- 1College of Veterinary Medicine, Chungnam National University, Daejeon, South Korea
- 2Department of Clinical Sciences, College of Veterinary and Animal Sciences, Jhang, Sub-Campus University of Veterinary and Animal Sciences, Lahore, Pakistan
- 3Department of Theriogenology and Biotechnology, College of Veterinary Medicine, Seoul National University, Seoul, South Korea
Extracellular vesicles (EVs) mediated intracellular communication plays an imperative role in the proper completion of different physiological events. Most of the bio-fluids are enriched with several subpopulations of EVs including exosomes and microvesicles (MVs), with the capacity of transferring different functional molecules (lipids, proteins, and nucleic acids) to target cells. Recipient cells upon receiving the signal molecules undergo different changes that positively affect the structural and functional integrity of the cells. This article was aimed to highlight the role of EVs secreted by gametes, the female reproductive tract, and the growing conceptus in the successful completion of different reproductive events related to gestation. EVs associated with the reproductive system are actively involved in the regulation of different physiological events including gamete maturation, fertilization, and embryo and fetal development. In the reproductive system, EVs mediated intracellular communication is not unidirectional but is rather regulated through crosstalk between the reproductive tract and the growing conceptus. These vesicles are secreted from the ovary, oviductal epithelium, endometrium, developing embryo, and the placenta. The cargo inside these vesicles exerts pleiotropic effects on both maternal and embryonic environments. A better understanding of the EVs-mediated crosstalk will be helpful in the development of useful tools serving both the diagnostic as well as therapeutic needs related to female fertility.
Introduction
Intercellular communication is considered imperative for the regulation and accomplishment of different physiological events including cellular proliferation and differentiation, gametogenesis, fertilization, and embryonic development (1). The success of pregnancy greatly depends on gametogenesis, fertilization, and an adequate uterine environment for embryonic development (2). These highly complex processes greatly rely on the crosstalk between the gametes and the different segments of the reproductive tract. The cells comprising a multicellular organism adopt different strategies to communicate with each other, including direct contact via membrane-bound signaling molecules and paracrine signaling via chemokines, cytokines, and hormones. Recently, an alternative mechanism has been described for intercellular communication involving the active participation of extracellular vesicles (EVs) that regulate diverse signaling pathways in targeting the cells (3, 4).
EVs are lipoproteinaceous membrane-enclosed nanometer-sized structures (5) released by almost all types of cells through different means of biogenesis. Moreover, these semi-spherical vesicles contain water-soluble components enclosed by a lipid bilayer, comprised mainly of disaturated lipids including gangliosides and sphingomyelin (6). EVs serve as a vehicle for the transportation of different proteins, lipids, RNAs (lncRNA, mRNA, small non-coding RNA, rRNA, and miRNA), and DNAs (dsDNA, ssDNA, and mtDNA) either locally or remotely (7, 8). The concentration of EVs and their cargo composition largely depend on physiological and environmental conditions (9). The contents of these vesicles can affect different physiological and pathological conditions through epigenetic and phenotypic modifications of both donor and target cells (10, 11). Furthermore, they actively participate in different biological activities such as immunomodulation, transportation, and propagation of infectious cargo including prions and retroviruses (12, 13), reproduction, candidate biomarkers of health and disease, and potential targets for therapeutics (14).
EVs are considered miniature versions of cells as they maintain the same orientation inside and outside the donor cells (15). Previously, EVs were classified based on origin, shape, size, and structural components as exosomes (40–100 nm), a product of multivesicular endosome (MVE) fusion (16, 17); microvesicles (MVs) or ectosomes (100–1,000 nm) that bud from the cell surface (18); oncosomes (1–10 μm) that protrude from the surface of cancer cells (19, 20); and apoptotic blebs (1–2 μm) released by cells undergoing apoptosis (21–23). However, the classification of EVs subtypes has been changed due to contradictory definitions and inaccurate expectations of unique biogenesis. According to the recent classification, EVs subtypes include small EVs, medium/large EVs, low-/middle-/high-density EVs, Annexin A5-stained EVs, CD63+/CD81+ EVs, podocyte EVs, hypoxic EVs, and large oncosomes (24). These vesicles have been isolated from physiological fluids including amniotic fluid (25, 26), ascites fluid (27), bile (28), blood (29), breast milk (30), cell cultures (31), cerebrospinal fluid (32, 33), plasma (34), saliva (35, 36), semen (37–39), urine (40, 41), and pathological effusions of the tumor (27). EVs secreted by donor cells can travel through the body fluids to deliver their contents to the target cells without degradation.
Recently, EVs such as exosomes and MVs have been isolated from the different segments of the male and female reproductive systems. EVs derived from the reproductive system regulates different reproductive events such as sperm maturation (42, 43), sperm capacitation and acrosome reaction (44, 45), oocyte maturation (46), fertilization (1), recognition of pregnancy (47, 48), implantation of pregnancy (49, 50), maintenance of pregnancy, and parturition (51). Moreover, EVs have been reported to regulate different reproductive pathologies such as early pregnancy loss, endometriosis, erectile dysfunction, gestational diabetes mellitus, gestational vascular complications, hypertension, polycystic ovaries, and preeclampsia (52). These pathological conditions may compromise fertility and even result in termination of pregnancy. EVs associated with the different phenomenon of reproductive physiology and pathology can potentially severe as biomarkers and vectors for drug delivery. Recently several reports have elucidated the effectiveness of EVs as mediators of the different phenomenon of reproductive physiology. The objective of this review is to highlight the potential roles of EVs in maternal reproductive physiology and their implications on successful pregnancy.
EVs and Sperm Maturation
Mammalian testicles produce fully differentiated sperm; however, they lack motility and fertility. Sperm become fully functional as they transit through the different segments of the male reproductive system (53). EVs secreted by the male reproductive tract including epididymosomes and prostasomes play a key role in the maturation process of sperm (40, (54)). Moreover, prostasomes contain chromogranin B responsible for the bactericidal activity and prevent immune cells to recognize sperm in the female reproductive tract (55). On the other side, after coitus sperm are exposed to the different segments of the female reproductive tract as they move from the vagina to the fertilization site after breeding. During their transit, the sperm plasma membrane experiences certain biochemical modifications causing remodeling of their surface molecules (56). These modifications occur possibly due to the crosstalk between the sperm and the female reproductive tract and are considered vital for functional maturity and fertility acquisition.
Recently, EVs have been isolated from the uterine (uterosomes) and oviductal (oviductosomes) fluids of murine (57, 58). These vesicles showed the expression of certain sperm essential proteins including sperm adhesion molecule 1 (SPAM1) and plasma membrane calcium pump (PMCA4). In vitro studies have confirmed the transfer of fertility regulating proteins from these EVs to sperm (57, 58). Moreover, recent studies have demonstrated the potential of EVs in preserving the structural and functional integrity of sperm against the damages induced during the freezing procedures (59, 60).
Uterosomes are EVs isolated from the uterine fluids (UFs) that are classified as both exosomes and MVs (40, 57). The cargo contained in uterosomes mainly includes nucleic acids such as mRNAs and miRNAs (61) and proteins such as SPAM1 and PMCA4a (57). Estrogen (E2) hormone is believed to be involved in regulating the expression of these macromolecules (40, 62). It was hypothesized that uterosomes are responsible for sperm capacitation, membrane stabilization (63), and final sperm maturation via miRNA transfer (64) (Table 1). Moreover, the presence of SPAM1 protein suggests the possible role of uterosomes in the inhibition of a premature acrosomal reaction during the uterine transit of the sperm (64).
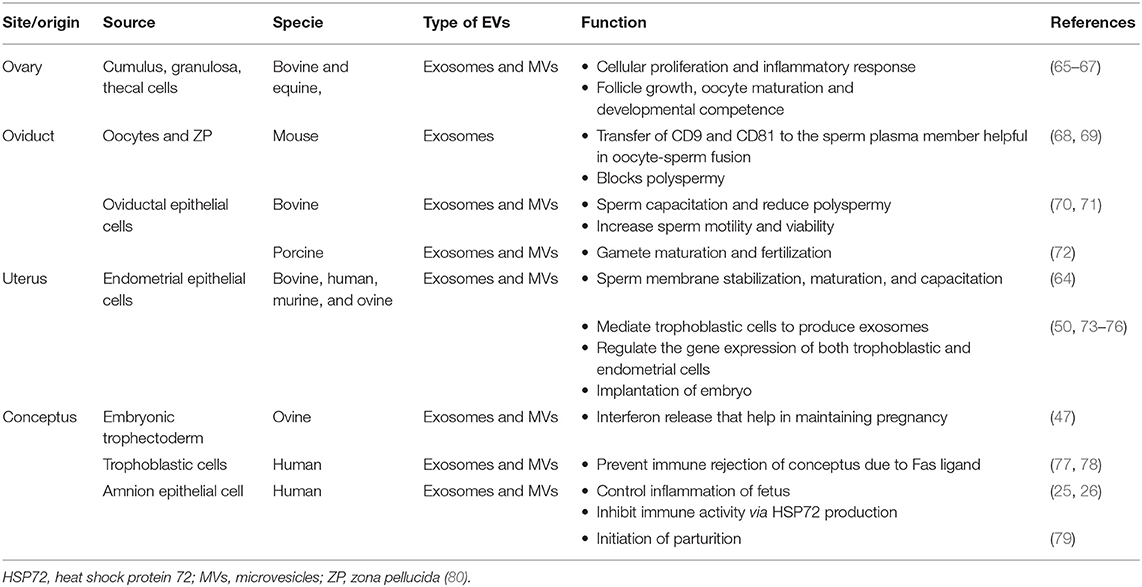
Table 1. Production and physiological roles of extracellular vesicles (EVs) in female reproductive tract.
Based on the shape, size, and expression of surface markers, oviductosomes are characterized as both exosomes and MVs (81). The cargo packed inside the oviductosomes includes proteins αV integrin (cell surface receptor), CD9 tetraspanin (adhesion molecule), heat shock protein A8 (HSPA8, & PMCA4), lactadherin (P-aminosalicylic acid, PAS6/7; or milk fat globule-epidermal growth factor-8, MFGE8), oviductal specific glycoprotein (OVGP), lipids, SPAM1, RNAs, and miRNAs (80). Oviductosomes-derived proteins are involved in different physiological events associated with sperm, such as SPAM1 involved in acrosome reaction (64); OVGP, increases sperm viability and motility (Table 1) (70), reduces the incidence of polyspermy through zona hardening (71), induces the phosphorylation of sperm-associated proteins during capacitation (82, 83), and modulates fertilization (84); HSPA8, affects fertilization and early embryo development (85); HSP90, interacts with the zona pellucida (ZP) of oocytes (86); PMCA4, associated with Ca2+ ion efflux ultimately affecting motility and fertility (87); and lactadherin, involved in ZP binding (88).
EVs and Oocyte Maturation
Ovarian follicles maturation and oocytes growth are highly associated (89) and considered crucial for effective embryo production (90). These highly complex processes are regulated by an extensive intercellular communication within the microenvironment of the follicle along with the functional and structural transformation of different cell types constituting the follicle (91). The crosstalk between oocytes and the surrounding follicular cells (theca and granulosa) is important for oocyte development (92), occurring mainly through gap junctions' proteins. Moreover, the follicular fluid comprising of different ions, metabolites, nucleic acids, and proteins, serves as an additional means of communication between oocytes and follicular cells. Oocytes achieve developmental competence through different signal transductions and molecular interactions that are mediated through the follicular fluid (93). These molecular interactions involve two-way traffic of certain crucial factors. These factors affect both the folliculogenesis and initiation of different signaling pathways involving different molecules such as insulin, transforming growth factor-beta (TGFB) and wingless/Int (WNT) signaling members (94, 95), growth factors (growth differentiation factor, GDF9; bone morphogenetic protein 4, BMP4) (96), and hormones (97).
Recent studies demonstrated the EVs including exosomes and MVs (small EVs) derived from the follicular fluid (65) and oocytes (68, 98). These vesicles mediate intercellular communication associated with follicular development and oocyte quality (65, 66, 99). In bovine, a negative correlation was reported between the exosomal concentration and follicular size (67). Moreover, the exosomes found in the bovine follicular fluid have been shown to affect the transcript abundance in oocytes and adjacent granulosa cells (100). Supplementation of in vitro maturation (IVM) medium with follicular fluid has been reported to increase the expansion of cumulus cells (101), stimulate granulosa cells proliferation (102), and enhance the blastocyst development rates (103). Rodrigues and his co-workers reported similar findings (104). The exosomal fraction of follicular fluid was believed to be the main reason for these improvements.
EVs indirectly affect the competence of oocytes by improving the function of cumulus cells (104). An in vitro study demonstrated the uptake of EVs in cumulus cells and their associated transzonal projections (103). During the early stages of oocyte maturation, transzonal projections extend from the cytoplasm of cumulus cells and mediate RNA transfer between cumulus cells and the oocyte (105). In addition to RNA, some researchers also propose that cytokines present in the exosomes regulate the various physiological aspects including proliferation and differentiation of cells, survival or atresia of follicles, and maturation of oocytes (106, 107). Recent studies confirmed the exosome-mediated transfer of miRNA (66) and mRNA (108) to bovine granulosa cells.
Follicular fluid exosomes have been reported to possess cytoprotective effects against stress (104). Under stressful conditions, the cellular contents are modified and the cell experiences an increased secretion of EVs that enhance the defense system and prevent cell death (109, 110). During oxidative stress, the granulosa cells-derived EVs showed a higher proportion of antioxidants and other substances associated with cellular defense as compared to the exosomes secreted during normal conditions (Table 2) (111). Similarly, another study identified the protective nature of follicular fluid exosomes against oxidative stress (108). In vitro treatment of oocytes with follicular fluid-derived exosomes reduced the apoptosis of cumulus cells and damage to oocytes caused by heat shock (104).
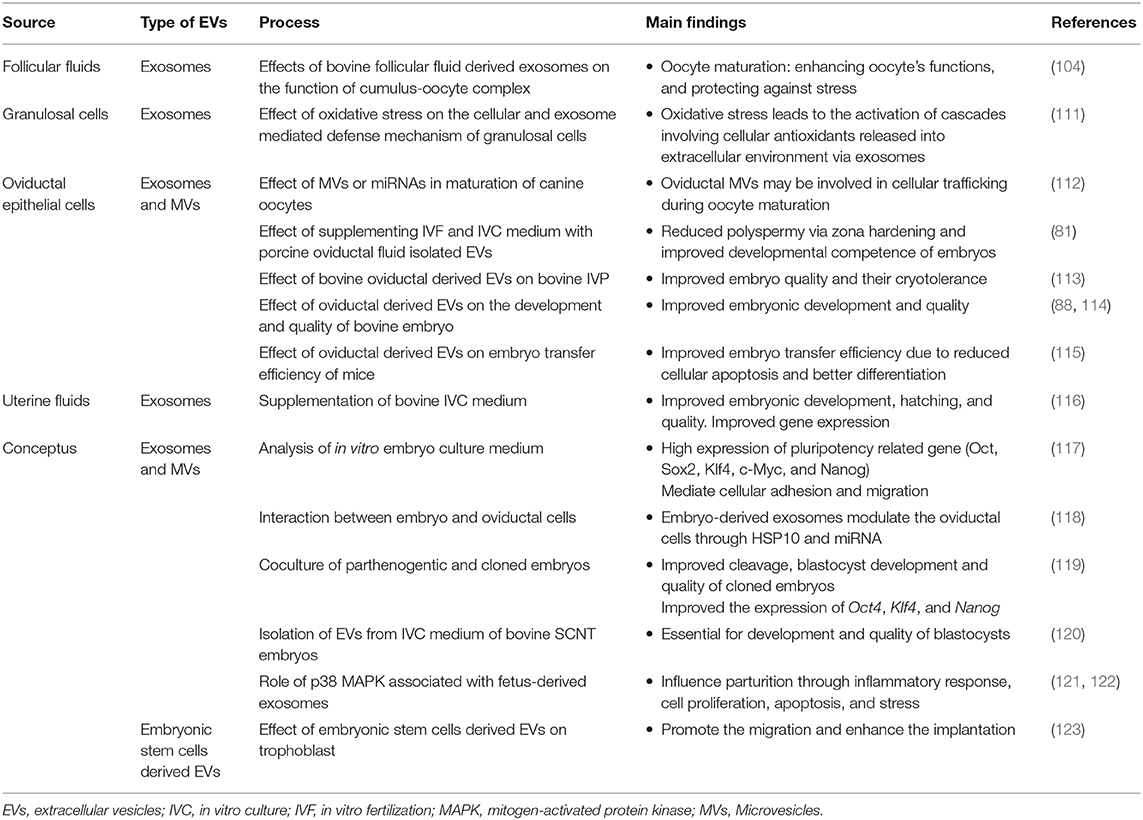
Table 2. In vitro studies involving the use of extracellular vesicles (EVs) isolated from luminal fluids of different segments of female reproductive tract.
Oviductosomes are considered as key players involved in the regulation of the gametes-oviduct interaction (64, 88). In contrast to other mammalian species, the canine oocytes are ovulated in an immature form. For acquiring developmental competence, oocytes need both intra- and extra-follicular (oviduct) environments (124). Therefore, the role of oviductosomes in oocytes maturation and survival may be more pronounced in canines as shown in Figure 1. A recent study revealed that supplementation of IVM medium with oviduct-derived MVs was beneficial to the maturation of canine oocytes as involved in cellular communications (112). It was found that MVs were uptake by ooplasm after 72 h of maturation, demonstrating their role in improved maturation.
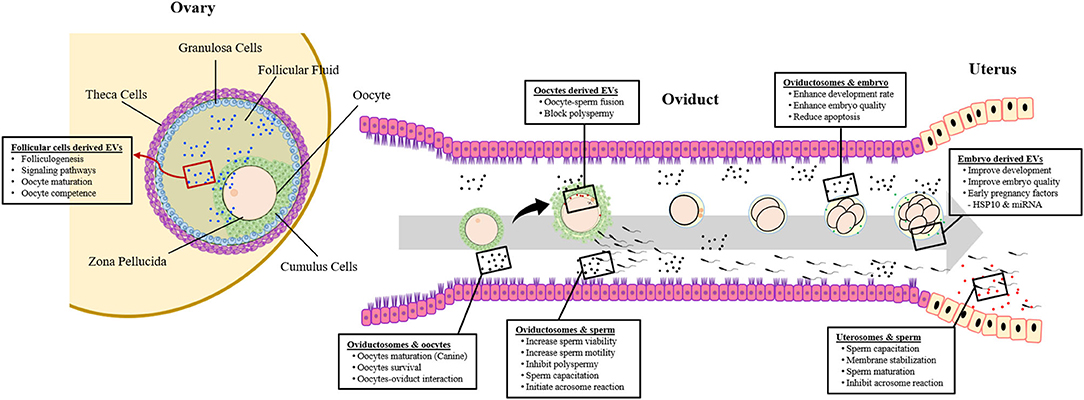
Figure 1. Extracellular vesicles (EVs) and crosstalk between gametes, embryo, and maternal reproductive system.
EVs and Fertilization
The fertilization process involves the fusion of haploid gametes (male and female) within the microenvironment of the oviduct to produce a genetically distinct individual. Fertilization consists of different events occurring in a precise order, such as the expansion of cumulus cells to facilitate sperm passage, attachment of sperm with ZP (125), and finally penetrating ZP. The acrosomal reaction is initiated as capacitated sperm binds with the ZP resulting in the biochemical modification of the sperm plasma membrane. All these changes enable the sperm to recognize the ZP and allows the entry of sperm into the perivitelline space (126–129). Within the perivitelline space, the sperm fuses with the oocyte's membrane resulting in the depolarization of ZP. The depolarized ZP triggers the cortical granules to release their contents such as plasminogen, acid phosphatase, peroxidases, etc., which helps to avoid polyspermy.
Reports indicate that oocytes secrete a limited number of EVs that interact with the other cells present in the close vicinity of the oocyte (55). During oocyte-sperm fusion, oocyte-derived EVs are secreted into the perivitelline space (68) and ultimately transferred to the sperm plasma membrane. Transfer of oocytes-derived EVs to the sperm plasma membrane is considered vital for oocyte-sperm fusion (Table 1). Tetraspanins including CD9 and CD81 have been reported to mediate the oocyte-sperm fusion process. Before fusion, CD9 primarily produced by the oocyte is mainly localized in the oocyte's plasma membrane especially in the region of the microvilli, whereas CD81 produced by the cumulus cells is localized in the inner region of ZP (130). When sperm penetrates the perivitelline space both CD9 and CD81 are transferred to the sperm plasma membrane via EVs (130, 131). The suggested role of CD9 in the regulation of gametes fusion was well-supported by the fact that CD9 deficient oocytes have structurally altered microvilli and cannot fuse with the sperm (132). CD81 participates in fertilization, especially in fusion-related events like acrosome reaction (133). It is also believed that CD81 may facilitate the transfer of CD9 from the oocytes to the sperm plasma membrane (130).
Masaru Okabe's group identified Izumo 1 (named after a Japanese shrine), a member of the tetraspanin family, to be associated with oocyte-sperm fusion (134). Izumo 1 is localized on the surface of sperm that had undergone the acrosomal reaction. A deficiency of Izumo protein results in the failure of sperm to fuse with the oocyte. In an attempt to understand the role of Izumo in oocyte-sperm fusion, researchers identified the binding partner of Izumo on the surface of an oocyte named Juno (69). In the same study, it was found that the interaction between Izumo and Juno receptors is essential for mammalian fertilization, as oocytes lacking Juno are unable to fuse with the normal acrosome reacted sperm. Moreover, Juno receptors are involved in the prevention of polyspermy. Once fertilization occurs, the receptivity of fertilized oocyte shuts down for the additional sperm. Juno receptors are highly expressed on the surface of oocytes before fertilization. The fusion of gametes is followed by the shedding of these receptors from the oocyte's membrane and redistribution within the field of vesicles localized in the perivitelline space. It is believed that Juno conjugated vesicles bind and neutralize the incoming acrosome reacted sperm (69).
A recent study demonstrated that the porcine oviductosomes showed the expression of oviduct specific glycoprotein (OVGP1), ribosomal protein S5 (RPS5), myosin heavy chain 9 (MYH9), and valosin containing proteins (VCP) (81). OVGP1 in association with MYH9 can bind with the gametes (72), indicating their possible role in gamete maturation and fertilization. Whereas, RPS5 and VCP proteins are involved in protein synthesis (135, 136). The same study revealed that the supplementation of the in vitro fertilization (IVF) medium with porcine oviductosomes reduced the incidence of polyspermy by the hardening of ZP. Similar findings were observed when the IVF medium was supplemented with porcine oviductal fluids (137, 138) or conditioned medium isolated from oviductal cells culture (139). The glycoprotein structure of ZP was modified by either OVGP1, lactoferrin, and osteopontin binding (140) or OVGP1 and MYH9 proteins derived from EVs coupled together and bind with ZP to exert their effect (81).
EVs and Preimplantation Embryonic Development
The physiological conditions during early embryonic development are considered crucial as their effects are translated in the subsequent growth. A successful pregnancy requires synchronized communication between the embryo and the female reproductive system. Any disturbance in this communication can either result in defective growth or lead to pregnancy termination (141). Recent studies have confirmed the active involvement of EVs in embryo-maternal cross talks. EVs identified in oviductal (44, 114) and uterine (50, 61) fluids, regulate the embryo-maternal interactions (64, 73). The composition of EVs contents varies according to the stage of pregnancy (74). Moreover, the preimplantation embryos are believed to produce EVs based on the high levels of EVs isolated from the embryo culture medium (119, 142). However, some research groups deny this fact, as they believe that EVs isolated from the culture medium may arise from the nutritional supplements used for embryo growth.
Oviduct-Derived EVs and Embryonic Development
Being the fertilization site, the oviduct represents the first environment to which embryos are exposed and also the site where embryo-maternal interactions start (143). Oviduct-derived EVs play an important role in mediating the embryo–maternal interactions during early embryonic development, leading to improved embryo quality and successful pregnancy (Figure 1). Almiñana et al. demonstrated the production of oviductal fluid EVs during both in vivo and in vitro conditions (88). Further experiments revealed an enhanced development rate and quality of embryos following supplementation of in vitro culture (IVC) conditions with in vivo-derived oviductosomes. It was concluded that in vitro-derived embryos can uptake the in vivo derived EVs from oviductal epithelial cells, resulting in improved competence. Similarly, improved development, quality, and cryo-tolerance of in vitro produced embryos were observed using EVs derived from bovine oviductal epithelial cells both in vivo and in vitro conditions (113, 114). Furthermore, the oviductal cells-derived EVs improved the efficiency of embryo transfer in mice, through reduced apoptosis and better differentiation of embryonic cells (115).
Uterus-Derived EVs and Embryonic Development
Following early embryonic development in the oviduct, the morula stage embryo enters the uterus on day 5 in bovine (144), and day 4–6 in ovine (73). Once in the uterus, the preimplantation embryo is dependent on the UFs secreted by the glandular cells lining the uterus (145). UFs contains amino acids, growth factors, lipids, proteins (146), and EVs containing bioactive substances required for embryonic development (50, 55, 147). EVs isolated from endometrial-epithelial cells and uterine mucus not only facilitate endometrium-embryo crosstalk but also help in the implantation of the embryo (50).
As the embryo arrives in the uterus intense embryo-maternal crosstalk starts, necessary for the maternal recognition of pregnancy. Burn and his coworkers demonstrated that during the early stage of pregnancy (15–17 days) in sheep, both trophectoderm and uterine epithelial cells actively communicate with each other via EVs (73). The endometrial epithelial cells of pregnant sheep produce exosomes containing endogenous jaagsiekte retroviruses (enJSRV) mRNA that causes the trophoblastic cells to release EVs containing interferons (47). As illustrated in Figure 2, interferons are cytokines having paracrine activity; causing reduced expression of estrogen (E2) and oxytocin receptors essential for the life of corpus luteum (CL) and progesterone (P4) production (148). P4 is a steroid hormone secreted by the CL required for pregnancy maintenance, elongation, and survival of conceptus (149). In addition to pregnancy supporting functions, P4 influences the secretions of EVs from the endometrial epithelial cells. In sheep, increased secretion of EVs from endometrial epithelial cells was observed during 10–14 days of the estrous cycle, the period of P4 dominance (150). Moreover, P4 up-regulates the miRNAs contents of EVs isolated from UFs that are supposed to modulate the BMP, phosphoinositide 3-kinase (PI3K), serine, or threonine kinase 1, and post-transcriptional silencing (150).
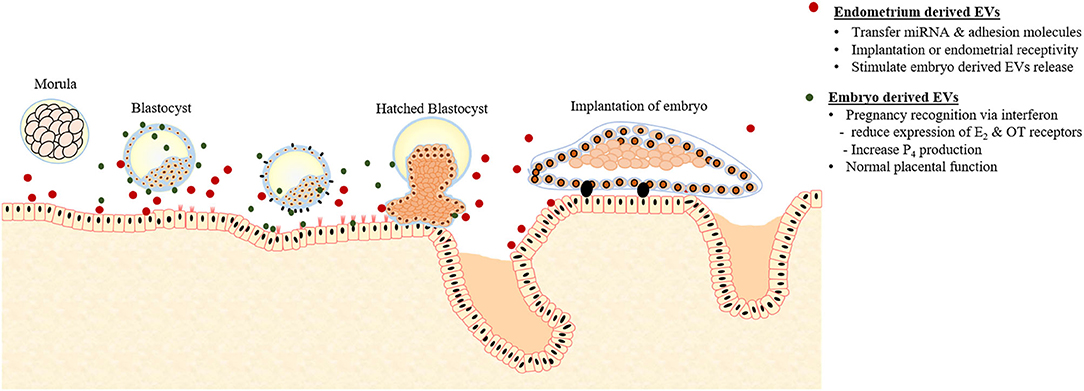
Figure 2. Extracellular vesicles (EVs) mediated crosstalk between early embryo and endometrium responsible for pregnancy recognition and implantation.
Furthermore, it was purposed that the EVs found in the UFs during early pregnancy might be involved in regulating the gene expression of trophoblastic cells and endometrial epithelial cells (75, 76). A recent study demonstrated that the supplementation of IVC medium with bovine UFs-derived EVs significantly improved the blastocyst development and hatching, and embryo quality in terms of cell number, and reduced apoptosis (116). Moreover, blastocysts treated with UFs-derived EV showed enhanced expression of interferon and acrogranin producing genes and reduced the expression of BAX and HSP70 genes.
Embryo-Derived EVs and Embryonic Development
In vitro studies have revealed higher success rates of embryo production when culture frequency was kept higher throughout the culture period (151, 152), suggesting the possible existence of the embryo to embryo communication. Moreover, in vitro produced mammalian embryo can regulate its development independently until the hatching stage (153). It was believed that embryos develop their microenvironment through the release of different growth factors with autocrine and paracrine effects (142, 154, 155). Research evidence have demonstrated the isolation of EVs from the in vitro embryo culture medium on day 3 and 5 post culture (117, 156). Transcript analysis revealed high expression of pluripotency related genes including OCT4, SOX2, KLF4, C-MYC, and NANOG (Table 2). Moreover, the cargo was also comprised of a variety of miRNA species that mediate cellular activities such as adhesion and migration (Figure 2). The number of embryo-derived EVs increases with the developmental stage, whereas their size depends on the quality of the embryo (157).
A previous study suggested that the equine embryos at day 8 produce exosomes that interact and modulate the function of oviductal epithelial cells through the transfer of early pregnancy factor (HSP10) and miRNA in vitro (118). Similarly, the conceptus-derived EVs were isolated from the bovine UFs on days 15 and 17 of pregnancy (158). These EVs contained proteins that can modify the endometrial response and are unique to the existence of conceptus such as Aldo-keto reductase family 1, macrophage-capping protein (CAPG), member B1 protein (AKR1B1).
The cloned porcine embryos showed a significant increase in the development competence when co-cultured with parthenogenetic embryos (119). The co-cultured environment improved the cleavage and blastocysts formation rates, cell number, and higher expression of reprogramming related genes OCT4, KLF4, and NANOG than the embryos cultured alone (Table 2). Analysis of the culture medium of individual embryo showed the presence of CD9-positive vesicles. It was concluded that these vesicles are responsible for the transfer of mRNA cargos between embryos as these vesicles can cross the ZP and finally internalized by blastomeres. Another study about bovine revealed that embryo-derived EVs exert a positive effect on growth, survival, and pregnancy rates (120). In addition, EVs supplemented medium resulted in a significantly improved rate of blastocyst development and embryonic quality in terms of higher cell numbers.
EVs, Embryo Implantation, and Fetal Development
EVs and Implantation
EVs derived from the endometrial epithelial cells play an active role in the transfer of signaling miRNAs and adhesion molecules either to blastocysts or to the adjacent endometrial cells affecting endometrial receptivity or implantation (Figure 2). The proteins contents of EVs derived from endometrium during the implantations stage contain a large number of adhesion molecules compared to the preimplantation stage EVs that contain proteins responsible for apoptosis (74). Moreover, treating endometrial epithelial cells with the EVs isolated from the UFs during the post-implantation period induced and up-regulated the expression of adhesion molecules such as vascular cell adhesion molecule 1 (VCAM) (74).
In a study, EVs isolated from the UFs of both cyclic and pregnant sheep reported containing miRNAs and proteins that are expressed by both trophectoderm of the conceptus and maternal endometrial epithelial cells (61). Similarly, another study identified that the endometrial epithelial cells are involved in the release of EVs in UFs. The cargo of these EVs includes specific miRNA populations such as has-miR-200c, has-miR-17, and has-miR-106a that are also found in the microenvironment of the embryo during implantation. It was hypothesized that these EVs may are involved in endometrial-embryo crosstalk during implantation (50). In mouse, the embryonic stem (ES) cells derived from the inner cellular mass (ICM) reported to secrete EVs containing fibronectin and laminin (123). These proteins interact with the integrin on the surface of the trophectoderm promoting the trophoblast migration. Furthermore, injecting ES cell-derived EVs in the blastocyst prior to injection in surrogate mother enhanced the implantation rate.
Placenta Derived EVs and Fetal Development
Following implantation, the placenta provides endocrine, nutritional, and oxygen support to the growing fetus. The placental cells including cytotrophoblasts, syncytiotrophoblasts, and mesenchymal stem cells reported to produce EVs that may also modify maternal physiology and fetal development (159). In sow, endometrial epithelial cells, maternal endothelial cells, chorioallantois, and trophectoderm secrete EVs on day 20th of pregnancy (160). Trophoblasts derived EVs carry molecules involved in the normal placental function. These EVs contain miRNAs and several important proteins that can modulate the angiogenesis within the trophectoderm. Moreover, the trophectoderm derived EVs penetrate and stimulate the proliferation of maternal endothelial cells. These molecules include fibronectin, which triggers pro-inflammatory events in early pregnancy (161); syncitin, associated with membrane fusion in syncytiotrophoblasts (162); Wnt/βcatenin-related molecules, involved in cell fusion (163); galectin-3, the interaction between placenta and endometrium (164); HLA-G5, immunosuppressive (162); prostaglandin E2, immunosuppressive; and 15d-PGJ2, a ligand to nuclear receptor PPARγ involved in parturition (165).
Placenta-derived EVs enter the maternal blood as early as the 6th week of gestation and are continuously secreted throughout the whole gestation (166). Taylor and his coworkers reported EVs in the maternal serum at 28–30 weeks of gestation (167). A recent in vitro study showed that the supplementation of IVC medium with pregnant sow serum inhibits apoptosis and promotes the cytoplasmic reorganization and differentiation of parthenogenetic embryos (168). Factors such as oxygen tension and glucose concentration regulate the release of EVs from the placenta. The concentration of placenta-derived EVs depends on the stage and physiological status of pregnancy. EVs concentration increases as pregnancy progress and is correlated with the mean blood flow through the uterine artery and weight of the placenta. During the first trimester of pregnancy, there is a 50-fold increase in the EVs concentration in maternal plasma (169). However, the plasma concentration of EVs and their associated bioactivity decreases in late pregnancy. EVs concentration was higher in the pregnancies that proceed normally till term than pregnancies that terminated preterm. Moreover, the concentration of placenta-derived EVs and their cargo composition is also associated with placental dysfunction. The EVs produced during the first trimester were more bioactive in promoting endothelial cell migration compared to the EVs produced during the second and third trimester (170). Therefore, fetal growth and placental function can be estimated by the quantification of EVs in maternal plasma.
Several studies showed excessive production of EVs during pregnancy with immunoregulatory properties for preventing the immuno-rejection of the fetus by the maternal immune system. During the first trimester, these vesicles contain Fas ligand (77, 78) that reacts with the maternal T lymphocytes to cause apoptosis and prevents anti-fetal immune response. Moreover, EVs also inhibit the expression of signal transduction molecules of T cells such as CD3 and JAK3 (167). The amniotic fluid is rich in EVs due to the secretion of fetal waste products and urine as shown in Figure 3 (25). It is believed that these EVs are involved in controlling the inflammation of different fetal compartments (Table 1). During the second trimester, EVs found in the amniotic fluid contain HSP72 that is a potent inhibitor of immune activity (Table 1) (26). In addition, amniotic fluid exosomes contents can reflect the physiological or pathological state of pregnancy around parturition (171). Amniotic fluid exosomes from different pathological conditions (spontaneous preterm birth, preterm premature rupture of membranes) and physiological conditions had different contents and exhibited different canonical pathways. These findings prove that amniotic fluid exosomes can be used as a diagnostic tool for preterm pathologies.
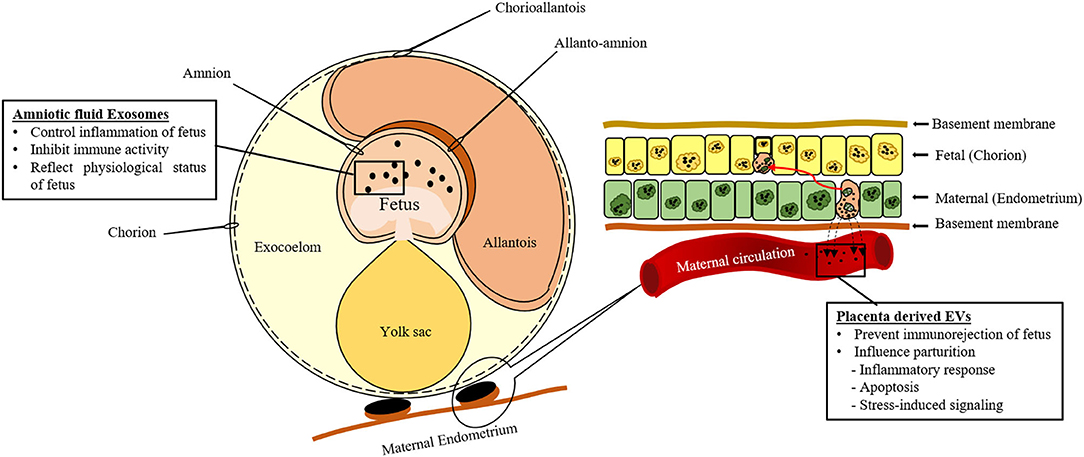
Figure 3. Schematic representation of extracellular vesicles (EVs) secreted by the conceptus during gestation.
EVs and Parturition
The mechanisms initiating parturitions are complex and involve both maternal and fetal sides. Leukocytes invasion, fetal endocrine factors, P4 withdrawal, and inflammation are the major events that trigger the switch of state from quiescent to active in gestational tissues, but little is known about the exact mechanisms involved in this transition. The hypothalamic-pituitary-adrenal axis is important to labor triggering factor and is already well-documented (172, 173). In mammals, except humans, parturition is initiated as serum P4 level drops down (174). In humans, the P4 level remains the same, but its function is blocked, a concept referred to as “Functional P4 withdrawal” (175). The withdrawal of P4 is followed by a cascade of endocrine changes, including E2 concentration increase, cortisol, prostaglandin, and oxytocin release, which ultimately lead to the cervical changes, uterine contractions, and membranes rupture (176). These events are also emphasized by the inflammatory reactions on the feto-maternal side (177) and EVs might play a role in these inflammatory processes.
EVs originate from both maternal and fetal sides and keep increasing throughout the pregnancy (178). These EVs carry pro-inflammatory factors that will subsequently influence the outcome of pregnancy by inducing pre-term or term parturition (169). On the maternal side, studies showed that parturition is associated with an increase in pro-inflammatory cytokines (179–181). One of the studies showed leukocyte invasion and increase mRNA expression of IL-1b, IL-6, and IL-8 in the cervix and myometrium near parturition (179). However, the cascade initiating this sterile inflammatory reaction was not investigated in these studies. Placenta-derived EVs might carry pro-inflammatory mediators that participate in the initiation of parturition, although research on the characterization of the different canonical pathways where these exosomes might be implicated are still ongoing (51).
Fetal cells exosomes from an amnion epithelial cell line can also induce an inflammatory reaction when delivered to myometrial and decidual cells (79). As fetal growth and maturation go by throughout the pregnancy, telomerase activity is reduced in fetal membranes, which leads to cellular aging and the expression of a senescence-associated secretory phenotype (SASP). It seems that oxidative stress-induced fetal cells would express senescence factors and thus inflammatory factors that would be conveyed through their exosomes to the maternal cells may initiate parturition. The cargo composition of the EVs derived from fetal cells exposed to oxidative stress were screened in another study (121). They demonstrated that p38 MAPK (mitogen-activated protein kinase) was found in high concentrations in the isolated EVs and the main factor influencing parturition. Moreover, p38 isoform is implicated in inflammatory responses, cell proliferation, apoptosis, and stress-induced signaling (122).
Regardless of their origin, late gestation exosomes release their cargo in specific sites, inducing inflammatory reactions in those sites only, subsequently avoiding systemic inflammation (178). The mechanisms behind the tropism exhibited by exosomes have not been elucidated yet, but Carboxyfluoroscein succinimidyl ester (CFSE)-labeled exosomes were localized in specific tissues: connective tissue of the maternal cervix, myometrium in the uterus, a labyrinth in the placenta and the epithelial layer in the fetal membranes. The same study showed that pre-term period exosomes, extracted at Day 18 of pregnancy, could influence the onset of parturition when injected on Day 15–16, regardless of the withdrawal of plasma P4 (178). This suggests that late gestation exosomes trigger the events that remodel and prepare the cervix and uterus for parturition (178).
The foregoing suggests that EVs are as important as endocrine factors in fetal-maternal communication especially in the initiation of parturition through their inflammatory effects. Pre-term exosomal cargo contains the highest level of inflammatory mediators when compared to the cargo from early pregnancy stage and the proteins contained there are implicated in biological functions that are essential to the late gestation period (leucocyte activation, neutrophils infiltration, chemotaxis, and cell movement) (178).
Conclusions
This review highlights the biological roles of EVs in different events of female reproductive physiology associated with the pregnancy. There is growing evidence in the literature that the functional molecules carried by the EVs can modulate different reproductive events such as gametes maturation, fertilization, blockage of polyspermy, development, and implantation of the embryo, fetal development, and parturition. In addition, EVs can serve as excellent carrier for drug delivery due to the ability to transfer their contents to the target cells. Based on the physiological role, EVs supplementations can be used to overcome the deficiencies and enhance the outcomes of in vitro embryo production. Moreover, EVs concentration and their contents can reflect the physiological or pathological state of different reproductive events. It can be helpful in the estimation of pregnancy term, fetal growth, placental function, and diagnosis of different pathological conditions. However, the available data regarding the potential role of EVs in reproductive physiology and pathology is still limited and requires further investigations. As better understanding of EVs mediated communication can improve the diagnostics and therapeutics for fertility related issues, pregnancy-associated abnormalities, and pregnancy loss.
Author Contributions
JC, MJK, and AYQ designed the manuscript, performed the literature review, and drafted the manuscript. JC and MJK supervised and critically reviewed the manuscript. AYQ, FYM, SB, SS, and XF participated in drafting, critically revising, and discussed the manuscript. All authors approved the manuscript for publication. All authors contributed to the article and approved the submitted version.
Funding
This research was supported by the funding provided by the Cooperative research program of RDA (CCAR), grant numbers PJ013954012019 and PJ014786012020.
Conflict of Interest
The authors declare that the research was conducted in the absence of any commercial or financial relationships that could be construed as a potential conflict of interest.
Acknowledgments
The authors would like to acknowledge the funding agency for their financial support.
References
1. Machtinger R, Laurent LC, Baccarelli AA. Extracellular vesicles: roles in gamete maturation, fertilization and embryo implantation. Hum Reprod Update. (2016) 22:182–93. doi: 10.1093/humupd/dmv055
2. Fortune J. Ovarian follicular growth and development in mammals. Biol Reprod. (1994) 50:225–32. doi: 10.1095/biolreprod50.2.225
3. Février B, Raposo G. Exosomes: endosomal-derived vesicles shipping extracellular messages. Curr Opin Cell Biol. (2004) 16:415–21. doi: 10.1016/j.ceb.2004.06.003
4. Montecalvo A, Larregina AT, Shufesky WJ, Beer Stolz D, Sullivan ML, Karlsson JM, et al. Mechanism of transfer of functional microRNAs between mouse dendritic cells via exosomes. Blood. (2012) 119:756–66. doi: 10.1182/blood-2011-02-338004
5. Kurian NK, Modi D. Extracellular vesicle mediated embryo-endometrial cross talk during implantation and in pregnancy. J Assist Reprod Genet. (2019) 36:189–98. doi: 10.1007/s10815-018-1343-x
6. Laulagnier K, Motta C, Hamdi S, Roy S, Fauvelle F, Pageaux J-F, et al. Mast cell-and dendritic cell-derived exosomes display a specific lipid composition and an unusual membrane organization. Biochem J. (2004) 380:161–71. doi: 10.1042/bj20031594
7. Raposo G, Stoorvogel W. Extracellular vesicles: exosomes, microvesicles, and friends. J Cell Biol. (2013) 200:373–83. doi: 10.1083/jcb.201211138
8. Xu R, Greening DW, Zhu H-J, Takahashi N, Simpson RJ. Extracellular vesicle isolation and characterization: toward clinical application. J Clin Investig. (2016) 126:1152–62. doi: 10.1172/JCI81129
9. Zappulli V, Friis KP, Fitzpatrick Z, Maguire CA, Breakefield XO. Extracellular vesicles and intercellular communication within the nervous system. J Clin Investig. (2016) 126:1198–207. doi: 10.1172/JCI81134
10. Lee Y, El Andaloussi S, Wood MJ. Exosomes and microvesicles: extracellular vesicles for genetic information transfer and gene therapy. Hum Mol Genet. (2012) 21:R125–34. doi: 10.1093/hmg/dds317
11. Yáñez-Mó M, Siljander PR-M, Andreu Z, Bedina Zavec A, Borràs FE, Buzas EI, et al. Biological properties of extracellular vesicles and their physiological functions. J Extracell Vesicles. (2015) 4:27066. doi: 10.3402/jev.v4.27066
12. Wiley RD, Gummuluru S. Immature dendritic cell-derived exosomes can mediate HIV-1 trans infection. Proc Natl Acad Sci USA. (2006) 103:738–43. doi: 10.1073/pnas.0507995103
13. Simpson RJ, Jensen SS, Lim JW. Proteomic profiling of exosomes: current perspectives. Proteomics. (2008) 8:4083–99. doi: 10.1002/pmic.200800109
14. Gould SJ, Raposo G. As we wait: coping with an imperfect nomenclature for extracellular vesicles. J Extracell Vesicles. (2013) 2:20389. doi: 10.3402/jev.v2i0.20389
15. Théry C, Ostrowski M, Segura E. Membrane vesicles as conveyors of immune responses. Nat Rev Immunol. (2009) 9:581–93. doi: 10.1038/nri2567
16. Pan B-T, Teng K, Wu C, Adam M, Johnstone RM. Electron microscopic evidence for externalization of the transferrin receptor in vesicular form in sheep reticulocytes. J Cell Biol. (1985) 101:942–8. doi: 10.1083/jcb.101.3.942
17. Harding CV, Heuser JE, Stahl PD. Exosomes: looking back three decades and into the future. J Cell Biol. (2013) 200:367–71. doi: 10.1083/jcb.201212113
18. György B, Szabó TG, Pásztói M, Pál Z, Misják P, Aradi B, et al. Membrane vesicles, current state-of-the-art: emerging role of extracellular vesicles. Cell Mol Life Sci. (2011) 68:2667–88. doi: 10.1007/s00018-011-0689-3
19. Morello M, Minciacchi V, De Candia P, Yang J, Posadas E, Kim H, et al. Large oncosomes mediate intercellular transfer of functional microRNA. Cell Cycle. (2013) 12:3526–36. doi: 10.4161/cc.26539
20. Minciacchi VR, Freeman MR, Di Vizio D. Extracellular vesicles in cancer: exosomes, microvesicles and the emerging role of large oncosomes. In: Seminars in Cell and Developmental Biology. Elsevier (2015). p. 41–51.
21. D'Asti E, Garnier D, Lee TH, Montermini L, Meehan B, Rak J. Oncogenic extracellular vesicles in brain tumor progression. Front Physiol. (2012) 3:294. doi: 10.3389/fphys.2012.00294
22. Rilla K, Siiskonen H, Tammi M, Tammi R. “Hyaluronan-coated extracellular vesicles—a novel link between hyaluronan and cancer,” in Advances in cancer research. Elsevier. (2014) p. 121–48. doi: 10.1016/B978-0-12-800092-2.00005-8
23. Cocucci E, Meldolesi J. Ectosomes and exosomes: shedding the confusion between extracellular vesicles. Trends Cell Biol. (2015) 25:364–72. doi: 10.1016/j.tcb.2015.01.004
24. Théry C, Witwer KW, Aikawa E, Alcaraz MJ, Anderson JD, Andriantsitohaina R, et al. Minimal information for studies of extracellular vesicles 2018 (MISEV2018): a position statement of the International Society for Extracellular Vesicles and update of the MISEV2014 guidelines. J Extracell Vesicles. (2018) 7:1535750. doi: 10.1080/20013078.2018.1535750
25. Keller S, Rupp C, Stoeck A, Runz S, Fogel M, Lugert S, et al. CD24 is a marker of exosomes secreted into urine and amniotic fluid. Kidney Int. (2007) 72:1095–102. doi: 10.1038/sj.ki.5002486
26. Asea A, Jean-Pierre C, Kaur P, Rao P, Linhares IM, Skupski D, et al. Heat shock protein-containing exosomes in mid-trimester amniotic fluids. J Reprod Immunol. (2008) 79:12–7. doi: 10.1016/j.jri.2008.06.001
27. Andre F, Schartz NE, Movassagh M, Flament C, Pautier P, Morice P, et al. Malignant effusions and immunogenic tumour-derived exosomes. Lancet. (2002) 360:295–305. doi: 10.1016/S0140-6736(02)09552-1
28. Masyuk AI, Huang BQ, Ward CJ, Gradilone SA, Banales JM, Masyuk TV, et al. Biliary exosomes influence cholangiocyte regulatory mechanisms and proliferation through interaction with primary cilia. Am J Physiol Gastrointest Liver Physiol. (2010) 299:G990–9. doi: 10.1152/ajpgi.00093.2010
29. Caby M-P, Lankar D, Vincendeau-Scherrer C, Raposo G, Bonnerot C. Exosomal-like vesicles are present in human blood plasma. Int Immunol. (2005) 17:879–87. doi: 10.1093/intimm/dxh267
30. Admyre C, Johansson SM, Qazi KR, Filén J-J, Lahesmaa R, Norman M, et al. Exosomes with immune modulatory features are present in human breast milk. J Immunol. (2007) 179:1969–78. doi: 10.4049/jimmunol.179.3.1969
31. Balaj L, Lessard R, Dai L, Cho Y-J, Pomeroy SL, Breakefield XO, et al. Tumour microvesicles contain retrotransposon elements and amplified oncogene sequences. Nat Commun. (2011) 2:1–9. doi: 10.1038/ncomms1180
32. Vella L, Sharples R, Lawson V, Masters C, Cappai R, Hill A. Packaging of prions into exosomes is associated with a novel pathway of PrP processing. J Pathol. (2007) 211:582–90. doi: 10.1002/path.2145
33. Street JM, Barran PE, Mackay CL, Weidt S, Balmforth C, Walsh TS, et al. Identification and proteomic profiling of exosomes in human cerebrospinal fluid. J. Transl. Med. (2012) 10:5:2–7. doi: 10.1186/1479-5876-10-5
34. Orozco AF, Jorgez CJ, Ramos-Perez WD, Popek EJ, Yu X, Kozinetz CA, et al. Placental release of distinct DNA-associated micro-particles into maternal circulation: reflective of gestation time and preeclampsia. Placenta. (2009) 30:891–7. doi: 10.1016/j.placenta.2009.06.012
35. Houali K, Wang X, Shimizu Y, Djennaoui D, Nicholls J, Fiorini S, et al. A new diagnostic marker for secreted Epstein-Barr virus–encoded LMP1 and BARF1 oncoproteins in the serum and saliva of patients with nasopharyngeal carcinoma. Clin Cancer Res. (2007) 13:4993–5000. doi: 10.1158/1078-0432.CCR-06-2945
36. Ogawa Y, Miura Y, Harazono A, Kanai-Azuma M, Akimoto Y, Kawakami H, et al. Proteomic analysis of two types of exosomes in human whole saliva. Biol Pharm Bull. (2011) 34:13–23. doi: 10.1248/bpb.34.13
37. Poliakov A, Spilman M, Dokland T, Amling CL, Mobley JA. Structural heterogeneity and protein composition of exosome-like vesicles (prostasomes) in human semen. Prostate. (2009) 69:159–67. doi: 10.1002/pros.20860
38. Park K-H, Kim B-J, Kang J, Nam T-S, Lim JM, Kim HT, et al. Ca2+ signaling tools acquired from prostasomes are required for progesterone-induced sperm motility. Sci Signal. (2011) 4:ra31. doi: 10.1126/scisignal.2001595
39. Aalberts M, van Dissel-Emiliani FM, van Adrichem NP, van Wijnen M, Wauben MH, Stout TA, et al. Identification of distinct populations of prostasomes that differentially express prostate stem cell antigen, annexin A1, and GLIPR2 in humans. Biol Reprod. (2012) 86:81–8. doi: 10.1095/biolreprod.111.095760
40. Pisitkun T, Shen R-F, Knepper MA. Identification and proteomic profiling of exosomes in human urine. Proc Natl Acad Sci USA. (2004) 101:13368–73. doi: 10.1073/pnas.0403453101
41. Nilsson J, Skog J, Nordstrand A, Baranov V, Mincheva-Nilsson L, Breakefield X, et al. Prostate cancer-derived urine exosomes: a novel approach to biomarkers for prostate cancer. Bri J Cancer. (2009) 100:1603–7. doi: 10.1038/sj.bjc.6605058
42. Caballero J, Frenette G, Sullivan R. Post testicular sperm maturational changes in the bull: important role of the epididymosomes and prostasomes. Vet Med Int. (2011) 2011:757194. doi: 10.4061/2011/757194
43. Sullivan R. Epididymosomes: role of extracellular microvesicles in sperm maturation. Front. Biosci. (2016) 8:106–14. doi: 10.2741/s450
44. Al-Dossary AA, Strehler EE, Martin-DeLeon PA. Expression and secretion of plasma membrane Ca2+-ATPase 4a (PMCA4a) during murine estrus: association with oviductal exosomes and uptake in sperm. PLoS ONE. (2013) 8:e80181. doi: 10.1371/journal.pone.0080181
45. Bathala P, Fereshteh Z, Li K, Al-Dossary AA, Galileo DS, Martin-DeLeon PA. Oviductal extracellular vesicles (oviductosomes, OVS) are conserved in humans: murine OVS play a pivotal role in sperm capacitation and fertility. Mol Hum Reprod. (2018) 24:143–57. doi: 10.1093/molehr/gay003
46. da Silveira JC, Carnevale EM, Winger Q, Bouma GJ. Regulation of ACVR1 and ID2 by cell-secreted exosomes during follicle maturation in the mare. Reprod Biol Endocrinol. (2014) 12:1–9. doi: 10.1186/1477-7827-12-44
47. Ruiz-González I, Xu J, Wang X, Burghardt RC, Dunlap KA, Bazer FW. Exosomes, endogenous retroviruses and toll-like receptors: pregnancy recognition in ewes. Reproduction. (2015) 149:281–91. doi: 10.1530/REP-14-0538
48. Familari M, Cronqvist T, Masoumi Z, Hansson SR. Placenta-derived extracellular vesicles: their cargo and possible functions. Reprod Fertil Dev. (2017) 29:433–47. doi: 10.1071/RD15143
49. Varela M, Spencer TE, Palmarini M, Arnaud F. Friendly viruses: the special relationship between endogenous retroviruses and their host. Ann N Y Acad Sci. (2009) 1178:157–72. doi: 10.1111/j.1749-6632.2009.05002.x
50. Ng YH, Rome S, Jalabert A, Forterre A, Singh H, Hincks CL, et al. Endometrial exosomes/microvesicles in the uterine microenvironment: a new paradigm for embryo-endometrial cross talk at implantation. PLoS ONE. (2013) 8:e58502. doi: 10.1371/journal.pone.0058502
51. Salomon C, Nuzhat Z, Dixon CL, Menon R. Placental exosomes during gestation: liquid biopsies carrying signals for the regulation of human parturition. Curr Pharm Des. (2018) 24:974–82. doi: 10.2174/1381612824666180125164429
52. Simon C, Greening DW, Bolumar D, Balaguer N, Salamonsen LA, Vilella F. Extracellular vesicles in human reproduction in health and disease. Endocr. Rev. (2018) 39:292–332. doi: 10.1210/er.2017-00229
53. Jones RC. Evolution of the epididymis. In: Robaire HB, Kluwer B, editors. The Epididymis From Molecules to Clinical Practice. A Comprehensive Survey of the Efferent Ducts, the Epididymis and the vas Deferens. New York, NY: Academic/Plenum Publishers (2002).
54. Saez F, Frenette G, Sullivan R. Epididymosomes and prostasomes: their roles in posttesticular maturation of the sperm cells. J. Androl. (2003) 24:149–54. doi: 10.1002/j.1939-4640.2003.tb02653.x
55. Tannetta D, Dragovic R, Alyahyaei Z, Southcombe J. Extracellular vesicles and reproduction–promotion of successful pregnancy. Cell Mol Immunol. (2014) 11:548–63. doi: 10.1038/cmi.2014.42
56. Yanagimachi R. Mammalian fertilization. The physiology of reproduction. New York, NY: Raven Press. (1994).
57. Griffiths GS, Galileo DS, Reese K, Martin-DeLeon PA. Investigating the role of murine epididymosomes and uterosomes in GPI-linked protein transfer to sperm using SPAM1 as a model. Mol. Reprod. Dev. (2008) 75:1627–36. doi: 10.1002/mrd.20907
58. Al-Dossary AA, Bathala P, Caplan JL, Martin-DeLeon PA. Oviductosome-Sperm Membrane Interaction in cargo delivery detection of fusion and underlying molecular players using three-dimensional super-resolution structured illumination microscopy (SR-SIM). J Biol Chem. (2015) 290:17710–23. doi: 10.1074/jbc.M114.633156
59. Mokarizadeh A, Rezvanfar M-A, Dorostkar K, Abdollahi M. Mesenchymal stem cell derived microvesicles: trophic shuttles for enhancement of sperm quality parameters. Reprod Toxicol. (2013) 42:78–84. doi: 10.1016/j.reprotox.2013.07.024
60. Qamar AY, Fang X, Kim MJ, Cho J. Improved post-thaw quality of canine semen after treatment with exosomes from conditioned medium of adipose-derived mesenchymal stem cells. Animals. (2019) 9:865. doi: 10.3390/ani9110865
61. Burns G, Brooks K, Wildung M, Navakanitworakul R, Christenson LK, Spencer TE. Extracellular vesicles in luminal fluid of the ovine uterus. PLoS ONE. (2014) 9:e90913. doi: 10.1371/journal.pone.0090913
62. Zhang H, Martin-DeLeon PA. Mo use Spam1 (PH-20) is a multifunctional protein: evidence for its expression in the female reproductive tract. Biol Reprod. (2003) 69:446–54. doi: 10.1095/biolreprod.102.013854
63. Griffiths GS, Galileo DS, Aravindan RG, Martin-DeLeon PA. Clusterin facilitates exchange of glycosyl phosphatidylinositol-linked SPAM1 between reproductive luminal fluids and mouse and human sperm membranes. Biol Reprod. (2009) 81:562–70. doi: 10.1095/biolreprod.108.075739
64. Martin-DeLeon PA. Uterosomes: exosomal cargo during the estrus cycle and interaction with sperm. Front. Biosci. (2016) 8:115–22. doi: 10.2741/s451
65. da Silveira JC, Veeramachaneni DR, Winger Q, Carnevale EM, Bouma GJ. Cell-secreted vesicles in equine ovarian follicular fluid contain miRNAs and proteins: a possible new form of cell communication within the ovarian follicle. Biol. Reprod. (2012) 86:71–10. doi: 10.1095/biolreprod.111.093252
66. Sohel MMH, Hoelker M, Noferesti SS, Salilew-Wondim D, Tholen E, Looft C, et al. Exosomal and non-exosomal transport of extra-cellular microRNAs in follicular fluid: implications for bovine oocyte developmental competence. PLoS ONE. (2013) 8:e78505. doi: 10.1371/journal.pone.0078505
67. Navakanitworakul R, Hung W-T, Gunewardena S, Davis JS, Chotigeat W, Christenson LK. Characterization and small RNA content of extracellular vesicles in follicular fluid of developing bovine antral follicles. Sci Rep. (2016) 6:1–14. doi: 10.1038/srep25486
68. Barraud-Lange V, Chalas Boissonnas C, Serres C, Auer J, Schmitt A, Lefvre B, et al. Membrane transfer from oocyte to sperm occurs in two CD9-independent ways that do not supply the fertilising ability of Cd9-deleted oocytes. Reprod. (2012) 144:53–66. doi: 10.1530/REP-12-0040
69. Bianchi E, Wright GJ. Izumo meets Juno: preventing polyspermy in fertilization. Cell Cycle. (2014) 13:2019–20. doi: 10.4161/cc.29461
70. Abe H, Sendai Y, Satoh T, Hoshi H. Bovine oviduct-specific glycoprotein: a potent factor for maintenance of viability and motility of bovine spermatozoa in vitro. Mol Reprod Dev. (1995) 42:226–32. doi: 10.1002/mrd.1080420212
71. Avilés M, Coy P, Rizos D. The oviduct: a key organ for the success of early reproductive events. Anim Front. (2015) 5:25–31. doi: 10.2527/af.2015-0005
72. Kadam K, D'Souza S, Bandivdekar A, Natraj U. Identification and characterization of oviductal glycoprotein-binding protein partner on gametes: epitopic similarity to non-muscle myosin IIA, MYH 9. Mol Hum Reprod. (2006) 12:275–82. doi: 10.1093/molehr/gal028
73. Burns GW, Brooks KE, Spencer TE. Extracellular vesicles originate from the conceptus and uterus during early pregnancy in sheep. Biol Reprod. (2016) 94:51–11. doi: 10.1095/biolreprod.115.134973
74. Kusama K, Nakamura K, Bai R, Nagaoka K, Sakurai T, Imakawa K. Intrauterine exosomes are required for bovine conceptus implantation. Biochem Biophys Res Commun. (2018) 495:1370–5. doi: 10.1016/j.bbrc.2017.11.176
75. Sakurai T, Bai H, Bai R, Arai M, Iwazawa M, Zhang J, et al. Coculture system that mimics in vivo attachment processes in bovine trophoblast cells. Biol. Reprod. (2012) 87:61–11. doi: 10.1095/biolreprod.112.100180
76. Bai R, Kusama K, Sakurai T, Bai H, Wang C, Zhang J, et al. The role of endometrial selectins and their ligands on bovine conceptus attachment to the uterine epithelium during peri-implantation period. Biol. Reprod. (2015) 93:1–11. doi: 10.1095/biolreprod.115.128652
77. Abrahams VM, Straszewski-Chavez SL, Guller S, Mor G. First trimester trophoblast cells secrete Fas ligand which induces immune cell apoptosis. Mol Hum Reprod. (2004) 10:55–63. doi: 10.1093/molehr/gah006
78. Frängsmyr L, Baranov V, Nagaeva O, Stendahl U, Kjellberg L, Mincheva-Nilsson L. Cytoplasmic microvesicular form of Fas ligand in human early placenta: switching the tissue immune privilege hypothesis from cellular to vesicular level. Mol Hum Reprod. (2005) 11:35–41. doi: 10.1093/molehr/gah129
79. Hadley EE, Sheller-Miller S, Saade G, Salomon C, Mesiano S, Taylor RN, et al. Amnion epithelial cell–derived exosomes induce inflammatory changes in uterine cells. Am J Obstet Gynecol. (2018) 219:478.e1–21. doi: 10.1016/j.ajog.2018.08.021
80. Al-Dossary AA, Martin-DeLeon PA. Role of exosomes in the reproductive tract oviductosomes mediate interactions of oviductal secretion with gametes/early embryo. Front Biosci. (2016) 21:1278–1285. doi: 10.2741/4456
81. Alcântara-Neto A, Fernandez-Rufete M, Corbin E, Tsikis G, Uzbekov R, Garanina A, et al. Oviduct fluid extracellular vesicles regulate polyspermy during porcine in vitro fertilisation. Reprod Fertil Dev. (2020) 32:409–18. doi: 10.1071/RD19058
82. Saccary L, She Y-M, Oko R, Kan FW. Hamster oviductin regulates tyrosine phosphorylation of sperm proteins during in vitro capacitation. Biol. Reprod. (2013) 89:31–11. doi: 10.1095/biolreprod.113.109314
83. Zhao Y, Yang X, Jia Z, Reid RL, Leclerc P, Kan F. Recombinant human oviductin regulates protein tyrosine phosphorylation and acrosome reaction. Reproduction. (2016) 152:561–73. doi: 10.1530/REP-16-0177
84. King RS, Anderson SH, Killian GJ. Effect of bovine oviductal estrus-associated protein on the ability of sperm to capacitate and fertilize oocytes. J Androl. (1994) 15:468–78.
85. Carmen Alminana-Brines C. Snooping on a private conversation between the oviduct and gametes/embryos. Animal Reprod. (2015) 12 :366–74. Available online at: https://hal.archives-ouvertes.fr/hal-01413579
86. Asquith KL, Baleato RM, McLaughlin EA, Nixon B, Aitken RJ. Tyrosine phosphorylation activates surface chaperones facilitating sperm-zona recognition. J Cell Sci. (2004) 117:3645–57. doi: 10.1242/jcs.01214
87. Schuh K, Cartwright EJ, Jankevics E, Bundschu K, Liebermann J, Williams JC, et al. Plasma membrane Ca2+ ATPase 4 is required for sperm motility and male fertility. J Biol Chem. (2004) 279:28220–6. doi: 10.1074/jbc.M312599200
88. Almiñana C, Corbin E, Tsikis G, Alcântara-Neto AS, Labas V, Reynaud K, et al. Oviduct extracellular vesicles protein content and their role during oviduct–embryo cross-talk. Reprod. (2017) 154:253–68. doi: 10.1530/REP-17-0054
89. Di Pietro C. Exosome-mediated communication in the ovarian follicle. J Assist Reprod Genet. (2016) 33:303–11. doi: 10.1007/s10815-016-0657-9
90. Fair T. Follicular oocyte growth and acquisition of developmental competence. Anim Reprod Sci. (2003) 78:203–16. doi: 10.1016/S0378-4320(03)00091-5
91. Eppig JJ. Oocyte control of ovarian follicular development and function in mammals. Reprod. (2001) 122:829–38. doi: 10.1530/rep.0.1220829
92. Eppig JJ, Wigglesworth K, Pendola FL. The mammalian oocyte orchestrates the rate of ovarian follicular development. Proc Natl Acad Sci, USA. (2002) 99:2890–4. doi: 10.1073/pnas.052658699
93. Armstrong DG, Webb R. Ovarian follicular dominance: the role of intraovarian growth factors and novel proteins. Rev Reprod. (1997) 2:139–46. doi: 10.1530/ror.0.0020139
94. Harwood BN, Cross SK, Radford EE, Haac BE, De Vries WN. Members of the WNT signaling pathways are widely expressed in mouse ovaries, oocytes, and cleavage stage embryos. Dev Dyn. (2008) 237:1099–111. doi: 10.1002/dvdy.21491
95. Luo S, Kleemann GA, Ashraf JM, Shaw WM, Murphy CT. TGF-β and insulin signaling regulate reproductive aging via oocyte and germline quality maintenance. Cell. (2010) 143:299–312. doi: 10.1016/j.cell.2010.09.013
96. Su Y-Q, Wu X, O'Brien MJ, Pendola FL, Denegre JN, Matzuk MM, et al. Synergistic roles of BMP15 and GDF9 in the development and function of the oocyte–cumulus cell complex in mice: genetic evidence for an oocyte–granulosa cell regulatory loop. Dev Biol. (2004) 276:64–73. doi: 10.1016/j.ydbio.2004.08.020
97. Patsoula E, Loutradis D, Drakakis P, Kallianidis K, Bletsa R, Michalas S. Expression of mRNA for the LH and FSH receptors in mouse oocytes and preimplantation embryos. Reproduction-Cambridge-. (2001) 121:455–61. doi: 10.1530/rep.0.1210455
98. Benammar A, Ziyyat A, Lefèvre B, Wolf J-P. Tetraspanins and mouse oocyte microvilli related to fertilizing ability. Reprod Sci. (2017) 24:1062–9. doi: 10.1177/1933719116678688
99. Sang Q, Yao Z, Wang H, Feng R, Wang H, Zhao X, et al. Identification of microRNAs in human follicular fluid: characterization of microRNAs that govern steroidogenesis in vitro and are associated with polycystic ovary syndrome in vivo. J Clin Endocrinol Metab. (2013) 98:3068–79. doi: 10.1210/jc.2013-1715
100. Dalanezi F, Ferreira J. Gene expression of in vitro maturated oocytes can be modulated by follicle exosomes from cows kept under thermo-neutral or heat- stress condition. Vet Zootech. (2016) 23:54. doi: 10.1071/RDv29n1Ab183
101. Hung W-T, Hong X, Christenson LK, McGinnis LK. Extracellular vesicles from bovine follicular fluid support cumulus expansion. Biol Reprod. (2015) 93:111–9. doi: 10.1095/biolreprod.115.132977
102. Hung W-T, Navakanitworakul R, Khan T, Zhang P, Davis JS, McGinnis LK, et al. Stage-specific follicular extracellular vesicle uptake and regulation of bovine granulosa cell proliferation. Biol Reprod. (2017) 97:644–55. doi: 10.1093/biolre/iox106
103. da Silveira JC, Andrade GM, del Collado M, Sampaio RV, Sangalli JR, Silva LA, et al. Supplementation with small-extracellular vesicles from ovarian follicular fluid during in vitro production modulates bovine embryo development. PLoS ONE. (2017) 12:e0179451. doi: 10.1371/journal.pone.0179451
104. Rodrigues TA, Tuna KM, Alli AA, Tribulo P, Hansen P, Koh J, et al. Follicular fluid exosomes act on the bovine oocyte to improve oocyte competence to support development and survival to heat shock. Reprod Fertil Dev. (2019) 31:888–97. doi: 10.1071/RD18450
105. Macaulay AD, Gilbert I, Caballero J, Barreto R, Fournier E, Tossou P, et al. The gametic synapse: RNA transfer to the bovine oocyte. Biol. Reprod. (2014) 91:91–12. doi: 10.1095/biolreprod.114.119867
106. Zolti M, Ben-Rafael Z, Meirom R, Shemesh M, Bider D, Mashiach S, et al. Cytokine involvement in oocytes and early embryos. Fertil Steril. (1991) 56:265–72. doi: 10.1016/S0015-0282(16)54483-5
107. Field SL, Dasgupta T, Cummings M, Orsi NM. Cytokines in ovarian folliculogenesis, oocyte maturation and luteinisation. Mol Reprod Dev. (2014) 81:284–314. doi: 10.1002/mrd.22285
108. Guay C, Regazzi R. Exosomes as new players in metabolic organ cross-talk. Diabetes Obes Metab. (2017) 19:137–46. doi: 10.1111/dom.13027
109. Eldh M, Ekström K, Valadi H, Sjöstrand M, Olsson B, Jernås M, et al. Exosomes communicate protective messages during oxidative stress; possible role of exosomal shuttle RNA. PLoS ONE. (2010) 5:e15353. doi: 10.1371/journal.pone.0015353
110. Carver KA, Yang D. N-acetylcysteine amide protects against oxidative stress–induced microparticle release from human retinal pigment epithelial cells. Investig Ophthalmol Vis Sci. (2016) 57:360–71. doi: 10.1167/iovs.15-17117
111. Saeed-Zidane M, Linden L, Salilew-Wondim D, Held E, Neuhoff C, Tholen E, et al. Cellular and exosome mediated molecular defense mechanism in bovine granulosa cells exposed to oxidative stress. PLoS ONE. (2017) 12:e0187569. doi: 10.1371/journal.pone.0187569
112. Lange-Consiglio A, Perrini C, Albini G, Modina S, Lodde V, Orsini E, et al. Oviductal microvesicles and their effect on in vitro maturation of canine oocytes. Reproduction. (2017) 154:167–80. doi: 10.1530/REP-17-0117
113. Lopera-Vásquez R, Hamdi M, Fernandez-Fuertes B, Maillo V, Beltrán-Breña P, Calle A, et al. Extracellular vesicles from BOEC in vitro embryo development and quality. PLoS ONE. (2016) 11:e0148083. doi: 10.1371/journal.pone.0148083
114. Lopera-Vasquez R, Hamdi M, Maillo V, Gutierrez-Adan A, Bermejo-Alvarez P, Ramírez MÁ, et al. Effect of bovine oviductal extracellular vesicles on embryo development and quality in vitro. Reproduction. (2017) 153:461–70. doi: 10.1530/REP-16-0384
115. Qu P, Zhao Y, Wang R, Zhang Y, Li L, Fan J, et al. Extracellular vesicles derived from donor oviduct fluid improved birth rates after embryo transfer in mice. Reprod Fertil Dev. (2019) 31:324–32. doi: 10.1071/RD18203
116. Qiao F, Ge H, Ma X, Zhang Y, Zuo Z, Wang M, et al. Bovine uterus-derived exosomes improve developmental competence of somatic cell nuclear transfer embryos. Theriogenology. (2018) 114:199–205. doi: 10.1016/j.theriogenology.2018.03.027
117. Giacomini E, Vago R, Sanchez AM, Podini P, Zarovni N, Murdica V, et al. Secretome of in vitro cultured human embryos contains extracellular vesicles that are uptaken by the maternal side. Sci Rep. (2017) 7:1–13. doi: 10.1038/s41598-017-05549-w
118. Bemis L, McCue P, Hatzel J, Bemis J, Ferris R. Evidence for production of early pregnancy factor (Hsp10), microRNAs and exosomes by day 8 equine embryos. J Equine Vet Sci. (2012) 7:398. doi: 10.1016/j.jevs.2012.05.010
119. Saadeldin IM, Kim SJ, Choi YB, Lee BC. Improvement of cloned embryos development by co-culturing with parthenotes: a possible role of exosomes/microvesicles for embryos paracrine communication. Cell Reprogram. (2014) 16:223–34. doi: 10.1089/cell.2014.0003
120. Qu P, Qing S, Liu R, Qin H, Wang W, Qiao F, et al. Effects of embryo-derived exosomes on the development of bovine cloned embryos. PLoS ONE. (2017) 12:e0174535. doi: 10.1371/journal.pone.0174535
121. Sheller S, Papaconstantinou J, Urrabaz-Garza R, Richardson L, Saade G, Salomon C, et al. Amnion-epithelial-cell-derived exosomes demonstrate physiologic state of cell under oxidative stress. PLoS ONE. (2016) 11:e0157614. doi: 10.1371/journal.pone.0157614
122. Martín-Blanco EJB. p38 MAPK signalling cascades: ancient roles and new functions. Bioeassys. (2000) 22:637–45. doi: 10.1002/1521-1878(200007)22:7<637::AID-BIES6>3.0.CO;2-E
123. Desrochers LM, Bordeleau F, Reinhart-King CA, Cerione RA, Ma A. Microvesicles provide a mechanism for intercellular communication by embryonic stem cells during embryo implantation. Nat Commun. (2016) 11958:1–11. doi: 10.1038/ncomms11958
124. Holst P, Phemister R. The prenatal development of the dog: preimplantation events. Biol Reprod. (1971) 5:194–206. doi: 10.1093/biolreprod/5.2.194
125. Jin M, Fujiwara E, Kakiuchi Y, Okabe M, Satouh Y, Baba SA, et al. Most fertilizing mouse spermatozoa begin their acrosome reaction before contact with the zona pellucida during in vitro fertilization. Proc Natl AcadSci USA. (2011) 108:4892–6. doi: 10.1073/pnas.1018202108
126. Chang MC. Fertilizing capacity of spermatozoa deposited into the fallopian tubes. Nature. (1951) 168:697–8. doi: 10.1038/168697b0
127. Austin C. The ‘capacitation’of the mammalian sperm. Nature. (1952) 170:326. doi: 10.1038/170326a0
128. Kirchoff C, Pera I, Derr P, Yeung C, Cooper T. The molecular biology of the sperm surface. Post testicular membrane remodeling. Adv Exp Med Biol. (1997) 424:221–32. doi: 10.1007/978-1-4615-5913-9_40
129. Aitken RJ, Nixon B. Sperm capacitation: a distant landscape glimpsed but unexplored. Mol Hum Reprod. (2013) 19:785–93. doi: 10.1093/molehr/gat067
130. Ohnami N, Nakamura A, Miyado M, Sato M, Kawano N, Yoshida K, et al. CD81 and CD9 work independently as extracellular components upon fusion of sperm and oocyte. Biol Open. (2012) 1:640–7. doi: 10.1242/bio.20121420
131. Miyado K, Yoshida K, Yamagata K, Sakakibara K, Okabe M, Wang X, et al. The fusing ability of sperm is bestowed by CD9-containing vesicles released from eggs in mice. Proc Natl Acad Sci USA. (2008) 105:12921–6. doi: 10.1073/pnas.0710608105
132. Runge KE, Evans JE, He Z-Y, Gupta S, McDonald KL, Stahlberg H, et al. Oocyte CD9 is enriched on the microvillar membrane and required for normal microvillar shape and distribution. Dev Biol. (2007) 304:317–25. doi: 10.1016/j.ydbio.2006.12.041
133. Tanigawa M, Miyamoto K, Kobayashi S, Sato M, Akutsu H, Okabe M, et al. Possible involvement of CD81 in acrosome reaction of sperm in mice. Mol Reprod Dev. (2008) 75:150–5. doi: 10.1002/mrd.20709
134. Inoue N, Ikawa M, Isotani A, Okabe M. The immunoglobulin superfamily protein Izumo is required for sperm to fuse with eggs. Nature. (2005) 434:234–8. doi: 10.1038/nature03362
135. Sharifulin D, Babaylova E, Kossinova O, Bartuli Y, Graifer D, Karpova G. Ribosomal protein S5e is implicated in translation initiation through its interaction with the N-terminal domain of initiation factor eIF2α. Chembiochem. (2013). 14:2136–43. doi: 10.1002/cbic.201300318
136. Shih Y-T, Hsueh Y-P. VCP and ATL1 regulate endoplasmic reticulum and protein synthesis for dendritic spine formation. Nat Commun. (2016) 7:1–16. doi: 10.1038/ncomms11020
137. Coy P, Cánovas S, Mondéjar I, Saavedra MD, Romar R, Grullón L, et al. Oviduct-specific glycoprotein and heparin modulate sperm–zona pellucida interaction during fertilization and contribute to the control of polyspermy. Proc Natl Acad Sci, USA. (2008) 105:15809–14. doi: 10.1073/pnas.0804422105
138. Batista RITP, Moro LN, Corbin E, Alminana C, Souza-Fabjan JMG, de Figueirêdo Freitas VJ, et al. Combination of oviduct fluid and heparin to improve monospermic zygotes production during porcine in vitro fertilization. Theriogenology. (2016) 86:495–502. doi: 10.1016/j.theriogenology.2016.01.031
139. Bureau M, Bailey JL, Sirard M-A. Influence of oviductal cells and conditioned medium on porcine gametes. Zygote. (2000) 8:139–44. doi: 10.1017/S0967199400000915
140. Gonçalves R, Staros A, Killian G. Oviductal fluid proteins associated with the bovine zona pellucida and the effect on in vitro sperm–egg binding, fertilization and embryo development. Reprod Domest Anim. (2008) 43:720–9. doi: 10.1111/j.1439-0531.2007.00978.x
141. Mahsoudi B, Li A, O'Neill C. Assessment of the long-term and transgenerational consequences of perturbing preimplantation embryo development in mice. Biol Reprod. (2007) 77:889–96. doi: 10.1095/biolreprod.106.057885
142. Katz-Jaffe MG, Schoolcraft WB, Gardner DK. Analysis of protein expression (secretome) by human and mouse preimplantation embryos. Fertil Steril. (2006) 86:678–85. doi: 10.1016/j.fertnstert.2006.05.022
143. Wolf E, Arnold G, Bauersachs S, Beier H, Blum H, Einspanier R, et al. Embryo-maternal communication in bovine–strategies for deciphering a complex cross-talk. Reprod Domest Anim. (2003) 38:276–89. doi: 10.1046/j.1439-0531.2003.00435.x
144. Betteridge KJ, Fléchon JE. The anatomy and physiology of pre-attachment bovine embryos. Theriogenology. (1988) 29:155–87. doi: 10.1016/0093-691X(88)90038-6
145. Bazer FW, Wu G, Johnson GA, Kim J, Song G. Uterine histotroph and conceptus development: select nutrients and secreted phosphoprotein 1 affect mechanistic target of rapamycin cell signaling in ewes. Biol Reprod. (2011) 85:1094–107. doi: 10.1095/biolreprod.111.094722
146. Forde N, Lonergan P. Transcriptomic analysis of the bovine endometrium: what is required to establish uterine receptivity to implantation in cattle? J Reprod Dev. (2012) 58:189–95. doi: 10.1262/jrd.2011-021
147. Zhang Y, Wang Q, Wang H, Duan E. Uterine fluid in pregnancy: a biological and clinical outlook. Trends Mol Med. (2017) 23:604–14. doi: 10.1016/j.molmed.2017.05.002
148. Roberts R, Ealy A, Alexenko A, Han C-S, Ezashi T. Trophoblast interferons. Placenta. (1999) 20:259–64. doi: 10.1053/plac.1998.0381
149. Spencer TE, Bazer FW. Biology of progesterone action during pregnancy recognition and maintenance of pregnancy. Front Biosci. (2002) 7:d1879–98. doi: 10.2741/spencer
150. Burns GW, Brooks KE, O'Neil EV, Hagen DE, Behura SK, Spencer TE. Progesterone effects on extracellular vesicles in the sheep uterus. Biol Reprod. (2018) 98:612–22. doi: 10.1093/biolre/ioy011
151. Ferry L, Mermillod P, Massip A, Dessy F. Bovine embryos cultured in serum-poor oviduct-conditioned medium need cooperation to reach the blastocyst stage. Theriogenology. (1994) 42:445–53. doi: 10.1016/0093-691X(94)90682-9
152. Hoelker M, Rings F, Lund Q, Ghanem N, Phatsara C, Griese J, et al. Effect of the microenvironment and embryo density on developmental characteristics and gene expression profile of bovine preimplantative embryos cultured in vitro. Reproduction. (2009) 137:415–25. doi: 10.1530/REP-08-0370
153. Schultz G, Heyner S. Growth factors in preimplantation mammalian embryos. Oxf Rev Reprod Biol. (1993) 15:43–81.
154. Bormann C, Swain J, Ni Q, Kennedy R, Smith G. Preimplantation embryo secretome identification. Fertil Steril. (2006) 86:S116. doi: 10.1016/j.fertnstert.2006.07.310
155. Hoelker M, Rings F, Lund Q, Phatsara C, Schellander K, Tesfaye D. Effect of embryo density on in vitro developmental characteristics of bovine preimplantative embryos with respect to micro and macroenvironments. Reprod Domest Anim. (2010) 45:e138–45. doi: 10.1111/j.1439-0531.2009.01535.x
156. Mellisho EA, Velásquez AE, Nuñez MJ, Cabezas JG, Cueto JA, Fader C, et al. Identification and characteristics of extracellular vesicles from bovine blastocysts produced in vitro. PLoS ONE. (2017) 12:e0178306. doi: 10.1371/journal.pone.0178306
157. Gardiner C, Ferriera J, Poli M, Turner K, Child T, Sargent I. IVF embryos release extracellular vesicles which may act as an indicator of embryo quality. J Extracell Vesicles. (2013) 2:20826. doi: 10.3402/jev.v2i0.20826
158. Nakamura K, Kusama K, Bai R, Sakurai T, Isuzugawa K, Godkin JD, et al. Induction of IFNT-stimulated genes by conceptus-derived exosomes during the attachment period. PLoS ONE. (2016) 11:e0158278. doi: 10.1371/journal.pone.0158278
159. Redman C, Sargent I. Circulating microparticles in normal pregnancy and pre-eclampsia. Placenta. (2008) 29:73–7. doi: 10.1016/j.placenta.2007.11.016
160. Bidarimath M, Khalaj K, Kridli RT, Kan FW, Koti M, Tayade C. Extracellular vesicle mediated intercellular communication at the porcine maternal-fetal interface: a new paradigm for conceptus-endometrial cross-talk. Sci Rep. (2017) 7:1–14. doi: 10.1038/srep40476
161. Atay S, Gercel-Taylor C, Suttles J, Mor G, Taylor DD. Trophoblast-derived exosomes mediate monocyte recruitment and differentiation. Am J Reprod Immunol. (2011) 65:65–77. doi: 10.1111/j.1600-0897.2010.00880.x
162. Tolosa J, Schjenken J, Clifton V, Vargas A, Barbeau B, Lowry P, et al. The endogenous retroviral envelope protein syncytin-1 inhibits LPS/PHA-stimulated cytokine responses in human blood and is sorted into placental exosomes. Placenta. (2012) 33:933–41. doi: 10.1016/j.placenta.2012.08.004
163. Matsuura K, Jigami T, Taniue K, Morishita Y, Adachi S, Senda T, et al. Identification of a link between Wnt/β-catenin signalling and the cell fusion pathway. Nat Commun. (2011) 2:1–9. doi: 10.1038/ncomms1551
164. Jeschke U, Hutter S, Heublein S, Vrekoussis T, Andergassen U, Unverdorben L, et al. Expression and function of galectins in the endometrium and at the human feto-maternal interface. Placenta. (2013) 34:863–72. doi: 10.1016/j.placenta.2013.07.005
165. Murthi P, Kalionis B, Cocquebert M, Rajaraman G, Chui A, Keogh RJ, et al. Homeobox genes and down-stream transcription factor PPARγ in normal and pathological human placental development. Placenta. (2013) 34:299–309. doi: 10.1016/j.placenta.2013.01.005
166. Mincheva-Nilsson L, Baranov V. The role of placental exosomes in reproduction. Am J Reprod Immunol. (2010) 63:520–33. doi: 10.1111/j.1600-0897.2010.00822.x
167. Taylor DD, Akyol S, Gercel-Taylor C. Pregnancy-associated exosomes and their modulation of T cell signaling. J Immunol. (2006) 176:1534–42. doi: 10.4049/jimmunol.176.3.1534
168. Jung N-H, Kim S-H, Kim D-S, Yoon J-T. Study on the in-vitro culture method for normal embryonic cell development of porcine parthenogenetic embryos. J Anim Reprod Biotechnol. (2020) 35:94–101. doi: 10.12750/JARB.35.1.94
169. Salomon C, Torres MJ, Kobayashi M, Scholz-Romero K, Sobrevia L, Dobierzewska A, et al. A gestational profile of placental exosomes in maternal plasma and their effects on endothelial cell migration. PLoS ONE. (2014) 9:e98667. doi: 10.1371/journal.pone.0098667
170. Mitchell MD, Peiris HN, Kobayashi M, Koh YQ, Duncombe G, Illanes SE, et al. Placental exosomes in normal and complicated pregnancy. Am J Obstet Gynecol. (2015) 213:S173–181. doi: 10.1016/j.ajog.2015.07.001
171. Dixon CL, Sheller-Miller S, Saade GR, Fortunato SJ, Lai A, Palma C, et al. Amniotic fluid exosome proteomic profile exhibits unique pathways of term and preterm labor. Endocrinology. (2018) 159:2229–40. doi: 10.1210/en.2018-00073
172. Smith R. Alterations in the hypothalamic pituitary adrenal axis during pregnancy and the placental clock that determines the length of parturition. J Reprod Immunol. (1998) 39:215–20. doi: 10.1016/S0165-0378(98)00023-0
173. Schwartz J, McMillen IC. Fetal hypothalamus-pituitary-adrenal axis on the road to parturition. Clin Exp Pharmacol Physiol. (2001) 28:108–12. doi: 10.1046/j.1440-1681.2001.03412.x
174. Zakar T, Hertelendy F. Progesterone withdrawal: key to parturition. Am J Obstet Gynecol. (2007) 196:289–96. doi: 10.1016/j.ajog.2006.09.005
175. Csapo AI, Pinto-Dantas CA. The effect of progesterone on the human uterus. Proc Natl Acad Sci USA. (1965) 54:1069. doi: 10.1073/pnas.54.4.1069
176. Weiss G. Endocrinology of parturition. J Clin Endocrinol Metab. (2000) 85:4421–5. doi: 10.1210/jc.85.12.4421
177. Kota SK, Gayatri K, Jammula S, Kota SK, Krishna S, Meher LK, et al. Endocrinology of parturition. Indian J Endocrinol Metab. (2013) 17:50–9. doi: 10.4103/2230-8210.107841
178. Sheller-Miller S, Trivedi J, Yellon SM, Menon R. Exosomes cause preterm birth in mice: evidence for paracrine signaling in pregnancy. Sci Rep. (2019) 9:1–18. doi: 10.1038/s41598-018-37002-x
179. Osman I, Young A, Ledingham MA, Thomson AJ, Jordan F, Greer IA, et al. Leukocyte density and pro-inflammatory cytokine expression in human fetal membranes, decidua, cervix and myometrium before and during labour at term. Mol Hum Reprod. (2003) 9:41–5. doi: 10.1093/molehr/gag001
180. Christiaens I, Zaragoza DB, Guilbert L, Robertson SA, Mitchell BF, Olson DM. Inflammatory processes in preterm and term parturition. J Reprod Immunol. (2008) 79:50–7. doi: 10.1016/j.jri.2008.04.002
Keywords: intracellular communications, biofluids, functional molecules, embryo, placenta
Citation: Qamar AY, Mahiddine FY, Bang S, Fang X, Shin ST, Kim MJ and Cho J (2020) Extracellular Vesicle Mediated Crosstalk Between the Gametes, Conceptus, and Female Reproductive Tract. Front. Vet. Sci. 7:589117. doi: 10.3389/fvets.2020.589117
Received: 30 July 2020; Accepted: 28 September 2020;
Published: 30 October 2020.
Edited by:
Islam M. Saadeldin, King Saud University, Saudi ArabiaReviewed by:
Ayman Abdel-Aziz Swelum, Zagazig University, EgyptLleretny Rodriguez-Alvarez, University of Concepcion, Chile
Copyright © 2020 Qamar, Mahiddine, Bang, Fang, Shin, Kim and Cho. This is an open-access article distributed under the terms of the Creative Commons Attribution License (CC BY). The use, distribution or reproduction in other forums is permitted, provided the original author(s) and the copyright owner(s) are credited and that the original publication in this journal is cited, in accordance with accepted academic practice. No use, distribution or reproduction is permitted which does not comply with these terms.
*Correspondence: Jongki Cho, cjki@cnu.ac.kr; Min Jung Kim, tinia19@snu.ac.kr