- 1Key Laboratory of Buffalo Genetics, Breeding and Reproduction Technology, Ministry of Agriculture and Guangxi Buffalo Research Institute, Chinese Academy of Agricultural Sciences, Nanning, China
- 2Dairy Science Department, National Research Centre, Giza, Egypt
- 3Institute of Animal and Dairy Sciences, University of Agriculture, Faisalabad, Pakistan
This study was aimed to evaluate the effects of Camelina sativa oil (CO) on fermentation kinetics and methane (CH4) production in rations with different roughage (R) to concentrate (C) ratios. Three total mixed rations (TMRs) were used as substrates (R70:C30, R50:C50, and R30:C70) supplemented with different levels of CO (0, 2, 4, 6, and 8% on dry matter basis) in an in vitro batch culture system. The enteric CH4 production was determined at different times of incubation while fermentation parameters were measured at the end of incubation. Results revealed that CO significantly decreased (P < 0.05) CH4 production at 48 h in medium (R50:C50) and low- (R30:C70) roughage diets than control. Camelina oil at all levels significantly (P < 0.05) affected ammonia nitrogen (NH3-N) and microbial protein (MCP) in all rations. Propionate concentration was increased by supplementing 8% CO to R70:C30 TMR, but it decreased with increasing levels of CO for low- and medium-roughage diets. Acetate concentration was significantly (P < 0.05) higher at 4% CO supplementation, but it decreased with 8% CO level in R30:C70 TMR. For all rations, CO decreased (P < 0.001) total bacteria, protozoa, and methanogens. Total fungi counts were affected by CO in all rations, especially with a 6% level in two rations (R30:C70 and R50:C50) and 8% level with high-roughage ration (R70:C30). Supplementation of CO in medium-roughage ration (R50:C50) showed a linear (P < 0.05) decrease in bacterial richness and evenness indices along with Shannon diversity as compared to the control. Moreover, CO also increased Firmicutes to Bacteroidetes ratio in all TMRs more effectively at higher levels. Camelina oil also affected the relative abundance of Prevotella in both low- and medium-roughage diets while increasing the abundance of Ruminobacter and Pseudobutyrivibrio. The present study concluded that CO enhanced fermentation kinetics while decreasing enteric in vitro CH4 production from fibrous diets. Thus, it may be considered as a potentially effective and environmentally friendly way of mitigating CH4 emission from livestock.
Introduction
Rumen fermentation is a major contributor to enteric methane (CH4) in global greenhouse gas (GHG) emissions and considered an important player in the climate-change scenario. Methane mitigation has been keenly sought as a potential strategy to reduce GHG emissions while improving overall feed efficiency in ruminants. It is mainly targeted to mediate rumen biohydrogenation by diverting metabolic hydrogen away from methanogenesis toward the production of volatile fatty acids (VFA) that can potentially enhance production efficiency in ruminants and reduce environmental hazards. An earlier study has proposed that limiting methanogenesis can subsequently increase the production of microbial biomass (1). Moreover, excess metabolic hydrogen escaped from methanogenesis can be incorporated into NADH, which could be subsequently utilized in VFA synthesis and other fermentation end products in the rumen (2, 3).
Various methane mitigation strategies have been employed to control CH4 formation in the rumen including use of chemicals (4), plant extracts (5, 6), and supplementation of vegetable oils (7–9). Compared with other methane mitigation strategies, supplementation of dietary fat or vegetable oils is potentially advantageous in terms of increasing dietary energy in high-producing animals while limiting CH4 production (10, 11). Recently, the trend of incorporating fats as a source of dietary energy in place of carbohydrates is becoming popular, which also contributes to CH4 mitigation (12). The unsaturated fatty acid contents of plant seeds and/or their oils can desirably influence the biohydrogenation process in the rumen (13).
The effect of plant oils such as rapeseed, sunflower, soybean, and linseed oils on rumen fermentation in diets with different roughage: concentrate ratios (R:C ratio) has been extensively studied (14–17). Studies have revealed that oilseeds can be one of the efficient ways to reduce enteric CH4 production from ruminants as they can mitigate CH4 by directly inhibiting rumen protozoa and methanogens while increasing biohydrogenation of polyunsaturated fatty acids (PUFA) to act as a sink for hydrogen produced by rumen microbes (8, 16, 18). The oil of Camelina sativa L. seed (CS), also known as false flax seed, is a rich source of unsaturated fatty acids (68–74%) especially oleic, linoleic, and linolenic acids (19, 20). This rich unsaturated fatty acid profile makes CS a high-quality fat supplement for ruminants. Moreover, CO is a quite stable oil despite having high PUFA contents owing to its rich antioxidant profile (21). Several studies have reported the use of CS meal or cake as a protein source in beef, lactating sheep, and dairy cow rations (22–24) while few have also used whole seed as a feed supplement (14, 20).
Studies have reported the nutritional benefits of CS on animal performance, but information regarding its potential effects on fermentation kinetics and ruminal microbiota is limited (13). Few studies have investigated the effect of oil extracted from whole camelina seed in dairy cows fed grass silage (25) and lambs (15). No study is available on the effect of CO on methane mitigation in high fibrous diets.
We hypothesized that PUFA contents (linolenic acid) of CO could potentially reduce CH4 production by mediating rumen biohydrogenation and microflora. Therefore, we aimed to evaluate the effect of camelina oil on in vitro CH4 production, rumen fermentation, and microbial populations in total mix rations (TMRs) with different roughage-to-concentrate ratios.
Materials and Methods
Substrates and Experimental Design
Three total mixed rations were prepared with different roughage-to-concentrate ratios, viz., 70:30, 50:50, and 30:70 coded as R70:C30, R50:C50, and R30:C70, respectively. Details of experimental TMRs are given in Table 1.
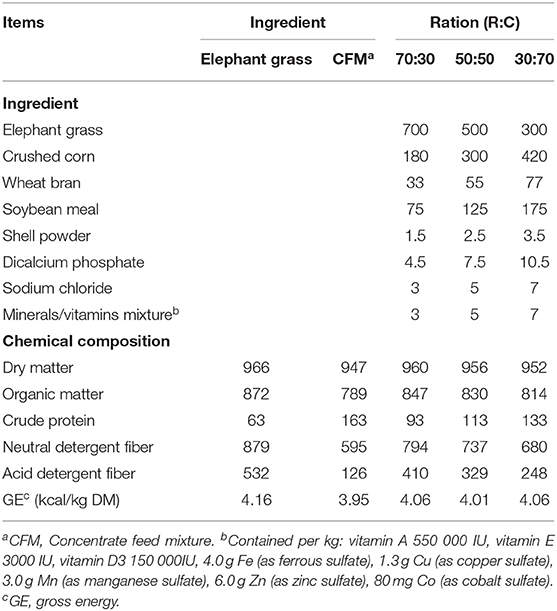
Table 1. Ingredients and chemical composition (g/kg DM) of the three total mixed rations with different elephant (R) to concentrate (C) ratios (N = 3).
Camelina oil was obtained from Mountain Rose Herbs Company, Denmark. An emulsion (oil-in-water) of CO was prepared using the ultrasonic bath (Sonics Vibra-Cell™, USA) by suspending in distilled water (1:9; v/v). This emulsion was supplemented in substrates at four levels including 2% (125 μL), 4% (250 μL), 6% (375 μL), and 8% (500 μL) on dry matter (DM) basis. Two in vitro batch culture runs were carried out separately for each ration with oil supplement. Each oil level was tested in three replicates with three blank vessels (no substrate) for each run (in total 6 replicates). Five-hundred milligram of each ration was taken in the bottle (180 mL) as a substrate, and an artificial buffer solution was added, followed by mixing ruminal fluid at a 4:1 ratio as reported previously (26). The buffer solution was prepared 1 day before starting the fermentation. The rumen fluid collected from three cannulated water buffaloes (Murrah × Chinese local with an average body weight of 450 kg) fed the same roughage and concentrate diet was used for in vitro fermentation. The ruminal digesta was collected from different places of the rumen in clean containers and strained through four layers of cheesecloth to get rumen fluid while flushing with CO2. A 50-mL ruminal buffer solution was injected into each bottle with flushing CO2, and after sealing, the bottles were kept in a pre-warmed incubator (at 39°C) for the next 48 h.
Sample Collection
During the incubation period, a 10-μL gas sample was taken from each bottle with a gas-tight syringe and manually injected into the GC system to determine the CH4 concentration at 3, 6, 9, 12, 24, and 48 h of incubation using the GC system (7890A, Agilent Technologies, USA) with a capillary column measuring 30 m × 0.32 × 0.25 mm film thickness (GC-14B, Shimadzu, USA). Five different concentrations of pure CH4 gas standards were used to develop calibration curves for the calculation of total CH4 produced. The total gas production volume was measured by a glass syringe (100 mL) before each CH4 measurement (data of gas production not shown).
After 48 h of incubation, the pH of rumen fluid was measured by a pH meter (HI 9024C; HANNA Instruments, Woonsocket, Rhode Island, USA), immediately after the opening of bottles. Samples of rumen fluid were separated into labeled plastic tubes (15 mL) for the analyses of microbial crude protein (MCP), ammonia nitrogen (NH3-N), and volatile fatty acid fractions including acetic (C2), propionic (C3), butyric (C4), and valeric (C5) acids and their isomers including isobutyric (iC4) and isovaleric (iC5) acids. After that, all the samples were stored at −20°C until further processing.
Chemical Analyses
The fatty acid profile of camelina seed oil samples was analyzed as described previously (27) and presented in Table 2. Briefly, 1.0 mL of n-hexane was added to 15 mg of CO and vortexed for 30 s followed by the addition of 1 mL of sodium methoxide (0.4 mol). The mixture was allowed to settle for 15 min after vortexing for 30 s. The upper phase, containing the fatty acid methyl ester (FAME), was recovered and analyzed by an Agilent 7890B gas chromatography (GC-FID) with a polar capillary column SP®-2560 100 m, 0.25 mm id, 0.2 μm film thickness. Helium was used as a carrier gas at a flow rate of 20 cm s-1 and split ratio 100:1. The column temperature profile was held at 100°C for 5 min, ramp to 240°C at 4°C min-1, and held at 240°C for 30 min. A sample volume of 1.0 μL was injected. The FAME was identified by comparing their relative and absolute retention times with FAME standards (from C4:0 to C24:0).
The proximate analysis of TMRs was carried out for DM (ID: 934.01), ash (ID: 942.05), and nitrogen (ID: 954.01), and ether extract (ID: 920.39) according to AOAC procedures (28). The TMR samples were also analyzed for neutral detergent fiber (NDF) and acid detergent fiber (ADF) (ID: 973.18), as described by Van Soest et al. (29) using an ANKOM2000 Fiber Analyzer Unit (ANKOM Technology Corp., Macedon, NY, USA). Neutral detergent fiber content was analyzed with heat-stable α-amylase and sodium sulfite per sample in the neutral detergent solution. The NDF and ADF were expressed, inclusive of residual ash. The gross energy (GE) of rations was determined by a bomb calorimeter (PARR Calorimeter, USA).
Samples of VFA fractions in rumen fluid (C2, C3, C4, C5, iC4, and iC5) were measured using the GC system (Model 7890A, Agilent Technologies, USA; column temperature = 120°C, injector temperature = 180°C, detector temperature = 180°C). 0.5 mL of meta-phosphoric acid (25%) was added to 1 mL of filtrate then centrifuged (1,2000 × g for 10 min), and supernatant (920 μL) plus 80 μL crotonic acid (as internal standard) in a GC bottle for a volatile fatty acid determination as reported previously (30). A portion of 4 mL filtrate was acidified with 4 mL of HCl (0.2 mol/L) and stored in a freezer (−20°C) for NH3-N using the indophenol method (31). For the determination of MCP, a 5-mL filtrate was then centrifuged at 800–1,000 rpm for 5 min at 4°C to remove feed particles. Then, 1.5 ml of supernatant was centrifuged at 12,000 rpm (4°C) for 15 min to collect the microbial biomass. After that, 0.5 mL (0.25 N) NaOH was added to the microbial biomass, mixed, and heated at 100°C in a water bath for 20 min. After this treatment, the mixture was centrifuged at 12,000 rpm (4°C) for 30 min, and the supernatant was collected for microbial CP analysis using the colorimetric method. Briefly, 100 μL supernatant was added to 5 mL Coomassie Brilliant Blue (G250, 95% ethanol, 85% phosphoric acid, and double-distilled water) and mixed well. Absorbance at 595 nm was checked by a 721 spectrophotometer colorimeter using 1 mg/ml bovine serum albumin solution (Sigma-Aldrich Co., LLC, St. Louis, Missouri, USA) as a standard equivalent (32). DNA of ruminal microbes was extracted from 1 mL of frozen samples of batch culture filtrate using the CTAB bead beating method (33).
Determination of Microbial Populations Using Real-Time PCR
Microbial populations in batch culture filtrate were determined through quantitative real-time PCR (qRT-PCR) by using a Roche LightCycler 480 RT-PCR machine (Roche, Basel, Switzerland). For the determination of methanogens and bacteria, we used a previously reported 16S-rRNA primer while for anaerobic fungi and protozoa, 18S-rRNA primers were used (34–36). Details of primers used in our study are presented in Table 3, while remaining procedures, including RT-PCR amplification profile and reaction mixture, were performed as reported in our previous study (8).
Sequencing of 16S-rRNA Gene for Determination of Rumen Bacterial Diversity
The DNA samples from one lower level (2%) and one higher level (8%) of CO were used for 16S rRNA gene sequencing to determine bacterial diversity. High-throughput (Illumina MiSeq) sequencing of the 16S rRNA gene was carried out using barcoded primers for the V3–V4 region (37). DNA libraries were sequenced using a 2 × 300 paired-end sequencing module (Illumina, San Diego). The taxonomic assignment of cleaned sequences was performed by aligning them against the SILVA database (Release128) using the Ribosomal Database Project (RDP) Classifier (http://rdp.cme.msu.edu/). Data about operational taxonomic units (OTU) were grouped taxonomically (at phylum and genus) for all treatment groups. The taxonomic classification of rumen bacteria in the steps mentioned above was performed as previously reported (38) using Qiime software (http://qiime.org/scripts/assign_taxonomy.htmL). Rarefaction curves and community bar plots were generated using R software (v2.3.2). Moreover, MOTHUR (V 1.31.2) software was used to analyze alpha diversity parameters.
Statistical Analysis
For each TMR, values recorded from three replicates of incubation run were averaged. Thus, within each TMR, there were six replicates per oil level (each corresponding to the average value recorded at two incubation runs), and each replicate was considered as an experimental unit. The effect of treatment on in vitro CH4, rumen fermentation, and alpha bacterial diversity parameters of each TMR was analyzed through the PROC GLM procedure of SAS (SAS Institute Inc., Cary, NC, USA, version 14, 2015) using the following mixed model:
where Yijk = is every observation of the ith TMR type (TMRi) with jth CO level (COj); μ is the overall mean; Eijk is the experimental error. Copy numbers of protozoa, methanogens, bacteria, and fungi were log-transformed, and then a Poisson regression model was fitted using the PROC GENMOD of SAS. The level of statistical significance for all analyses was P < 0.05.
Results
Chemical Composition of Diets and Fatty Acid Profile of Camelina Oil
Increasing the level of roughage ratio (elephant grass) in TMR decreased crude protein and increased NDF and ADF contents (Table 1). However, DM, OM, and GE were similar among the three TMRs. Analysis of fatty acid profile revealed linolenic acid as the most abundant fatty acid followed by linoleic, oleic, and arachidonic acids in CO (Table 2). Total unsaturated fatty acid (UFA) concentrations of CO were far greater than the total saturated fatty acids (831 vs. 131 mg/kg).
In vitro Methane Production
Treatment significantly affected the in vitro CH4 production in three rations at different hours of incubation (Table 4). For ration R70:C30, CO linearly increased (P < 0.05) CH4 production at 3, 6, 9, and 12 h of incubation than control. However, after 48 h of incubation CH4 production was linearly (P > 0.05) decreased with supplementation of CO (Table 4). For ration R50:C50, CO almost linearly (P < 0.001) decreased CH4 production after 24 h of incubation than control. For ration R30:C70, CO linearly increased (P < 0.05) CH4 production at 3, 6, 9, and 12 h of incubation but linearly (P < 0.001) decreased CH4 production after 48 h of incubation than control. Moreover, all TMRs exhibited a decrease in CH4 production with all CO levels compared to the control after 48 h.
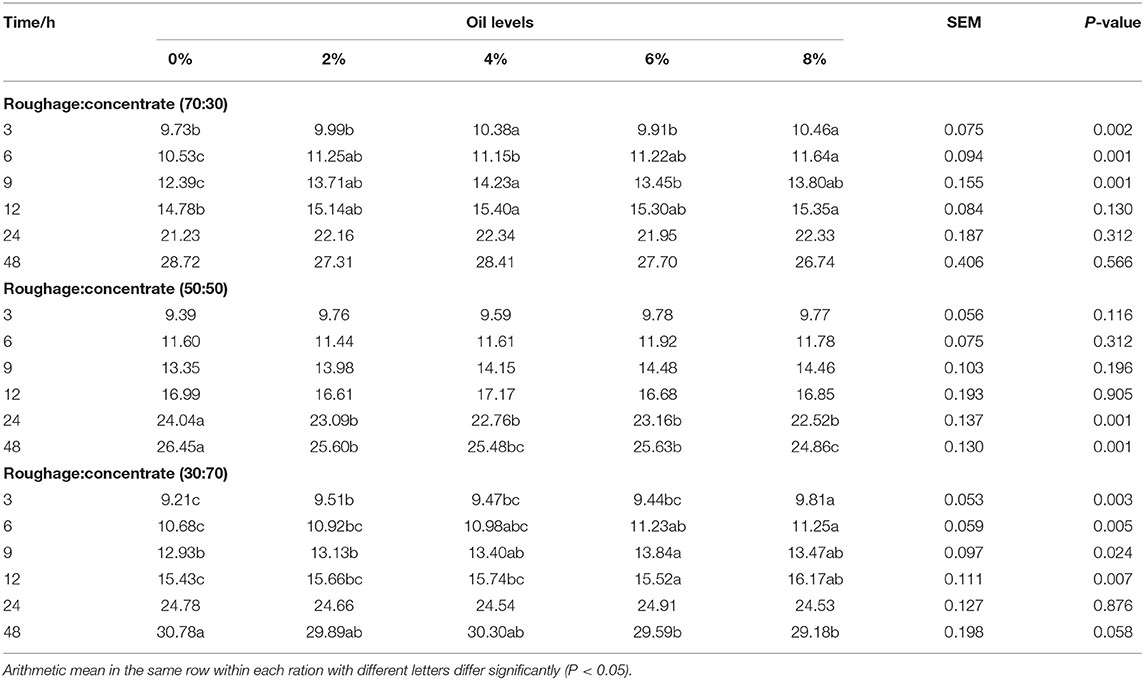
Table 4. In vitro methane (CH4) production (mL) kinetics of three total mixed rations supplemented with different levels of camelina oil (N = 6).
In vitro Rumen Fermentation Kinetics
Ruminal pH did not differ among treatments with both high- and medium-roughage rations, but increasing levels of CO linearly (P < 0.0001) decreased the pH in low-roughage ration (Table 5). Dietary CO levels, as well as the type of ration, significantly affected NH3-N and MCP concentrations (Table 5). The concentrations of NH3-N and MCP were increased with the supplementation of CO in low- and medium-roughage rations. On the other hand, both NH3-N and MCP concentrations were linearly decreased with CO supplementation in the high-roughage ration. The concentration of total VFAs increased with the increase in concentrate ratio in TMRs. Dietary supplementation of CO at 4% in high-roughage ration showed a significant increase in total VFAs. For ration R70:C30, propionic acid was linearly increased (P < 0.016) with an increasing level of CO; however, the A/P ratio was decreased (P < 0.05) with 8% CO as compared to the control. For the R50:C50 ration, 2% CO increased (p < 0.018) the concentration of C3, while higher levels showed a negative effect on C3 yield. The C4 (P < 0.003), C5 branched-chain VFA (P < 0.031), and C5 linearly (P < 0.001) decreased with the increasing level of CO. For R30:C70 TMR, 4% of CO significantly increased the cross C2, C4, and C5 branched-chain VFA and C5, while significantly decreasing (P < 0.014) the C3 concentration.
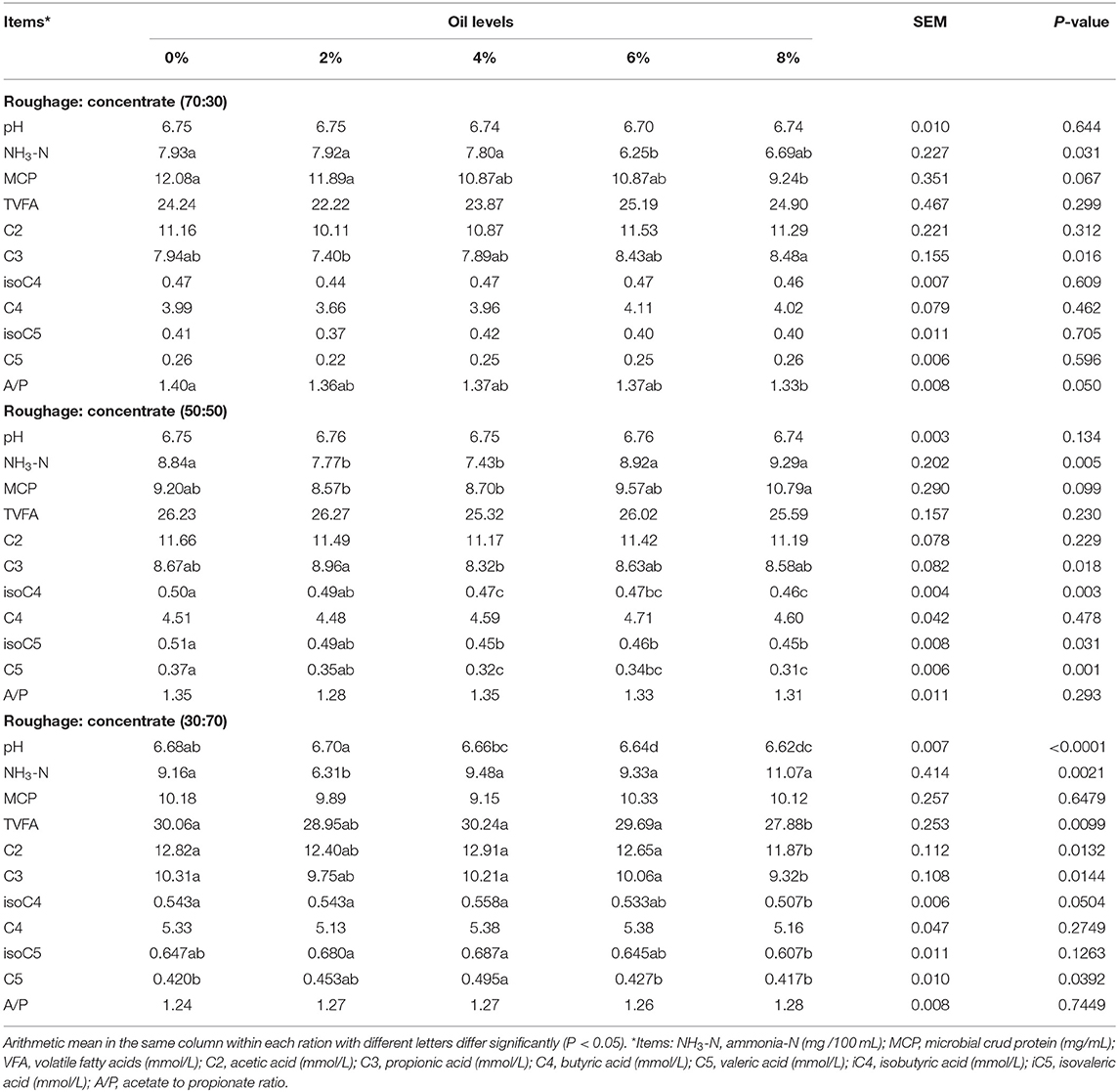
Table 5. In vitro fermentation parameters of three total mixed rations supplemented with different levels of camelina oil (N = 6).
In vitro Rumen Microbial Populations
According to qRT-PCR results, type of ration and the level of CO significantly (linear and quadratic effects, P < 0.05) affected rumen microbial populations (Table 6). The number of protozoa, methanogens, and total bacteria was linearly and quadratically decreased (P < 0.0001) with CO supplementation in all rations. However, 8% CO increased the number of protozoa, methanogens, and total bacteria as compared to the control. Moreover, CO affected the total fungal counts in all rations, especially at 6% in the low-roughage ration (R30:C70) and 8% level in the high-roughage ration (R70:C30).
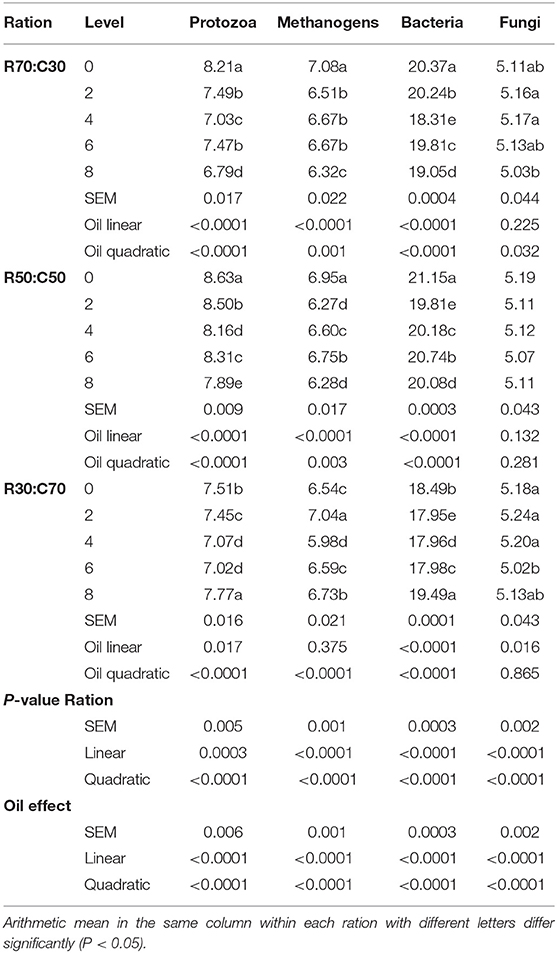
Table 6. Ruminal microbiota population (log colony forming units/mL) of three total mixed rations supplemented with different levels of camelina oil (N = 3).
In vitro Bacterial Diversity
Taxonomy Statistics and Rarefaction Curves
A total of 3164 OTU were identified through analysis of 16S-rRNA gene sequence data in the three TMRs supplemented with three CO levels (0, 2%, and 8%). The taxonomic data revealed 23 phyla, 40 classes, 86 orders, 157 families, 364 genera, and 719 species of rumen bacteria detected in rumen filtrate. Shared and unique OTU for three rations with different levels of CO are presented in Figure 1. The total number of OTU increased in the high-roughage ration (R70) in both control and supplemented groups. The higher number of unique OTUs individually identified in the control group was observed with a medium-roughage ration followed by low CO level with high- and low-roughage rations, respectively. The number of observed species (sobs) at the OTU level was greater in the high-roughage ration (R70:C30) as compared to other groups (Figure 2).
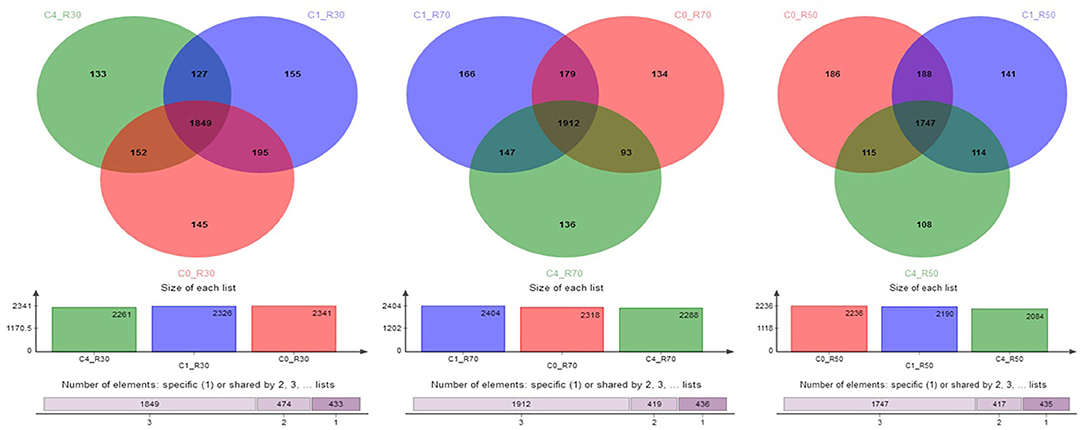
Figure 1. Shared and unique OTUs across groups. C0_R70: control with 70% high roughage; C1_R70: 1% camelina oil level with 70% high roughage; C4_R70: 4% camelina oil level with 70% high roughage; C0_R30: control with 30% low roughage; C1_R30: M1: 1% camelina oil level with 30% low roughage; C4_R30: 4% camelina oil level with 30% low roughage; C0_R50: control with 50% medium roughage; C1_R50: M1: 1% camelina oil level with 50% medium roughage; C4_R50: 4% camelina oil level with 50% medium roughage.
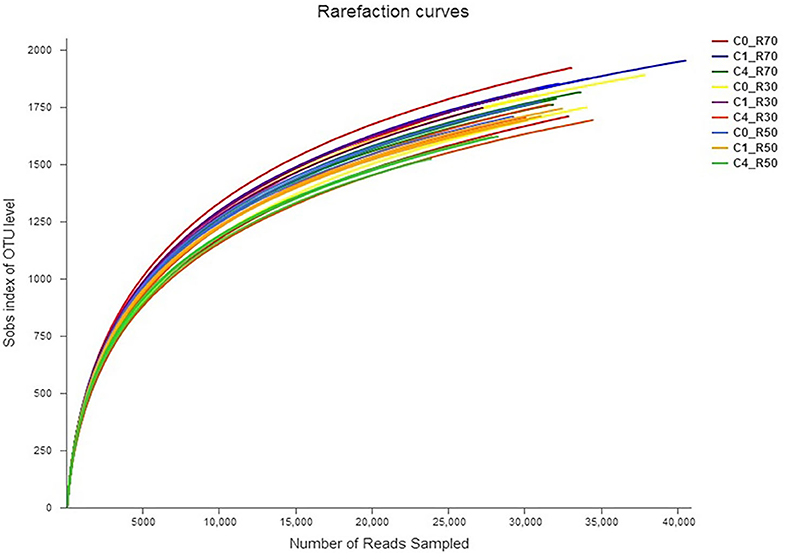
Figure 2. Rarefaction curve showing bacterial richness across samples. C0_R70: control with 70% high roughage; C1_R70: 1% camelina oil level with 70% high roughage; C4_R70: 4% camelina oil level with 70% high roughage; C0_R30: control with 30% low roughage; C1_R30: M1: 1% camelina oil level with 30% low roughage; C4_R30: 4% camelina oil level with 30% low roughage; C0_R50: control with 50% medium roughage; C1_R50: M1: 1% camelina oil level with 50% medium roughage; C4_R50: 4% camelina oil level with 50% medium roughage.
Bacterial Diversity Indices
Results of alpha bacterial diversity indices are presented in Table 7. For the R70:C30 diet, the richness (sobs, ace, and Chao) and diversity (Shannon and Simpson) indices were increased (P > 0.05) with supplementation of the lower level of CO (2%) as compared to the high level (8%) and control in a high-roughage diet (R70). On the other hand, the treatment showed a linear (P < 0.05) decrease in all richness and evenness indices as well as Shannon diversity in the medium roughage ration (R50:C50). However, the low-roughage ration (R30:C70) showed a similar trend for richness and Shannon indices that were observed in the high-roughage diet (R70) supplemented with a 2% CO level as compared to others. The ace index was significantly (P < 0.042) increased with a 2% CO level.
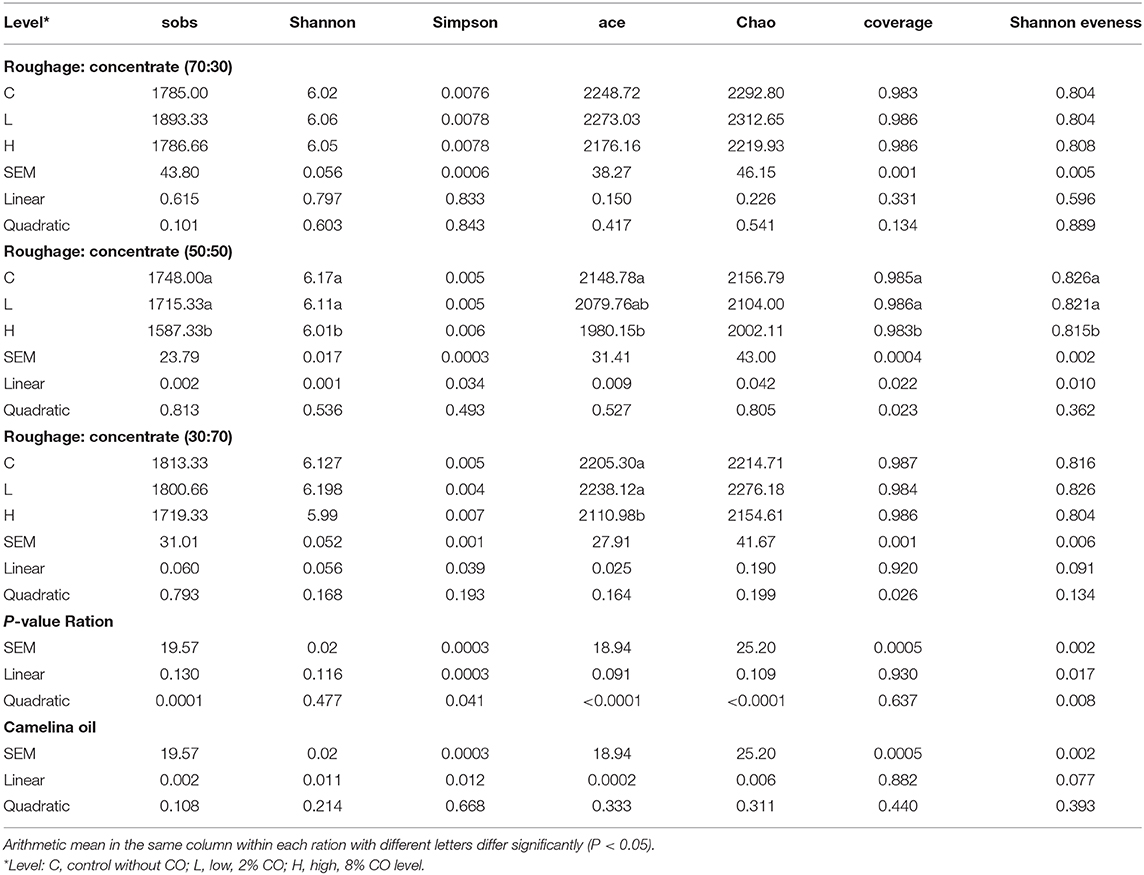
Table 7. Effect of camelina oil supplementation and three total mixed rations alpha microbial diversity estimators (N = 3).
The Relative Abundance of Bacterial Phyla
The relative abundance of different bacterial phyla observed in our study is presented in Figure 3. Results revealed five major bacterial phyla, namely, Bacteroidetes (41.77–51.08%), Firmicutes (30.44–43.26%), Proteobacteria (4.10–8.95%), Spirochaetes (1.33–4.09%), and Kiritimatiellaeota (1.14–2.60%), which were observed in all three rations. Moreover, two unique phyla Fibrobacteres and Lentisphaerae were only detected in the R70:C30 diet. The phylum Synergistetes was observed in the medium-roughage (R50:C50) diet only. Three bacterial phyla (Bacteroidetes, Firmicutes, and Proteobacteria) constituted >98% of total rumen bacteriome. The type of ration and level of CO significantly (P < 0.05) affected the bacterial populations. The population of Firmicutes (P < 0.04) and Proteobacteria (P < 0.007) was affected by the low and medium level of roughage in all treatment groups. However, supplementation of 2% CO increased (P < 0.01) the relative abundance of Bacteroidetes in the low-roughage ration. Moreover, the relative abundance of Spirochaetes was decreased with the increase in CO level in the high-roughage ration while no difference (P > 0.18) was observed in the low- and medium-roughage rations at all CO levels.
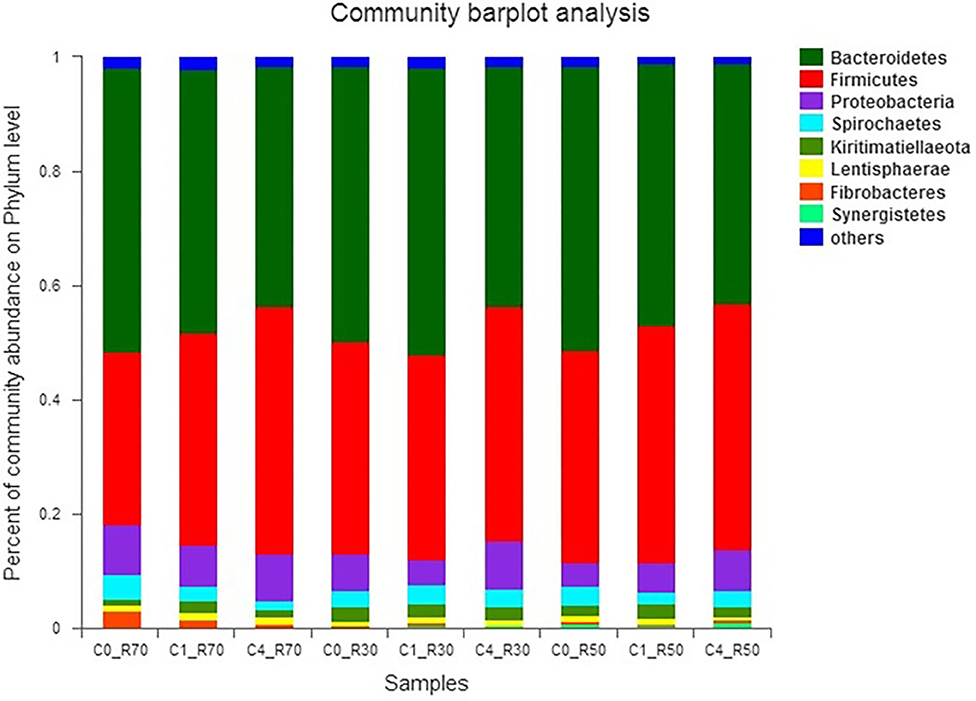
Figure 3. Relative abundance of bacterial phyla across different treatment groups. C0_R70: control with 70% high roughage; C1_R70: 1% camelina oil level with 70% high roughage; C4_R70: 4% camelina oil level with 70% high roughage; C0_R30: control with 30% low roughage; C1_R30: M1: 1% camelina oil level with 30% low roughage; C4_R30: 4% camelina oil level with 30% low roughage; C0_R50: control with 50% medium roughage; C1_R50: M1: 1% camelina oil level with 50% medium roughage; C4_R50: 4% camelina oil level with 50% medium roughage.
The Relative Abundance of Bacteria at the Genus Level
The effects of ration and CO on the relative abundance of bacterial genera are presented in Figure 4. We detected a total of 364 bacterial genera among which 11 major genera included Rikenellaceae_RC9_gut_group (9.5–15.25%), norank_f_F08, Prevotella_1 (6.82–18.18%), Ruminobacter (2.32–6.59%), unclassified_o_Clostridales (2.91–5.40%), Christensenellaceae_R-7_group (2.41–4.34%), Ruminococcaceae_NK4A214_group (1.68–3.93%), Pseudobutyrivibrio (1.21–3.06%), Ruminococcaceae_UCG-010 (1.71–2.76%), norank_o_WCHB1-41 (1.14–2.57%), and Succiniclasticum (1.10–2.75%). These bacterial phyla constituted about 55% of the total bacteriome. The higher level of CO decreased (P > 0.109) the abundance of Rikenellaceae_RC9_gut_group in low- (R30) and medium- (R50) roughage diets. However, norank_f_F08 was decreased (P < 0.007) in high (R70) roughage than other diets. The abundance of the Prevotella_1 genus was affected (P > 0.135) by both the type of ration and treatment. However, a higher CO level increased (P < 0.013) the relative abundance of Ruminobacter in low- (R30) and medium- (R50) roughage diets than low CO and control groups, but this genus showed similar abundance in high-roughage (R70) groups. Moreover, the abundance of unclassified_o_Clostridales was linearly increased (P = 0.106) in all three TMRs with the increase in CO as compared to the control. The Ruminococcus as cellulolytic bacteria (Ruminococcaceae_NK4A214_group) showed variation among different rations, and the highest (P < 0.007) abundance was observed in treated medium- (R50) roughage ration. However, Ruminococcaceae_UCG-005 (ranged from 0.50 to 2.50%) showed a shift in high-roughage rations compared to other rations (P < 0.004). A low level of CO favored Succiniclasticum in the low roughage ration but reduced (P > 0.495) its abundance in the high-roughage diet (R70). The Pseudobutyrivibrio increased (P < 0.013) in low- and medium-roughage rations supplemented with a high CO level (8%), but no difference in its abundance was observed in high-roughage rations (both treated and untreated). However, a high level of CO increased (P = 0.055) the relative abundance of Butyrivibrio 2 (ranged from 0.80 to 2.03%) in all TMRs.
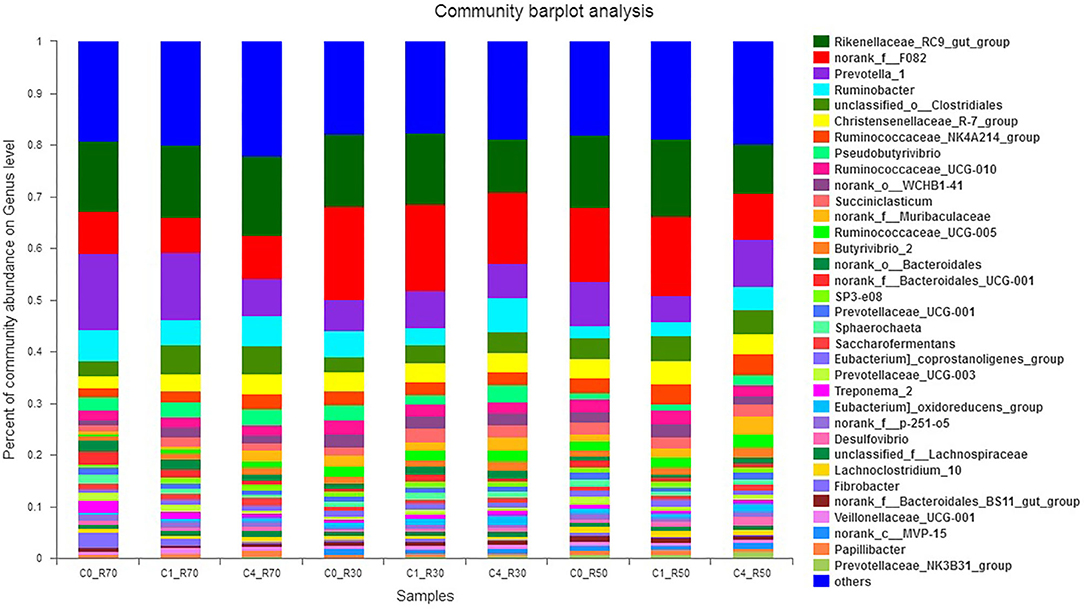
Figure 4. Relative abundance of bacterial genera across different treatment groups. C0_R70: control with 70% high roughage; C1_R70: 1% camelina oil level with 70% high roughage; C4_R70: 4% camelina oil level with 70% high roughage; C0_R30: control with 30% low roughage; C1_R30: M1: 1% camelina oil level with 30% low roughage; C4_R30: 4% camelina oil level with 30% low roughage; C0_R50: control with 50% medium roughage; C1_R50: M1: 1% camelina oil level with 50% medium roughage; C4_R50: 4% camelina oil level with 50% medium roughage.
Discussion
Our hypothesis that supplementation of camelina oil (rich in PUFA) could affect ruminal bacterial community composition leading to subsequent changes in ruminal fermentation and metabolite production was proved in findings observed in this study.
Fatty Acid Profile of Camelina Oil
The analysis of the fatty acid profile of CO showed that linolenic acid was dominant fatty acid (>30%), and total unsaturated FA represented >83% of the total fatty acid contents. Previously, total unsaturated fatty acid concentrations up to 86.3, 87.6, and 87.5% have been reported in camelina oil in different studies (25, 39, 40), respectively. However, some studies have also reported lower unsaturated fatty acid fractions (especially, oleic, linoleic, and linolenic acid) ranging from 68 to 74% of total fatty acids (19, 20). These variations in oil composition are mainly due to different extraction methods and seed quality owing to different agro-climatic conditions of crop production. Our study observed a little bit lower levels of UFA (83%) in CO as compared to earlier report (87% UFA observed by (25). A high concentration of UFA can act as an alternate hydrogen sink during bio-hydrogenation (41) resulting in a decrease in CH4 production in the rumen (42); however, it may also decrease fiber digestibility (42).
Methane Production
In the present study, we evaluated the effect of increasing levels of CO on in vitro fermentation and methane production. Dietary lipids are one of the promising ways to mitigate methane production in ruminants (18). The use of lipids to reduce enteric CH4 production is a better strategy as compared to chemical feed additives like monensin and antibiotics. Recently, a study has reported adverse effects of fatty acids, especially PUFA, on methanogenesis in the rumen (18). The anti-methanogenic effects of PUFA generally get intensified with the increase in double bond number per FA, as suggested by Czerkawski and Clapperton (43). These findings support our observation in the present in vitro study. Moreover, another study has shown more severe toxic effects of linolenic acid (C18:3) than linoleic acid (C18:3) contents of linseed on cellulolytic bacteria (44). Our findings on CH4 production are consistent with this observation because linolenic acid contents were more than 30% of the total FA in CO used in our study. The decrease in CH4 production was observed after 48 h of incubation in response to the supplementation of CO in all rations. Previously, Wang et al. (18) reported that camelina seeds decreased CH4 (ml/g DM) in a ration having a roughage-to-concentrate ratio of 60:40.
Our findings are in agreement with Bayat et al. (17), who reported a decrease in daily CH4 emission in lactating cows fed with different forage to concentrate ratios (low and high) supplemented with sunflower oil. Moreover, dietary supplementation of CO (at 10 g/kg DM) in a 50% roughage ration has shown to reduce CH4 production up to 4.8%. Variable effects of unsaturated fatty acids on CH4 emission might be associated with the nature of FA (double bond number per FA), type of oil (free oil or whole seed), and composition (roughage-to-concentrate ratio) of the rations (25).
Rumen Kinetics
It is the first report on the potential effects of CO on in vitro fermentation kinetics using a rumen inoculum from water buffalo. Therefore, due to the scarcity of available information on the subject matter, we compared our findings with studies that used different parts of camelina seed (whole seeds or seed meal) or other vegetable oils. Rumen kinetics such as NH3 and VFAs are major end products of rumen fermentation (45). As mentioned before, the majority of earlier studies have used camelina seeds (14, 20) or camelina meal or cake (23, 24), but only two reports are available regarding the use of camelina oil in dairy cattle (25) and fattening sheep (15). All levels of camelina oil linearly decreased rumen pH in R30:C70 ration in the present study; however, no effect of CO on pH was observed in other diets as reported previously (25). Moreover, NH3-N was affected in all three diets and linearly decreased with a high concentrate ration. A similar pattern was observed regarding the concentration of MCP. However, Wang et al. (18) found that NH3 was high after 24 h of incubation in 60% forage and 40% concentrate diet supplemented with camelina seeds. They suggested that the increase in NH3 contents in rumen might indicate accelerated lysis of microbes or reduced nitrogen utilization. However, these findings are in disagreement with the present study, where NH3 decreased in high-roughage diet, and this variation might be attributed to the diet composition (different R:C ratios), form (oil vs. seeds), and level of supplemental camelina. Another possible reason for the decrease in NH3-N might be the inhibition of bacteria caused by antioxidant compounds of CO (46). Different essential oils and/or plant extracts have also shown to inhibit NH3-producing bacteria (Prevotella spp. and R. amylophilus) up to 77% in sheep (47).
The VFAs are end-products of ruminal fermentation and provide ruminants with about 70% of metabolizable energy (48). The supplementation of CO increased propionate contents in R70:C30 while decreasing it in the other two diets except in the R50:C50 ration (only at 2% CO), which showed a higher propionate than the control group. Additionally, acetate production showed a similar trend like propionate, exhibiting the diet-specific effect of CO on total and individual VFA contents (49). Our findings are in agreement with previous study reporting an increase in the propionate and decrease in total VFA and acetate concentrations in a response to the supplementation of camelina seed (5%) in the diet (55% alfalfa hay: 45% concentrate) under in vitro conditions (50). In contrast, Hurtaud and Peyraud (20) observed a significant (P < 0.001) decrease in rumen acetate and increase (P = 0.014) in propionate in cows fed camelina seeds in the diet (59:41 R:C ratio). Our findings indicate that relatively higher amounts of fiber in the diet and level of CO supplementation can influence molar VFA proportions as well as fiber-degrading bacteria that are particularly sensitive to high-oil diets (44). Therefore, a decrease in acetate and an increase in MCP concentrations observed in R50:C50 and R30:C70 rations suggest a potential inhibition of fiber-degrading bacteria by CO (51).
Rumen Microbial Population
Our study revealed significant effects of supplementation of camelina oil on ruminal microbial populations, as shown in Table 6. Inhibition of specific microbial population observed in this study might be due to the direct toxic effects of CO on rumen microbes and/or higher non-fermentable fatty acids by replacing readily fermentable carbohydrates (52, 53).
The diet composition significantly affects the diversity and abundance of microbial populations owing to different nutrient contents and fermentation profiles (54). Similar findings have been reported earlier in cows fed high-roughage or high-concentrate diets (55). A significant decrease in methanogenic archaea was observed in goats when diet changed from alfalfa hay to a mixture of oats and alfalfa hay (56). In our study, camelina oil significantly affected the composition of ruminal microbiota, which is mainly attributed to the potential effects of its fatty acid contents. The significant decrease in the number of protozoa and methanogens well-correlated with the results of the CH4 yield observed in this study. The rumen archaea use hydrogen to yield CH4 to facilitate fiber digestion (57). The inhibition of methanogens is potentially beneficial owing to its significant association with methane emission and animal productivity (57).
The increasing levels of CO levels in diets had no effect on ruminal protozoa (58). However, the decrease in bacterial nitrogen and the number of cellulolytic bacteria have been observed in diets supplemented with 8% dietary lipids (50). Moreover, Bayat et al. (25) reported that CO exhibited no effect on protozoa, total bacteria, methanogens, fungi, and fiber-degrading bacteria. Variable results reported by different studies may be attributed to the dietary form of camelina used in the diet, composition of feed ingredients, roughage-to-concentrate ratio, and dose of oil supplements (25, 49, 59). Another explanation of our findings is the manipulation of rumen biohydrogenation as PUFA (such as linolenic acid) contents of CO could use hydrogen produced by rumen microbes serving as an alternate hydrogen sink (9).
Rumen Bacterial Diversity
Our study revealed significant effects of CO and ration (roughage-to-concentrate ratio) on ruminal bacterial diversity. Both dietary and treatment factors altered ruminal bacterial communities as well as their richness and diversity indices, as reported previously (13). In addition, shifts in ruminal microbial community and decrease in richness and diversity indices in response to supplementation of camelina seeds as compared to the dietary fat have been reported earlier (13). The study suggested two possible reasons for these findings: (1) higher saturated fatty acid contents of dietary fat (Megalac) posed deleterious effects on rumen environment and (2) toxic effects of PUFA on rumen bacteria such as cellulolytic bacteria.
Our study revealed higher bacterial species richness both in high-roughage diets and in the lower level of CO as revealed by rarefaction curves. The four phyla, including Bacteroidetes, Firmicutes, Proteobacteria, and Spirochaetes, were detected as major phyla in our study, which is in agreement with earlier reports (13, 60). However, change in roughage-to-concentrate ratio favored two phyla Fibrobacteres and Lentisphaerae in the R70:C30 diet and one phylum Synergistetes in R50:C50 diet.
Two dominant phyla Firmicutes and Bacteroidetes constitute a major portion of rumen bacteriome. The Firmicutes produce propionate and butyrate, while Bacteroidetes produce short-chain fatty acids (SCFAs) by fermenting polysaccharides and cellulosic plant material with potential beneficial effects (61–63). In our study, an increase in Firmicutes to Bacteroidetes ratio was observed in all roughage rations more specifically at higher levels (8%) of CO. Earlier study has reported the potential association of this ratio of two major bacterial phyla (Firmicutes: Bacteroidetes) with energy homeostasis in animals (adipogenesis) and milk-fat yield in dairy cattle (64). An increase in this ratio in response to CO supplementation in our study correlated well with higher concentrations of propionate and butyrate observed in the present study. Moreover, acetate is produced by Bacteroidetes as a result of the fermentation of indigestible carbohydrates, which decreased in all treated TMRs. Camelina oil possesses abundant polyphenols and PUFA (mainly C18:3) contents that exhibited more obvious results against Gram -ve bacteria (13, 18, 44).
At the genus level, no data is available on the effects of the supplementation of CO on the rumen microbiome. Only one study has reported the effects of camelina seeds on microbial diversity (13). Our study revealed shifts in the relative abundance of the Rikenellaceae RC9 gut group with a high level of CO in low- and medium-roughage rations, which is in agreement with previous findings observed with the use of Tucumã oil in TMR (65). Prevotella was observed as a second major bacterial genus in our present study (Figure 4). Higher and lower levels of CO affected the relative abundance of Prevotella spp. that fluctuated among three rations. A low level of CO in the high-roughage ration (R70) showed a higher abundance of Prevotella spp. as compared to low- (R30:C70) and medium-roughage (R50:C50) rations. Contrarily, an increase in the relative abundance of Prevotella species (P. bryantii and P. ruminicola) in response to supplementation of higher levels of plant essential oils has been reported earlier (66). Moreover, dietary protein contents have shown to positively affect the relative abundance of Prevotella (60). However, the Prevotella abundance was decreased by 8% dietary ether extract, which is in agreement with our findings observed with a high level of CO in the present study (13). Variable findings observed in previous reports might be due to the type of oil, its levels, and the composition of diets. Moreover, studies have also revealed the supportive role of Prevotella in fiber digestion and utilization through facilitating other rumen microbes (13, 60, 66, 67). In our study, a decrease in acetate production with a high-roughage ration was observed in response to the shifting of Ruminococcaceae_UCG-005 in high-roughage rations (60) possibly due to the toxic effects of linolenic acid contents (of camelina oil) on Ruminococci spp. (13).
Ruminal fermentation of starch by Ruminobacter and Succiniclasticum usually yields acetic and succinic acids (68). Our findings regarding the decrease of Succiniclasticum in the high-roughage ration are consistent with earlier report (60). On the other side, Ruminobacter abundance was decreased by a lower level of CO (2%) in low- and medium-roughage rations but increased with a high level of CO (8%) in all rations. Fermentation of polysaccharides by rumen bacteria (Butyrivibrio and Pseudobutyrivibrio) produces formic, butyric, and acetate acids in the rumen (69). The present study showed a higher level of butyric acid with supplementation of CO at 4 and 6% levels in all rations, which is mainly attributed to the increased number of Butyrivibrio species. Additionally, a decrease in butyric acid contents with the increase in roughage level was observed; however, the relative abundance of Pseudobutyrivibrio and Butyrivibrio showed a similar trend in all three TMRs and decreased with supplementation of CO at a lower level for low- and medium-roughage diets. However, Dai et al. (13) reported that supplementation of camelina seeds decreased (P < 0.05) Butyrivibrio without affecting the relative abundance of Pseudobutyrivibrio. A possible reason for decreased Butyrivibrio and Pseudobutyrivibrio with the low CO level and their increase with the high CO level might be attributed to the first shock of the toxic effects of linolenic acid on cellulolytic bacteria. Moreover, Pseudobutyrivibrio is more resistant than Butyrivibrio in the presence of C18:2n6, and C18:3n3, as well as Butyrivibrio, has significant negative association and sensitivity to toxic effects of PUFA (47, 70). However, previous study has reported a linear decrease in Butyrivibrio and Pseudobutyrivibrio with increasing levels of starch while the linear increase was observed with increasing fiber contents in the diet, which is consistent with our findings (60). This shows that Butyrivibrio and Pseudobutyrivibrio preferably ferment structural carbohydrates to yield energy. Additionally, similar functions of Eubacterium and Butyrivibrio regarding the fermentation of structural carbohydrates have been reported earlier (71). Conclusively, our study revealed desirable effects of camelina oil on rumen microbiota and fermentation kinetics while reducing CH4 emission which is advantageous in terms of increasing feed efficiency and reducing environmental hazards.
Conclusions
Camelina oil reduced CH4 production in total mixed rations, revealing its potential as a feed additive to mitigate overall methane emissions from livestock production. Supplementation of CO at the 8% level had adversely affected the diversity and evenness of bacterial populations. However, CO also increased Firmicutes to Bacteroidetes ratio in all rations particularly at a higher level of supplementation (8%). Camelina oil also negatively affected the Prevotella in both low- and medium-roughage diets while increasing the abundance of Ruminobacter and Pseudobutyrivibrio, revealing its potential to favor the production of formate, butyrate, and acetate. Conclusively, our study revealed that supplementation of 4–6% CO in dairy rations is suitable to improve nutrient digestion and utilization while reducing CH4 production. However, further in vivo studies are required to confirm our findings and to optimize the best level of CO to improve feed efficiency in ruminants while mitigating methane emanation.
Data Availability Statement
The datasets presented in this study can be found in online repositories. The names of the repository/repositories and accession number(s) can be found at: https://www.ncbi.nlm.nih.gov/, SRR10072455.
Ethics Statement
The animal study was reviewed and approved by Ethics Committee of Guangxi Buffalo Research Institute, Chinese Academy of Agriculture Sciences, China.
Author Contributions
HE: conceptualization, data curation, and writing—original draft. HE, ML, and FH: methodology. FH: software. CY: validation and supervision. HE and FH: formal analysis and investigation. ML, LP, XL, KP, and CY: resources. FH and CY: writing—review & editing. HE and ML: project administration. CY: funding acquisition. All authors contributed to the article and approved the submitted version.
Funding
Funds from the National Natural Science Foundation of China (No. 31560649), National Key Research and Development Program of China (2016YFD0500507 and 2018YFD0501600), and the Guangxi Natural Science Foundation (2018GXNSFAA281162) were utilized to conduct this study.
Conflict of Interest
The authors declare that the research was conducted in the absence of any commercial or financial relationships that could be construed as a potential conflict of interest.
Acknowledgments
HE was awarded ASEN Talented Young Scientist fellowship from Guangxi Science and Technology Department, China.
References
2. Chalupa W. Manipulating rumen fermentation. J Anim Sci. (1977) 45:585–99. doi: 10.2527/jas1977.453585x
3. Ungerfeld EM. Shifts in metabolic hydrogen sinks in the methanogenesis-inhibited ruminal fermentation: a meta-analysis. Front Microbiol. (2015) 6:37. doi: 10.3389/fmicb.2015.00037
4. Eckard R, Grainger C, De Klein C. Options for the abatement of methane and nitrous oxide from ruminant production: a review. Livest Sci. (2010) 130:47–56. doi: 10.1016/j.livsci.2010.02.010
5. Patra AK, Saxena J. A new perspective on the use of plant secondary metabolites to inhibit methanogenesis in the rumen. Phytochemistry. (2010) 71:1198–222. doi: 10.1016/j.phytochem.2010.05.010
6. Cieslak A, Szumacher-Strabel M, Stochmal A, Oleszek W. Plant components with specific activities against rumen methanogens. Animal. (2013) 7:253–65. doi: 10.1017/S1751731113000852
7. Patra AK, Yu Z. Effects of essential oils on methane production and fermentation by, and abundance and diversity of, rumen microbial populations. Appl Environ Microbiol. (2012) 78:4271–80. doi: 10.1128/AEM.00309-12
8. Ebeid HM, Mengwei L, Kholif AE, Hassan F-U, Lijuan P, Xin L, et al. Moringa oleifera oil modulates rumen microflora to mediate in vitro fermentation kinetics and methanogenesis in total mix rations. Curr Microbiol. (2020) 77:1271–82. doi: 10.1007/s00284-020-01935-2
9. Guyader J, Eugène M, Noziere P, Morgavi D, Doreau M, Martin C. Influence of rumen protozoa on methane emission in ruminants: a meta-analysis approach 1. Animal. (2014) 8:1816–25. doi: 10.1017/S1751731114001852
10. Jenkins TC. Lipid metabolism in the rumen. J Dairy Sci. (1993) 76:3851–63. doi: 10.3168/jds.S0022-0302(93)77727-9
11. Grainger C, Beauchemin KA. Can enteric methane emissions from ruminants be lowered without lowering their production? Anim Feed Sci Technol. (2011) 166-167, 308–20. doi: 10.1016/j.anifeedsci.2011.04.021
12. Islam M, Lee S-S. Advanced estimation and mitigation strategies: a cumulative approach to enteric methane abatement from ruminants. J Anim Sci Technol. (2019) 61:122. doi: 10.5187/jast.2019.61.3.122
13. Dai X, Weimer PJ, Dill-Mcfarland KA, Brandao VL, Suen G, Faciola AP. Camelina seed supplementation at two dietary fat levels change ruminal bacterial community composition in a dual-flow continuous culture system. Front Microbiol. (2017) 8:2147. doi: 10.3389/fmicb.2017.02147
14. Halmemies-Beauchet-Filleau A, Kokkonen T, Lampi A-M, Toivonen V, Shingfield K, Vanhatalo A. Effect of plant oils and camelina expeller on milk fatty acid composition in lactating cows fed diets based on red clover silage. J Dairy Sci. (2011) 94:4413–30. doi: 10.3168/jds.2010-3885
15. Noci F, Monahan F, Moloney A. The fatty acid profile of muscle and adipose tissue of lambs fed camelina or linseed as oil or seeds. Animal. (2011) 5:134–47. doi: 10.1017/S1751731110001485
16. El-Sherbiny M, Cieslak A, Pers-Kamczyc E, Szczechowiak J, Kowalczyk D, Szumacher-Strabel M. A nanoemulsified form of oil blends positively affects the fatty acid proportion in ruminal batch cultures. J Dairy Sci. (2016) 99:399–407. doi: 10.3168/jds.2015-9328
17. Bayat A, Ventto L, Kairenius P, Stefański T, Leskinen H, Tapio I, et al. Dietary forage to concentrate ratio and sunflower oil supplement alter rumen fermentation, ruminal methane emissions, and nutrient utilization in lactating cows. Trans Animal Sci. (2017) 1:277–86. doi: 10.2527/tas2017.0032
18. Wang S, Kreuzer M, Braun U, Schwarm A. Effect of unconventional oilseeds (safflower, poppy, hemp, camelina) on in vitro ruminal methane production and fermentation. J Sci Food Agric. (2017) 97:3864–70. doi: 10.1002/jsfa.8260
19. Flachowsky G, Langbein T, Böhme H, Schneider A, Aulrich K. Effect of false flax expeller combined with short-term vitamin E supplementation in pig feeding on the fatty acid pattern, vitamin E concentration and oxidative stability of various tissues. J Anim Physiol Anim Nutr. (1997) 78:187–95. doi: 10.1111/j.1439-0396.1997.tb00870.x
20. Hurtaud C, Peyraud J. Effects of feeding camelina (seeds or meal) on milk fatty acid composition and butter spreadability. J Dairy Sci. (2007) 90:5134–45. doi: 10.3168/jds.2007-0031
21. Abramovic H, Abram V. Physico-chemical properties, composition and oxidative stability of Camelina sativa oil. Food Technol Biotechnol. (2005) 43:63–70. Available online at: https://hrcak.srce.hr/110424
22. Moloney A, Woods V, Crowley J. A note on the nutritive value of camelina meal for beef cattle. Irish J Agr Food Res. (1998) 37:243–7. Available online at: https://www.jstor.org/stable/25562337
23. Moriel P, Nayigihugu V, Cappellozza B, Gonçalves E, Krall J, Foulke T, et al. Camelina meal and crude glycerin as feed supplements for developing replacement beef heifers. J Anim Sci. (2011) 89:4314–24. doi: 10.2527/jas.2010-3630
24. Szumacher-Strabel M, Cieślak A, Zmora P, Pers-Kamczyc E, Bielińska S, Stanisz M, et al. Camelina sativa cake improved unsaturated fatty acids in ewe's milk. J Sci Food Agric. (2011) 91:2031–7. doi: 10.1002/jsfa.4415
25. Bayat A, Kairenius P, Stefański T, Leskinen H, Comtet-Marre S, Forano E, et al. Effect of camelina oil or live yeasts (Saccharomyces cerevisiae) on ruminal methane production, rumen fermentation, and milk fatty acid composition in lactating cows fed grass silage diets. J Dairy Sci. (2015) 98:3166–81. doi: 10.3168/jds.2014-7976
26. Szumacher-Strabel M, Martin S, Potkanski A, Cieslak A, Kowalczyk J. Changes in fermentation processes as the effect of vegetable oil supplementation in in vitro studies. J Anim Feed Sci. (2004) 13:215–8. doi: 10.22358/jafs/73843/2004
27. Zahran HA, Tawfeuk HZ. Physicochemical properties of new peanut (Arachis hypogaea L.) varieties. OCL. (2019) 26:19. doi: 10.1051/ocl/2019018
28. AOAC. Official Methods of Analysis. Association of Official Analytical Chemists. 16th ed. Washington, DC (1997).
29. Van Soest PV, Robertson J, Lewis B. Methods for dietary fiber, neutral detergent fiber, and nonstarch polysaccharides in relation to animal nutrition. J Dairy Sci. (1991) 74:3583–97. doi: 10.3168/jds.S0022-0302(91)78551-2
30. Qin W. Determination of rumen volatile fatty acids by means of gas chromatography. J Nanjing Agric Univ. (1982) 4:110–6.
31. Weatherburn M. Phenol-hypochlorite reaction for determination of ammonia. Anal Chem. (1967) 39:971–4. doi: 10.1021/ac60252a045
32. Makkar H, Sharma O, Dawra R, Negi S. Simple determination of microbial protein in rumen liquor. J Dairy Sci. (1982) 65:2170–3. doi: 10.3168/jds.S0022-0302(82)82477-6
33. Yu Z, Morrison M. Improved extraction of PCR-quality community DNA from digesta and fecal samples. BioTechniques. (2004) 36:808–12. doi: 10.2144/04365ST04
34. Sylvester JT, Karnati SK, Yu Z, Morrison M, Firkins JL. Development of an assay to quantify rumen ciliate protozoal biomass in cows using real-time PCR. J Nutr. (2004) 134:3378–84. doi: 10.1093/jn/134.12.3378
35. Denman SE, Mcsweeney CS. Development of a real-time PCR assay for monitoring anaerobic fungal and cellulolytic bacterial populations within the rumen. FEMS Microbiol Ecol. (2006) 58:572–82. doi: 10.1111/j.1574-6941.2006.00190.x
36. Denman SE, Tomkins NW, Mcsweeney CS. Quantitation and diversity analysis of ruminal methanogenic populations in response to the antimethanogenic compound bromochloromethane. FEMS Microbiol Ecol. (2007) 62:313–22. doi: 10.1111/j.1574-6941.2007.00394.x
37. Klindworth A, Pruesse E, Schweer T, Peplies J, Quast C, Horn M, et al. Evaluation of general 16S ribosomal RNA gene PCR primers for classical and next-generation sequencing-based diversity studies. Nucleic Acids Res. (2013) 41:e1. doi: 10.1093/nar/gks808
38. Caporaso JG, Kuczynski J, Stombaugh J, Bittinger K, Bushman FD, Costello EK, et al. QIIME allows analysis of high-throughput community sequencing data. Nat Methods. (2010) 7:335. doi: 10.1038/nmeth.f.303
39. Fröhlich A, Rice B. Evaluation of Camelina sativa oil as a feedstock for biodiesel production. Ind Crops Prod. (2005) 21:25–31. doi: 10.1016/j.indcrop.2003.12.004
40. Chantsalnyam B, Otgonbayar C, Enkhtungalag O, Odonmajig P. Physical and chemical characteristics and fatty acids composition of seeds oil isolated from Camelina sativa (L) cultivated in Mongolia. Mongolian J Chem. (2013) 14:80–3. doi: 10.5564/mjc.v14i0.205
41. Johnson KA, Johnson DE. Methane emissions from cattle. J Anim Sci. (1995) 73:2483–92. doi: 10.2527/1995.7382483x
42. Lins TDA, Terry S, Silva R, Pereira L, Jancewicz L, He M, et al. Effects of the inclusion of Moringa oleifera seed on rumen fermentation and methane production in a beef cattle diet using the rumen simulation technique (Rusitec). Animal. (2019) 13:283–91. doi: 10.1017/S1751731118001428
43. Czerkawski J, Clapperton J. Fats as energy-yielding compounds in the ruminant diet. In: Proceedings-Easter School in Agricultural Science, Nottingham, UK: University of Nottingham (1984). doi: 10.1016/B978-0-408-10864-5.50018-8
44. Maia MR, Chaudhary LC, Figueres L, Wallace RJ. Metabolism of polyunsaturated fatty acids and their toxicity to the microflora of the rumen. Antonie Van Leeuwenhoek. (2007) 91:303–14. doi: 10.1007/s10482-006-9118-2
45. Russell J. Rumen Microbiology and Its Role in Ruminant Nutrition. Ithaca, NY: Cornell University (2002). p. 19.
46. Kutlu H, Tekeli A, çelik L. Plant extracts; a new rumen moderator in ruminant diets. Tekirdag Ziraat Fakültesi Dergisi. (2007) 4:71–9.
47. Wallace RJ, Chaudhary LC, Mckain N, Mcewan NR, Richardson AJ, Vercoe PE, et al. Clostridium proteoclasticum: a ruminal bacterium that forms stearic acid from linoleic acid. FEMS Microbiol Lett. (2006) 265:195–201. doi: 10.1111/j.1574-6968.2006.00487.x
48. Christaki E, Bonos E, Giannenas I, Florou-Paneri P. A review: aromatic plants as a source of bioactive compounds. Agriculture. (2012) 2:228–43. doi: 10.3390/agriculture2030228
49. Benchaar C, Romero-Pérez G, Chouinard P, Hassanat F, Eugene M, Petit H, et al. Supplementation of increasing amounts of linseed oil to dairy cows fed total mixed rations: effects on digestion, ruminal fermentation characteristics, protozoal populations, and milk fatty acid composition. J Dairy Sci. (2012) 95:4578–90. doi: 10.3168/jds.2012-5455
50. Brandao V, Dai X, Paula E, Silva L, Marcondes M, Shenkoru T, et al. Effect of replacing calcium salts of palm oil with camelina seed at 2 dietary ether extract levels on digestion, ruminal fermentation, and nutrient flow in a dual-flow continuous culture system. J Dairy Sci. (2018) 101:5046–59. doi: 10.3168/jds.2017-13558
51. Carro M, Ranilla M, Martín-García A, Molina-Alcaide E. Comparison of microbial fermentation of high-and low-forage diets in Rusitec, single-flow continuous-culture fermenters and sheep rumen. Animal. (2009) 3:527–34. doi: 10.1017/S1751731108003844
52. Dohme F, Machmüller A, Estermann B, Pfister P, Wasserfallen A, Kreuzer M. The role of the rumen ciliate protozoa for methane suppression caused by coconut oil. Lett Appl Microbiol. (1999) 29:187–92. doi: 10.1046/j.1365-2672.1999.00614.x
53. Newbold CJ, De La Fuente G, Belanche A, Ramos-Morales E, Mcewan NR. The role of ciliate protozoa in the rumen. Front Microbiol. (2015) 6:1313. doi: 10.3389/fmicb.2015.01313
54. Jin D, Kang K, Wang H, Wang Z, Xue B, Wang L, et al. Effects of dietary supplementation of active dried yeast on fecal methanogenic archaea diversity in dairy cows. Anaerobe. (2017) 44:78–86. doi: 10.1016/j.anaerobe.2017.02.007
55. Shanks OC, Kelty CA, Archibeque S, Jenkins M, Newton RJ, Mclellan SL, et al. Community structures of fecal bacteria in cattle from different animal feeding operations. Appl Environ Microbiol. (2011) 77:2992–3001. doi: 10.1128/AEM.02988-10
56. Mohammadzadeh H, Yáñez-Ruiz DR, Martínez-Fernandez G, Abecia L. Molecular comparative assessment of the microbial ecosystem in rumen and faeces of goats fed alfalfa hay alone or combined with oats. Anaerobe. (2014) 29:52–8. doi: 10.1016/j.anaerobe.2013.11.012
57. Dong L, Zhang T, Diao Q. Effect of dietary supplementation of moringa oleifera on the production performance and fecal methanogenic community of lactating dairy cows. Animals. (2019) 9:262. doi: 10.3390/ani9050262
58. Halmemies-Beauchet-Filleau A, Shingfield KJ, Simpura I, Kokkonen T, Jaakkola S, Toivonen V, et al. Effect of incremental amounts of camelina oil on milk fatty acid composition in lactating cows fed diets based on a mixture of grass and red clover silage and concentrates containing camelina expeller. J Dairy Sci. (2017) 100:305–24. doi: 10.3168/jds.2016-11438
59. Shingfield KJ, Ahvenjärvi S, Toivonen V, Vanhatalo A, Huhtanen P, Griinari JM. Effect of incremental levels of sunflower-seed oil in the diet on ruminal lipid metabolism in lactating cows. Br J Nutr. (2008) 99:971–83. doi: 10.1017/S0007114507853323
60. Li R, Teng Z, Lang C, Zhou H, Zhong W, Ban Z, et al. Effect of different forage-to-concentrate ratios on ruminal bacterial structure and real-time methane production in sheep. PLoS ONE. (2019) 14:e0214777. doi: 10.1371/journal.pone.0214777
61. Deusch S, Camarinha-Silva A, Conrad J, Beifuss U, Rodehutscord M, Seifert J. A structural and functional elucidation of the rumen microbiome influenced by various diets and microenvironments. Front Microbiol. (2017) 8:1605. doi: 10.3389/fmicb.2017.01605
62. Indugu N, Vecchiarelli B, Baker LD, Ferguson JD, Vanamala JK, Pitta DW. Comparison of rumen bacterial communities in dairy herds of different production. BMC Microbiol. (2017) 17:190. doi: 10.1186/s12866-017-1098-z
63. Xin J, Chai Z, Zhang C, Zhang Q, Zhu Y, Cao H, et al. Comparing the microbial community in four stomach of dairy cattle, yellow cattle and three yak herds in qinghai-tibetan plateau. Front Microbiol. (2019) 10:1547. doi: 10.3389/fmicb.2019.01547
64. Jami E, White BA, Mizrahi I. Potential role of the bovine rumen microbiome in modulating milk composition and feed efficiency. PLoS ONE. (2014) 9:e85423. doi: 10.1371/journal.pone.0085423
65. Ramos AFO, Terry SA, Holman DB, Breves G, Pereira LGR, Silva AGM, et al. Tucumã oil shifted ruminal fermentation, reducing methane production and altering the microbiome but decreased substrate digestibility within a RUSITEC fed a mixed hay – concentrate diet. Front Microbiol. (2018) 9:1647. doi: 10.3389/fmicb.2018.01647
66. Mcintosh F, Williams P, Losa R, Wallace R, Beever D, Newbold C. Effects of essential oils on ruminal microorganisms and their protein metabolism. Appl Environ Microbiol. (2003) 69:5011–4. doi: 10.1128/AEM.69.8.5011-5014.2003
67. Petri R, Schwaiger T, Penner G, Beauchemin K, Forster R, Mckinnon J, et al. Changes in the rumen epimural bacterial diversity of beef cattle as affected by diet and induced ruminal acidosis. Appl Environ Microbiol. (2013) 79:3744–55. doi: 10.1128/AEM.03983-12
68. Sun B, Wang X, Bernstein S, Huffman MA, Xia D-P, Gu Z, et al. Marked variation between winter and spring gut microbiota in free-ranging Tibetan Macaques (Macaca thibetana). Sci Rep. (2016) 6:26035. doi: 10.1038/srep26035
69. Palevich N, Kelly WJ, Ganesh S, Rakonjac J, Attwood GT. Butyrivibrio hungatei MB2003 competes effectively for soluble sugars released by Butyrivibrio proteoclasticus B316T during growth on xylan or pectin. Appl Environ Microbiol. (2019) 85:e02056–e02018. doi: 10.1101/395103
70. Moon CD, Pacheco DM, Kelly WJ, Leahy SC, Li D, Kopečný J, et al. Reclassification of Clostridium proteoclasticum as Butyrivibrio proteoclasticus comb. nov., a butyrate-producing ruminal bacterium. Int J Syst Evol Microbiol. (2008) 58:2041–5. doi: 10.1099/ijs.0.65845-0
Keywords: camelina oil, methane, rumen fermentation, methanogenesis, bacterial diversity
Citation: Ebeid HM, Hassan F-u, Li M, Peng L, Peng K, Liang X and Yang C (2020) Camelina sativa L. Oil Mitigates Enteric in vitro Methane Production, Modulates Ruminal Fermentation, and Ruminal Bacterial Diversity in Buffaloes. Front. Vet. Sci. 7:550. doi: 10.3389/fvets.2020.00550
Received: 08 April 2020; Accepted: 13 July 2020;
Published: 26 August 2020.
Edited by:
Zora Varadyova, Slovak Academy of Sciences, SlovakiaReviewed by:
Malgorzata Szumacher-Strabel, Poznan University of Life Sciences, PolandAnuraga Jayanegara, Bogor Agricultural University, Indonesia
Copyright © 2020 Ebeid, Hassan, Li, Peng, Peng, Liang and Yang. This is an open-access article distributed under the terms of the Creative Commons Attribution License (CC BY). The use, distribution or reproduction in other forums is permitted, provided the original author(s) and the copyright owner(s) are credited and that the original publication in this journal is cited, in accordance with accepted academic practice. No use, distribution or reproduction is permitted which does not comply with these terms.
*Correspondence: Chengjian Yang, eWNqMDc0NkBzaW5hLmNvbQ==
†These authors have contributed equally to this work