- 1Tropical Crops Genetic Resources Institute, Chinese Academy of Tropical Agricultural Sciences, Haikou, China
- 2College of Animal Science and Veterinary Medicine, Shanxi Agricultural University, Taigu, China
- 3College of Animal Sciences and Technology, Hainan University, Haikou, China
The study investigated amelioration effects of coconut oil (CO) on growth performance, nutrient digestibility, ruminal fermentation, and blood metabolites in Hainan Black goat kids. Twenty-four Hainan Black goat kids (10 days of age) were assigned randomly to four treatments for 90 days, including pre-weaning (10–70 d of age) and post-weaning (70-100 d of age) days. The treatment regimens were control (CON), low CO (LCO), medium CO (MCO), and high CO (HCO) with 0, 4, 6, 8 g CO per goat per day, respectively. During the pre-weaning period, the average daily gain (ADG) linearly and quadratically increased (P < 0.05), whereas the average daily feed intake (ADFI) linearly decreased, and the feed conversion ratio (FCR) also decreased linearly and quadratically by increasing CO supplementation (P < 0.05). During the post-weaning period, increasing CO supplementation linearly and quadratically increased the BW at 100 days and ADG (P < 0.05), but quadratically decreased the ADFI and FCR (P < 0.05). The digestibility of ether extract (EE) linearly and quadratically increased with increasing CO supplementation (P < 0.05). Supplementation of CO linearly increased ruminal pH (P < 0.05), but linearly decreased (P < 0.05) ammonia-N, total VFAs, molar proportions of acetate, ruminal microbial enzyme activity of carboxymethyl-cellulase, cellobiase, xylanase, pectinase and α-amylase, and number of total protozoa, the abundance of Ruminococcus albus, Ruminococcus flavefaciens, Fibrobacter succinogenes, Butyrivibrio fibrisolvens, Prevotella ruminicola, and Ruminobacter amylophilus. The estimated methane emission decreased linearly and quadratically with increasing CO addition (P < 0.05). The serum concentration of triglycerides (TG), non-esterified fatty acids (NEFA) and growth hormone (GH) linearly (P < 0.05) increased by raising the CO supplementation. The present results indicate that CO supplementation at 6 g/day per goats is optimum due to improved growth performance and decreased estimated methane emission. Supplementation CO up to 8 g/day depressed growth and feed conversion due to its suppression of growth performance, rumen protozoa, cellulolytic bacteria and microbial enzyme activity, and reduced ADF and ADF digestibility.
Introduction
Goats are important meat-producing animals and goat meat is well-appreciated by consumers worldwide, especially in developing countries (1). Hainan Black goats are the main goat breed in South China, characterized by a good adaptability to the local hot and wet weather (2). Hainan Black goat meat is also very popular in South China because of its delicious flavors. However, Hainan Black goats exhibit slow growth rates and small body sizes, resulting in poor carcass characteristics (3).
Manipulation of the rumen microbial ecosystem to enhance fiber digestion, reduce the excretion of methane and urea, in order to improve the production performance of ruminants is one of the most important goals for animal nutritionists (4, 5). Dietary fats have been used to improve ruminant growth performance and modify meat characteristics with human health benefits (6). Moreover, fat supplementation in the diet of newborn lambs would be considered an effective mechanism to modify the rumen microbiome (7, 8). Therefore, further understanding of the effects of fat on rumen fermentation may help to offer a nutritional strategy to reduce rumen methane emissions and improve the quality of ruminant products.
Among all the lipid feedstocks, vegetable oils, oilseed, and calcium salts of fatty acids are the most appropriate for application in ruminant diets (9). Coconut oil is a cheaper, tastier, and readily available feed resource for ruminants (10). Coconut oil is a highly saturated oil (About 90% saturation), which is rich in medium chain fatty acids (MCFAs) (11). MCFA have been reported to reduce fat deposition due to their faster metabolism and reduced storage in adipocytes (12). Furthermore, coconut oil has been proven to exert positive environmental effects by enhancing rumen fermentation via limiting the production of methane and modifying microbial populations (13–15). There have been discrepancies in the results obtained by studies conducted to evaluate the effects of coconut oil supplementation on nutrient digestibility, growth performance and body composition of ruminants. The studies reported by Ding et al. (16) found that supplementing 12 g CO or 0.48 g/kg BW daily, showed a strong methane reduction as well as a decrease in the number of methanogen and Fibrobacter succinogenes in Tibetan sheep. Similar effects were also observed by Liu et al. (17) who reported that supplementation with 0.52 g/kg BW CO in sheep decreased methane emissions by reducing the methanogen and protozoa populations without negatively affecting the growth performance or reduction of rumen total VFA. Besides, the anti-methane effects of CO were also observed in swamp buffalo (18) and dairy cows (19), and neither study identified negative effects of CO on DMI, nutrient digestibility or ruminal fermentation. However, a study on beef heifers with different levels of CO demonstrated a linear decrease in CH4 production without affecting the DMI or giving rise to negative effects on DMI and digestibility at lower doses, with only the highest dose of 375 g/d yielding undesirable effects on the DMI and digestibility (20). Another study in lambs revealed that CO supplementation at 50 g/kg in the concentrate improved the feed conversion ratio and carcass traits of lambs, but its higher inclusion in ruminant diets has negative effects on growth and feed conversion due to its depressing impact on rumen protozoa which results in lower fiber digestibility (10). We hypothesized that in ruminant species, the level of fat, and the nature of the basal diet may determine the variable effects of CO on ruminal microbes.
Considering the inconsistent results regarding the impact of CO supplementation on growth performance, nutrient digestibility, and ruminal fermentation, as well as the limited research performed in goat kids, this study was undertaken to investigate the effects of coconut oil on growth performance, nutrient digestion, ruminal fermentation, and blood metabolites in Hainan Black goat kids.
Materials and Methods
Animals and Experimental Design
The animal and experiment protocols were approved by the Animal Care and Use Committee of Chinese Academy of Tropical Agricultural Sciences (ACUCC), Hainan, PR China. Twenty-four Hainan Black goat kids averaging 10 days of age and 2.05 ± 0.16 kg of body weight (BW) were randomly assigned to four treatment regimens. The treatments consisted of control (CON), low CO (LCO), medium CO (MCO) and high CO (HCO) dosages containing 0, 4, 6, 8 g of CO per goat daily, respectively. The CO supplement was purchased commercially and sprayed into the back of the kids' mouth using a small syringe, twice a day at 0700 and 1700 h throughout the experimental period. From 10 to 70 days of age (weaning), the goat kids were fed with a milk replacer (2% of BW) twice a day at 0800 h and 1800 h for 30 days, after which the daily milk portion was decreased by half until weaning. The goats were weighed weekly to calculate the amount of milk replacer to be administered. The goats were also offered an ad libitum concentrate and dried king grass in a cafeteria system during the whole experimental period, and the dietary concentrate to forage ratio was maintained at 50:50 based on an air-dry matter. All goats were fed the same concentrate mixture. The post-weaning feeding management for all goats was kept identical that of the pre-weaning phase, except for the fact that administration of the milk replacer stopped at 70 days of age. The ingredients and chemical composition of the experimental diets were illustrated in Table 1. Fresh water was available to the goats for drinking throughout the experimental period. The animals were weighed at 10, 70, and 100 days of age before feeding, and the average daily gain (ADG) was recorded.
Data Collection and Sampling Procedures
The milk intake of individual goats was measured during the pre-weaning period. Feed offered and refusals for each goat were also recorded on a daily basis throughout the experimental period so as to calculate the daily DM intake (DMI). The goats were dosed via the esophagus with 1 g of chromic oxide in a paper capsule twice daily (07:00 and 19:00 h) from 78–87 days of age. The chromic oxide powder was used as a digestion marker to estimate the fecal excretion. From 83–87 days of age, Fecal pellets were collected from the rectum at 7:00, 15:00, and 24:00, then representative samples of the feces were pooled. The samples of feeds, refusals and feces were pooled for each goat, dried at 60°C for 48 h, ground to pass a 1 mm sieve, and preserved for chemical composition analysis. The apparent nutrient digestibility was calculated according to our prior studies (21).
Samples of rumen fluid were collected using an oral stomach tube at 07:00 by 70 days of age. The initial 100 mL ruminal fluid extracted was discarded, and the next 100 mL was retained. The fluid's pH values were immediately measured using a pH meter (PHS-3C, Shanghai Leijun experimental instrument Co., Ltd., Shanghai, China). After pH measurement, the rumen fluid was filtered through four layers of cheesecloth and subsampled for various determinations. A 5 ml filtrate was preserved by adding 1 mL of 250 g/L meta-phosphoric acid or 1 mL of 20 g/L H2SO4 to determine the VFA and NH3 concentrations, respectively. These samples were then frozen at −20°C until further analysis. About 50 mL of filtrate was collected and frozen at −80°C for DNA extraction, and another 40 mL of filtrate was used to determine the activity of ruminal enzymes according to the method described by Agarwal (22).
At 70 and 100 days of age, about 5 ml of blood was collected from the jugular vein and harvested into tubes without anticoagulant before the morning feeding at 100 days of age. Serum samples were then centrifuged at 3,000 × g for 15 min at 4°C and stored at −20°C until the assay.
Chemical Analyses
Oven-dried samples were analyzed for DM method 934.01), OM (method 942.05), nitrogen (method 976.05), ether extract (method 973.18) and acid detergent fiber (ADF; method 973.18) according to AOAC methods (23). The neutral detergent fiber (aNDF) was analyzed using methods described by Van Soest et al. (24) with heat stable alpha amylase and sodium sulfite utilized in the NDF procedure, and results were expressed inclusive of residual ash. Ruminal VFA concentration was measured by gas chromatography (HP Agilent 6890N, Santa Clara, CA, USA) with a flame ionization detector equipped with an HP-INNOWAX (19091N-133) capillary column (30 m × 0.25 mm × 0.25 μm). Two microliter of fluid samples were injected with a syringe, and the injector and detector temperature were programmed at 200 and 220°C, respectively. Nitrogen was used as a carrier flowing at 5.5 mL/min. A program altered oven temperature from 80 to 170°C at 15°C/min and then held it at 170°C for 1.5 min. Ruminal VFA were expressed on the basis of absolute concentrations (mM) and molar proportions (mol/100 mol total VFA). Ruminal ammonia-N concentration was determined by a colorimetric spectrophotometer (UV2100, Shanghai Younike instrument Co., Ltd., Shanghai, China) according to AOAC methods (2000). Subsequently ruminal fluid samples were sonicated at 4°C in an ice bath with a 30 s pulsation rate for 10 min, then centrifuged at 3,000 × g at 4°C for 20 min. The resulting supernatant was used for estimation of the enzyme activity (carboxymethyle cellulase, cellobiase, xylanase, pectinase, α-amylase and protease) as described by Agarwal et al. (22). Serum parameters including glucose, cholesterol, and triglycerides were determined by using the BH13 MD 1600 (America) automatic biochemical analyzer. Serum level of non-esterified fatty acids (Nanjing Jiancheng Bioengineering Institute, Nanjing, China) and growth hormone (Shanghai Fankel Industrial Co., Ltd, Shanghai, China) were determined by using enzyme-linked immunosorbent assay (ELISA) kits according to the manufacturer's instructions.
DNA Extraction and Quantitative Real-Time PCR
Microbial DNA was extracted from 0.5 g of rumen fluid by using a Fastpure Bacteria DNA Isolation Mini Kit (Vazyme, Version 8.1). Subsequently, agarose gel electrophoresis and the NanoDrop 2000 Spectrophotometer (NanoDrop Technologies, USA), were used to evaluate the quality and quantity of DNA, respectively. The extracted DNA was then kept frozen at −20°C for real time PCR analysis. Populations of Ruminococcus albus, Ruminococcus flavefaciens, F. succinogenes, Butyrivibrio fibrisolvens, Prevotella ruminicola, and Ruminobacter amylophilus were estimated using real time PCR as a proportion of the total number of bacteria. The sequences of all primers were synthesized by Tianyi Huiyuan Biotechnology Co., Ltd and displayed in Table 2. All real-time PCR reactions were carried out in triplicate and run on Applied Biosystems 7500 Fast real-time quantitative PCR systems. The reaction mixture (20 μL) contained 10 μL SYBR Color qPCR Master Mix (Vazyme Biotechnology Co., Ltd., Nanjing, China), 0.4 μL 10 μmol/L PCR Forward Primer, 0.4 μL 10 μmol/L PCR Reverse Primer, 0.4 μL ROX Reference Dye (50×), 6.8 μL ddH2O and 2 μL of the template DNA. The quantity of DNA was measured in triplicate for each sample using the ND-1000 UV spectrophotometer (NanoDrop Technologies, USA), and the mean values were estimated. PCR was implemented according to the following conditions: Degeneration at 95°C for 60 s; PCR reaction at 95°C for 15 s and 60°C for 30 s, with 40 cycles; dissociation stage.
Statistical Analyses
Data analysis was conducted using the SAS mixed model procedure (Proc Mixed; SAS, 2002). Analysis of variance (ANOVA) was performed to examine the effects of the respective treatment regimens on growth performance, nutrient digestibility, ruminal fermentation, and blood metabolites. Linear and quadratic effects were tested using the CONTRAST statement of SAS with coefficients estimated based on the CON application rates. Differences between the treatment regimens were detected by the Duncan's multiple range test. The P-value for statistical significance was set at P ≤ 0.05, unless otherwise noted P ≤ 0.10 was considered as a tendency approaching significance.
Results
Dry Matter Intake, Average Daily Gain, and Feed Conversion Ratio
Dry matter intake, average daily gain and feed conversion ratio were delineated in Table 3. The dry matter intake (DMI) exhibited a linear decline (P < 0.05) with increasing CO supplementation for pre-weaned and post-weaned goats, and was lower for HCO than for control, LCO, and MCO (P < 0.05). Meanwhile the average daily gain (ADG) for pre-weaned and post-weaned goats increased linearly (P < 0.05) and quadratically (P < 0.05) with increasing CO supplementation and was higher for MCO than that for control, LCO, and HCO (P < 0.05). The feed conversion ratio (FCR) for pre-weaned and post-weaned goats decreased linearly (P < 0.05) and quadratically (P < 0.05) with increasing CO supplementation, and was lower for MCO group than control and HCO (P < 0.05).
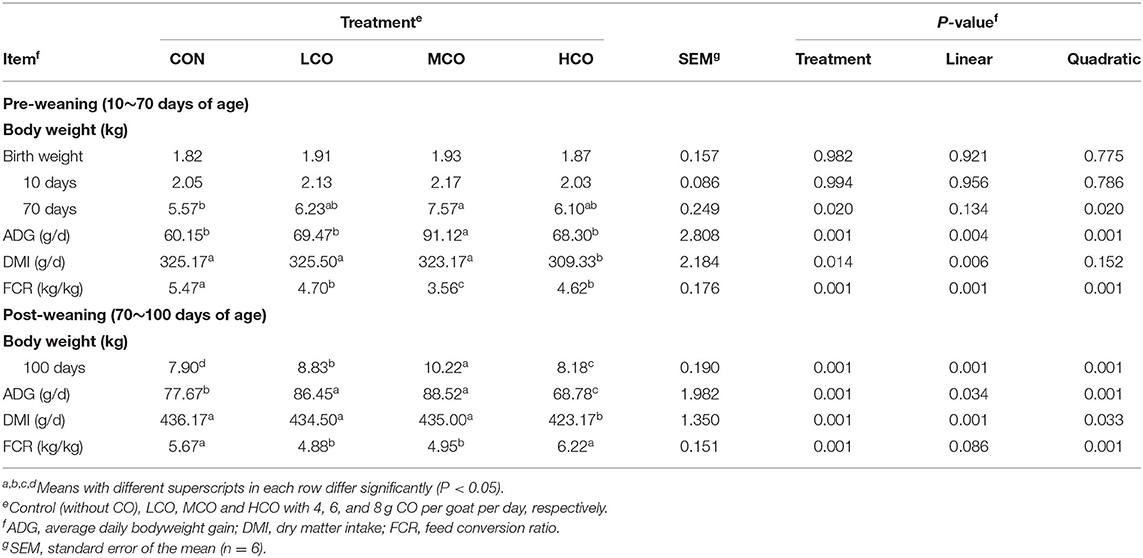
Table 3. Effects of coconut oil on dry matter intake, average daily gain and feed conversion ratio in goat kids.
Nutrient Digestibility and Ruminal Fermentation Parameters
As presented in Table 4, the digestibility of crude protein (CP) was not affected by CO addition. The digestibility of DM, OM, aNDF and ADF decreased linearly (P < 0.05) with increasing CO supplementation, and was lower for MCO than that of control, LCO and HCO groups (P < 0.05). However, the digestibility of EE increased linearly (P < 0.05) and quadratically (P < 0.05) with increasing CO supplementation, and was higher for MCO and HCO than control and LCO (P < 0.05).
Furthermore, ruminal pH increased linearly (P < 0.05) with increasing CO supplementation and was higher for HCO and LCO than control (P < 0.05). Total ruminal VFA concentration linearly decreased (P < 0.05) and was lower for HCO group than other three groups (P < 0.05). The molar proportions of propionate, valerate, and the ratio of acetate to propionate were not affected (P > 0.05), but the molar proportions of acetate, butyrate, isobutyrate and isovalerate linearly (P < 0.05) decreased with increasing CO supplementation, and was lower for the HCO than for control, LCO and MCO (P < 0.05). Ruminal ammonia N content linearly reduced by increasing CO supplementation (P < 0.05). The estimated methane emission decreased linearly (P < 0.05) and quadratically (P < 0.05) with increasing CO supplementation and was lower for the LCO, MCO, and HCO than control (P < 0.05).
Ruminal Microbial Enzyme Activity and Populations of Ruminal Cellulolytic Bacteria
The enzymatic activities of caboxymethyl-cellulase, cellobiase, xylanase, pectinase and α-amylase linearly (P < 0.05) decreased with increasing CO supplementation, and were lower for HCO than the control (P < 0.05) (Table 5).
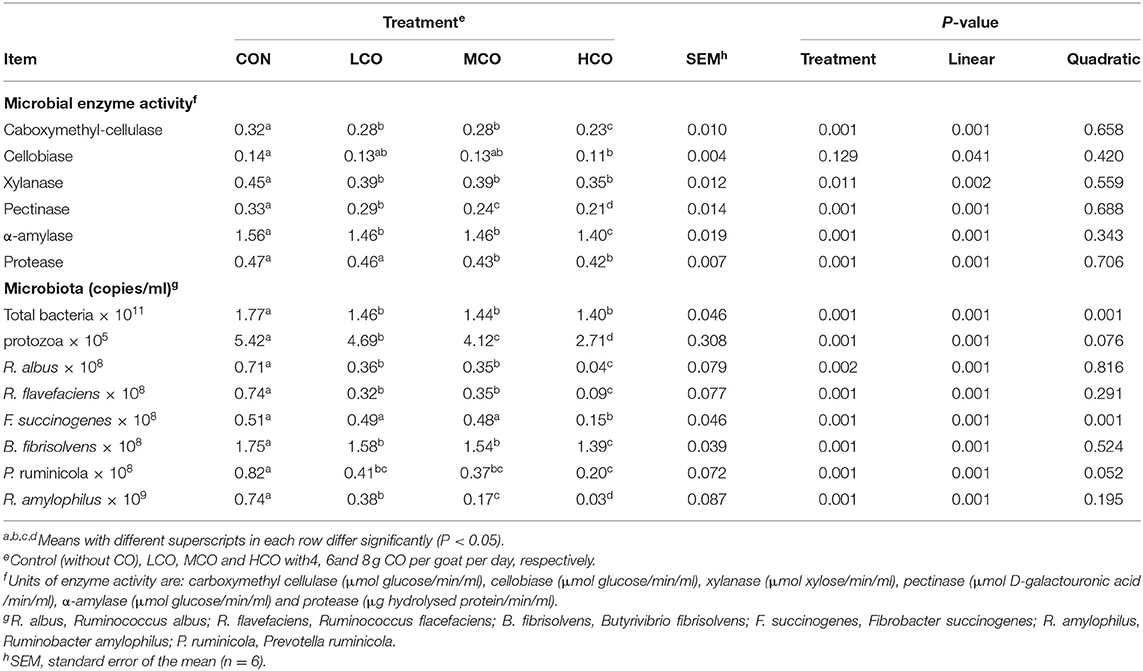
Table 5. Effects of coconut oil on rumen microbial enzyme activity and ruminal microflora in goat kids.
Total bacterial and F. succinogenes populations linearly (P < 0.05) and quadratically (P < 0.05) decreased, and the populations of R. albus, R. flavefaciens, B. fibrisolvens, P. ruminicola, and R. amylophilus decreased linearly (P < 0.05) with increasing CO supplementation (Table 5).
Blood Metabolites
The serum concentration of TGs, NEFAs, and GH linearly (P < 0.05) increased with increasing CO supplementation and was higher for HCO group than for the control (P < 0.05). Nevertheless, serum glucose and TC were not affected by CO supplementation (P > 0.05) for pre-weaning and post-weaning goats (Table 6).
Discussion
Growth Performance of Goat Kids
An appropriate amount of energy supply is the key to ensure and promote the healthy and rapid growth and development of young ruminants. Dietary MCFA (medium chain fatty acid) can effectively reduce body fat deposition and improve lipid concentration. However, there have been discrepancies in the results of coconut oil supplementation on nutrient digestibility, growth performance, etc. The decrease in DM intake with increasing CO supplementation was consistent with the findings of other studies, in which DM intake was decreased by CO supplementation (25, 50, and 75 g/kg of concentrate) in the diet of lambs (10). This reduced DM intake was not surprising, since the negative effects of CO on DMI may be a consequence of higher energy density in the diet (25, 26). Moreover, higher CO inclusion have been shown to be related to decreased NDF digestion (27) and palatability (28). Hollmann and Beede (29) also reported that CO replacement of ground corn in the diets of lactating dairy cows with CO lead to a significant reduction in the DMI. Linear and quadratic increments in the ADG with higher CO supplementation were observed in our experiment. Meanwhile, FCR in this study quadratically decreased with CO supplementation during the pre-weaning and post-weaning periods. This could be attributed to the improvement in the energy intake level and EE digestibility by CO supplementation, essentially due to the higher EE levels of CO-supplemented diets. Similarly, Dutta et al. (30) reported a gradual increase in ADG up to 50 g/kg fat supplementation, but above this level it declined. Unlike our finding, Bhatt et al. (10) reported that increasing CO supplementation had no effect on ADG of lambs during the pre-weaning and post-weaning periods, which might be due to the heat stress in their study.
Nutrient Digestibility
Adding appropriate fat or fatty acid into ruminates can promote nutrient digestibility (8). The linear EE digestibility increments with higher CO supplementation observed in this study were also reported by Bhatt et al. (10). No change was observed in CP digestibility, but DM, OM, ADF, and NDF digestibility linearly decreased when increasing CO feeding portions. Similar results have been reported after CO addition (29, 31). This phenomenon could be the result of several factors. For instance, CO supplementation could markedly reduce the number of rumen protozoa (10). Rumen protozoa exhibited cellulase, hemicellulase, and pectinase activities (32–34), which may explain their role in NDF digestion. Additionally, rumen protozoa may also alter the number of cellulolytic bacteria, and thus affect the extent of ruminal fiber fermentation (35). In the present study, the reduction in NDF digestibility was consistent with a reduction in protozoal numbers by increasing CO supplementation, and this maybe a reason for reduced NDF and ADF digestibility by increasing CO feeding portions.
Ruminal Fermentation, Microorganism Population and Enzyme Activities
Fat can be used as carrier of fat-soluble vitamins and promote the absorption and utilization of fat-soluble vitamins, negative effects on rumen microbes, fiber digestion, and fermentation (10, 14). The pH in rumen fluid linearly increased with increasing CO supplementation and was higher for LCO and HCO than MCO and control group, which was similar with the observation of Pilajun et al. (36), who reported that ruminal pH was directly proportional to the dosage of CO replacing sunflower oil from 250 to 750 g/kg in steers. Ruminal microbes can utilize ruminal ammonia-N derived from protein degradation for microbial protein synthesis (37). The lower ammonia-N levels produced by goats receiving CO supplementation were likely due to decreased protease activity, and reduced rumen bacterial and protozoal populations.
CO supplementation linearly decreased the total VFA concentration in the rumen. The results of this study were consistent with those obtained by Machmüller et al. (38), who found that CO supplementation tended to decrease the total VFA concentration. This finding could be due to the inhibitory effect of fatty acids on fiber digestion (39) and toxicity of fats to microorganisms (40). Moreover, no differences were observed in the molar proportion of propionate, but the molar proportions of acetate and butyrate were decreased with higher levels of CO supplementation. The possible explanation for this phenomenon was that CO inhibited bacteria and protozoa that are not related to Selenomonas ruminantium, which is essential to propionate production (41). Ruminal cellulolytic bacteria and protozoa produce cellulolytic enzymes and degrade dietary fiber to acetate (42). Thus, the lower acetate molar proportion resulted from the decrease in activity of carboxymethyl-cellulase, cellobiase and xylanase as well as the total population of bacteria, protozoa, and cellulolytic bacteria (R. albus, R. flavefaciens, B. fibrisolvens, and F. succinogenes) following CO addition. Being a by-product of carbohydrate fermentation, butyrate is produced by ruminal protozoa (43). Similarly, Hristov et al. (41) reported that CO supplementation inhibited both protozoa and important butyrate producers in the rumen, such as B. fibrisolvens. Hence, the decrease in the population of protozoa with CO supplementation observed in the present study also provides evidence for the reduction in the molar proportion of butyrate. In agreement with this study, similar findings in total VFA concentration (38), proportion of acetate (17), propionate (17), and A:P ratio (17) were reported in other previous studies. In contrast, Bozzolo et al. (44) found that dietary supplementation of 50 g/kg of CO had no significant effect on the concentration of VFA in the rumen of lambs for a period of 2 weeks directly after weaning. The inconsistency in these results could be due to that in their experiment, the lambs among the treatment fed the same level of fatty acids included in the diet, whereas in our experiment, goat kids in each treatment were fed a diet with different levels of total energy intake.
Calculation of ruminal methane production using VFAs based on this study's procedure demonstrated that CO supplementation elicited a significantly linear and quadratic decline in methane production. The protozoa populations were also linearly reduced by CO addition. These results were consistent with those of in vitro (45) and in vivo studies (15, 28), which have confirmed the methane-suppressing effect of CO supplementation in ruminants.
The linear decrease in the total population of bacteria, protozoa, R. amylophilus, and predominant cellulolytic bacteria (R. albus, R. flavefaciens, B. fibrisolvens, and F. succinogenes) with increasing CO supplementation suggested that CO modulates the ruminal microorganisms in a dose-dependent manner. The toxicity of Medium-chain saturated FAs to the ruminal microbiota has been well-documented. Work by Hristov et al. (41) has confirmed that CO supplementation results in statistically significant suppression of microbial flow. Inhibition of total bacterial counts, cellulolytic and amylolytic species secondary to CO administration was reported by Dong et al. (45). In vitro study carried out by Patra and Yu (8) also reported that CO exerted inhibitory effects on protozoa and cellulolytic bacteria (F. succinogenes and R. flavefaciens). This decrease might be explained by the inhibitory effect of CO on protozoa or certain bacteria species that suppress the growth of cellulolytic bacteria in the rumen. The linear decrease in NDF digestibility with CO supplementation also provides evidence for the potential inhibitory effects of CO on rumen cellulolytic bacteria.
Rumen enzyme activity is closely related to the growth status of ruminal bacteria and then affects the degradation ability to nutrient (46). In the present study, the linear decrease in the enzymatic activities of caboxymethyl-cellulase, cellobiase, xylanase, pectinase, α-amylase, and protease with increasing CO supplementation confirmed the modulation of ruminal microbial activity by CO. Additionally, the decreased enzymatic activities of caboxymethyl-cellulase, cellobiase, xylanase, and pectinase were primarily attributed to the suppression of cellulolytic bacteria growth, hence resulting in a decreased NDF and ADF digestibility. P. ruminicola and R. amylophilus are able to secrete large amounts of α-amylase (47). The linear decrease in the enzymatic activities of α-amylase noticed in this study coincided with the decrease in the total number of P. ruminicola and R. amylophilus with increasing CO supplementation. In addition, the linear decrease in protease enzymatic activities was related to the inhibitory effect of CO on proteolytic bacteria. This finding was supported by the decreased ruminal ammonia-N concentration and CP digestibility.
Serum Biochemical Parameters
The serum concentration of glucose and TC were not affected by the treatments. In contrast, studies conducted in finishing heifers (48) and lambs (10) found an increase in serum cholesterol levels following CO supplementation. The discrepancy is attributed to the difference in animals in these studies. However, the serum concentration of TGs and NEFAs linearly increased with augmentation of CO supplementation. Circulating NEFAs derived from digestive tract absorption and adipose tissue release could be used to reflect the mobilization of body fat and metabolism of fatty acids (49, 50). In the present study, the higher blood concentrations of NEFA in HCO supplementation reflected the promoting of body fat mobilization as indicated by negative BW changes compared with the goats in MCO group. Furthermore, serum concentrations of GH are affected by the nutrient level and growth performance (51). In this present study, serum concentrations of GH exhibited a linear increase with increments in CO supplementation. This supports the hypothesis that optimum CO supplementation could result in positive responses of serum GH concentration and improvement of the goat kids' growth performance.
Conclusion
In summary, CO supplementation at 6 g/day per goats is optimum in goat kids due to improved ADG and feed conversion efficiency and decreased estimated methane emission. Supplementation CO up to 8 g/day depressed growth and feed conversion due to its suppression of growth performance, rumen protozoa, cellulolytic bacteria (R. albus, R. flavefaciens, B. fibrisolvens, and F. succinogenes) and microbial enzyme activity (caboxymethyl-cellulase, cellobiase, xylanase, pectinase, α-amylase, and protease), and reduced ADF and ADF digestibility.
Data Availability Statement
The original contributions presented in the study are included in the article/Supplementary Materials, further inquiries can be directed to the Corresponding authors.
Ethics Statement
The animal study was reviewed and approved by Committee on laboratory animal ethics of Tropical Crops Genetic Resources Institute (TCGRI). Written informed consent was obtained from the owners for the participation of their animals in this study.
Author Contributions
LS, WX, and QL designed the experiment. LS, YZ, and LW conducted the experiment. YZ, TC, and GH collected and analyzed data. LS and WX prepared the manuscript. All authors contributed to the article and approved the submitted version.
Funding
This work received funding from National Natural Science Foundation of China (31802090), Central Public-interest Scientific Institution Basal Research Fund for Chinese Academy of Tropical Agricultural Sciences (1630032017035), and Project of Quality and Safety Evaluation of Tropical Feed Resources (2130109).
Conflict of Interest
The authors declare that the research was conducted in the absence of any commercial or financial relationships that could be construed as a potential conflict of interest.
Acknowledgments
The authors also thank the staff of the Key Laboratory of Crop Gene Resources and Germplasm Enhancement in Southern China, Chinese Academy of Tropical Agricultural Sciences.
Abbreviations
ADF, acid detergent fiber; ADG, average daily gain; BW, body weight; CO, coconut oil; CP, crude protein; DM, dry matter; DMI, dry matter intake; average daily feed intake, ADFI; EE, ether extract; FCR, Feed conversion ratio; GH, growth hormone; NDF, neutral detergent fiber; NEFA, non-esterified fatty acid; OM, organic matter; RT-PCR, real time polymerase chain reaction; TG, triglyceride; VFA, volatile fatty acids.
References
1. Devendra C. Concluding synthesis and the future for sustainable goat production. Small Rumin Res. (2010) 89:125–30. doi: 10.1016/j.smallrumres.200912034
2. Xu TS, Zhang XH, Gu LH, Zhou HL, Rong G, Sun WP. Identification and characterization of genes related to the development of skeletal muscle in the Hainan black goat. Biosci Biotechnol Biochem. (2012) 76:238–44. doi: 10.1271/bbb110461
3. Wang D, Zhou L, Zhou H, Hou G, Shi L, Li M., et al Effects of nutritional level of concentrate-based diets on meat quality and expression levels of genes related to meat quality in Hainan black goats. Anim Sci J. (2015) 86:166–73. doi: 10.1111/asj12251
4. Patra AK, Kamra DN, Agarwal N. Effect of plant extracts on in vitro methanogenesis, enzyme activities and fermentation of feed in rumen liquor of buffalo. Anim Feed Sci Technol. (2006) 128:276–91. doi: 10.1016/j.anifeedsci.200511001
5. Calsamiglia S, Ferret A, Reynolds CK, Kristensen NB, van Vuuren AM. Strategies for optimizing nitrogen use by ruminants. Animal. (2010) 4:1184–96. doi: 10.1017/S1751731110000911
6. Fiorentini G, Carvalho IP, Messana JD, Canesin RC, Castagnino PS, Lage JF, et al. Effect of lipid sources with different fatty acid profiles on intake, nutrient digestion and ruminal fermentation of feedlot Nellore steers. Asian-Australas J Anim Sci. (2015) 28:1583–91. doi: 10.5713/ajas150130
7. Machmüller A. Medium-chain fatty acids and their potential toreduce methanogenesis in domestic ruminants. Agric Ecosyst Environ. (2006) 112:107–14. doi: 10.1016/j.agee.200508010
8. Patra AK, Yu Z. Effects of coconut and fish oils on ruminal methanogenesis, fermentation, and abundance and diversity of microbial populations in vitro. J Dairy Sci. (2013) 96:1782–92. doi: 10.3168/jds2012-6159
9. Duckett SK, Gillis MH. Effects of oil source and fish oil addition on ruminal biohydrogenation of fatty acids and conjugated linoleic acid formation in beef steers fedfinishing diets. J Anim Sci. (2010) 88:2684–91. doi: 10.2527/jas2009-2375
10. Bhatt RS, Soren NM, Tripathi MK, Karim SA. Effects of different levels of coconut oil supplementation on performance, digestibility, rumen fermentation and carcass traits of Malpura lambs. Anim Feed Sci Technol. (2011) 164:29–37. doi: 10.1016/j.anifeedsci.201011021
11. Bhatnagar AS, Prasanth Kumar PK, Hemavathy J, Gopala Krishna AG. Fatty acid composition, oxidative stability, and radical scavenging activity of vegetable oil blends with coconut oil. J Am Oil Chem Soc. (2009) 86:991–9. doi: 10.1007/s11746-009-1435-y
12. Han J, Hamilton JA, Kirkland JL, Corkey BE, Guo W. Medium-chain oil reduces fat mass and down-regulates expression of adipogenic genes in rats. Obes Res. (2003) 11:734–44. doi: 10.1038/oby2003103
13. Jouany JP. Effect of rumen protozoa on nitrogen utilization by ruminants. J Nutr. (1996) 126:1335–46. doi: 10.1093/jn/126suppl_41335S
14. Kongmun P, Wanapat M, Pakdee P, Navanukraw C, Zu Y. Manipulation of rumen fermentation and ecology of swamp buffalo by coconut oil and garlic powder supplementation. Livest Sci. (2011) 135:84–92. doi: 10.1016/j.livsci.201006131
15. Debruyne S, Ruiz-González A, Artiles-Ortega E, Ampe B, Van Den Broeck W, De Keyser E, et al. Supplementing goat kids with coconut medium chain fatty acids in early life influences growth and rumen papillae development until 4 months after supplementation but effects on in vitro methane emissions and the rumen microbiota are transient. J Anim Sci. (2018) 96:1978–95. doi: 10.1093/jas/sky070
16. Ding X, Long R, Zhang Q, Huang X, Guo X, Mi J. Reducing methane emissions and the methanogen population in the rumen of Tibetan sheep by dietary supplementation with coconut oil. Trop Anim Health Prod. (2012) 44:1541–45. doi: 10.1007/s11250-012-0103-7
17. Liu H, Vaddella V, Zhou D. Effects of chestnut tannins and coconut oil on growth performance, methane emission, ruminal fermentation, and microbial populations in sheep. J Dairy Sci. (2011) 94:6069–77. doi: 10.3168/jds2011-4508
18. Liu H, Puchala R, LeShure S, Gipson TA, Flythe MD, Goetsch AL. Effects of lespedeza condensed tannins alone or with monensin, soybean oil, and coconut oil on feed intake, growth, digestion, ruminal methane emission, and heat energy by yearling Alpine doelings. J Anim Sci. (2019) 97:885–99. doi: 10.1093/jas/sky452
19. Faciola AP, Broderick GA. Effects of feeding lauric acid or coconut oil on ruminal protozoa numbers, fermentation pattern, digestion, omasal nutrient flow, and milk production in dairy cows. J Dairy Sci. (2014) 97:5088–100. doi: 10.3168/jds2013-7653
20. Jordan E, Lovett DK, Monahan FJ, Callan J, Flynn B, O'Mara FP. Effect of refined coconut oil or copra meal on methane output and on intake and performance of beef heifers. J Anim Sci. (2006) 84:162–70. doi: 10.2527/2006841162x
21. Shi L, Xun W, Yue W, Zhang C, Ren Y, Liu Q, et al. Effect of elemental nano-selenium on feed digestibility, rumen fermentation, and purine derivatives in sheep. Anim Feed Sci Technol. (2011) 163:136–42. doi: 10.1016/j.anifeedsci.201010016
22. Agarwal N, Kamra DN, Chaudhary LC, Agarwal I, Sahoo A, Pathak NN. Microbial status and rumen enzyme profile of crossbred calves fed on different microbial feed additives. Lett Appl Microbiol. (2002) 34:329–36. doi: 10.1046/j.1472-765X.200201092x
23. Association of Official Analytical Chemists (AOAC). Methods of Analysis. 17th ed. Arlington, VA: Association of Official Analytical Chemists (2000).
24. Van Soest PJ, Robertson JB, Lewis BA. Methods for dietary fiber, neutral detergent fiber and non-starch polysaccharides in relation to animal nutrition. J Dairy Sci. (1991) 74:3583–97. doi: 10.3168/jdsS0022-0302(91)78551-2
25. Allen MS. Effects of diet on short-term regulation of feed intake by lactating dairy cattle. J. Dairy Sci. (2000) 83:1598–624. doi: 10.3168/jdsS0022-0302(00)75030-2
26. Onetti SG, Shaver RD, Bertics SJ, Grummer RR. Influence of corn silage particle length on the performance of lactating dairy cows fed supplemental tallow. J Dairy Sci. (2003) 86:2949–57. doi: 10.3168/jdsS0022-0302(03)73892-2
27. Dohme F, Machmüller A, Wasserfallen A, Kreuzer M. Ruminal methanogenesis as influenced by individual fatty acids supplemented to complete ruminant diets. Lett Appl Microbiol. (2001) 32:47–51. doi: 10.1046/j.1472-765x.200100863x
28. Machmüller A, Kreuzer M. Methane suppression by coconut oil and associated effects on nutrient and energy balance in sheep. Can J Anim Sci. (1999) 79:65–72. doi: 10.4141/A98-079
29. Hollmann M, Beede DK. Comparison of effects of dietary coconut oil and animal fat blend on lactational performance of Holstein cows fed a high-starch diet. J Dairy Sci. (2012) 95:1484–99. doi: 10.3168/jds2011-4792
30. Dutta TK, Agnihotri MK, Rao SBN. Effect of supplemental palm oil on nutrient utilization, feeding economics and carcass characteristics in post weaned Muzafarnagari lambs under feedlot conditions. Small Rumin Res. (2008) 78:66–73. doi: 10.1016/j.smallrumres.200805002
31. Lee C, Hristov AN, Heyler KS, Cassidy TW, Long M, Corl BA, et al. Effects of dietary protein concentration and coconut oil supplementation on nitrogen utilization and production in dairy cows. J Dairy Sci. (2011) 94:5544–57. doi: 10.3168/jds2010-3889
32. Williams AG, Coleman GS. Hemicellulose-degrading enzymes in rumen ciliate protozoa. Curr Microbiol. (1985) 12:85–90. doi: 10.1007/BF01567397
34. Orpin CG. The role of ciliate protozoa and fungi in the rumen digestion of plant-cell walls. Anim Feed Sci Technol. (1984) 10:121–43. doi: 10.1016/0377-8401(84)90003-8
35. Kurihara Y, Takechi T, Shibata F. Relationship between bacteria and ciliate protozoa in the rumen of sheep fed on a purified diet. J Agric Sci Cambridge. (1978) 90:373–81. doi: 10.1017/S0021859600055489
36. Pilajun R, Wanapat M. Effect of coconut oil and mangosteen peel supplementation on ruminal fermentation, microbial protein synthesis in swamp buffaloes. Livest Sci. (2011) 141:148–54. doi: 10.1016/j.livsci.201105013
37. Atasoglu C, Newbold CJ, Wallace RJ. Incorporation of [(15)N] ammonia by the cellulolytic ruminal bacteria Fibrobacter succinogenes BL2, Ruminococcus albus SY3, and Ruminococcus flavefaciens 17. Appl Environ Microbiol. (2001) 67:2819–22. doi: 10.1128/AEM.67.62819-28222001
38. Machmüller A, Soliva CR, Kreuzer M. Effect of coconut oil and defaunation treatment on methanogenesis in sheep. Repord Nutr Dev. (2003) 43:41–55. doi: 10.1051/rnd:2003005
39. Oldick BS, Firkins JL, St-Pierre NR. Estimation of microbial nitrogen flow to the duodenum of cattle based on dry matter intake and diet composition. J Dairy Sci. (1999) 82:1497–511. doi: 10.3168/jdsS0022-0302(99)75377-4
40. Castillejos L, Calsamiglia S, Ferret A. Effect of essential oil active compounds on rumen microbial fermentation and nutrient flow in in vitro systems. J Dairy Sci. (2006) 89:2649–58. doi: 10.3168/jdsS0022-0302(06)72341-4
41. Hristov AN, Vander Pol M, Agle M, Zaman S, Schneider C, Ndegwa P, et al. Effect of lauric acid and coconut oil on ruminal fermentation, digestion, ammonia losses from manure, and milk fatty acid composition in lactating cows. J Dairy Sci. (2009) 92:5561–82. doi: 10.3168/jds2009-2383
42. Wang Y, McAllister TA. Rumen microbes, enzymes and feed digestion-a review. Asian-Aust. J Anim Sci. (2002) 15:1659–76. doi: 10.5713/ajas20021659
43. Williams AG, Coleman GS. The rumen protozoa. In: Hobson PN and Stewart CS, editors. The Rumen Microbial Ecosystem. 2nd ed. London: Blackie Academic and Professional (1997). p. 73–120.
44. Bozzolo G, Bouillier-Oudot M, Candau M. Effect of coconut oil in the post-weaning starter diet on growth and carcass qualities of male lambs weaned early and intensively fattened in winter. Reprod Nutr Dev. (1993) 33:165–81. doi: 10.1051/rnd:19930209
45. Dong Y, Bae HD, McAllister TA, Mathison GW, Cheng KJ. Lipid-induced depression of methane production and digestibility in the artificial rumen system (RUSITEC). Can J Anim Sci. (1997) 77:269–78. doi: 10.4141/A96-078
46. Zhang YL, Liu Q, Wang C, Pei CX, Li H-Y, Wang YX, et al. Effects of supplementation of Simmental steers ration with 2-methylbutyrate on rumen microflora, enzyme activities and methane production. Anim Feed Sci Technol. (2015) 199:84–92. doi: 10.1016/j.anifeedsci.201411003
47. Mentschel J, Leiser R, Mülling C, Pfarrer C. Butyric acid stimulates rumen mucosa development in the calf mainly by a reduction of apoptosis. Arch Anim Nutr. (2001) 55:85–102. doi: 10.1080/17450390109386185
48. Bindel DJ, Drouillard JS, Titgemeyer EC, Wessels RH, Löest CA. Effects of ruminally protected choline and dietary fat on performance and blood metabolites of finishing heifers. J Anim Sci. (2000) 78:2497–503. doi: 10.2527/200078102497x
49. Grummer RR. Nutritional and management strategies for the prevention of fatty liver in dairy cattle. Vet J. (2008) 176:10–20. doi: 10.1016/j.tvjl.200712033
50. Li HQ, Liu Q, Wang C, Yang ZM, Guo G, Huo WJ, et al. Effects of dietary supplements of rumen-protected folic acid on lactation performance, energy balance, blood parametersand reproductive performance in dairy cows. Anim Feed Sci Technol. (2016) 213:55–63. doi: 10.1016/j.anifeedsci.201601005
Keywords: coconut oil, growth performance, nutrient digestibility, ruminal fermentation, blood metabolites
Citation: Shi L, Zhang Y, Wu L, Xun W, Liu Q, Cao T, Hou G and Zhou H (2020) Moderate Coconut Oil Supplement Ameliorates Growth Performance and Ruminal Fermentation in Hainan Black Goat Kids. Front. Vet. Sci. 7:622259. doi: 10.3389/fvets.2020.622259
Received: 28 October 2020; Accepted: 08 December 2020;
Published: 23 December 2020.
Edited by:
Demin Cai, Yangzhou University, ChinaReviewed by:
Siaka Seriba Diarra, University of the South Pacific, FijiGiuseppe Conte, University of Pisa, Italy
Copyright © 2020 Shi, Zhang, Wu, Xun, Liu, Cao, Hou and Zhou. This is an open-access article distributed under the terms of the Creative Commons Attribution License (CC BY). The use, distribution or reproduction in other forums is permitted, provided the original author(s) and the copyright owner(s) are credited and that the original publication in this journal is cited, in accordance with accepted academic practice. No use, distribution or reproduction is permitted which does not comply with these terms.
*Correspondence: Wenjuan Xun, xunwenjuan991@163.com; Qiang Liu, liuqiangabc@163.com
†These authors have contributed equally to this work