- 1Department of Disease Control, Faculty of Veterinary Medicine, Hokkaido University, Sapporo, Japan
- 2Department of Advanced Pharmaceutics, Faculty of Veterinary Medicine, Hokkaido University, Sapporo, Japan
- 3Agriculture Research Department, Animal Research Center, Hokkaido Research Organization, Shintoku, Japan
- 4Department of Veterinary Clinical Medicine, Faculty of Veterinary Medicine, Hokkaido University, Sapporo, Japan
- 5School of Veterinary Medicine, Rakuno Gakuen University, Ebetsu, Japan
- 6Shibetsu Animal Hospital, Shibetsu, Japan
- 7Department of Veterinary Medical Science, Faculty of Agriculture, University of Miyazaki, Miyazaki, Japan
- 8Department of Antibody Drug Development, Graduate School of Medicine, Tohoku University, Sendai, Japan
- 9New Industry Creation Hatchery Center, Tohoku University, Sendai, Japan
- 10Research and Development Center, Fuso Pharmaceutical Industries, Ltd., Osaka, Japan
- 11New Business and International Business Development, Fuso Pharmaceutical Industries, Ltd., Osaka, Japan
- 12Division of Bioresources, Research Center for Zoonosis Control, Hokkaido University, Sapporo, Japan
- 13Global Station for Zoonosis Control, Global Institution for Collaborative Research and Education (GI-CoRE), Hokkaido University, Sapporo, Japan
Bovine mycoplasmosis caused by Mycoplasma bovis results in pneumonia and mastitis in cattle. We previously demonstrated that the programmed death 1 (PD-1)/PD-ligand 1 (PD-L1) pathway is involved in immune dysfunction during M. bovis infection and that prostaglandin E2 (PGE2) suppressed immune responses and upregulated PD-L1 expression in Johne's disease, a bacterial infection in cattle. In this study, we investigated the role of PGE2 in immune dysfunction and the relationship between PGE2 and the PD-1/PD-L1 pathway in M. bovis infection. In vitro stimulation with M. bovis upregulated the expressions of PGE2 and PD-L1 presumably via Toll-like receptor 2 in bovine peripheral blood mononuclear cells (PBMCs). PGE2 levels of peripheral blood in infected cattle were significantly increased compared with those in uninfected cattle. Remarkably, plasma PGE2 levels were positively correlated with the proportions of PD-L1+ monocytes in M. bovis-infected cattle. Additionally, plasma PGE2 production in infected cattle was negatively correlated with M. bovis-specific interferon (IFN)-γ production from PBMCs. These results suggest that PGE2 could be one of the inducers of PD-L1 expression and could be involved in immunosuppression during M. bovis infection. In vitro blockade assays using anti-bovine PD-L1 antibody and a cyclooxygenase 2 inhibitor significantly upregulated the M. bovis-specific IFN-γ response. Our study findings might contribute to the development of novel therapeutic strategies for bovine mycoplasmosis that target PGE2 and the PD-1/PD-L1 pathway.
Introduction
Bovine mycoplasmosis caused by Mycoplasma bovis is characterized by chronic pneumonia, therapy-resistant mastitis, otitis, and arthritis (1–4). M. bovis has several immunosuppressive characteristics in vitro, such as inhibition of proliferative response of bovine peripheral blood mononuclear cells (PBMCs), induction of apoptosis in bovine lymphocytes, and delay of apoptosis in bovine monocytes, along with suppressed production of interferon (IFN)-γ and tumor necrosis factor (TNF)-α (5–7). These characteristics could cause chronic progression of the disease and, especially during the lung infection, and allow coinfection with other bacteria and viruses (2, 3). However, the mechanisms underlying the dysfunction in M. bovis infection have remained unclear.
Programmed death-1 (PD-1) is an immunoinhibitory receptor that is expressed on activated T cells and has been involved in immune dysfunction during various chronic infections (8–10). After binding of PD-ligand 1 (PD-L1), PD-1 induces T-cell dysfunction by inhibiting T-cell receptor signaling. This immune dysfunction is called T cell exhaustion. On the other hand, treatment with monoclonal antibodies (mAbs) specific to PD-1 or PD-L1 is capable of reactivating functions of exhausted T cells. PD-1/PD-L1 could be a potential therapeutic target in patients with chronic infections. We previously demonstrated that the expression of PD-1 on T cells and PD-L1 on monocytes were significantly increased in M. bovis-infected cattle (11). Furthermore, the blockade of PD-1/PD-L1 pathway by antibodies in vitro activated immune responses in M. bovis-infected cattle (11). Therefore, the T cell exhaustion caused by PD-1/PD-L1 might be involved in the immune dysfunction during bovine mycoplasma.
Prostaglandin E2 (PGE2) is one of the lipid mediators that are derived from arachidonic acid synthesized by cyclooxygenase isoenzymes (COX-1 and COX-2) (12, 13). It is known that PGE2 promotes the immune dysfunction associated with several tumors and chronic inflammation (14, 15). Interestingly, recent reports on cancer have shown a relationship between PGE2 and the PD-1/PD-L1 pathway (16, 17). Our previous study demonstrated that PGE2 suppressed the Th1 response in cattle and upregulated PD-L1 expression in bovine PBMCs in vitro (18). In addition, PGE2 showed immune dysfunction effects in other bovine chronic diseases, Johne's disease (18), which is known to be a chronic bovine disease by Mycobacterium avium subsp. paratuberculosis (MAP) and bovine leukemia virus (BLV) infection (19). Furthermore, the dual blocking of PGE2 and the PD-1/PD-L1 pathway substantially enhanced the MAP and BLV-specific T-cell reaction in cattle. However, the involvement of PGE2 in the immune dysfunction of bovine mycoplasmosis has not yet been fully investigated.
In this study, we investigated the role of PGE2 in immune dysfunction and the relationship between PGE2 and the PD-1/PD-L1 pathway in M. bovis infection. We believe that our findings will help in the development of novel strategies for bovine mycoplasmosis.
Materials and Methods
Bacterial Strain
M. bovis strain PG45 (ATCC25523) was used in the experiments of this study. M. bovis was cultured in NK broth (Miyarisan Pharmaceutical, Tokyo, Japan) at 37°C for 72 h and collected by centrifugation. The bacteria were washed with phosphate-buffered saline (PBS), and colony-forming units were counted using the NK agar plate (Miyarisan Pharmaceutical) by dilution method. The bacteria were then resuspended in RPMI 1640 medium (Sigma-Aldrich, St. Louis, MO, USA) containing 10% heat-inactivated fetal bovine serum (FBS) (Thermo Fisher Scientific, Waltham, MA, USA), 2 mM L-glutamine, 100 U/ml penicillin, and 100 μg/ml streptomycin (Thermo Fisher Scientific) and stored at −80°C until use.
Ethical Approval
All experimental procedures were conducted following approval from the local committee for animal studies according to the Hokkaido University (17–24). Written informed consent was obtained from all owners of cattle sampled in this study.
Bovine Samples
Peripheral blood samples of cattle were obtained from adult Holstein-breed cattle in Hokkaido, Japan. Cattle infected with M. bovis were diagnosed clinically and microbiologically at Rakuno Gakuen University and Hokkaido University. M. bovis infection was confirmed with PCR by using clinical samples as described previously (21). The symptoms of infected cattle included pneumonia, arthritis, and otitis media. Control blood samples of M. bovis-uninfected cattle were obtained from adult Holstein-breed cattle in Hokkaido, Japan. Negative control cattle were serologically negative for M. bovis infection according to enzyme-linked immunosorbent assay (ELISA). Briefly, ELISA plates (Thermo Fisher Scientific) were coated with 100 μl of solubilized M. bovis (PG45, 50 μg/ml in carbonate buffer) as the target antigen at 37°C for 17 h. After washing the plates four times with a wash solution (PBS with 0.1% Tween20), 100 μl of serum sample was added to each plate. After incubation at 37°C for 1 h, the plates were washed three times with TSB-T [PBS with 50 mM Tris, 0.1% bovine serum albumin (BSA), and 0.05% Tween20] and incubated with skim milk (Wako, Osaka, Japan) as a protein blocker at 37°C for 2 h. After washing the plates thrice with TSB-T, protein G-conjugated horseradish peroxidase (Rockland Immunochemicals, Pottstown, PA, USA) was added to the wells and the plates were incubated at 37°C for 1 h. After washing the plates thrice with TSB-T, 3-ethylbenzothiazolin-6-sulfonic acid (ABTS; Sera Care, Milford, MA, USA) was added to the wells and the optical density was measured at 415 nm using a plate reader (iMarkTM Microplate Absorbance Reader, Bio-Rad, Hercules, CA, USA).
Cell Preparation and Culture
Bovine PBMCs were purified from blood samples with density gradient centrifugation using Percoll (GE Healthcare, Little Chalfont, England, UK). As described previously (20), CD14+ cells were freshly isolated from bovine PBMCs using the autoMACS Pro System (Miltenyi Biotec, Bergisch Gladbach, Germany) with anti-bovine CD14 mAb (CAM36A, Washington State University Monoclonal Antibody Center, Pullman, WA, USA), and anti-mouse IgG1 MicroBeads (Miltenyi Biotec). CD14− cells were prepared from negative fractions of CD14+ cell sorting. The purity of cell populations was confirmed using FACS Verse (BD Biosciences, San Jose, CA, USA). Only highly pure populations (>90%) were used for experiments.
PBMCs, CD14+ cells, or CD14− cells (1 × 106 cells) from uninfected cattle were seeded into each well of a 48-well flat-bottom plate (Corning Inc., Corning, NY, USA) with RPMI 1640 medium (Sigma-Aldrich) containing 10% heat-inactivated FBS (Thermo Fisher Scientific), 2 mM L-glutamine, 100 U/ml penicillin, and 100 μg/ml streptomycin (Thermo Fisher Scientific) and were cultured in the presence of live M. bovis at a multiplicity of infection (MOI) of 0.1:1, 1:1, or 10:1; 1.5 ng/ml of heat-killed M. bovis; 2.5 μM of PGE2 (Cayman Chemical, Ann Arbor, MI, USA); or 100 ng/ml of fibroblast-stimulating lipopeptide-1 (FSL-1; Adipogen Life Sciences, San Diego, CA, USA) at 37°C under 5% CO2 for 24 h. Heat-killed M. bovis was prepared by heating the bacteria to 70°C for 10 min.
To investigate the effects of blocking TLR2 signaling, PBMCs (1 × 106 cells) from uninfected cattle were incubated with sparstolonin B (SsnB; Sigma-Aldrich) in the presence of 100 ng/ml of FSL-1 or 1.5 ng/ml of heat-killed M. bovis in a 48-well flat-bottom plate (Corning Inc.) at 37°C under 5% CO2 for 24 h. DMSO (Nacalai Tesque, Kyoto, Japan) was used as negative controls.
To assess the effect of soluble factors on PD-L1 expression, 24-h culture supernatants of PBMCs incubated with live M. bovis were collected and fresh PBMCs were cultured in these culture supernatants. To prepare 24-h culture supernatants, PBMCs (1 × 106 cells) from uninfected cattle were seeded and were cultured with or without live M. bovis at a MOI of 0.1:1, 1:1, or 10:1 as described above. After centrifugation (300 × g for 3 min), the culture supernatants of PBMCs were collected. Bacteria and cell-free supernatants were obtained by filtration through a 0.2-μm filter (Pall Life Sciences, Washington, NY, USA). To confirm that the supernatants were not contaminated with M. bovis, each supernatant was incubated in NK broth (Miyarisan Pharmaceutical) for 72 h and the broth samples were tested with PCR targeting M. bovis-specific gene (21). Then, fresh PBMCs were isolated from the same individual and cultured in the 24-h culture supernatants at 37°C under 5% CO2 for 24 h.
Quantitation of PGE2 by ELISA
To investigate whether M. bovis antigen and TLR2 signaling promote PGE2 production, PBMCs, CD14+ cells, or CD14− cells (1 × 106 cells) from uninfected cattle were incubated with live M. bovis, heat-killed M. bovis, or FSL-1 (Adipogen Life Sciences) as described above. Culture supernatants were collected, and PGE2 levels were measured using Prostaglandin E2 Express ELISA kit (Cayman Chemical), according to the manufacturer's instructions. The optical density was read at 450 nm in a microplate reader (Corona Electronics, Tokyo, Japan). To compare PGE2 levels in peripheral blood between infected cattle and uninfected cattle, PGE2 concentrations in serum or plasma were measured using ELISA.
Flow Cytometric Analysis of PD-L1
To investigate PD-L1 expression on immune cells, PBMCs were blocked with PBS containing 10% goat serum (Thermo Fisher Scientific) at 37°C for 15 min and were washed and stained with anti-bovine PD-L1 mAb (4G12, rat IgG2a; 20) or rat IgG2a isotype control (R35-95, BD Biosciences) in the presence of anti-CD11b mAb (CC126, mouse IgG2b, Bio-Rad, Hercules, USA) at room temperature for 20 min. After washing with PBS containing 1% BSA (Sigma-Aldrich), cells were stained with PerCp/Cy5.5-conjugated anti-CD14 mAb (CAM36A, mouse IgG1, Washington State University Monoclonal Antibody Center), APC-conjugated anti-rat immunoglobulin polyclonal Ab (Southern Biotech, Birmingham, AL, USA), FITC-conjugated anti-mouse IgG2b polyclonal Ab (Beckman Coulter, Fullerton, CA, USA), and Fixable Viability Dye eFluor 780 (eBioscience, San Diego, CA, USA) at room temperature for 20 min. PerCp/Cy5.5-conjugated mouse IgG1 isotype control (15H6, Southern Biotech) and mouse IgG2b isotype control (A-1, Southern Biotech) were used as isotype-matched control antibodies for fluorescence minus one staining. mAbs CAM36A and 15H6 were conjugated with PerCp/Cy5.5 using the Lightning-Link PerCp/Cy5.5 Antibody Labeling Kit (Innova Biosciences, Cambridge, England, UK). Cells were then washed and immediately analyzed using FACS Verse (BD Biosciences) and FACSuite Software (BD Biosciences).
IFN-γ Assay
To investigate IFN-γ levels in peripheral blood of infected cattle, IFN-γ concentration in plasma were measured using Bovine IFN-γ ELISA development kit (Mabtech, Nacka Strand, Sweden). To investigate M. bovis-specific IFN-γ response in infected cattle, PBMCs (4 × 105 cells) from infected cattle were incubated with 1.5 μg/ml of heat-killed M. bovis in 96-well plates (Corning, Inc.) at 37°C under 5% CO2 for 5 days. Subsequently, culture supernatants were obtained from individual wells and were tested for bovine IFN-γ using the ELISA kit (Mabtech) as described above.
Immunohistochemical Assays of PD-L1 and PGE2
Immunohistochemical assays were performed as previously described (18), with slight modification. Briefly, lung tissue was collected from M. bovis-infected cattle with pneumonia (Japanese Black, ale, 2 months old). M. bovis infection was confirmed with the loop-mediated isothermal amplification assay at Miyazaki University as described previously (22). Then, sections of the lung lesions were immunohistochemically stained for PGE2 and PD-L1 using anti-PGE2 polyclonal Ab (ab2318, Abcam, Cambridge, England, UK) and anti-PD-L1 mAb (6C11-3A11, Rat IgG2a; 22).
Functional Analysis of Combined COX-2 Inhibition and PD-L1 Blockade
To evaluate T-cell response against the bacterial antigenic stimulation, cell proliferation assay was performed. PBMCs isolated from M. bovis-infected cattle were labeled with carboxyfluorescein diacetate succinimidyl ester (CFSE) (Thermo Fisher Scientific) and cultured with 1.5 μg/ml of heat-killed M. bovis in 96-well plates (Corning, Inc.) with RPMI 1640 medium (Sigma-Aldrich) supplemented with 10% heat-inactivated FBS (Thermo Fisher Scientific), 2 mM L-glutamine, 100 U/ml penicillin, and 100 μg/ml streptomycin (Thermo Fisher Scientific) at 37°C with 5% CO2 for 5 days. Then, PBMCs were harvested and incubated in PBS containing 10% goat serum (Sigma-Aldrich) at room temperature for 15 min to prevent non-specific reactions. The cells were then stained with anti-CD4-Alexa Fluor 647 (CC30; Bio-Rad), anti-CD8-PerCp/Cy5.5 (CC63, Bio-Rad), anti-IgM-PE/Cy7 antibodies (IL-A30; Bio-Rad), and Fixable Viability Dye eFluor 780 (eBioscience, San Diego, CA, USA) at room temperature for 20 min. mAb CC30 was pre-labeled with Alexa Fluor 647 Zenon Mouse IgG1 Labeling Kit (Thermo Fisher Scientific). mAbs CC63 and IL-A30 were conjugated with PerCp/Cy5.5 and PE/Cy7, respectively, with Lightning-Link Conjugation Kits (Innova Biosciences). The cells were then washed with PBS containing 1% BSA (Sigma-Aldrich) and analyzed immediately by FACS Verse (BD Biosciences) and FACSuite Software (BD Biosciences).
To investigate the effects of dual blockade using a COX-2 inhibitor and anti-PD-L1 mAb, PBMCs (4 × 105 cells) from infected cattle were incubated with 10 μg/ml of anti-bovine PD-L1 mAb (4G12; 21) and/or 10 μM of meloxicam (Sigma-Aldrich) in the presence of 1.5 μg/ml of heat-killed M. bovis, or 1.0 μg/ml of anti-CD3 mAb (MM1A, Washington State University Monoclonal Antibody Center) and 1.0 μg/ml of anti-CD28 mAb (CC220, Bio-Rad) in 96-well plates (Corning, Inc.) with RPMI 1640 medium (Sigma-Aldrich) supplemented with 10% heat-inactivated FBS (Thermo Fisher Scientific), 2 mM L-glutamine, 100 U/ml penicillin, and 100 μg/ml streptomycin (Thermo Fisher Scientific) at 37°C under 5% CO2 for 5 days. DMSO (Nacalai Tesque) and rat IgG (Sigma-Aldrich) were used as negative controls. Culture supernatants were obtained from individual wells and were tested for IFN-γ production using the ELISA kit (Mabtech) as described above.
Statistical Analysis
Differences were identified using Dunnett's test, Mann–Whitney U-test, and Steel–Dwass test. Correlation statistics were analyzed using Spearman correlation analysis. The statistical analysis program MEPHAS (http://www.gen-info.osaka-u.ac.jp/MEPHAS/) was used to perform statistical analysis. A P < 0.05 was considered statistically significant.
Results
M. bovis Infection Upregulated PD-L1 Expression via PGE2
Our previous study showed that expression of PD-L1 on monocytes was increased in PBMCs of M. bovis-infected cattle (11). To confirm whether M. bovis directly upregulates PD-L1 expression in bovine PBMCs during in vitro infection, PBMCs from uninfected cattle were cultured with or without live M. bovis and PD-L1 expression was analyzed on monocytes by flow cytometry. As shown in Figure S1, CD11b+CD14+ monocytes were gated and analyzed for expression of PD-L1. PD-L1 expression on CD11b+CD14+ monocytes was upregulated in the culture with live M. bovis when compared with that in the culture without live M. bovis (Figure 1A). Our recent studies showed that PGE2 was one of the inducers of PD-L1 expression in Johne's disease. Thus, to identify whether PGE2 induced by live M. bovis upregulated PD-L1 expression, PGE2 production in culture supernatants was assessed using ELISA. PGE2 production was increased when PBMCs were cultured with live M. bovis. Interestingly, PGE2 production was positively correlated with PD-L1 expression on CD11b+CD14+ monocytes (Figures 1B,C). To confirm whether innate immune response against M. bovis contribute to PGE2 production and PD-L1 expression in the PBMC cultures, PBMCs from uninfected cattle were cultured with or without heat-killed M. bovis (Figures 1D–F). Heat-killed M. bovis also upregulated PD-L1 expression on CD11b+CD14+ monocytes in line with increased PGE2 production in culture supernatants (Figures 1D–F). To test whether culture supernatants containing PGE2 induced by M. bovis upregulated PD-L1 expression, PBMCs from uninfected cattle were cultured in supernatant from PBMCs that were co-cultured with live M bovis for 24 h. PD-L1 expression on CD11b+CD14+ monocytes cultured in 24-h culture supernatants of PBMCs with live M. bovis was upregulated when compared with that without live M. bovis (Figure 1G). For further confirmation, PBMCs from uninfected cattle were incubated with PGE2 and PD-L1 expression on monocytes was analyzed by flow cytometry. PD-L1 expression on CD11b+CD14+ monocytes incubated with PGE2 was increased when compared with that treated with negative controls (Figure 1H). These results indicate that M. bovis could upregulate PD-L1 expression on monocytes via PGE2.
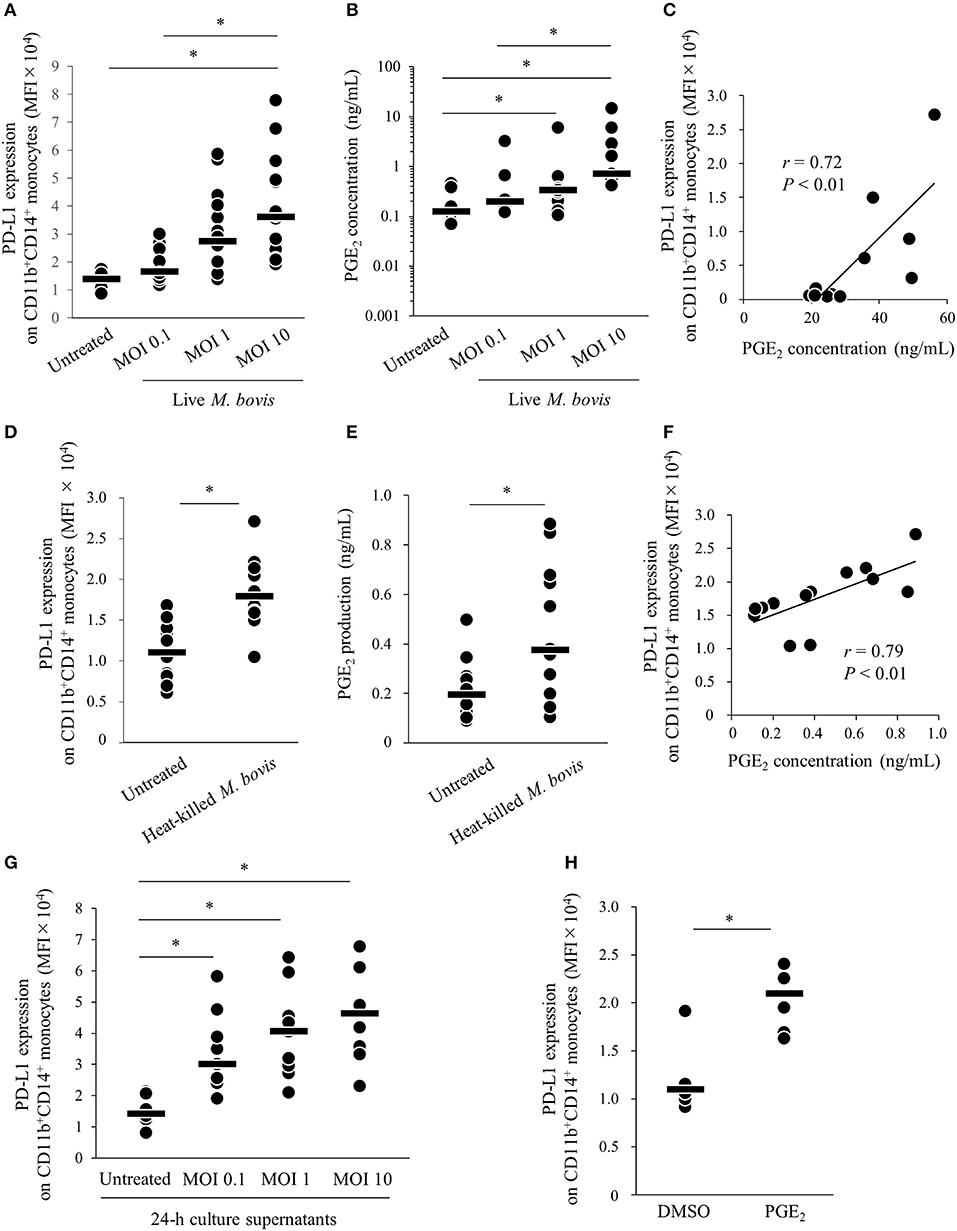
Figure 1. M. bovis upregulates PD-L1 and PGE2 expression. (A–F) PBMCs from M. bovis-uninfected cattle were incubated with (A–C) live M. bovis (MOI of 0.1, 1, or 10) or (D–F) heat-killed M. bovis (1.5 ng/ml) for 24 h. (A) PD-L1 expression on CD11b+CD14+ monocytes (untreated: n = 14, MOI 0.1: n = 14, MOI 1: n = 14, MOI 10: n = 14) was determined using flow cytometry. (B) PGE2 levels in culture supernatants (untreated: n = 9, MOI 0.1: n = 9, MOI 1: n = 9, MOI 10: n = 9) were determined using ELISA. (C) Positive correlation is noted between PD-L1 expression on CD11b+CD14+ monocytes and PGE2 levels under live M. bovis stimulation (MOI of 10, n = 11). (D) PD-L1 expression on CD11b+CD14+ monocytes (untreated: n = 13, heat-killed M. bovis: n = 13) were determined using flow cytometry. (E) PGE2 levels in culture supernatants (untreated: n = 13, heat-killed M. bovis: n = 13) were determined using ELISA. (F) Positive correlation is noted between PD-L1 expression on CD11b+CD14+ monocytes and PGE2 levels under heat-killed M. bovis stimulation (n = 13). (G) PD-L1 expression on CD11b+CD14+ monocytes cultured in 24-h culture supernatant of PBMCs with or without live M. bovis (untreated: n = 9, MOI 0.1: n = 9, MOI 1: n = 9, MOI 10: n = 9) were analyzed by flow cytometry. (H) PBMCs from uninfected cattle were incubated with PGE2, and PD-L1 expression on CD11b+CD14+ monocytes (DMSO: n = 6, PGE2: n = 6) was determined using flow cytometry. The bars are the median of the values (A,B,D,E,G,H). Statistical significance was determined by the Steel–Dwass test (A,B,G) or Mann–Whitney U-test (D,E,H). Correlation statistics were analyzed using Spearman's correlation analysis (C,F). *P < 0.05.
To identify the major cell type that produced PGE2 in the PBMC culture, PGE2 productions from isolated CD14+ and CD14− cells cultured with live M. bovis were measured using ELISA. PGE2 production from CD14+ cells was significantly increased when compared with that from CD14− cells (Figure 2A). In the CD14+ fraction, PD-L1 expression on CD11b+CD14+ monocytes cultured with live M. bovis was increased (Figure 2B). These results represent that CD14+ cells could be a major cell type producing PGE2 against M. bovis.
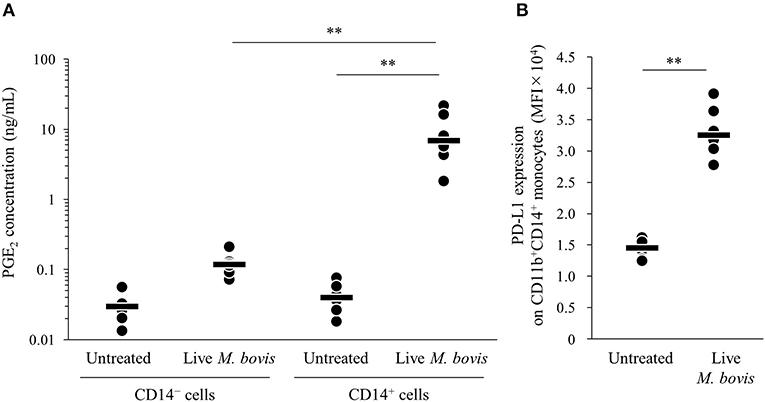
Figure 2. PGE2 production from CD14+ cells induced by M. bovis. CD14+ cells or CD14− cells from uninfected cattle were incubated with live M. bovis (MOI of 10) for 24 h. (A) PGE2 production was determined by ELISA (CD14− cells untreated: n = 6, CD14− cells Live M. bovis: n = 6, CD14+ cells untreated: n = 6, CD14+ cells M. bovis: n = 6). (B) PD-L1 expression on CD14+ cells (untreated: n = 6, live M. bovis: n = 6) was determined by flow cytometry. The bars are the median of the values (A,B). Statistical significance was determined by the Steel–Dwass test (A) or Mann–Whitney U-test (B). **P < 0.01.
Induction of PGE2 via Toll-Like Receptor (TLR) 2
Because PGE2 production was increased by heat-killed M. bovis, we hypothesized that pattern-recognition receptors recognized M. bovis and their signaling induced PGE2 production. Previous research showed that other mycoplasma, M. fermentans, which was first isolated from the human urogenital tract (25), induced PGE2 production via TLR2 in human monocytes (26, 27). Thus, to test whether PGE2 production and PD-L1 upregulation were induced in bovine PBMCs under TLR2 stimulation, PBMCs from uninfected cattle were incubated with a TLR2/6 agonist (FSL-1, 28), which is a synthetic lipoprotein of M. salivarium, which been implicated in eye and ear disorders, oral infection, septic arthritis, and periodontal disease in human (28), and PGE2 production in culture supernatants and PD-L1 expression on monocytes were analyzed. FSL-1 upregulated PGE2 production from PBMCs and PD-L1 expression on CD11b+CD14+ monocytes (Figures 3A,B). Consistent with the results of M. bovis stimulation, PGE2 production was positively correlated with PD-L1 expression on CD11b+CD14+ monocytes under FSL-1 stimulation (Figure 3C). Inhibition of TLR2/4 signaling by a selective antagonist (sparstolonin B; SsnB) decreased PGE2 production induced by FSL-1 and M. bovis stimulation (Figure 3D). CD14+ cells are considered as one of the major cell types expressing TLR2 (29). These results suggest that M. bovis could induce PGE2 production from monocytes presumably via TLR2.
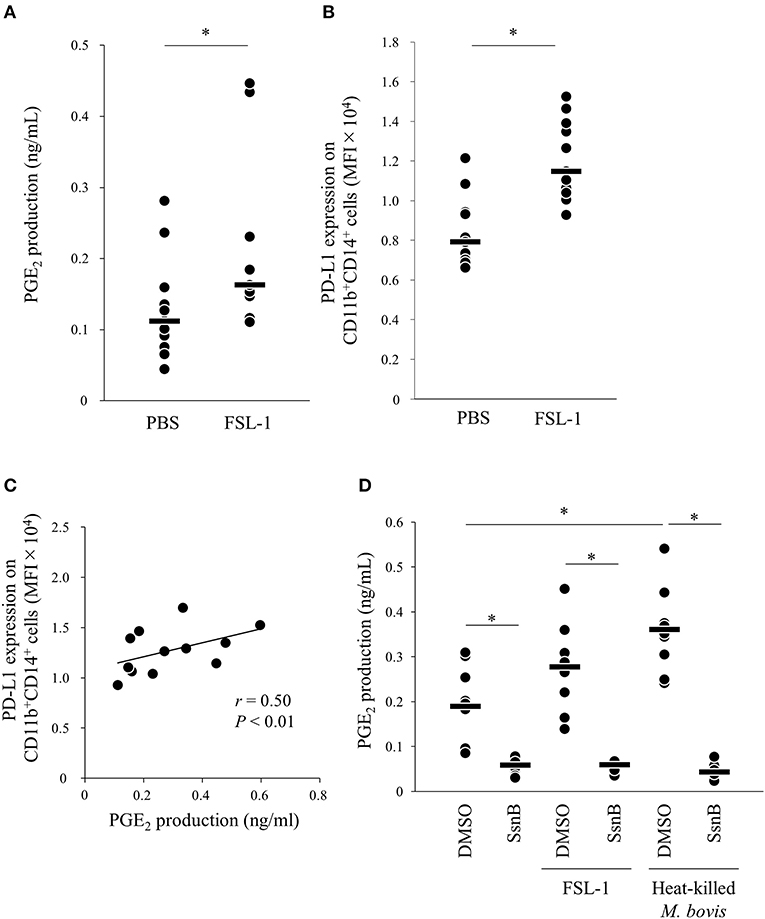
Figure 3. TLR2 signaling upregulates PD-L1 and PGE2 expression. PBMCs from uninfected cattle were incubated with FSL-1 (100 ng/ml). (A) PGE2 production was determined by ELISA (PBS: n = 11, FSL-1: n = 11). (B) PD-L1 expression on CD14+ cells (PBS: n = 11, FSL-1: n = 11) was determined by flow cytometry. (C) Positive correlation is noted between PD-L1 expression on CD11b+CD14+ monocytes and PGE2 levels under FSL-1 stimulation (n = 13). (D) PBMCs from uninfected cattle were incubated with FSL-1 (100 ng/ml) or heat-killed M. bovis (1.5 ng/ml) under inhibition of TLR signaling by SsnB and PGE2 production was determined by ELISA (DMSO: n = 9, SsnB: n = 9, DMSO and FSL-1: n = 9, SsnB and FSL-1: n = 9, DMSO and M. bovis: n = 9, SsnB and M. bovis: n = 9). The bars are the median of the values (A,B,D). Statistical significance was determined by the Mann–Whitney U-test (A,B) or the Steel–Dwass test (D). Correlation statistics were analyzed using Spearman's correlation analysis (C). *P < 0.05.
Upregulation of PGE2 Production in M. bovis-Infected Cattle
We found that M. bovis induced PGE2 production and upregulated PD-L1 expression in vitro. To confirm that PGE2 is associated with immune dysfunction in bovine mycoplasmosis, we analyzed serum PGE2 levels in cattle naturally infected with M. bovis, which were diagnosed in the previous studies (21, 30). Serum PGE2 levels were significantly increased in M. bovis-infected cattle when compared with that in uninfected cattle (Figure 4A). No differences in serum PGE2 levels were observed among the mycoplasmosis types with different clinical symptoms in the M. bovis-infected cattle (Figure 4B). Because PBMC samples were not available for the previous clinical samples used in Figures 4A,B, we collected blood samples of M. bovis-infected cattle and analyzed plasma levels of PGE2 and other immunological factors (Figures 4C–E). Interestingly, plasma levels of PGE2 were positively correlated with the proportions of PD-L1+CD11b+CD14+ cells in infected cattle (Figure 4C). To investigate the relationship between PGE2 and immune response, plasma IFN-γ levels and IFN-γ production against heat-killed M. bovis from PBMCs of infected cattle were analyzed using ELISA and their correlation with plasma PGE2 levels. Plasma IFN-γ levels and IFN-γ production against M. bovis were negatively correlated with plasma PGE2 levels in infected cattle (Figures 4D,E). These results suggest that PGE2 is associated with PD-L1 expression and immune dysfunction in M. bovis-infected cattle.
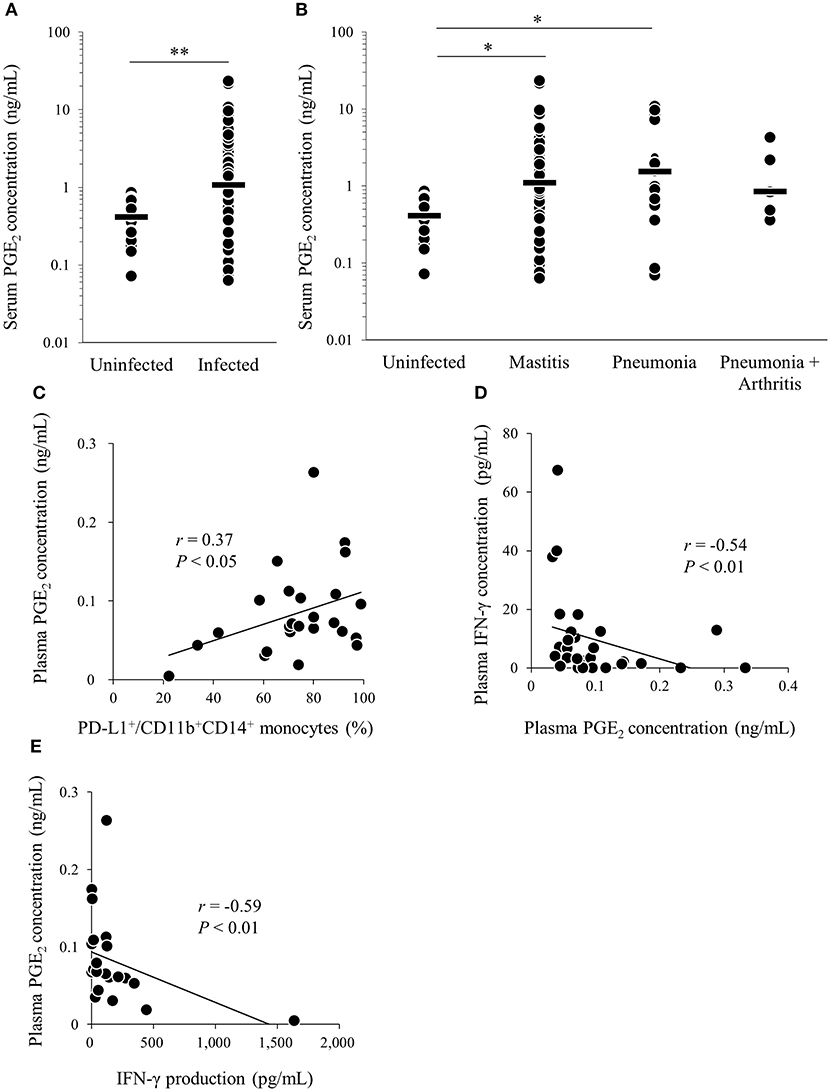
Figure 4. Analysis of PGE2 in cattle infected with M. bovis. (A,B) Serum PGE2 levels in M. bovis-infected cattle (n = 89; Mastitis: n = 62, Pneumonia: n = 21, Pneumonia with arthritis: n = 6) and uninfected cattle (n = 18) was determined by ELISA. (C–E) Correlation between plasma PGE2 levels in M. bovis-infected cattle with arthritis (n = 13), otitis (n = 10), pneumonia (n = 5), and other factors. (C) Correlation between plasma PGE2 levels and plasma IFN-γ levels (n = 29). (D) Correlation between the plasma levels of PGE2 and the proportions of PD-L1+ monocytes (n = 25). (E) Correlation between plasma PGE2 levels and IFN-γ production from PBMCs against heat-killed M. bovis (n = 25). The bars are the median of the values (A,B). Statistical significance was determined by the Mann–Whitney U-test (A) or the Steel–Dwass test (B). Correlation statistics were analyzed using Spearman's correlation analysis (C–E). *P < 0.05, **P < 0.01.
Expressions of PD-L1 and PGE2 Associated With Pneumonic Lung Lesions in M. bovis-Infected Cattle
To determine the expressions of PD-L1 and PGE2 associated with lung lesions in infected cattle, immunohistochemical analysis was conducted on pneumonic lung tissues from cattle with M. bovis infection. PD-L1 was expressed on macrophages and fibroblasts infiltrating the lesions (Figure 5A), and PGE2 was produced by epithelial cells and macrophages infiltrating the lesions (Figure 5C). On the other hand, the expressions of PD-L1 and PGE2 in the healthy lung of M. bovis-uninfected cattle were very weak (Figures 5B,D).
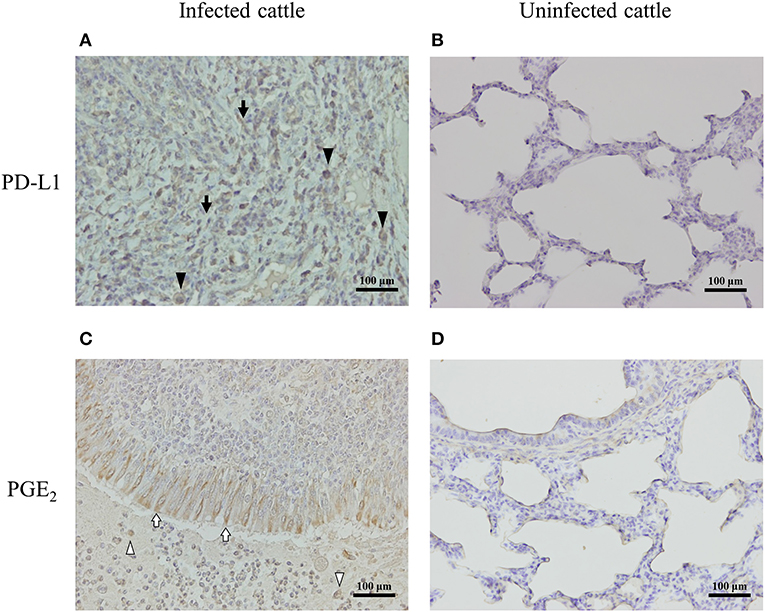
Figure 5. PD-L1 and PGE2 expression in the lung of M. bovis-infected cattle with pneumonia. Immunohistochemical staining of PD-L1 (A,B) and PGE2 (C,D) in lung tissues of cattle with or without M. bovis infection was performed using anti-bovine PD-L1 mAb (6C11-3A11) and human PGE2 polyclonal Ab (rabbit polyclonal). Black arrowheads, PD-L1 positive macrophages; black arrows, PD-L1 positive fibroblasts; white arrowheads, PGE2-positive macrophages; white arrows, PGE2-positive epithelial cells.
Combined Blockade of the PD-1/PD-L1 Pathway and PGE2 Enhanced the M. bovis IFN-γ Response
We previously reported that PD-1/PD-L1 blockade by anti-bovine PD-1/PD-L1 mAbs enhanced the M. bovis-specific IFN-γ response in vitro (11). Recent research showed that the combined blockade of the PD-1/PD-L1 pathway and PGE2 by anti-PD-L1 mAb and a COX-2 inhibitor enhanced immune responses against several pathogens (18, 19, 31). Thus, we evaluated the immune enhancement of dual blockade of the PD-1/PD-L1 pathway and PGE2 with regard to the M. bovis-specific IFN-γ response using anti-PD-L1 mAb and COX-2 inhibitor, meloxicam. As shown in Figure S2, heat-killed M. bovis stimulation induced the proliferative response of CD4+ and CD8+ T cells in the cultivated PBMCs isolated from M. bovis-infected cattle. IFN-γ production from PBMCs stimulated with T-cell stimulators (combination of anti-CD3 mAb and anti-CD28 mAb; Figure 6A) or heat-killed M. bovis antigen (Figure 6B) was significantly increased by anti-PD-L1 mAb or the combination of meloxicam and anti-PD-L1 mAb when compared with that in negative controls (combination of DMSO and rat IgG). These results obtained by combining meloxicam and anti-PD-L1 mAb support their potentials as novel treatments for M. bovis infection.
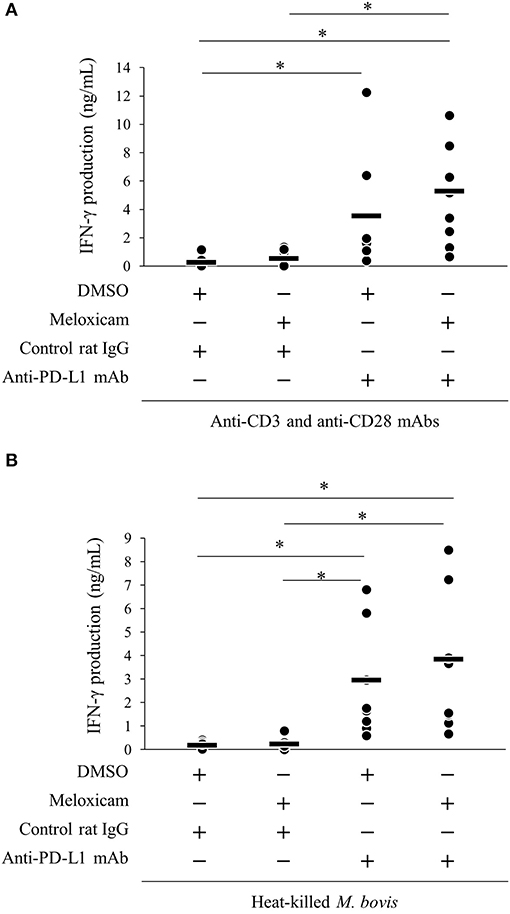
Figure 6. Activation of IFN-γ responses by the combination of meloxicam and anti-PD-L1 Ab. PBMCs from cattle infected with M. bovis (n = 7) were incubated with meloxicam and anti-PD-L1 mAb in the presence of anti-bovine CD3 and CD28 mAbs (A) or heat-killed M. bovis (B). IFN-γ production in culture supernatants was determined by ELISA. The bars are the median of the values (A,B). Statistical significance was determined by the Steel–Dwass test (A,B). *P < 0.05.
Discussion
Bovine mycoplasmosis, especially associated with M. bovis infection, is spreading globally, including in North America, Europe, and Japan (32–36). M. bovis is a highly contagious pathogen, and there are no effective vaccines owing to its immunosuppressive effects. Although antibiotics are potentially effective against M. bovis, strains resistant to antibiotics have recently been emerging and spreading (37, 38). Therefore, a novel strategy for the control of M. bovis infection is required.
Our previous study showed that PD-1-expressing T cells and PD-L1-expressing monocytes were increased in M. bovis-infected cattle and that PD-1 and PD-L1 were closely associated with a decreased IFN-γ response against M. bovis (11). On the other hand, the mechanism of PD-L1 upregulation during M. bovis infection remains unknown. In this study, we revealed that PGE2 produced by monocytes was one of the inducers of PD-L1 expression. In addition, we demonstrated that in vitro blockade of the PD-1/PD-L1 pathway and PGE2 by anti-bovine PD-L1 Ab and a COX-2 inhibitor enhanced the IFN-γ response against M. bovis. These results suggest that the PD-1/PD-L1 pathway and PGE2 have therapeutic potentials for the control of M. bovis infection.
PGE2 is typically known as a pro- and anti-inflammatory mediator and its synthesis is induced by COX-2 and PGE synthases (12, 13). Previous research in cancer showed relationships between PGE2 and the PD-1/PD-L1 pathway (16, 17). In human melanoma cells, COX-2 was positively correlated with PD-L1 expression (16). In a mouse model of bladder tumor, tumor-infiltrating PD-L1-expressing cells showed high levels of the PGE2-forming enzymes, namely, microsomal PGE2 synthase 1 (mPGES1) and COX-2 (17). Therefore, PGE2 was recently implicated in the induction of PD-L1 expression. Our previous study showed that PGE2 upregulated PD-L1 expression on bovine PBMCs and was associated with the progression of Johne's disease, which is a chronic bacterial infection of cattle caused by MAP (18). Then, we hypothesized that this immune dysfunction via PGE2-PD-L1 axis is commonly involved in bovine chronic infections. Therefore, this study focused on M. bovis infection, which is known to show immune dysfunction induced by exhausted T cell during the disease progression (11). In this study, we demonstrated that M. bovis induced PGE2 production from monocytes in line with the upregulation of PD-L1 expression on monocytes (Figures 1A–F). Additionally, we showed that the levels of plasma PGE2 and proportions of circulating PD-L1+ monocytes were positively correlated (Figure 4C). These results indicate that PGE2 could be associated with PD-L1 expression in M. bovis infection. Several reports showed that PGE2 activates STAT3 signaling (18, 39). In tumor studies, STAT3 regulates PD-L1 expression transcriptionally by binding to its promoter (40, 41). These results indicate that PGE2 induced by M. bovis could upregulate PD-L1 expression on monocytes via STAT3 signaling. On the other hand, many researchers have reported that PD-L1 expression can be induced by other factors, such as IFN-γ and TNF-α production (41, 42). A previous report showed that M. bovis induced IFN-γ and TNF-α production from PBMCs of uninfected cattle (30). Further studies on other cytokines are warranted to confirm the mechanisms of PD-L1 expression during M. bovis infection.
TLRs are pattern-recognition receptors that play important roles in early innate recognition and host immune responses against several pathogens (43, 44). TLR2 plays the most important role in Mycoplasma infection. TLR2 recognizes lipoproteins from Mycoplasma spp. including M. bovis (25, 26, 45–48). TLR2/MyD88 signaling induces PGE2 production by COX-2 transcription via NF-κB (49), during not only Mycoplasma infection (27, 50, 51) but also other bacterial infection such as Mycobacterium bovis (52), Mycobacterium leprae (53), and Staphylococcus aureus (54). TLR2 expression has been demonstrated in several immune cells, such as lymphocytes, monocytes (28), and some types of non-hematopoietic cells, such as epithelial cells (55). A previous study in humans showed that CD14+ monocytes expressed the highest level of TLR2 in blood (56). Indeed, the current study revealed that CD14+ monocytes were a major cell type producing PGE2 in vitro response to M. bovis (Figure 2A). In addition, immunohistochemical analysis demonstrated that macrophages infiltrating lung lesions and epithelial cells in lung lesions from infected cattle expressed PGE2 (Figure 5B). These findings suggest that M. bovis could induce PGE2 from monocytes.
TLR4 recognize bacterial lipopolysaccharide and peptidoglycan, which are the components of bacterial cell wall (30, 43). Although Mycoplasmas do not have cell wall (57), several researchers reported the relationships between TLR4 and the inflammation during Mycoplasma infection (55, 58, 59). Shimizu et al. (60) have demonstrated that M. pneumoniae induced TNF-α production from macrophages through autophagy and TLR4. This inflammatory response could be need to cytoadherence of live bacteria. Our current study demonstrated that inactivated M. bovis and TLR2 stimulation induced PGE2 production (Figures 1D, 3A). Inhibition of TLR signaling by SsnB decreased PGE2 production induced by FSL-1 and inactivated M. bovis (Figure 3D). On the other hand, SsnB selectively blocks not only TLR2- but also TLR4-mediated inflammatory signaling (61). Taken together, although M. bovis is recognized via TLR2 and/or TLR4, the results of this study suggest that M. bovis could induce PGE2 production via mainly TLR2 signaling. However, further studies focusing on TLR4 and M. bovis must be needed to elucidate the relationship between M. bovis infection and PGE2.
PGE2 suppresses Th1 immune responses, limiting the functions of natural killer (NK) cells, CD4 T cells, and cytotoxic T cells via specific EP2 and EP4 receptors (14). After binding EP2 or EP4, PGE2 induces the expression of anti-inflammatory and immunosuppressive genes by activating the cyclic AMP (cAMP)/protein kinase A/cAMP response element binding protein pathway (14). In the current study, increased plasma PGE2 levels were strongly correlated with lower M. bovis-specific IFN-γ production (Figure 4D). Additionally, plasma levels of PGE2 and proportions of circulating PD-L1+CD11b+CD14+ monocytes were positively correlated (Figure 4C). These results indicate that decreased IFN-γ levels in bovine mycoplasmosis might be associated with the upregulation of PGE2 and PD-L1+ cells. On the other hand, PGE2 can inhibit not only adaptive immunity but also innate immunity. A previous report in humans demonstrated that PGE2 inhibited neutrophil functions, such as neutrophil extracellular trap (NET) formation, via EP2 and EP4 (58). Interestingly, in several reports, it is shown that M. bovis could inhibit NET formation and escape from neutrophil killing (59). Therefore, PGE2 in M. bovis infection might play a role as not only an inducer of PD-L1 expression but also a direct suppressor of other immune responses, such as T cell and neutrophil responses. Further studies focusing on inhibition induced by PGE2 might help elucidate the mechanisms of immune dysfunction in M. bovis infection.
Our previous studies demonstrated that blockade of the PD-1/PD-L1 pathway and/or PGE2 effectively improved antigen-specific immune reactions in bovine chronic diseases, such as bovine leukemia virus infection, Johne's disease, and anaplasmosis (18–20, 23, 24, 60, 62, 63, 69). Effective reactivation by dual blockade could be because of PGE2 function that inhibition of T-cell activation and regulates PD-L1 expression. Indeed, single blockade of the PD-1/PD-L1 pathway reactivated the M. bovis-specific IFN-γ response in vitro (Figure 6B). Therefore, we evaluated the immune activation associated with the combined treatment anti-PD-L1 mAb with a COX-2 inhibitor in vitro. The combined treatment significantly increased IFN-γ production in response to M. bovis. Although a significant difference between single and dual blockade of the PD-1/PD-L1 pathway and PGE2 was not observed, IFN-γ production tended to be increased by meloxicam and anti-PD-L1 mAb. To support the efficacy of the combined treatment, further investigation is needed. The combination of PD-1/PD-L1 blockade with COX-2 inhibition has therapeutic potential for controlling M. bovis infection.
In other bovine mycoplasmosis, contagious bovine pleuropneumonia caused by Mycoplasma mycoides subsp. mycoides biotype Small Colony (MmmSC), the number of MmmSC-specific IFN-γ-secreting CD4+ T cells was positively correlated with disease recovery (64, 65). IFN-γ promotes the activation and proliferation of T cells and macrophages, which play vital roles in host defenses in the lungs. Previous studies showed that macrophage dysfunction was induced by Mycoplasma spp., including M. bovis, and promoted persistent infection (2, 66–68). Immune reactivation by combination therapy of anti-PD-L1 mAb with COX-2 inhibitor could help in the development of potential methods for the control of M. bovis infection.
Recently, our previous studies reported the establishment of anti-bovine PD-L1 rat-bovine chimeric Ab, and a clinical study on BLV infection was conducted (19, 23). Interestingly, T cell function, which involved IFN-γ response and the proliferation of anti-BLV-specific CD4+ T cells, was restored in cattle inoculated with chimeric Ab, while BLV provirus loads were significantly reduced (19, 23). In addition, combined treatment of chimeric Ab with COX-2 inhibitor synergistically decreased BLV provirus loads, clearly demonstrating that this treatment induced antiviral activities (19). However, the in vivo effect of combined treatment of chimeric Ab with COX-2 inhibitor in M. bovis infection is still unclear and should be determined in further experiments on infected cattle to support the efficacy of this novel treatment approach for clinical application.
In conclusion, the present study found that M. bovis can induce PGE2 and upregulate PD-L1 expression on monocytes and that M. bovis-specific IFN-γ response can be upregulated by dual blockade of the PD-1/PD-L1 pathway and PGE2. Thus, PGE2 plays an important role in immune dysfunction and is related with the PD-1/PD-L1 pathway. Our findings might contribute to the development of novel strategies for manipulating M. bovis-specific T-cell responses to prevent disease progression.
Data Availability Statement
All datasets generated for this study are included in the article/Supplementary Material.
Author Contributions
SK, SGot, SM, and KO were responsible for the conception and design of the study. SGot, SK, YH, JK, TO, NM, YSa, KW, EM, AK, RU, SY, MKK, YK, KY, and MTo performed the experiments. SGot, SK, TO, YSu, SM, and KO analyzed the data. SGon, HH, MK, MTa, ET, RU, SY, MKK, YK, KY, and MTo provided intellectual input, field samples, laboratory materials, reagents, and/or analytic tools. SGot and SK wrote the manuscript. SK, TO, SM, and KO contributed to the revision of the manuscript. All authors reviewed and approved the final manuscript.
Funding
This work was supported by grants-in-aid for Scientific Research from Japan Society for the Promotion of Science (JSPS) and by grants from the Project of the NARO, Bio-oriented Technology Research Advancement Institution (Research program on development of innovative technology; number 26058 BC to SK and the special scheme project on regional developing strategy; grant 16817557 to SK). This research was also supported by Platform Project for Supporting Drug Discovery and Life Science Research [Basis for Supporting Innovative Drug Discovery and Life Science Research (BINDS)] from AMED under Grant Number JP19am0101078 to YK. The funders had no role in study design, data collection and analysis, decision to publish, or preparation of the manuscript.
Conflict of Interest
KY and MTo are employed by Fuso Pharmaceutical Industries, Ltd. SGot, SK, TO, NM, YSa, YSu, SM, and KO are authors of a patent application covering materials and techniques described in this paper (PCT/JP2018/27041).
The remaining authors declare that the research was conducted in the absence of any commercial or financial relationships that could be construed as a potential conflict of interest.
Acknowledgments
We are grateful to Dr. Hideyuki Takahashi, Dr. Yasuyuki Mori, and Dr. Tomio Ibayashi for their valuable advice and discussions. We would like to thank Enago for the English language review.
Supplementary Material
The Supplementary Material for this article can be found online at: https://www.frontiersin.org/articles/10.3389/fvets.2020.00012/full#supplementary-material
References
1. Gagea MI, Bateman KG, Shanahan RA, van Dreumel T, McEwen BJ, Carman S, et al. Naturally occurring Mycoplasma bovis-associated pneumonia and polyarthritis in feedlot beef calves. J Vet Diagn Invest. (2006) 18:29–40. doi: 10.1177/104063870601800105
2. Caswell JL, Archambault M. Mycoplasma bovis pneumonia in cattle. Anim Health Res Rev. (2007) 8:161–86. doi: 10.1017/S1466252307001351
3. Caswell JL, Bateman KG, Cai HY, Castillo-Alcala F. Mycoplasma bovis in respiratory disease of feedlot cattle. Vet Clin North Am Food Anim Pract. (2010) 26:365–79. doi: 10.1016/j.cvfa.2010.03.003
4. Fox LK. Mycoplasma mastitis: causes, transmission, and control. Vet Clin North Am Food Anim Pract. (2012) 28:225–37. doi: 10.1016/j.cvfa.2012.03.007
5. Vanden Bush TJ, Rosenbusch RF. Mycoplasma bovis induces apoptosis of bovine lymphocytes. FEMS Immunol Med Microbiol. (2002) 32:97–103. doi: 10.1111/j.1574-695X.2002.tb00540.x
6. van der Merwe J, Prysliak T, Perez-Casal J. Invasion of bovine peripheral blood mononuclear cells and erythrocytes by Mycoplasma bovis. Infect Immun. (2010) 78:4570–8. doi: 10.1128/IAI.00707-10
7. Mulongo M, Prysliak T, Scruten E, Napper S, Perez-Casal J. In vitro infection of bovine monocytes with Mycoplasma bovis delays apoptosis and suppresses production of gamma interferon and tumor necrosis factor alpha but not interleukin-10. Infect Immun. (2013) 82:62–71. doi: 10.1128/IAI.00961-13
9. Blackburn SD, Shin H, Haining WN, Zou T, Workman CJ, Polley A, et al. Coregulation of CD8+ T cell exhaustion by multiple inhibitory receptors during chronic viral infection. Nat Immunol. (2009) 10:29–37. doi: 10.1038/ni.1679
10. Khaitan A, Unutmaz D. Revisiting immune exhaustion during HIV infection. Curr HIV/AIDS Rep. (2011) 8:4–11. doi: 10.1007/s11904-010-0066-0
11. Goto S, Konnai S, Okagawa T, Nishimori A, Maekawa N, Gondaira S, et al. Increase of cells expressing PD-1 and PD-L1 and enhancement of IFN-γ production via PD-1/PD-L1 blockade in bovine mycoplasmosis. Immun Inflamm Dis. (2017) 5:355–63. doi: 10.1002/iid3.173
12. Phipps RP, Stein SH, Roper RL. A new view of prostaglandin E regulation of the immune response. Immunol Today. (1991) 12:349–52. doi: 10.1016/0167-5699(91)90064-Z
13. Morita I. Distinct functions of COX-1 and COX-2. Prostaglandins Other Lipid Mediat. (2002) 68–9:165–75. doi: 10.1016/S0090-6980(02)00029-1
14. Martínez-Colón GJ, Moore BB. Prostaglandin E2 as a regulator of immunity to pathogens. Pharmacol Ther. (2018) 185:135–46. doi: 10.1016/j.pharmthera.2017.12.008
15. Wang D, Dubois RN. Eicosanoids and cancer. Nat Rev Cancer. (2010) 10:181–93. doi: 10.1038/nrc2809
16. Botti G, Fratangelo F, Cerrone M, Liguori G, Cantile M, Anniciello AM, et al. COX-2 expression positively correlates with PD-L1 expression in human melanoma cells. J Transl Med. (2017) 15:46. doi: 10.1186/s12967-017-1150-7
17. Prima V, Kaliberova LN, Kaliberov S, Curiel DT, Kusmartsev S. COX2/mPGES1/PGE2 pathway regulates PD-L1 expression in tumor-associated macrophages and myeloid-derived suppressor cells. Proc Natl Acad Sci USA. (2017) 114:1117–22. doi: 10.1073/pnas.1612920114
18. Sajiki Y, Konnai S, Okagawa T, Nishimori A, Maekawa N, Goto S, et al. Prostaglandin E2 induction suppresses the Th1 immune responses in cattle with Johne's disease. Infect Immun. (2018) 86:e00910–17. doi: 10.1128/IAI.00910-17
19. Sajiki Y, Konnai S, Okagawa T, Nishimori A, Maekawa N, Goto S, et al. Prostaglandin E2-induced immune exhaustion and enhancement of antiviral effects by anti-PD-L1 antibody combined with COX-2 inhibitor in bovine leukemia virus infection. J Immunol. (2019) 203:1313–24. doi: 10.4049/jimmunol.1900342
20. Ikebuchi R, Konnai S, Okagawa T, Yokoyama K, Nakajima C, Suzuki Y, et al. Blockade of bovine PD-1 increases T cell function and inhibits bovine leukemia virus expression in B cells in vitro. Vet Res. (2013) 44:59 doi: 10.1186/1297-9716-44-59
21. Higuchi H, Iwano H, Kawai K, Ohta T, Obayashi T, Hirose K, et al. A simplified PCR assay for fast and easy mycoplasma mastitis screening in dairy cattle. J Vet Sci. (2011) 12:191–3. doi: 10.4142/jvs.2011.12.2.191
22. Higa Y, Uemura R, Yamazaki W, Goto S, Goto Y, Sueyoshi M. An improved loop-mediated isothermal amplification assay for the detection of Mycoplasma bovis. J Vet Med Sci. (2016) 78:1343–6. doi: 10.1292/jvms.15-0459
23. Ikebuchi R, Konnai S, Okagawa T, Yokoyama K, Nakajima C, Suzuki Y, et al. Influence of PD-L1 cross-linking on cell death in PD-L1- expressing cell lines and bovine lymphocytes. Immunology. (2014) 142:551–61. doi: 10.1111/imm.12243
24. Nishimori A, Konnai S, Okagawa T, Maekawa N, Ikebuchi R, Goto S, et al. In vitro and in vivo antivirus activity of an anti-programmed death-ligand 1 (PD-L1) rat-bovine chimeric antibody against bovine leukemia virus infection. PLoS ONE. (2017) 12:e0174916. doi: 10.1371/journal.pone.0174916
25. Ruiter M, Wentholt HM. The occurrence of a pleuropneumonia-like organism in fuso-spirillary infections of the human genital mucosa. J Invest Dermatol. (1952) 18:313–25. doi: 10.1038/jid.1952.36
26. Krausse-Opatz B, Schmidt C, Fendrich U, Bialowons A, Kaever V, Zeidler H, et al. Production of prostaglandin E2 in monocytes stimulated In vitro by Chlamydia trachomatis, Chlamydophila pneumoniae, and Mycoplasma fermentans. Microb Pathog. (2004) 37:155–61. doi: 10.1016/j.micpath.2004.06.007
27. Kandasamy P, Zarini S, Chan ED, Leslie CC, Murphy RC, Voelker DR. Pulmonary surfactant phosphatidylglycerol inhibits Mycoplasma pneumoniae-stimulated eicosanoid production from human and mouse macrophages. J Biol Chem. (2011) 286:7841–53. doi: 10.1074/jbc.M110.170241
28. Shibata K, Hasebe A, Into T, Yamada M, Watanabe T. The N-terminal lipopeptide of a 44-kDa membrane-bound lipoprotein of Mycoplasma salivarium is responsible for the expression of intercellular adhesion molecule-1 on the cell surface of normal human gingival fibroblasts. J Immunol. (2000) 165:6538–44 doi: 10.4049/jimmunol.165.11.6538
29. Razin S, Yogev D, Naot Y. Molecular biology and pathogenicity of mycoplasmas. Microbiol Mol Biol Rev. (1998) 62:1094–156. doi: 10.1128/MMBR.62.4.1094-1156.1998
30. Gondaira S, Higuchi H, Iwano H, Nakajima K, Kawai K, Hashiguchi S, et al. Cytokine mRNA profiling and the proliferative response of bovine peripheral blood mononuclear cells to Mycoplasma bovis. Vet Immunol Immunopathol. (2015) 165:45–53. doi: 10.1016/j.vetimm.2015.03.002
31. Chen JH, Perry CJ, Tsui YC, Staron MM, Parish IA, Dominguez CX, et al. Prostaglandin E2 and programmed cell death 1 signaling coordinately impair CTL function and survival during chronic viral infection. Nat Med. (2015) 21:327–34. doi: 10.1038/nm.3831
32. Janardhan KS, Hays M, Dyer N, Oberst RD, Debey BM. Mycoplasma bovis outbreak in a herd of North American bison (Bison bison). J Vet Diagn Invest. (2010) 22:797–801. doi: 10.1177/104063871002200528
33. Nicholas RA. Bovine mycoplasmosis: silent and deadly. Vet Rec. (2011) 168:459–62. doi: 10.1136/vr.d2468
34. Higuchi H, Iwano H, Gondaira S, Kawai K, Nagahata H. Prevalence of Mycoplasma species in bulk tank milk in Japan. Vet Rec. (2011) 169:442. doi: 10.1136/vr.d5331
35. Higuchi H, Gondaira S, Iwano H, Hirose K, Nakajima K, Kawai K, et al. Mycoplasma species isolated from intramammary infection of Japanese dairy cows. Vet Rec. (2013) 172:557. doi: 10.1136/vr.101228
36. Lerner U, Amram E, Ayling RD, Mikula I, Gerchman I, Harrus S, et al. Acquired resistance to the 16-membered macrolides tylosin and tilmicosin by Mycoplasma bovis. Vet Microbiol. (2014) 168:365–71. doi: 10.1016/j.vetmic.2013.11.033
37. Amram E, Mikula I, Schnee C, Ayling RD, Nicholas RA, Rosales RS, et al. 16S rRNA gene mutations associated with decreased susceptibility to tetracycline in Mycoplasma bovis. Antimicrob Agents Chemother. (2015) 59:796–802. doi: 10.1128/AAC.03876-14
38. Sulyok KM, Kreizinger Z, Wehmann E, Lysnyansky I, Bányai K, Marton S, et al. Mutations associated with decreased susceptibility to seven antimicrobial families in field and laboratory-derived Mycoplasma bovis strains. Antimicrob Agents Chemother. (2017) 61:e01983–16. doi: 10.1128/AAC.01983-16
39. Frias MA, Rebsamen MC, Gerber-Wicht C, Lang U. Prostaglandin E2 activates Stat3 in neonatal rat ventricular cardiomyocytes: a role in cardiac hypertrophy. Cardiovasc Res. (2006) 73:57–65. doi: 10.1016/j.cardiores.2006.09.016
40. Song TL, Nairismägi ML, Laurensia Y, Lim JQ, Tan J, Li ZM, et al. Oncogenic activation of the STAT3 pathway drives PD-L1 expression in natural killer/T-cell lymphoma. Blood. (2018) 132:1146–58. doi: 10.1182/blood-2018-01-829424
41. Lim SO, Li CW, Xia W, Cha JH, Chan LC, Wu Y, et al. Deubiquitination and stabilization of PD-L1 by CSN5. Cancer Cell. (2016) 30:925–39. doi: 10.1016/j.ccell.2016.10.010
42. Marzec M, Zhang Q, Goradia A, Raghunath PN, Liu X, Paessler M, et al. Oncogenic kinase NPM/ALK induces through STAT3 expression of immunosuppressive protein CD274 (PD-L1, B7-H1). Proc Natl Acad Sci USA. (2008) 105:20852–7. doi: 10.1073/pnas.0810958105
43. Kopp EB, Medzhitov R. The Toll-receptor family and control of innate immunity. Curr Opin Immunol. (1999) 11:13–8. doi: 10.1016/S0952-7915(99)80003-X
44. Brown J, Wang H, Hajishengallis GN, Martin M. TLR-signaling networks: an integration of adaptor molecules, kinases, and cross-talk. J Dent Res. (2011) 90:417–27. doi: 10.1177/0022034510381264
45. Chu HW, Jeyaseelan S, Rino JG, Voelker DR, Wexler RB, Campbell K, et al. TLR2 signaling is critical for Mycoplasma pneumoniae-induced airway mucin expression. J Immunol. (2005) 174:5713–9. doi: 10.4049/jimmunol.174.9.5713
46. Muneta Y, Uenishi H, Kikuma R, Yoshihara K, Shimoji Y, Yamamoto R, et al. Porcine TLR2 and TLR6: identification and their involvement in Mycoplasma hyopneumoniae infection. J Interferon Cytokine Res. (2003) 23:583–90. doi: 10.1089/107999003322485080
47. He J, You X, Zeng Y, Yu M, Zuo L, Wu Y. Mycoplasma genitalium-derived lipid-associated membrane proteins activate NF-kappaB through toll-like receptors 1, 2, and 6 and CD14 in a MyD88-dependent pathway. Clin Vaccine Immunol. (2009) 16:1750–7. doi: 10.1128/CVI.00281-09
48. Wang Y, Liu S, Li Y, Wang Q, Shao J, Chen Y, et al. Mycoplasma bovis-derived lipid-associated membrane proteins activate IL-1β production through the NF-κB pathway via toll-like receptor 2 and MyD88. Dev Comp Immunol. (2015) 55:111–8. doi: 10.1016/j.dci.2015.10.017
49. Echizen K, Hirose O, Maeda Y, Oshima M. Inflammation in gastric cancer: Interplay of the COX-2/prostaglandin E2 and Toll-like receptor/MyD88 pathways. Cancer Sci. (2016) 107:391–7. doi: 10.1111/cas.12901
50. Mitsunari M, Yoshida S, Shoji T, Tsukihara S, Iwabe T, Harada T, et al. Macrophage-activating lipopeptide-2 induces cyclooxygenase-2 and prostaglandin E(2) via toll-like receptor 2 in human placental trophoblast cells. J Reprod Immunol. (2006) 72:46–59. doi: 10.1016/j.jri.2006.02.003
51. Müller C, Tufa DM, Chatterjee D, Mühlradt PF, Schmidt RE, Jacobs R. The TLR-2/TLR-6 agonist macrophage-activating lipopeptide-2 augments human NK cell cytotoxicity when PGE2 production by monocytes is inhibited by a COX-2 blocker. Cancer Immunol Immunother. (2015) 64:1175–84. doi: 10.1007/s00262-015-1723-3
52. Almeida PE, Roque NR, Magalhães KG, Mattos KA, Teixeira L, Maya-Monteiro C, et al. Differential TLR2 downstream signaling regulates lipid metabolism and cytokine production triggered by Mycobacterium bovis BCG infection. Biochim Biophys Acta. (2014) 1841:97–107. doi: 10.1016/j.bbalip.2013.10.008
53. Mattos KA, D'Avila H, Rodrigues LS, Oliveira VG, Sarno EN, Atella GC, et al. Lipid droplet formation in leprosy: toll-like receptor-regulated organelles involved in eicosanoid formation and Mycobacterium leprae pathogenesis. J Leukoc Biol. (2010) 87:371–84. doi: 10.1189/jlb.0609433
54. Falahee PC, Dahmubed D, Borjesson DL, Miller LS, Simon SI. Staphylococcus aureus recognition by hematopoietic stem and progenitor cells via TLR2/MyD88/PGE2 stimulates granulopoiesis in wounds. Blood. (2013) 122:1770–8. doi: 10.1182/blood-2012-11-466268
55. Muir A, Soong G, Sokol S, Reddy B, Gomez MI, Van Heeckeren A, et al. Toll-like receptors in normal and cystic fibrosis airway epithelial cells. Am J Respir Cell Mol Biol. (2004) 30:777–83. doi: 10.1165/rcmb.2003-0329OC
56. Flo TH, Halaas O, Torp S, Ryan L, Lien E, Dybdahl B, et al. Differential expression of Toll-like receptor 2 in human cells. J Leukoc Biol. (2001) 69:474–81.
58. Domingo-Gonzalez R, Martínez-Colón GJ, Smith AJ, Smith CK, Ballinger MN, Xia M, et al. Inhibition of neutrophil extracellular trap formation after stem cell transplant by prostaglandin E2. Am J Respir Crit Care Med. (2016) 193:186–97. doi: 10.1164/rccm.201501-0161OC
59. Gondaira S, Higuchi H, Nishi K, Iwano H, Nagahata H. Mycoplasma bovis escapes bovine neutrophil extracellular traps. Vet Microbiol. (2017) 199:68–73. doi: 10.1016/j.vetmic.2016.12.022
60. Shimizu T, Kimura Y, Kida Y, Kuwano K, Tachibana M, Hashino M, et al. Cytadherence of Mycoplasma pneumoniae induces inflammatory responses through autophagy and toll-like receptor 4. Infect Immun. (2014) 82:3076–86. doi: 10.1128/IAI.01961-14
61. Liang Q, Wu Q, Jiang J, Duan J, Wang C, Smith MD, et al. Characterization of sparstolonin B, a Chinese herb-derived compound, as a selective Toll-like receptor antagonist with potent anti-inflammatory properties. J Biol Chem. (2011) 286:26470–9. doi: 10.1074/jbc.M111.227934
62. Okagawa T, Konnai S, Deringer JR, Ueti MW, Scoles GA, Murata S, et al. Cooperation of PD-1 and LAG-3 contributes to T-cell exhaustion in Anaplasma marginale-infected cattle. Infect Immun. (2016) 84:2779–90. doi: 10.1128/IAI.00278-16
63. Ikebuchi R, Konnai S, Shirai T, Sunden Y, Murata S, Onuma M, et al. Increase of cells expressing PD-L1 in bovine leukemia virus infection and enhancement of anti-viral immune responses In vitro via PD-L1 blockade. Vet Res. (2011) 42:103. doi: 10.1186/1297-9716-42-103
64. Dedieu L, Balcer-Rodrigues V, Yaya A, Hamadou B, Cisse O, Diallo M, et al. Gamma interferon-producing CD4 T-cells correlate with resistance to Mycoplasma mycoides subsp. mycoides S.C. infection in cattle. Vet Immunol Immunopathol. (2005) 107:217–33. doi: 10.1016/j.vetimm.2005.04.011
65. Dedieu L, Balcer-Rodrigues V, Cisse O, Diallo M, Niang M. Characterization of the lymph node immune response following Mycoplasma mycoides subsp. Mycoides SC infection in cattle. Vet Res. (2006) 37:579–91. doi: 10.1051/vetres:2006020
66. Lai JF, Zindl CL, Duffy LB, Atkinson TP, Jung YW, van Rooijen N, et al. Critical role of macrophages and their activation via MyD88-NFκB signaling in lung innate immunity to Mycoplasma pneumoniae. PLoS ONE. (2010) 5:e14417. doi: 10.1371/journal.pone.0014417
67. Shaw BM, Simmons WL, Dybvig K. The Vsa shield of Mycoplasma pulmonis is antiphagocytic. Infect Immun. (2012) 80:704–9. doi: 10.1128/IAI.06009-11
68. Deeney AS, Maglennon GA, Chapat L, Crussard S, Jolivet E, Rycroft AN. Mycoplasma hyopneumoniae evades phagocytic uptake by porcine alveolar macrophages in vitro. Vet Res. (2019) 50:51. doi: 10.1186/s13567-019-0667-6
Keywords: immunoinhibitory molecules, PD-1, PD-L1, T-cell exhaustion, prostaglandin E2, immune dysfunction, Mycoplasma bovis, cattle
Citation: Goto S, Konnai S, Hirano Y, Kohara J, Okagawa T, Maekawa N, Sajiki Y, Watari K, Minato E, Kobayashi A, Gondaira S, Higuchi H, Koiwa M, Tajima M, Taguchi E, Uemura R, Yamada S, Kaneko MK, Kato Y, Yamamoto K, Toda M, Suzuki Y, Murata S and Ohashi K (2020) Upregulation of PD-L1 Expression by Prostaglandin E2 and the Enhancement of IFN-γ by Anti-PD-L1 Antibody Combined With a COX-2 Inhibitor in Mycoplasma bovis Infection. Front. Vet. Sci. 7:12. doi: 10.3389/fvets.2020.00012
Received: 09 October 2019; Accepted: 08 January 2020;
Published: 20 February 2020.
Edited by:
Paul M. Coussens, Michigan State University, United StatesReviewed by:
Jayne Hope, University of Edinburgh, United KingdomRobin James Flynn, University of Liverpool, United Kingdom
Copyright © 2020 Goto, Konnai, Hirano, Kohara, Okagawa, Maekawa, Sajiki, Watari, Minato, Kobayashi, Gondaira, Higuchi, Koiwa, Tajima, Taguchi, Uemura, Yamada, Kaneko, Kato, Yamamoto, Toda, Suzuki, Murata and Ohashi. This is an open-access article distributed under the terms of the Creative Commons Attribution License (CC BY). The use, distribution or reproduction in other forums is permitted, provided the original author(s) and the copyright owner(s) are credited and that the original publication in this journal is cited, in accordance with accepted academic practice. No use, distribution or reproduction is permitted which does not comply with these terms.
*Correspondence: Satoru Konnai, konnai@vetmed.hokudai.ac.jp