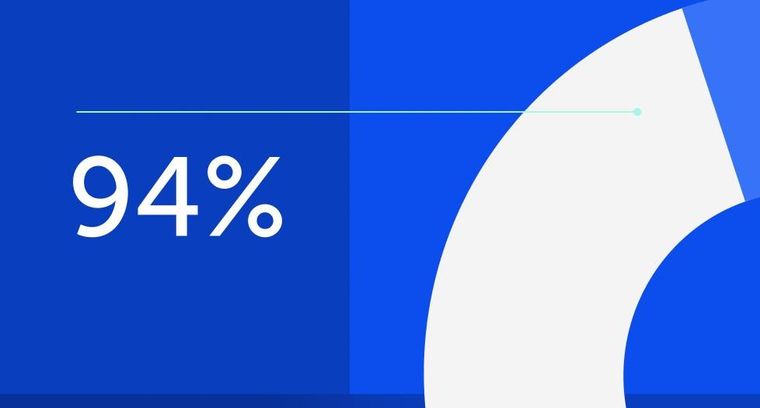
94% of researchers rate our articles as excellent or good
Learn more about the work of our research integrity team to safeguard the quality of each article we publish.
Find out more
BRIEF RESEARCH REPORT article
Front. Vet. Sci., 06 November 2020
Sec. Veterinary Epidemiology and Economics
Volume 7 - 2020 | https://doi.org/10.3389/fvets.2020.583930
This article is part of the Research TopicAntimicrobial Resistance in Zoonotic Bacteria in Developing Countries: The Role of Food Animal Production in Public HealthView all 11 articles
Shiga toxin-producing Escherichia coli (STEC) and Listeria monocytogenes are worldwide recognized zoonotic pathogens. Recent reports have emerged about the circulation of antimicrobial-resistant STEC and L. monocytogenes isolates. To assess the frequency of antimicrobial resistance and related genes in these pathogens, we studied 45 STEC and 50 L. monocytogenes isolates locally recovered from different sources. Antimicrobial susceptibility testing was performed by disk-diffusion method, and the genomic sequences of three selected STEC and from all 50 L. monocytogenes isolates were analyzed for antibiotic resistance genes. Four STEC and three L. monocytogenes isolates were phenotypically resistant to at least one of the antibiotics tested. Resistance genes aph(3″)-Ib, aph(3′)-Ia, aph(6)-Id, blaTEM−1B, sul2, mef (A), and tet(A) were found in a human STEC ampicillin-resistant isolate. All L. monocytogenes isolates harbored fosX, lin, mdrL, lde fepA, and norB. Overall resistance in L. monocytogenes and STEC was low or middle. However, the high load of resistance genes found, even in susceptible isolates, suggests that these pathogens could contribute to the burden of antimicrobial resistance.
Shiga toxin-producing Escherichia coli (STEC) and Listeria monocytogenes are well-recognized zoonotic pathogens circulating in Uruguay (1, 2). In humans, STEC can produce watery or bloody diarrhea (WD, BD) or even more severe conditions such as hemorrhagic colitis (HC) or hemolytic–uremic syndrome (HUS). HUS can be lethal in the early stages or leave long-term sequelae; ~20% of children who suffer it require chronic dialysis or kidney transplant. STEC has also been rarely associated with urinary tract infections (3, 4). Cattle and other food production animals are the main known reservoir for STEC, and the transmission to humans occurs by direct contact with them or through the ingestion of foods or water contaminated with its feces (5). Although controversial, antibiotics such as gentamicin, azithromycin, fosfomycin, and meropenem are recommended to the treatment of human STEC infections to avoid the development of most severe diseases (6).
Listeria monocytogenes is the etiologic agent of invasive listeriosis, a severe food-borne disease that mainly affects elderly, immunocompromised people, pregnant women, and infants. L. monocytogenes is widely distributed in nature, including the bowel of cattle, so it has multiple opportunities to enter the food production and supply chain. Although human invasive listeriosis is rare, it has high rates of hospitalization and case fatality (7, 8).
Listeria monocytogenes is susceptible to most clinically relevant groups of antibiotics active against Gram-positive bacteria, except for intrinsic resistance to fosfomycin, older quinolones, sulfamethoxazole, oxacillin, and expanded-spectrum cephalosporins (8, 9). The first-line therapy for listeriosis is ampicillin or penicillin G, with or without the addition of gentamicin. For beta-lactam-allergic patients, the therapy of choice is trimethoprim-sulfamethoxazole or vancomycin (8–10).
Likewise, antibiotics are used in veterinary medicine for the treatment and prevention of infectious diseases, but also, they have been used for a long time for animal growth promotion and improved productivity. These situations contribute to the selection of resistant bacteria, including STEC and L. monocytogenes, which could then be transmitted to humans, also facilitating the spread of antibiotic resistance genes (6, 11).
Reports have emerged about the circulation of antimicrobial-resistant L. monocytogenes isolates worldwide (11). Similarly, antimicrobial-resistant STEC isolates were reported in Brazil and Mexico among other countries (6), highlighting the role as reservoir of resistance genes and recommending the surveillance of its susceptibility profiles.
The aim of this study was to assess the frequency of antibiotic resistance against different drugs used in human and veterinary medicine in a set of STEC and L. monocytogenes isolates and to analyze the presence of possible related genes.
We studied a collection of 45 STEC and 50 L. monocytogenes isolates. All of them were received at the Bacteriology and Virology Department (University of the Republic, School of Medicine) between 2010 and 2019 to confirm the identification and to determine pathotype and serotype. All STEC received until the end of 2017 were included in this study. STEC isolates were from different sources: human samples (n = 7), six isolates from feces of children ≤5 years old and one belonging to the serogroup O157 from urine of an adult woman; food samples (n = 37), all recovered from beef (Bos taurus); and animal sample (n = 1) isolated from feces of a healthy cow (see Supplementary Table 1).
Listeria monocytogenes isolates were selected as a convenience sample from a total of 498 isolates received, including different serotypes, sources, and year of isolation (see Supplementary Table 2). Human isolates (n = 29) were obtained from blood, placenta, amniotic fluid, and cerebrospinal fluid samples. The food isolates (n = 21) were recovered from frozen food, ready-to-eat food, deli meat, and cheese.
STEC isolates were serotyped and analyzed by PCR for the presence of stx1/2, eae, and ehxA virulence genes (1).
Listeria monocytogenes strains were serotyped using a combination of multiplex PCR and agglutination tests with commercially available Listeria antisera to one and four somatic antigens as we previously described (3).
All STEC isolates were studied by disk-diffusion method according to the guidelines for Enterobacteriaceae of Clinical and Laboratory Standards Institute (CLSI) (12). We used Mueller–Hinton agar plates, and the antimicrobials tested were ampicillin (AMP), amoxicillin-clavulanic acid (AMC), cefuroxime (CXM), fosfomycin-trometamol (FOT), cefepime (FEP), cefotaxime (CTX), ceftazidime (CAZ), cefoxitin (FOX), ceftriaxone (CRO), ciprofloxacin (CIP), gentamicin (CN), imipenem (IPM), meropenem (MEM), and trimethoprim-sulfamethoxazole (SXT) (Oxoid®). Plates were incubated at 35 ± 2°C in ambient air during 16–18 h, and the result interpretation was done according to Clinical and Laboratory Standards Institute (CLSI) breakpoints (Table 2A, Enterobacteriaceae M02 and M07) (12). E. coli ATCC 25922 was used as quality control.
Listeria monocytogenes antimicrobial susceptibility testing was performed by disk-diffusion method according to the recommendations for L. monocytogenes of the European Committee on Antimicrobial Susceptibility Testing (EUCAST) (13). Mueller–Hinton agar plates supplemented with 5% of mechanically defibrinated horse blood and 20 mg/L β-NAD (MH-F) were prepared “in-house.” A panel of six antibiotics was tested: benzylpenicillin (1 μg), gentamicin (10 μg), trimethoprim-sulfamethoxazole (1.25 μg/23.75 μg), meropenem (10 μg), erythromycin (15 μg), and ciprofloxacin (5 μg) with Oxoid® disks. Cultures were incubated at 35°C with 5% CO2 for 18–20 h.
Streptococcus pneumoniae ATCC 49619 and Staphylococcus aureus ATCC 25923 were used as quality control strains. L. monocytogenes-specific clinical breakpoints of EUCAST were used for penicillin, meropenem, erythromycin, and trimethoprim-sulfamethoxazole; for gentamicin and ciprofloxacin, interpretation was done according to the clinical breakpoint value for Staphylococcus spp.
The genomic DNA from three selected human STEC isolates (corresponding to different serogroups, two fully susceptible, and one resistant only to ampicillin) and from all L. monocytogenes isolates was extracted with the DNA blood and tissue kit (Qiagen®) and subjected to whole-genome sequencing by Illumina MiSeq platform with Nextera XT library prep kits (USA) and TruSeq Nano library kit. The reads were de novo assembled with SPAdes version 3.13.1 (14). Genomic sequences of STEC and L. monocytogenes were analyzed for resistance genes using the software ABRicate with the databases ResFinder, CARD, NCBI AMRFinderPlus, and MEGARes (update April 19, 2020). For L. monocytogenes, we also searched for the antimicrobial resistance genes fepA, lde, and penA using BLAST tool because these genes have been reported in these bacteria but were not included in the databases mentioned above.
STEC serogroup distribution was as follows: O157 (36 isolates), O26 (3), O145 (2), O45 (1), O103 (1), O111 (1), and O153 (1). Serogroup distribution according to the source is shown in Figure 1.
Figure 1. Distribution of analyzed Shiga toxin-producing Escherichia coli (STEC) serogroups according to the source. Uruguay, 2010−2017.
Four out of 45 STEC analyzed (8.8%) showed resistance to at least one of the antimicrobials tested (O26:H11, 2 isolates; O157:H7, 1 and O111:HNM, 1) (see Table 1).
Table 1. Characteristics of resistant Shiga toxin-producing Escherichia coli (STEC) isolates analyzed.
Only one STEC O157 isolate (obtained from beef sample) was resistant (2.8%); on the other hand, three out of the nine non-O157 (32%) analyzed included were resistant. Resistance to ampicillin was observed in all (n = 4) the resistant STEC analyzed; additionally, three isolates were also resistant to trimethoprim-sulfamethoxazole and one to gentamicin (see Table 1).
Resistance genes found in the only sequenced resistant STEC isolate (serogroup O111, isolated from a child with HUS, see Table 1) were aph(3″)-Ib, aph(3′)-Ia, aph(6)-Id, blaTEM−1B, sul2, and tet(A) (minimum identity and coverage of 88%). We did not find these genes in the other two susceptible STEC sequenced (see Supplementary Table 1). One STEC O145:H25 susceptible to all antibiotics tested bears the fosA7 gene, associated with resistance to fosfomycin (see Supplementary Table 1). Also, the three STEC isolates sequenced carried among others the mdfA, mphB, and mef (A) genes.
Among L. monocytogenes isolates analyzed, 27 belonged to serotype 1/2b, 20 to 4b, and 3 to 1/2a. Forty-seven isolates of L. monocytogenes were susceptible to all antibiotics tested. Two isolates were resistant to ciprofloxacin (serotypes 4b and 1/2b, both from food origin), and one isolate was resistant to erythromycin (serotype 4b, human source) (see Table 2). Serotype distribution according to the source is shown in the Figure 2.
Figure 2. Distribution of analyzed Listeria monocytogenes serotypes according to the source. Uruguay, 2010–2019.
We identified the resistance genes fosX, lin, norB, lde, mdrL, and fepA in all analyzed genomes, with a minimum identity and coverage of 90% (see Supplementary Table 2).
The overall resistance frequency found in STEC (8.8 %) suggests that its local contribution to the burden of antimicrobial resistance seems low and comparable to that previously reported in a similar study of Spain-País Vasco (15). Probably, the percentage of resistance could have been higher if we had included the tetracycline and chloramphenicol disks in the susceptibility assays (16).
Only 1 out of 36 STEC O157 isolates analyzed was resistant (2.8%); however, 3 of the 9 non-O157 (32%) were resistant. This figure coincides with results obtained by Sasaki et al. (17) and would be related to the fact that STEC non-O157 may acquire genes for antimicrobial resistance more easily than STEC O157 isolates do. Resistance to ampicillin was observed in all the resistant STEC isolates analyzed. Beta-lactamase TEM-1 is the most prevalent enzyme responsible for resistance to ampicillin in gram-negative bacteria, and the encoding genes are usually located in mobile genetic elements. In this sense, the resistance genes found aph(3″)-Ib, aph(3′)-Ia, aph(6)-Id, blaTEM−1B, and sul2 are generally located in class 1 integrons (18) as was previously reported by Colello et al. in STEC isolates recovered from animals in neighboring Argentina (19). We also found tet(A), fosA7, and mef (A), mphB genes, responsible for tetracycline, fosfomycin, and macrolide resistance, respectively. Due to economic reasons, we could only analyze the genome of three STEC isolates. We hope to carry out the whole-genome sequencing (WGS) on the remaining STEC isolates to detect other resistance genes.
Taking together the STEC isolated from beef and animal source and assuming that all the beef isolates come from the bowel of the cattle, we noticed that only 2 of these 38 (5.2%) were resistant, whereas 2 of the 7 (28.5%) isolated from humans showed resistance. These figures are similar to those previously reported by Oporto et al. in Spain-País Vasco. The difference could be explained in part for which was said above about serogroup behavior and also by selection pressure due to the frequent use of aminopenicillins in humans, especially in children (15, 17, 20). However, we cannot rule out that STEC have been acquired by cross contamination during meat processing or handling.
Treatment with antibiotics in the HUS phase is controversial; some authors do not recommend them (21), and others suggest that the early use (e.g., BD stage) of azithromycin, fosfomycin, aminoglycosides, and meropenem can be a therapeutic option (22–24). In this set of STEC, one was resistant to gentamicin, and none showed resistance to meropenem nor fosfomycin by disk diffusion assay. However, one of these fosfomycin-susceptible isolates carried the fosA7 gene. According to this finding, that gene was also detected in a fosfomycin-susceptible E. coli obtained from a Japanese river. In this Japanese isolate, the fosA gene was truncated, thus explaining the observed phenotype. However, in our STEC isolate, the fosA7 gene was complete; therefore, the in vitro susceptibility to fosfomycin could be due to the fact that the gene is not fully expressed, or its level of expression is extremely low. Nevertheless, this finding highlights the role of STEC as a reservoir of transferable resistance genes (25).
The role of azithromycin in the prevention of HUS cases remains to be assessed knowing that mef (A), unlike mph(A) gene, has a poor role in resistance to this antibiotic (26).
The obtained results show that STEC deserves special attention considering the local circulation of antibiotic-resistant full-pathogenic strains, in both humans and animals, and knowing that some of them harbor transferable resistance genes. The spread of these strains and its resistance genes will surely continue and even increase if this situation is not addressed.
CLSI and EUCAST guidelines include minimal inhibitory concentration (MIC) breakpoints for three or four antibiotics, respectively, for L. monocytogenes, and some years ago, EUCAST incorporated the disk-diffusion method for the same antibiotics (13, 27). In both guidelines, the culture medium contains horse blood, which is not everywhere commercially available. These difficulties may have led researchers to use alternative culture media and/or to interpret their results based on criteria defined for other microorganisms.
The results of this study using EUCAST guidelines show that L. monocytogenes local isolates remain fully susceptible to penicillin, gentamicin, trimethoprim-sulfamethoxazole, and meropenem. We found a low frequency of ciprofloxacin (two isolates) and erythromycin resistance (one isolate). It is important to highlight that these antibiotics are not therapeutic options for treatment of invasive infections in humans. Resistance frequency found in our study was similar to those previously reported by other authors using microdilution methods according to CLSI or EUCAST recommendations for L. monocytogenes. In the USA, Davis et al. tested 90 L. monocytogenes isolates recovered from human, food, animal, and the environment and found only 2% of ciprofloxacin resistance but not resistance to penicillin G, ampicillin, erythromycin, gentamicin, and trimethoprim-sulfamethoxazole (28). In Poland, Kuch et al. analyzed 344 human isolates (recovered between 1997 and 2013) and did not find resistance to ampicillin, penicillin, meropenem, erythromycin, trimethoprim-sulfamethoxazole, levofloxacin, gentamicin, vancomycin, nor rifampicin (29). In Australia, Wilson et al. using gradient diffusion test, found resistance to ciprofloxacin (2%) and erythromycin (1%) among 100 L. monocytogenes isolates originating from food between 1988 and 2016; no resistance was observed to penicillin G or tetracycline (30).
On the other hand, in Argentina, Prieto et al. found higher frequency of resistance to erythromycin (30%) among 250 food and human disease-related L. monocytogenes isolates recovered between 1992 and 2012 but no resistance to penicillin G, ampicillin, trimethoprim-sulfamethoxazole, gentamicin, tetracycline, nor rifampin (31).
Our results and those of the aforementioned studies differ from others in which resistance to beta-lactams is reported with high frequency, a cause for concern since this group of antibiotics is the first line of treatment for invasive listeriosis (32–35). However, these studies do not use standardized culture media for L. monocytogenes and interpret their results based on the criteria defined for Staphylococcus or Enterococcus; these factors may explain such discrepancies at least partially.
Genomic sequences analysis revealed the presence of the resistance genes fosX, lin, norB, lde, mdrL, and fepA in all L. monocytogenes strains studied.
Listeria monocytogenes is intrinsically resistant to fosfomycin due to the lack of expression of transport systems through the membrane. Also, the presence of fosX gene could explain another resistance mechanism in L. monocytogenes, since it was globally present in all strains analyzed here as well as in all the 100 studied by Hurley et al. (36). The FosX protein catalyzes the hydration of fosfomycin breaking the oxirane ring (37).
The lin gene was detected in all the analyzed strains and encoded for a lincomycin resistance ABC-F type ribosomal protection protein, a member of the ATP-binding cassette F (ABC-F) proteins (38). We did not find descriptions of this mechanism in L. monocytogenes, but we did find the lin gene in almost all genomes of this species in NCBI Pathogen Detection Isolates Browser (https://www.ncbi.nlm.nih.gov/pathogens/) suggesting that this mechanism could be involved in the natural resistance to lincomycin.
Macrolide resistance in L. monocytogenes has been linked to the methyl-transferase coding gene ermB and to efflux mechanisms mediated by multidrug efflux transporter of Listeria (MdrL) (39). We found the mdrL gene in all the analyzed genomes but not the ermB gene in any of them.
Fluoroquinolone resistance in L. monocytogenes seems to be primarily due to efflux pumps, principally through overexpression of the lde and fepA genes (31, 40, 41). NorB is a member of the major facilitator superfamily (MFS) of transporters that confers resistance to hydrophilic quinolones (norfloxacin and ciprofloxacin) and hydrophobic quinolones (sparfloxacin and moxifloxacin). The norB gene has been found by us and other authors in the analyzed genomic sequences of L. monocytogenes (30, 42).
The presence of the genes mdrL, lde, fepA, and norB coding for the respective efflux pumps seems to be universal in the L. monocytogenes isolates analyzed in this study; however, only two isolates were resistant to ciprofloxacin and one to erythromycin. Therefore, additional mechanisms or the level of expression of these genes could explain the differences in susceptibility to fluoroquinolones and macrolides. Likewise, 45 of the 50 strains analyzed had ciprofloxacin inhibition zones near the cut-off point (±5 mm) for Staphylococcus spp. (data not shown).
Antimicrobial-resistant L. monocytogenes and STEC isolates are present at a low-middle frequency. However, the high load of resistance genes found suggests that these pathogens could contribute to the local burden of antimicrobial resistance. A nationwide detailed study is necessary to determine the prevalence of resistant L. monocytogenes and STEC strains (including the resistance to antibiotics not tested in this work to STEC as tetracycline and chloramphenicol) and also to know the involved genes.
Genomic sequences of STEC and Listeria monocytogenes used in this study are available at https://www.ncbi.nlm.nih.gov/sra/ and the access numbers are in the Supplementary Material (see Table SRA with Accession numbers).
MM and SV performed the characterization of STEC and Listeria monocytogenes, DNA extraction, analysis of sequences, data processing, scientific discussion, and drafted the article. BD'A performed bioinformatic analysis of genomic sequences and drafted the article. CC, VB, and AC conducted the susceptibility studies to L. monocytogenes and STEC. LB performed scientific discussion and drafted the article. GV performed the characterization of STEC and Listeria monocytogenes, analysis of sequences, data processing, and scientific discussion and drafted the article. All the authors approved the manuscript to be published.
This work was partially supported by Comisión Sectorial de Investigación Científica (CSIC-UdelaR) program I+D grant N° 308 (responsable MM). SV received a Ph.D. grant from the National Agency for Research and Innovation (ANII).
The authors declare that the research was conducted in the absence of any commercial or financial relationships that could be construed as a potential conflict of interest.
We thank Elton Burnett for allowing us the sequencing of 40 strains analyzed in this work.
The Supplementary Material for this article can be found online at: https://www.frontiersin.org/articles/10.3389/fvets.2020.583930/full#supplementary-material
1. Varela G, Gómez-Garcia O, Ochoa T. Diarrheagenic Escherichia coli in children from Uruguay, Colombia and Peru. In: Torres AG, editor. Pathogenic Escherichia coli in Latin America. Potomac, MD: Bentham Books. (2010). p. 209–222. doi: 10.2174/978160805192211001010209
2. Braga V, Vázquez S, Vico V, Pastorino V, Mota MI, Legnani M, et al. Prevalence and serotype distribution of Listeria monocytogenes isolated from foods in Montevideo-Uruguay. Braz J Microbiol. (2017) 48:689–94. doi: 10.1016/j.bjm.2017.01.010
3. Rivas M, Chinen I, Guth BEC. Enterohemorrhagic (Shiga toxin-producing) Escherichia coli. In: Torres AG, editor. Escherichia coli in the Americas. Cham: Springer (2016). p. 97–123. doi: 10.1007/978-3-319-45092-6_5
4. Gadea MDP, Deza N, Mota MI, Carbonari C, Robatto M, D'Astek B, et al. Two cases of urinary tract infection caused by Shiga toxin-producing Escherichia coli O157:H7 strains. Rev Argent Microbiol. (2012) 44:94–96.
5. Terajima J, Izumiya H, Hara-Kudo Y, Ohnishi M. Shiga toxin (verotoxin)-producing Escherichia coli and foodborne disease: a review. Food Saf. (2017) 5:35–53. doi: 10.14252/foodsafetyfscj.2016029
6. Mir RA, Kudva IT. Antibiotic-resistant Shiga toxin-producing Escherichia coli: an overview of prevalence and intervention strategies. Zoonoses Public Health. (2019) 66:1–13. doi: 10.1111/zph.12533
7. Vázquez-Boland JA, Kuhn M, Berche P, Chakraborty T, Domínguez-Bernal G, Goebel W, et al. Listeria pathogenesis and molecular virulence determinants. Clin Microbiol Rev. (2001) 14:584–640. doi: 10.1128/CMR.14.3.584-640.2001
8. Lungu B, O'Bryan CA, Muthaiyan A, Milillo SR, Johnson MG, Crandall PG, et al. Listeria monocytogenes: antibiotic resistance in food production. Foodborne Pathog Dis. (2011) 8:569–78. doi: 10.1089/fpd.2010.0718
9. Troxler R, von Graevenitz A, Funke G, Wiedemann B, Stock I. Natural antibiotic susceptibility of Listeria species: L. grayi, L. innocua, L. ivanovii, L. monocytogenes, L. seeligeri and L. welshimeri strains. Clin Microbiol Infect. (2000) 6:525–35. doi: 10.1046/j.1469-0691.2000.00168.x
10. Swaminathan B, Gerner-Smidt P. The epidemiology of human listeriosis. Microbes Infect. (2007) 9:1236–43. doi: 10.1016/j.micinf.2007.05.011
11. Olaimat AN, Al-Holy MA, Shahbaz HM, Al-Nabulsi AA, Abu Ghoush MH, Osaili TM, et al. Emergence of antibiotic resistance in Listeria monocytogenes isolated from food products: a comprehensive review. Compr Rev Food Sci Food Saf. (2018) 17:1277. doi: 10.1111/1541-4337.12387
12. Clinical and Laboratory Standards Institute (CLSI). Performance Standards for Antimicrobial Susceptibility Testing, 29th ed. CLSI supplement M100. Wayne, PA: Clinical and Laboratory Standards Institute (2019).
13. European Committee on Antimicrobial Susceptibility Testing. Breakpoint Tables for Interpretation of MICs and Zone Diameters. Version 9.0. (2019) Available online at: https://www.eucast.org/fileadmin/src/media/PDFs/EUCAST_files/Breakpoint_tables/v_9.0_Breakpoint_Tables.pdf
14. D'Alessandro B, Pérez Escanda V, Balestrazzi L, Iriarte A, Pickard D, Yim L, et al. A novel prophage identified in strains from Salmonella enterica serovar enteritidis is a phylogenetic signature of the lineage ST-1974. Microb Genomics. (2018) 4:e000161. doi: 10.1099/mgen.0.000161
15. Oporto B, Ocejo M, Alkorta M, Marimón JM, Montes M, Hurtado A. Zoonotic approach to Shiga toxin-producing Escherichia coli: integrated analysis of virulence and antimicrobial resistance in ruminants and humans. Epidemiol Infect. (2020) 147:e164. doi: 10.1017/S0950268819000566
16. Cergole-Novella MC, Pignatari ACC, Castanheira M, Guth BEC. Molecular typing of antimicrobial-resistant Shiga-toxin-producing Escherichia coli strains (STEC) in Brazil. Res Microbiol. (2011) 162:117–23. doi: 10.1016/j.resmic.2010.09.022
17. Sasaki Y, Usui M, Murakami M, Haruna M, Kojima A, Asai T, et al. Antimicrobial resistance in Shiga toxin-producing Escherichia coli O157 and O26 isolates from beef cattle. Jpn J Infect Dis. (2012) 65:117–21.
18. Cocchi S, Grasselli E, Gutacker M, Benagli C, Convert M, Piffaretti JC. Distribution and characterization of integrons in Escherichia coli strains of animal and human origin. FEMS Immunol Med Microbiol. (2007) 50:126–32. doi: 10.1111/j.1574-695X.2007.00242.x
19. Colello R, Krüger A, Conza JD, Rossen JWA, Friedrich AW, Gutkind G, et al. Antimicrobial resistance in class 1 integron-positive Shiga toxin-producing Escherichia coli isolated from cattle, pigs, food and farm environment. Microorganisms. (2018) 6:99. doi: 10.3390/microorganisms6040099
20. Catenaccio V, Pereira I, Lucas L, Telechea H, Speranza N, Giachetto G. Uso de antibióticos en la comunidad: el plan ceibal como herramienta para promover un uso adecuado. Rev Med Urug. (2014) 30:104–11.
21. Freedman SB, Xie J, Neufeld MS, Hamilton WL, Hartling L, Tarr PI, et al. Shiga toxin–producing Escherichia coli infection, antibiotics, and risk of developing hemolytic uremic syndrome: a meta-analysis. Clin Infect Dis. (2016) 62:1251–8. doi: 10.1093/cid/ciw099
22. Joseph A, Cointe A, Mariani, Kurkdjian P, Rafat C, Hertig A. Shiga toxin-associated hemolytic uremic syndrome: a narrative review. Toxins. (2020) 12:67. doi: 10.3390/toxins12020067
23. Nitschke M, Sayk F, Härtel C, Roseland RT, Hauswaldt S, Steinhoff J, et al. association between azithromycin therapy and duration of bacterial shedding among patients with Shiga toxin–producing enteroaggregative Escherichia coli O104:H4. JAMA. (2012) 307:1046–52. doi: 10.1001/jama.2012.264
24. Ikeda K, Ida O, Kimoto K, Takatorige T, Nakanishi N, Tatara K. Effect of early fosfomycin treatment on prevention of hemolytic uremic syndrome accompanying Escherichia coli O157:H7 infection. Clin Nephrol. (1999) 52:357–62.
25. Gomi R, Matsuda T, Matsumura Y, Yamamoto M, Tanaka M, Ichiyama S, et al. Whole-genome analysis of antimicrobial-resistant and extraintestinal pathogenic Escherichia coli in river water. Appl Environ Microbiol. (2017) 83:e02703–16. doi: 10.1128/AEM.02703-16
26. Gomes C, Ruiz-Roldán L, Mateu J, Ochoa TJ, Ruiz J. Azithromycin resistance levels and mechanisms in Escherichia coli. Sci Rep. (2019) 9:6089. doi: 10.1038/s41598-019-42423-3
27. Clinical and Laboratory Standards Institute (CLSI). Methods for Antimicrobial Dilution and Disk Susceptibility Testing of Infrequently Isolated or Fastidious Bacteria, 3rd ed. CLSI guideline M45. Wayne, PA: Clinical and Laboratory Standards Institute (2015).
28. Davis JA, Jackson CR. Comparative antimicrobial susceptibility of Listeria monocytogenes, L. innocua, L. welshimeri. Microb Drug Resist. (2009) 15:27–32. doi: 10.1089/mdr.2009.0863
29. Kuch A, Goc A, Belkiewicz K, Filipello V, Ronkiewicz P, Gołebiewska A, et al. Molecular diversity and antimicrobial susceptibility of Listeria monocytogenes isolates from invasive infections in Poland (1997–2013). Sci Rep. (2018) 8:14562. doi: 10.1038/s41598-018-32574-0
30. Wilson A, Gray J, Scott Chandry P, Fox EM. Phenotypic and genotypic analysis of antimicrobial resistance among Listeria monocytogenes isolated from Australian food production chains. Genes. (2018) 9:80. doi: 10.3390/genes9020080
31. Prieto M, Martínez C, Aguerre L, Rocca MF, Cipolla, Callejo R. Antibiotic susceptibility of Listeria monocytogenes in Argentina. Enferm Infecc Microbiol Clin. (2016) 34:91–5. doi: 10.1016/j.eimc.2015.03.007
32. Jamali H, Paydar M, Ismail S, Looi CY, Wong WF, Radmehr B, et al. Prevalence, antimicrobial susceptibility and virulotyping of Listeria species and Listeria monocytogenes isolated from open-air fish markets. BMC Microbiol. (2015) 15:144. doi: 10.1186/s12866-015-0476-7
33. Aras Z, Ardiç M. Occurrence and antibiotic susceptibility of Listeria species in Turkey meats. Korean J Food Sci Anim Resour. (2015) 35:669. doi: 10.5851/kosfa.2015.35.5.669
34. Sharma S, Sharma V, Dahiya DK, Khan A, Mathur M, Sharma A. Prevalence, virulence potential, and antibiotic susceptibility profile of Listeria monocytogenes isolated from bovine raw milk samples obtained from Rajasthan, India. Foodborne Pathog Dis. (2017) 14:132–40. doi: 10.1089/fpd.2016.2118
35. Obaidat MM, Bani Salman AE, Lafi SQ, Al-Abboodi AR. Characterization of Listeria monocytogenes from three countries and antibiotic resistance differences among countries and listeria monocytogenes serogroups. Lett Appl Microbiol. (2015) 60:609–14. doi: 10.1111/lam.12420
36. Hurley D, Luque-Sastre L, Parker CT, Huynh S, Eshwar AK, Nguyen SV, et al. Whole-genome sequencing-based characterization of 100 Listeria monocytogenes isolates collected from food processing environments over a four-year period. mSphere. (2019) 4:e00252–19. doi: 10.1128/mSphere.00252-19
37. Castañeda-García A, Blázquez J, Rodríguez-Rojas A. Molecular mechanisms and clinical impact of acquired and intrinsic fosfomycin resistance. Antibiotics. (2013) 2:217. doi: 10.3390/antibiotics2020217
38. Ero R, Kumar V, Su W, Gao YG. Ribosome protection by ABC-F proteins—molecular mechanism and potential drug design. Protein Sci. (2019) 28:684–93. doi: 10.1002/pro.3589
39. Mata MT, Baquero F, Pérez-Díaz JC. A multidrug efflux transporter in Listeria monocytogenes. FEMS Microbiol Lett. (2000) 187:185–8. doi: 10.1111/j.1574-6968.2000.tb09158.x
40. Godreuil S, Galimand M, Gerbaud G, Jacquet C, Courvalin P. Efflux pump Lde is associated with fluoroquinolone resistance in Listeria monocytogenes. Antimicrob. Agents Chemother. (2003) 47:704–8. doi: 10.1128/AAC.47.2.704-708.2003
41. Guérin F, Galimand M, Tuambilangana F, Courvalin P, Cattoir V. Overexpression of the novel MATE fluoroquinolone efflux pump FepA in Listeria monocytogenes is driven by inactivation of its local repressor FepR. PLoS ONE. (2014) 9:e106340. doi: 10.1371/journal.pone.0106340
Keywords: antimicrobial resistance, Shiga toxin-producing Escherichia coli (STEC), Listeria monocytogenes, zoonotic pathogens, resistance genes
Citation: Mota MI, Vázquez S, Cornejo C, D'Alessandro B, Braga V, Caetano A, Betancor L and Varela G (2020) Does Shiga Toxin-Producing Escherichia coli and Listeria monocytogenes Contribute Significantly to the Burden of Antimicrobial Resistance in Uruguay? Front. Vet. Sci. 7:583930. doi: 10.3389/fvets.2020.583930
Received: 15 July 2020; Accepted: 09 October 2020;
Published: 06 November 2020.
Edited by:
Josefina Leon-Felix, Consejo Nacional de Ciencia y Tecnología (CONACYT), MexicoReviewed by:
Gabriel Arriagada, Universidad de O'Higgins, ChileCopyright © 2020 Mota, Vázquez, Cornejo, D'Alessandro, Braga, Caetano, Betancor and Varela. This is an open-access article distributed under the terms of the Creative Commons Attribution License (CC BY). The use, distribution or reproduction in other forums is permitted, provided the original author(s) and the copyright owner(s) are credited and that the original publication in this journal is cited, in accordance with accepted academic practice. No use, distribution or reproduction is permitted which does not comply with these terms.
*Correspondence: Gustavo Varela, Z3ZhcmVsYTE5NjFAZ21haWwuY29t; Z3ZhcmVsYUBoaWdpZW5lLmVkdS51eQ==
†These authors have contributed equally to this work
Disclaimer: All claims expressed in this article are solely those of the authors and do not necessarily represent those of their affiliated organizations, or those of the publisher, the editors and the reviewers. Any product that may be evaluated in this article or claim that may be made by its manufacturer is not guaranteed or endorsed by the publisher.
Research integrity at Frontiers
Learn more about the work of our research integrity team to safeguard the quality of each article we publish.