottir B, Lange S, Gudmundsdottir S, Bøgwald J, Dalmo RA. Ontogeny of humoral immune parameters in fish. Fish Shellfish Immunol. (2005) 19:429–39. doi: 10.1016/j.fsi.2005.03.010
179. Saurabh S, Sahoo PK. Lysozyme: an important defence molecule of fish innate immune system. Aquac Res. (2008) 39:223–39. doi: 10.1111/j.1365-2109.2007.01883.x
180. Sunyer JO, Boshra H, Lorenzo G, Parra D, Freedman B, Bosch N. Evolution of complement as an effector system in innate and adaptive immunity. Immunol Res. (2003) 27:549–64. doi: 10.1385/IR:27:2–3:549
181. Demers NE, Bayne CJ. The immediate effects of stress on hormones and plasma lysozyme in rainbow trout. Dev Comp Immunol. (1997) 21:363–73. doi: 10.1016/S0145-305X(97)00009-8
182. Sunyer JO, Tort L, Lambris JD. Structural C3 diversity in fish: characterization of five forms of C3 in the diploid fish Sparus aurata. J Immunol. (1997) 158:2813–21.
183. Lie Ø, Evensen Ø, Sørensen A, Frøysadal E. Study on lysozyme activity in some fish species. Dis Aquat Organ. (1989) 6:1–5. doi: 10.3354/dao006001
184. Goshima M, Sekiguchi R, Matsushita M, Nonaka M. The complement system of elasmobranches revealed by liver transcriptome analysis of a hammerhead shark, Sphyrna zygaena. Dev Comp Immunol. (2016) 61:13–24. doi: 10.1016/j.dci.2016.03.009
185. Köbis JM, Rebl A, Kühn C, Korytár T, Köllner B, Goldammer T. Comprehensive and comparative transcription analyses of the complement pathway in rainbow trout. Fish Shellfish Immunol. (2015) 42:98–107. doi: 10.1016/j.fsi.2014.10.032
186. Parry RM, Chandan RC, Shahani KM. A rapid and sensitive assay of muramidase. Proc Soc Exp Biol Med. (1965) 119:384–6. doi: 10.3181/00379727-119-30188
187. Osserman EF, Lawlor DP. Serum and urinary lysozyme (muramidase) in monocytic and monomyelocytic leukemia. J Exp Med. (1966) 124:921–52. doi: 10.1084/jem.124.5.921
188. Virella G. Electrophoresis of lysozyme into Microscoccus-containing agarose gel: quantitative and analytical applications. Clin Chim Acta. (1977) 75:107–15. doi: 10.1016/0009-8981(77)90505-8
189. Simide R, Richard S, Prévot-D'Alvise N, Miard T, Gaillard S. Assessment of the accuracy of physiological blood indicators for the evaluation of stress, health status and welfare in Siberian sturgeon (Acipenser baerii) subject to chronic heat stress and dietary supplementation. Int Aquat Res. (2016) 8:121–35. doi: 10.1007/s40071-016-0128-z
190. Eslamloo K, Akhavan SR, Fallah FJ, Henry MA. Variations of physiological and innate immunological responses in goldfish (Carassius auratus) subjected to recurrent acute stress. Fish Shellfish Immunol. (2014) 37:147–53. doi: 10.1016/j.fsi.2014.01.014
191. Caruso D, Schlumberger O, Dahm C, Proteau J-P. Plasma lysozyme levels in sheatfish Silurus glanis (L.) subjected to stress and experimental infection with Edwardsiella tarda. Aquac Res. (2002) 33:999–1008. doi: 10.1046/j.1365-2109.2002.00716.x
192. Mock A, Peters G. Lysozyme activity in rainbow trout, Oncorhynchus mykiss (Walbaum), stressed by handling, transport and water pollution. J Fish Biol. (1990) 37:873–85. doi: 10.1111/j.1095-8649.1990.tb03591.x
193. Yildiz H. Plasma lysozyme levels and secondary stress response in rainbow trout, Oncorhynchus mykiss (Walbaum) after exposure to leteux-meyer mixture. Turk J Vet Anim Sci. (2006) 30:265–9. Available online at: https://dergipark.org.tr/tr/download/article-file/132614
194. Caruso D, Lazard J. Subordination stress in Nile tilapia and its effect on plasma lysozyme activity. J Fish Biol. (1999) 55:451–4. doi: 10.1111/j.1095-8649.1999.tb00690.x
195. Sunyer JO, Tort L. Natural hemolytic and bactericidal activities of sea bream Sparus aurata serum are effected by the alternative complement pathway. Vet Immunol Immunopathol. (1995) 45:333–45. doi: 10.1016/0165-2427(94)05430-Z
196. Mauri I, Romero A, Acerete L, Mackenzie S, Roher N, Callol A, et al. Changes in complement responses in Gilthead seabream (Sparus aurata) and European seabass (Dicentrarchus labrax) under crowding stress, plus viral and bacterial challenges. Fish Shellfish Immunol. (2011) 30:182–8. doi: 10.1016/j.fsi.2010.10.006
197. Montero D, Marrero M, Izquierdo M, Robaina L, Vergara J, Tort L. Effect of vitamin E and C dietary supplementation on some immune parameters of gilthead seabream (Sparus aurata) juveniles subjected to crowding stress. Aquaculture. (1999) 171:269–78. doi: 10.1016/S0044-8486(98)00387-1
198. Rotllant J, Tort L. Cortisol and glucose responses after acute stress by net handling in the sparid red porgy previously subjected to crowding stress. J Fish Biol. (1997) 51:21–8. doi: 10.1111/j.1095-8649.1997.tb02510.x
199. Herron CL, Cogliati KM, Dolan BP, Munakata A, Schreck CB. Stress up-regulates oxidative burst in juvenile Chinook salmon leukocytes. Fish Shellfish Immunol. (2018) 80:655–9. doi: 10.1016/j.fsi.2018.06.038
200. Narra MR, Rajender K, Reddy RR, Murty US, Begum G. Insecticides induced stress response and recuperation in fish: biomarkers in blood and tissues related to oxidative damage. Chemosphere. (2017) 168:350–7. doi: 10.1016/j.chemosphere.2016.10.066
201. Caipang CMA, Berg I, Brinchmann MF, Kiron V. Short-term crowding stress in Atlantic cod, Gadus morhua L. modulates the humoral immune response. Aquaculture. (2009) 295:110–5. doi: 10.1016/j.aquaculture.2009.06.036
202. Karadag H, Firat Ö, Firat Ö. Use of oxidative stress biomarkers in Cyprinus carpio L. for the evaluation of water pollution in Ataturk Dam Lake (Adiyaman, Turkey). Bull Environ Contam Toxicol. (2014) 92:289–93. doi: 10.1007/s00128-013-1187-0
203. Montero R, Strzelczyk JE, Chan JTH, Verleih M, Rebl A, Goldammer T, et al. Dawn to dusk: Diurnal rhythm of the immune response in rainbow trout (Oncorhynchus mykiss). Biology. (2020) 9:1–13. doi: 10.3390/biology9010008
204. Shahsavani D, Mohri M, Gholipour Kanani H. Determination of normal values of some blood serum enzymes in Acipenser stellatus Pallas. Fish Physiol Biochem. (2010) 36:39–43. doi: 10.1007/s10695-008-9277-3
205. Svoboda M, Kouril J, Hamáčková J, Kaláb P, Savina L, Svobodová Z, et al. Biochemical profile of blood plasma of tench (Tinca tinca L.) during pre- and postspawning period. Acta Vet Brno. (2001) 70:259–68. doi: 10.2754/avb200170030259
206. Lulijwa R, Alfaro AC, Merien F, Meyer J, Young T. Advances in salmonid fish immunology: a review of methods and techniques for lymphoid tissue and peripheral blood leucocyte isolation and application. Fish Shellfish Immunol. (2019) 95:44–80. doi: 10.1016/j.fsi.2019.10.006
207. Goetz FW, Planas JV, Díaz M, Iliev DB, MacKenzie S. Culture of fish head kidney mononuclear phagocytes and muscle satellite cells: valuable models for aquaculture biotechnology research. in Aquaculture Biotechnology (Oxford: Wiley-Blackwell). p. 207–21. doi: 10.1002/9780470963159.ch13
208. Anderson DP, Dixon OW, Lizzio EF. Immunization and culture of rainbow trout organ sections in vitro. Vet Immunol Immunopathol. (1986) 12:203–11. doi: 10.1016/0165-2427(86)90124-8
209. Martorell Ribera J, Nipkow M, Viergutz T, Brunner RM, Bochert R, Koll R, et al. Early response of salmonid head-kidney cells to stress hormones and toll-like receptor ligands. Fish Shellfish Immunol. (2020) 98:950–61. doi: 10.1016/j.fsi.2019.11.058
210. Pierrard M-A, Roland K, Kestemont P, Dieu M, Raes M, Silvestre F. Fish peripheral blood mononuclear cells preparation for future monitoring applications. Anal Biochem. (2012) 426:153–65. doi: 10.1016/j.ab.2012.04.009
211. Miller NW, Deuter A, Clem LW. Phylogeny of lymphocyte heterogeneity: the cellular requirements for the mixed leucocyte reaction with channel catfish. Immunology. (1986) 59:123–8.
212. Forlenza M, Walker PD, de Vries BJ, Wendelaar Bonga SE, Wiegertjes GF. Transcriptional analysis of the common carp (Cyprinus carpio L.) immune response to the fish louse Argulus japonicus Thiele (Crustacea: Branchiura). Fish Shellfish Immunol. (2008) 25:76–83. doi: 10.1016/j.fsi.2007.12.013
213. Scapigliati G, Buonocore F, Randelli E, Casani D, Meloni S, Zarletti G, et al. Cellular and molecular immune responses of the sea bass (Dicentrarchus labrax) experimentally infected with betanodavirus. Fish Shellfish Immunol. (2010) 28:303–11. doi: 10.1016/j.fsi.2009.11.008
214. Duchiron C, Betoulle S, Reynaud S, Deschaux P. Lindane increases macrophage-activating factor production and intracellular calcium in rainbow trout (Oncorhynchus mykiss) leukocytes. Ecotoxicol Environ Saf. (2002) 53:388–96. doi: 10.1016/S0147-6513(02)00007-6
215. Reynaud S, Deschaux P. The effects of 3-methylcholanthrene on lymphocyte proliferation in the common carp (Cyprinus carpio L.). Toxicology. (2005) 211:156–64. doi: 10.1016/j.tox.2005.02.015
216. Rymuszka A, Sierosławska A, Bownik A, Skowroński T. In vitro effects of pure microcystin-LR on the lymphocyte proliferation in rainbow trout (Oncorhynchus mykiss). Fish Shellfish Immunol. (2007) 22:289–92. doi: 10.1016/j.fsi.2006.06.002
217. Yin D-q, Hu S-q, Gu Y, Wei L, Liu S-s, Zhang A-q. Immunotoxicity of bisphenol A to Carassius auratus lymphocytes and macrophages following in vitro exposure. J Environ Sci. (2007) 19:232–7. doi: 10.1016/S1001-0742(07)60038-2
218. Kammann U, Riggers JC, Theobald N, Steinhart H. Genotoxic potential of marine sediments from the North Sea. Mutat Res. (2000) 467:161–8. doi: 10.1016/S1383-5718(00)00030-9
219. Kemenade BV, Verburg-Van Kemenade BML, Nowak B, Engelsma MY, Weyts FAA. Differential effects of cortisol on apoptosis and proliferation of carp B-lymphocytes from head kidney, spleen and blood. Fish Shellfish Immunol. (1999) 9:405–15. doi: 10.1006/fsim.1998.0197
220. Lammel T, Tsoukatou G, Jellinek J, Sturve J. Development of three-dimensional (3D) spheroid cultures of the continuous rainbow trout liver cell line RTL-W1. Ecotoxicol Environ Saf. (2019) 167:250–8. doi: 10.1016/j.ecoenv.2018.10.009
221. Kralik P, Ricchi M. A basic guide to real time PCR in microbial diagnostics: definitions, parameters, and everything. Front Microbiol. (2017) 8:108. doi: 10.3389/fmicb.2017.00108
222. Urquhart K, Collins C, Monte M, Sokolowska J, Secombes C, Collet B. Individual measurement of gene expression in blood cells from Rainbow trout Oncorhynchus mykiss (Walbaum). J Exp Appl Anim Sci. (2016) 2:1. doi: 10.20454/jeaas.2016.1077
223. Zhang A, Zhou X, Wang X, Zhou H. Characterization of two heat shock proteins (Hsp70/Hsc70) from grass carp (Ctenopharyngodon idella): evidence for their differential gene expression, protein synthesis and secretion in LPS-challenged peripheral blood lymphocytes. Comp Biochem Physiol B Biochem Mol Biol. (2011) 159:109–14. doi: 10.1016/j.cbpb.2011.02.009
224. Ferencz Á Juhász R Butnariu M Deér KA Varga IS Nemcsók J. Expression analysis of heat shock genes in the skin, spleen and blood of common carp (Cyprinus carpio) after cadmium exposure and hypothermia. Acta Biol Hung. (2012) 63:15–25. doi: 10.1556/ABiol.63.2012.1.2
225. Fulladosa E, Deane E, Ng AHY, Woo NYS, Murat JC, Villaescusa I. Stress proteins induced by exposure to sublethal levels of heavy metals in sea bream (Sparus sarba) blood cells. Toxicol Vitr. (2006) 20:96–100. doi: 10.1016/j.tiv.2005.06.005
226. Irwin MR, Cole SW. Reciprocal regulation of the neural and innate immune systems. Nat Rev Immunol. (2011) 11:625–32. doi: 10.1038/nri3042
227. Elenkov E, Chrousos C. Stress Hormones, Th1/Th2 patterns, pro/anti-inflammatory cytokines and susceptibility to disease. Trends Endocrinol Metab. (1999) 10:359–68. doi: 10.1016/S1043-2760(99)00188-5
228. Vihervaara A, Duarte FM, Lis JT. Molecular mechanisms driving transcriptional stress responses. Nat Rev Genet. (2018) 19:385–97. doi: 10.1038/s41576-018-0001-6
229. Lightman SL. The neuroendocrinology of stress: a never ending story. J Neuroendocrinol. (2008) 20:880–4. doi: 10.1111/j.1365-2826.2008.01711.x
230. Denslow ND, Garcia-Reyero N, Barber DS. Fish “n” chips: the use of microarrays for aquatic toxicology. Mol Biosyst. (2007) 3:172–7. doi: 10.1039/B612802P
231. Götting M, Nikinmaa MJ. Transcriptomic analysis of young and old erythrocytes of fish. Front Physiol. (2017) 8:1–11. doi: 10.3389/fphys.2017.01046
232. Kumar Roy A. Microarray analysis of fish genomic data for enhancing aquaculture productivity of India. Ann Proteom Bioinform. (2017) 1:6–17. doi: 10.29328/journal.hpbr.1001002
233. Douglas SE. Microarray studies of gene expression in fish. Omi A J Integr Biol. (2006) 10:474–89. doi: 10.1089/omi.2006.10.474
234. Claver JA, Quaglia AIE. Comparative morphology, development, and function of blood cells in non-mammalian vertebrates. J Exot Pet Med. (2009) 18:87–97. doi: 10.1053/j.jepm.2009.04.006
235. Puente-Marin S, Nombela I, Ciordia S, Mena MC, Chico V, Coll J, et al. In silico functional networks identified in fish nucleated red blood cells by means of transcriptomic and proteomic profiling. Genes. (2018) 9:40202. doi: 10.3390/genes9040202
236. Morera D, Roher N, Ribas L, Balasch JC, Doñate C, Callol A, et al. RNA-Seq reveals an integrated immune response in nucleated erythrocytes. PLoS ONE. (2011) 6:e26998. doi: 10.1371/journal.pone.0026998
237. Li Z, Liu X, Liu J, Zhang K, Yu H, He Y, et al. Transcriptome profiling based on protein-protein interaction networks provides a core set of genes for understanding blood immune response mechanisms against Edwardsiella tarda infection in Japanese flounder (Paralichthys olivaceus). Dev Comp Immunol. (2018) 78:100–13. doi: 10.1016/j.dci.2017.09.013
238. Puente-Marin S, Nombela I, Chico V, Ciordia S, Mena CM, Perez L, Coll J, Ortega-Villaizan MdM. Potential role of rainbow trout erythrocytes as mediators in the immune response induced by a DNA vaccine in fish. Vaccines. (2019) 7:60. doi: 10.3390/vaccines7030060
239. Dahle MK, Wessel Ø, Timmerhaus G, Nyman IB, Jørgensen SM, Rimstad E, et al. Transcriptome analyses of Atlantic salmon (Salmo salar L.) erythrocytes infected with piscine orthoreovirus (PRV). Fish Shellfish Immunol. (2015) 45:780–90. doi: 10.1016/j.fsi.2015.05.049
240. Lewis JM, Hori TS, Rise ML, Walsh PJ, Currie S. Transcriptome responses to heat stress in the nucleated red blood cells of the rainbow trout (Oncorhynchus mykiss). Physiol Genomics. (2010) 42:361–73. doi: 10.1152/physiolgenomics.00067.2010
241. Akbarzadeh A, Günther OP, Houde AL, Li S, Ming TJ, Jeffries KM, et al. Developing specific molecular biomarkers for thermal stress in salmonids. BMC Genom. (2018) 19:749. doi: 10.1186/s12864-018-5108-9
242. Rebl A, Verleih M, Nipkow M, Altmann S, Bochert R, Goldammer T. Gradual and acute temperature rise induces crossing endocrine, metabolic and immunological pathways in maraena whitefish (Coregonus maraena). Front Genet. (2018) 9:241. doi: 10.3389/fgene.2018.00241
243. Rebl A, Goldammer T. Under control: the innate immunity of fish from the inhibitors' perspective. Fish Shellfish Immunol. (2018) 77:328–49. doi: 10.1016/j.fsi.2018.04.016
244. Harris J, Bird DJ. Modulation of the fish immune system by hormones. Vet Immunol Immunopathol. (2000) 77:163–76. doi: 10.1016/S0165-2427(00)00235-X
245. Zhou C, Xiao S, Liu Y, Mou Z, Zhou J, Pan Y, et al. Comprehensive transcriptome data for endemic Schizothoracinae fish in the Tibetan Plateau. Sci Data. (2020) 7:28. doi: 10.1038/s41597-020-0361-6
246. Qi D, Chao Y, Wu R, Xia M, Chen Q, Zheng Z, et al. Transcriptome analysis provides insights into the adaptive responses to hypoxia of a schizothoracine fish (Gymnocypris eckloni). Front Physiol. (2018) 9:1–12. doi: 10.3389/fphys.2018.01326
247. Gracey AY, Troll JV, Somero GN. Hypoxia-induced gene expression profiling in the euryoxic fish Gillichthys mirabilis. Proc Natl Acad Sci USA. (2001) 98:1993–8. doi: 10.1073/pnas.98.4.1993
248. Le Q, Hu J, Cao X, Kuang S, Zhang M, Yu N, et al. Transcriptomic and cortisol analysis reveals differences in stress alleviation by different methods of anesthesia in Crucian carp (Carassius auratus). Fish Shellfish Immunol. (2019) 84:1170–9. doi: 10.1016/j.fsi.2018.10.061
249. Carmona SJ, Teichmann SA, Ferreira L, Macaulay C, Stubbington MJT, Cvejic A, et al. Single-cell transcriptome analysis of fish immune cells provides insight into the evolution of vertebrate immune cell types. Genome Res. (2017) 27:451–61. doi: 10.1101/gr.207704.116
250. Vogel C, Marcotte EM. Insights into the regulation of protein abundance from proteomic and transcriptomic analyses. Nat Rev Genet. (2012) 13:227–32. doi: 10.1038/nrg3185
251. Schwanhäusser B, Busse D, Li N, Dittmar G, Schuchhardt J, Wolf J, et al. Global quantification of mammalian gene expression control. Nature. (2011) 473:337–42. doi: 10.1038/nature10098
252. Ryan SN, Pankhurst NW, Wells RM. A possible role for ubiquitin in the stress response of the teleost fish blue mao mao (Scorpis violaceus). Physiol Zool. (1995) 68:1077–92. doi: 10.1086/physzool.68.6.30163794
253. Karim M, Puiseux-Dao S, Edery M. Toxins and stress in fish: proteomic analyses and response network. Toxicon. (2011) 57:959–69. doi: 10.1016/j.toxicon.2011.03.018
254. Kumar VB, Jiang I-F, Yang H-H, Weng C-F. Effects of serum on phagocytic activity and proteomic analysis of tilapia (Oreochromis mossambicus) serum after acute osmotic stress. Fish Shellfish Immunol. (2009) 26:760–7. doi: 10.1016/j.fsi.2009.03.005
255. Yi S, Liu L-F, Zhou L-F, Zhao B-W, Wang W-M, Gao Z-X. Screening of biomarkers related to ovarian maturation and spawning in blunt snout bream (Megalobrama amblycephala) based on metabolomics and transcriptomics. Mar Biotechnol. (2020) 22:180–93. doi: 10.1007/s10126-019-09943-5
256. Xiao M, Qian K, Wang Y, Bao F. GC-MS metabolomics reveals metabolic differences of the farmed Mandarin fish Siniperca chuatsi in recirculating ponds aquaculture system and pond. Sci Rep. (2020) 10:6090. doi: 10.1038/s41598-020-63252-9
257. Sitjà-Bobadilla A, Gil-Solsona R, Estensoro I, Piazzon MC, Martos-Sitcha JA, Picard-Sánchez A, et al. Disruption of gut integrity and permeability contributes to enteritis in a fish-parasite model: a story told from serum metabolomics. Parasit Vectors. (2019) 12:486. doi: 10.1186/s13071-019-3746-7
258. Young T, Alfaro AC. Metabolomic strategies for aquaculture research: a primer. Rev Aquac. (2018) 10:26–56. doi: 10.1111/raq.12146
259. Kokushi E, Uno S, Harada T, Koyama J. 1H NMR-based metabolomics approach to assess toxicity of bunker a heavy oil to freshwater carp, Cyprinus carpio. Environ Toxicol. (2012) 27:404–14. doi: 10.1002/tox.20653
260. Zhang H, Zhao L. Influence of sublethal doses of acetamiprid and halosulfuron-methyl on metabolites of zebra fish (Brachydanio rerio). Aquat Toxicol. (2017) 191:85–94. doi: 10.1016/j.aquatox.2017.08.002
261. Kokushi E, Uno S, Pal S, Koyama J. Effects of chlorpyrifos on the metabolome of the freshwater carp, Cyprinus carpio. Environ Toxicol. (2015) 30:253–60. doi: 10.1002/tox.21903
262. Samuelsson LM, Förlin L, Karlsson G, Adolfsson-Erici M, Larsson DGJ. Using NMR metabolomics to identify responses of an environmental estrogen in blood plasma of fish. Aquat Toxicol. (2006) 78:341–9. doi: 10.1016/j.aquatox.2006.04.008
263. Carbajal A, Monclús L, Tallo-Parra O, Sabes-Alsina M, Vinyoles D, Lopez-Bejar M. Cortisol detection in fish scales by enzyme immunoassay: biochemical and methodological validation. J Appl Ichthyol. (2018) 34:967–70. doi: 10.1111/jai.13674
264. Guardiola FA, Cuesta A, Esteban MÁ. Using skin mucus to evaluate stress in gilthead seabream (Sparus aurata L.). Fish Shellfish Immunol. (2016) 59:323–30. doi: 10.1016/j.fsi.2016.11.005
265. Félix AS, Faustino AI, Cabral EM, Oliveira RF. Noninvasive measurement of steroid hormones in zebrafish holding-water. Zebrafish. (2013) 10:110–5. doi: 10.1089/zeb.2012.0792
Keywords: erythrocytes, hematology, leukocytes, teleost fishes, well-being, transcriptomics, proteomics, stress
Citation: Seibel H, Baßmann B and Rebl A (2021) Blood Will Tell: What Hematological Analyses Can Reveal About Fish Welfare. Front. Vet. Sci. 8:616955. doi: 10.3389/fvets.2021.616955
Received: 13 October 2020; Accepted: 10 February 2021;
Published: 30 March 2021.
Edited by:
Edward Narayan, The University of Queensland, AustraliaReviewed by:
Janicke Nordgreen, Norwegian University of Life Sciences, NorwayYifei Yang, The University of Queensland, Australia
Copyright © 2021 Seibel, Baßmann and Rebl. This is an open-access article distributed under the terms of the Creative Commons Attribution License (CC BY). The use, distribution or reproduction in other forums is permitted, provided the original author(s) and the copyright owner(s) are credited and that the original publication in this journal is cited, in accordance with accepted academic practice. No use, distribution or reproduction is permitted which does not comply with these terms.
*Correspondence: Alexander Rebl, cmVibEBmYm4tZHVtbWVyc3RvcmYuZGU=