- 1Small Animals Surgery, Tierspital, Zurich, Switzerland
- 2Department of Health Sciences and Technology, Institute for Biomechanics, ETH Zurich, Zurich, Switzerland
- 3Spine Center, Schön Klinik München Harlaching, Munich, Germany
- 4Academic Teaching Hospital and Spine Research Institute, Paracelsus Private Medical University Salzburg, Salzburg, Austria
- 5Department of Health Sciences, University of Potsdam, Potsdam, Germany
- 6Missouri Orthopaedic Institute, University of Missouri, Columbia, SC, United States
Serious knee pain and related disability have an annual prevalence of approximately 25% on those over the age of 55 years. As curative treatments for the common knee problems are not available to date, knee pathologies typically progress and often lead to osteoarthritis (OA). While the roles that the meniscus plays in knee biomechanics are well characterized, biological mechanisms underlying meniscus pathophysiology and roles in knee pain and OA progression are not fully clear. Experimental treatments for knee disorders that are successful in animal models often produce unsatisfactory results in humans due to species differences or the inability to fully replicate disease progression in experimental animals. The use of animals with spontaneous knee pathologies, such as dogs, can significantly help addressing this issue. As microscopic and macroscopic anatomy of the canine and human menisci are similar, spontaneous meniscal pathologies in canine patients are thought to be highly relevant for translational medicine. However, it is not clear whether the biomolecular mechanisms of pain, degradation of extracellular matrix, and inflammatory responses are species dependent. The aims of this review are (1) to provide an overview of the anatomy, physiology, and pathology of the human and canine meniscus, (2) to compare the known signaling pathways involved in spontaneous meniscus pathology between both species, and (3) to assess the relevance of dogs with spontaneous meniscal pathology as a translational model. Understanding these mechanisms in human and canine meniscus can help to advance diagnostic and therapeutic strategies for painful knee disorders and improve clinical decision making.
Introduction
The knee is one of the joints most commonly affected by osteoarthritis (OA), usually secondary to anterior cruciate ligament (ACL), meniscal injuries, trauma, or overuse (1). Approximately 25% of people over the age of 55 have suffered from a significant episode of knee pain in the past year of their life, with half of these people reporting associated disability (1). As curative treatments for these common knee problems are not available to date, the diseases typically progresses, often leading to OA and the associated chronic pain and disability. With aging of the population and increasing obesity, the prevalence and socioeconomic impact of painful knee pathologies are expected to rise (1, 2).
The knee joint is composed of the fibrous joint capsule, the synovial membrane, the joint cavity with synovial fluid (SF), menisci, ligaments, and bones lined with articular cartilage. The overall function of the knee joint is to allow motion and provide stability for load transfer between femur and tibia. Anatomy and function of the knee undergo age-related changes caused by shifts in cell density and phenotypes and molecular, structural, and mechanical alterations of the extracellular matrix (ECM), influencing the load distribution and joint kinematics. Damage to any of the knee structures can lead to loss of biological and biomechanical homeostasis and contribute to progressive degeneration of the whole joint. Despite the clinical importance of pain associated with degenerative joint disease, it is still unclear which structures of the knee are specifically painful and to what extent. While the meniscus plays a crucial role in biomechanics of the knee, its role in pain generation is not fully clear. Explaining the biological mechanisms underlying meniscus pathophysiology can therefore help to advance therapeutic strategies against progression of painful knee disorders.
Limitations to effective clinical application of translational joint research can be caused not only by species-related differences in metabolism, biology, and biomechanics but also by the inability to fully replicate the course of spontaneous pathology in animals. Pre-existing pathophysiological mechanisms underlying progressive knee degeneration in aging humans are commonly not active in experimental animals (3), causing overestimation of therapeutic effects (3, 4). As an example, human knees often have been under the influence of altered mechanical loading, catabolism, and inflammation for a long time before becoming symptomatic. Therefore, it can be expected that the healing responses of the symptomatic human knee are diminished when compared with joints of experimental animals that are younger and/or treated at or near the time of insult. The use of animal models with spontaneous joint pathology can significantly help address this limitation to effective translational joint research.
Both microscopic and macroscopic anatomy of the canine and human menisci are similar, however, biomolecular differences underlying meniscus pathologies in human and dog, have not yet been fully elucidated. Therefore, the aims of this review are (1) to provide an overview of the anatomy, physiology, and pathology of the human and canine meniscus, (2) to compare the known signaling pathways involved in spontaneous meniscus pathology between both species, and (3) to assess the relevance of dogs with spontaneous meniscal pathology as effective translational models for clinical application to human meniscal pathology.
Human Meniscus
Anatomy and Physiology
The medial and lateral menisci are semicircular fibrocartilages anchored to the tibia and femur by meniscal ligaments. Although the gross anatomy of human and canine menisci is similar, small differences can be found in the meniscal attachments to femur and tibia (5).
The contributions of the menisci to knee joint function include absorption and distribution of mechanical loads, congruity and stabilization, lubrication, nutrition, and mechano- and proprioception (6). The meniscal tissue is composed of water (±70%), collagens (±20%), glycosaminoglycans (GAG) (±1%), non-collagenous proteins (1%), and cells (7, 8). Collagen type I is a major component of the ECM, which is produced by the cells. Collagen type I is distributed throughout the whole meniscus, from the peripheral to inner area, organized in circumferential fibers to culminate in the anterior (cranial) and posterior (caudal) menisco-tibial ligaments (7, 9, 10). Collagen type II is found mainly in the inner avascular zone, where it shows an organized network of circumferential and radial fibers (7, 10). An organized network of proteoglycans (aggrecan, decorin, and biglycan) is also predominantly located in inner zone (7, 11). Adhesion glycoproteins such as fibronectin and thrombospondin connect cells with the surrounding ECM (8). No major differences in composition are found between human and canine meniscus (4).
Cells found in human and canine menisci are classified as chondrocytes and fibrochondrocytes, distributed according to the ECM they produce: chondrocyte-like cells are located in inner (avascular) part of the menisci, particularly in the anterior and posterior horns, whereas fibrochondrocytes are found in the outer (vascular) layers (7, 8). Meniscal cells are involved in responses to mechanical loading, osmolarity, and pressure (12). Mechanical loading is crucial for the health and function of the meniscus, as it can drive either anti-inflammatory or pro-inflammatory responses as well as influence the balance of ECM turnover toward anabolism or catabolism, depending on magnitude, frequency, and duration, in both humans and dogs (12, 13). Mechanisms involved in mechanosensing and osmosensing of meniscal fibrochondrocytes are not fully understood. Nevertheless, cell substrate-mediated responses, mainly driven by calcium, have been identified in vitro (14–16). Meniscal cells also contribute to the joint lubrication by secreting mucoproteins into the SF (17), produced by penetration of a plasma filtrate from vascularized synovial membrane. Apart from plasma proteins, SF also contains molecules secreted by articular chondrocytes and synovial cells, including hyaluronan and lubricin, respectively. The function of the SF is to facilitate joint movement, absorb mechanical loads, and provide transport medium for exchange of gases, nutrients, and waste products. Importantly, the SF in injured and degenerative joints contains pro-inflammatory cytokines, catabolic enzymes, and pain mediators, spreading them to non-affected parts of the joint and promoting disease progression and pain (17).
In both human and dog, approximately 25% of the meniscus (outer part) is vascularized, while the inner part receives nutrition by diffusion from the SF (18, 19). Therefore, the outer zone has higher capacity to heal spontaneously, while inner meniscus has much more limited healing capacity. Healing mechanisms in the vascularized zone include cell-mediated tissue repair (by stem cells, neutrophils, macrophages, and lymphocytes), tissue-remodeling molecules, oxygen, and nutrients. As the inner meniscus is not connected to the bloodstream, inner meniscal tears have little capacity to heal, typically resulting in maceration and degeneration of the affected meniscal tissue (7). Innervation of the meniscus coincides with the vascularization pattern, as most nerves are associated with vessels. The outer one-third of the meniscus and the anterior and posterior horns are innervated by nerves providing proprioceptive and sensory function (6). Mechanoreceptors are located at the horns and attachment structures, whereas free nerve endings are found throughout the meniscus, except for the inner one-third of the meniscal body (20).
Pathology
Meniscal lesions represent the most common intra-articular knee injury and are the most frequent cause for knee surgery in humans (7, 21). The younger population typically suffers from traumatic meniscal injuries (e.g., due to sports) with or without associated ligament ruptures, while older people are affected by degenerative tears that can be symptomatic or asymptomatic (8, 22). Importantly, meniscal damage is associated with main painful knee pathologies both in human and dog (6, 23, 24). Common human knee pathologies are described below. Although less is known about underlying pathophysiological mechanisms on canine stifle disorders, these mechanisms are thought to be similar.
Aging of the Knee Joint
The prevalence of knee pain increases with age (1). The normal aging process is caused by a progressive loss of cell function and ability to effectively maintain the ECM. Therefore, age-related changes in menisci and cartilage of both human and dog arise from natural senescence process (25, 26). The effects of aging on meniscus in human include loss of collagen fiber organization, decreased cell function, and reduced cell density, loss of water content, and associated detrimental changes to its material properties (25, 27). Anisotropies in the ECM give rise to variations in the distribution of local stress and strain and alter cell and ECM responses to mechanical loading (27, 28). Structural disorganization of the ECM can progress to meniscal lesions (29). Physiological loading has beneficial effects on the aging meniscus by promoting transport of nutrients through the inner avascular part. However, the meniscal repair capacity is reduced with age such that even physiological loading can result in pathology (26, 30).
Cruciate Ligament Ruptures
Traumatic ligament ruptures are common in athletes and physically active people, while ligament degeneration associated with OA affects the elderly (31). Ligament ruptures alter joint kinematics and cause instability and abnormal loading, contact areas, and contact pressures. As a consequence of abnormal knee biomechanics, the meniscus is at higher risk for impingement and damage. ACL ruptures and meniscal tears eventually result in OA (23). It has been suggested that up to 80% of knees with an injured ACL demonstrate evidence of OA and meniscal damage at 5–15 years after initial injury (32). Age-related decline in ligament structure includes ECM degeneration and shifts in cell density and phenotypes both in human and dog (33, 34).
Osteoarthritis
Osteoarthritis, the most prevalent form of skeletal disease, represents a leading cause of disability in middle and old age. OA affects 40% of people above 70 years of age, being more prevalent than any other form of arthritis (35). OA is associated with a progressive loss of articular cartilage; however, the details of its etiology and pathogenesis remain unclear. OA knees are characterized by degenerative destruction of articular cartilage, meniscal tears and degeneration, and changes in ligament integrity accompanied by pain. Due to the complex nature of the knee joint, OA can be both a consequence and a cause of meniscal tears (23). A spontaneous or traumatic meniscal tear can disturb knee biomechanics, leading to OA, but knee OA can also cause a meniscal tear by abnormal loading and breakdown of meniscal structure (22). Meniscal vascular densities are increased in human patients with OA. However, the contribution of meniscal angiogenesis to symptom progression and joint pathology in OA is unclear (36).
Normal aging process and degeneration of menisci can be accelerated by several risk factors, such as gene polymorphisms. A polymorphic gene consists of an allele that causes abnormal gene expression and protein production, influencing the onset and severity of associated disorders (37). Several studies identified gene polymorphisms that can significantly influence the pathology of the knee and stifle of humans and dogs, respectively. Gene polymorphisms can alter promoter activity, influence transcription factor binding, and may result in shorter transcripts or faster product decay. Investigating polymorphisms associated with meniscus pathology could provide insight into disease mechanisms, reveal therapeutic targets, and help identify patients at high risk. However, apart from two studies that reported an association of polymorphisms in growth differentiation factor 5 (GDF5) and CD40 with meniscus injury in Chinese population (38, 39), polymorphisms associated with meniscal injury are still unknown. Targeting molecular pathways regulated by polymorphic genes may become important in the development of patient-specific treatments. Other risk factors that contribute to the progression of meniscal pathology include systemic inflammation, bacterial infection, overloading (e.g., due to overweight), and injury (23, 40, 41).
Translational Models for Meniscal Pathology
Animal models are crucial in facilitating translation of basic research findings to practical clinical applications that ultimately enhance human health and quality of life. Biological mechanisms of meniscal damage and repair have been tested both in small and large animal models (7, 42, 43). In general, large animal models appear more suitable for meniscal biological studies than small animals. Large animals such as sheep or dogs possess a joint anatomy and tissue composition close to humans. Their tissue characteristics include comparable molecular diffusion distances and biomechanical behavior, making large animals more relevant for translational therapeutic testing. As an example, sheep have meniscal dimensions, vascularization pattern, cellularity, and collagen structure similar to humans (7, 9, 44). However, large animal models require higher costs, especially if studies are focused on long-term effects of experimental treatments. Small animals such as rodents and rabbits can be successfully used for uncovering biological mechanisms underlying tissue functions as well as for pilot therapeutic studies. However, their distinct anatomy and physiology make them less relevant for translational therapeutic testing. As an example, rabbit menisci contain significantly more cells, more expansive vascularization pattern, and different collagen structure compared with humans (7, 9, 45). Therefore, the choice of animal to be used to test a given hypothesis depends on several factors including study design, length, budget, outcome measures, stage of development, and intended application (46, 47). Detailed reviews on translational animal models for degenerative disorders of the knee are available (4, 46).
To date, most studies aimed at translational meniscal research have focused on simulating the effects of partial or total meniscectomies in animal models, with the goal of studying the roles of the deficient meniscus in the development of OA and methods to mitigate associated joint pathology (7). However, results obtained from these studies cannot fully assess the effects of degenerative or traumatic meniscal tears in the development and progression of OA. Animal models where meniscal injury is induced by transection of the cranial cruciate ligament (CCL), equivalent to ACL, allow for study of mechanical and degenerative meniscal pathology associated with instability, but are also limited in clinical applicability based on untreated instability and the inability to mimic age-related degenerative changes in the knee.
The most frequently used large animal model for knee pathologies is the dog (47). The canine stifle has similar anatomical and physiological characteristics to the human knee (4, 48, 49). The canine meniscus has comparable vascularization pattern, cellularity, collagen structure, and similar permeability (7, 45). However, dog’s menisci differ from human menisci in biomechanical properties such as aggregate modulus, shear modulus and anatomy, indicating that caution is needed when choosing the dog as a model for biomechanical studies (5, 45). Two types of canine meniscal pathology can be distinguished: experimentally induced and spontaneous.
Experimentally Induced Meniscal Pathology in Dog
Experimentally induced meniscal pathology is created using several canine models designed to study meniscal deficiency and associated OA, as well as meniscal repair and regeneration treatment strategies. In these models, summarized in Table 1, meniscal pathology is either surgically created (meniscectomy, meniscal release) or indirectly induced with CCL transection. As outlined above, each of these models have limitations with respect to clinical applicability. For this reason, accurate modeling of meniscal degeneration may require study of spontaneously occurring meniscal pathology (47).
Spontaneously Occurring Meniscal Pathology in Dog
Although experimentally induced pathologies have certain advantages, such as reduced disease variability among subjects, spontaneous meniscal pathologies in canine patients are highly relevant for translational medicine, especially when analyzing degenerative processes. Spontaneously occurring meniscal pathology can be well studied in dogs, as they are prone to CCL disease with concurrent or subsequent meniscal tears and secondary OA (6, 49) (Figures 1 and 2). Canine CCL ruptures occur most commonly due to a chronic degenerative weakening of the ligament causing premature failure and joint instability (6, 55, 56). Consequently, femoro-tibial subluxation causes shearing forces on the meniscus, which ultimately fails presumably adding to pain and joint inflammation. Another common type of meniscal pathology in dogs is the degenerative tear (57). These tears can present with fraying of the free edge, centrally located horizontal tears, fringe tags, interstitial tears, radial tears, and/or extensive fibrillation of the meniscus. These meniscal lesions are almost invariably accompanied by articular cartilage lesions ranging from softening to full-thickness defects and associated subchondral bone changes. Both traumatic and degenerative meniscal tears are main causes of degenerative joint disease in canine patients (17, 23). It has been estimated that approximately 20% of dogs older than 1 year of age are affected by OA (49). Similarities between canine and human patients are not only related to disease progression but also to environmental factors (58).
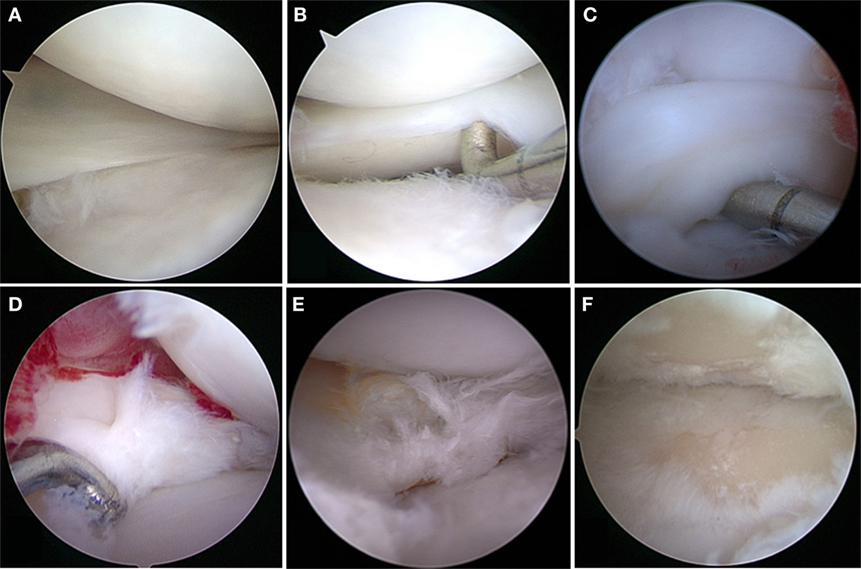
Figure 1. Meniscal tears in dogs. (A) Grossly normal meniscus with outerbridge grade 1 cartilage pathology; (B) grossly normal meniscus with outerbridge grade 2 cartilage pathology; (C) displaced bucket handle tear; (D) acute complex tears without gross evidence of degeneration; (E) complex tears with gross evidence of degeneration; (F) outerbridge grade 4 of both femoral and tibial articular surface associated with meniscal tear.
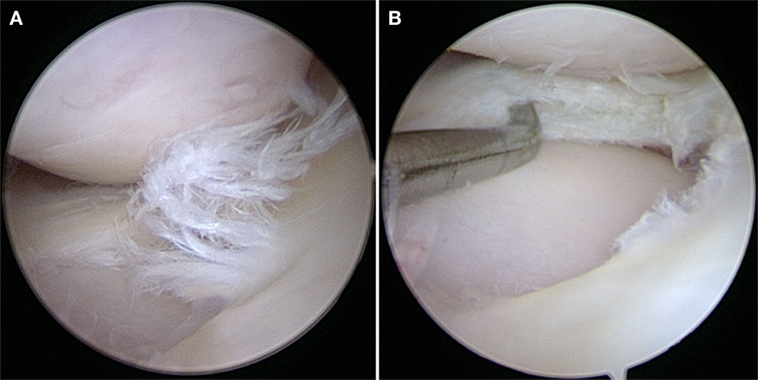
Figure 2. (A) A degenerative meniscal tear characterized by fibrillation, softening, and discoloration. (B) An horizontal cleavage tear is apparent when probing the mid-substance of the meniscus after performing a partial meniscectomy.
Dogs with spontaneously occurring meniscus disorders are useful for studying the roles of meniscus in stifle/knee pathologies and for therapeutic testing. Another important advantage of canine patients is the possibility to assess pain via direct observation focused on the degree of lameness, gait analysis, and subjective rating scales. Subjective rating scales range from single visual analog scale assessments to extensive questionnaires typically completed by the animal’s owners. The questionnaires, comparable to questionnaires used in human clinical trials, assess pain through its impact on health-related quality of life. Although quality of life and reduction of pain are important endpoints in human clinical trials, similar pain scoring systems do not exist for other large animal species (59). Apart from the scoring systems, biomarkers can be significant diagnostic tools to assess progression of the disease and the level of pain. OA biomarkers that may possibly differentiate between spontaneous CCL disease and other types of OA in canine patients have been identified (60).
Furthermore, diagnostic imaging, arthroscopic interventions, and postoperative management similar to human medical practice are routinely used in veterinary medicine (4). Depending on a study’s design and objectives—especially in the context of translation of meniscal therapies—certain similarities and differences between human and canine meniscal pathophysiology and biological mechanisms underlying progression of meniscal degeneration and related pain may be of specific importance and will hence be discussed in more detail in the following sections.
Biological Hallmarks of Meniscal Pathology in Human and Dog
Progression of knee pathology is a result of both chemical and mechanical perturbations arising from complex interactions between all tissues of the knee. As one of these tissues, the meniscus can have active roles in disease progression and pain. Typical biological hallmarks that are seen during progression of knee disorders are inflammation, ECM degradation, and oxidative damage. Molecules and pathways involved in both age-related and trauma-induced damage of the meniscus are illustrated below and summarized in Table 2 and Figure 3.
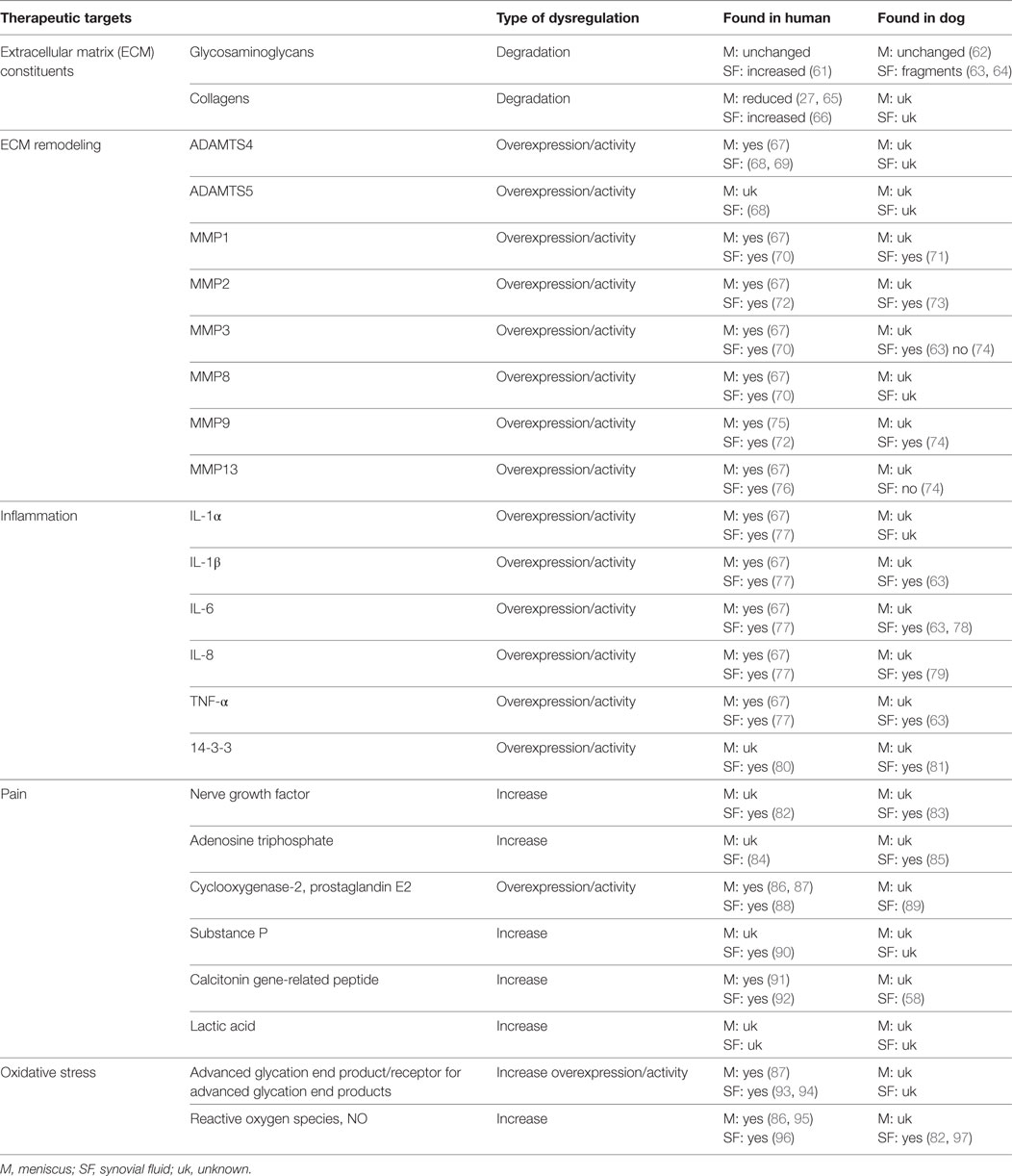
Table 2. Possible therapeutic targets in menisci and SF associated with aging and degenerative knee/stifle disorders.
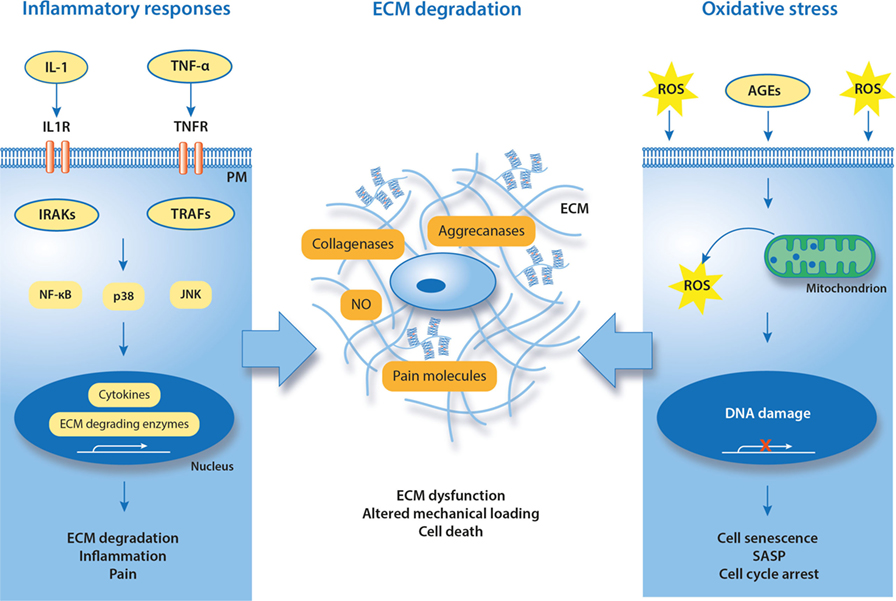
Figure 3. Known and putative molecular hallmarks of painful meniscal pathology. Suggested mechanisms in meniscal pathophysiology are inflammation and oxidative stress that together contribute to degradation of ECM. IL-1, interleukin-1; TNF-α, tumor necrosis factor alpha; IL1R, interleukin-1 receptor; TNFR, TNF receptor; TRAF, TNF receptor-associated factor; IRAK, interleukin receptor-associated kinase; ROS, reactive oxygen species; NO, nitric oxide; SASP, senescence-associated secretory phenotype; AGEs, advanced glycation end products; PM, plasma membrane; ECM, extracellular matrix.
Inflammatory Responses
It has been confirmed that inflammation is a key factor in progression of many of the human knee disorders leading to OA (98). Although articular chondrocytes and cells of the synovial membrane are main contributors to the inflammatory and catabolic reactions of the knee, cells of ligaments and menisci also overexpress pro-inflammatory and catabolic mediators. In addition, pro-inflammatory cytokines can be produced by immune cells that infiltrate joints due to trauma or age-related cartilage degeneration. Severe inflammatory responses in synovium and synovial membrane are characteristic for immune-mediated disorders (e.g., rheumatoid arthritis), but pro-inflammatory cytokines are also active in OA joints (99). In both cases, cytokines stimulate cells of neighboring tissues (chondrocytes, ligaments, and menisci), contributing to disease progression. The SF plays an important role as it spreads inflammatory and catabolic mediators within the knee joint.
Interleukin-1 (IL-1α, IL-1β) and tumor necrosis factor alpha (TNF-α) are consistently found in degenerative joints. IL-1β and TNF-α can stimulate chondrocytes to induce the expression of collagenases (MMPs) and aggrecanases (ADAMTSs), promoting degradation of the articular tissues’ ECM. At the same time, IL-1β and TNF-α activate the expression of other pro-inflammatory and pain-generating mediators (100, 101). General mechanisms for IL-1β and TNF-α action in chondrocytes is via cell surface receptors. Through its receptor IL-1R1, IL-1β can activate nuclear factor-κB (NF-κB), p38 mitogen-activated protein kinase (MAPK), and/or c-Jun N-terminal protein kinase (JNK). The activation of these signaling molecules results in transcription and translation of genes for inflammatory cytokines, ECM-degrading enzymes, and molecules that promote pain. The interaction of TNF-α with its receptor TNFR1 also can activate NF-κB and MAPK pathways, through tumor necrosis factor receptor-associated factors (67).
Meniscal cells and tissues can be stimulated by pro-inflammatory cytokines, providing a good model to study involvement of inflamed meniscus in disease progression as well as for therapeutic testing. It has been reported that primary cells derived from degenerative human menisci and stimulated with pro-inflammatory factors (e.g., IL-1β) exhibit similar inflammatory and catabolic responses as chondrocytes. These cells overexpressed ECM-degrading enzymes (MMP1, MMP3, MMP13, andADAMTS4), cytokines (IL-1α, IL-1β, and IL-6), chemokines (IL-8, CXCL1, CXCL2, and CSF1), and components of the NF-κB pathway, and underexpressed collagen (67). In human meniscal explants, IL-1α and IL-1β impair intrinsic repair capacity by increasing expression of MMPs. In addition, MMP inhibitors can reduce MMP activity and enhance repair in meniscal explants, confirming the importance of MMPs in meniscal homeostasis (100, 101). Similar data were obtained in porcine and ovine meniscal in vitro models (102). These studies all together suggest that meniscal cells are responsive to inflammatory stimulation and thus can contribute to progression of knee degeneration and pain. However, inflammatory mechanisms in canine meniscus have not yet been thoroughly investigated.
Although inflammation-related cell and tissue culture studies with canine menisci are rare, the inflammatory profile of the painful canine stifle has previously been studied in canine patients and animal models. Similar to humans, the SF of dogs with CCL transection-induced OA and naturally occurring CCL ruptures contains increased levels of IL-1β, IL-6, IL-8, TNF-α, MMP1, MMP3, MMP9, C-reactive protein, and 14-3-3-g, compared with healthy SF (58, 63, 78, 79, 81, 103, 104). Although the role of 14-3-3 proteins in joint inflammation is not yet established, it was shown in humans that these proteins control cell cycle, cell growth, differentiation, survival, apoptosis, migration, and spreading (80, 105). SF of the canine stifle also contains adenosine triphosphate (ATP), with a possible role in pain sensation (85). A correlation between the concentration of SF ATP and OA knee pain has previously been demonstrated in humans (84), but not yet in dogs. Apart from SF, elevated IL-1β, IL-6, and IL-10 were found in the synovial membrane of dogs with both OA and CCL ruptures (106). In addition, inflammation of SF is associated with depletion of proteoglycans from articular cartilage and possibly also from menisci. The activity of IL-1β, IL-6, and MMP3 in SF of canine stifle joints with naturally acquired CCL ruptures correlates with GAG content in the SF, but the source of GAG has not been determined (63, 107). Synovium and SF-related inflammatory responses in humans do not only occur in OA and ligament ruptures but also after meniscal injuries (108). However, the association between canine meniscal pathology, SF inflammation, and inflammatory responses of meniscal cells remains largely unknown.
Aside from chondrocytes, infiltrating immune cells can be another source of inflammation, although their roles in meniscal pathology and OA are unclear. Macrophages, T-cells, mast cells, B-cells, and plasma cells can be found in human and canine OA synovium, where they release inflammatory cytokines into SF (109, 110). The synovial membrane and the CCL of dogs with CCL ruptures contain significantly increased levels of antibodies to type I and type II collagen, IgG, and IgM, and increased expression of intercellular cell adhesion molecule-1 and IL-8, when compared with healthy controls (55, 103, 111). Canine OA SF contains macrophage-like cells that express significantly more collagenolytic enzymes, tartrate-resistant acid phosphatase (TRAP), and cathepsin K compared with healthy SF (104). Importantly, synovial macrophage-like cells that produce TRAP and cathepsin K are significant features of the CCL ruptures in dogs, but not in humans, suggesting species-specific differences in immune responses associated with cruciate ligament ruptures (33). Although these findings provide first evidence on the involvement of immune cells in inflammation and cartilage destruction, exact mechanisms and relative contribution to OA remain unknown in both species. Furthermore, acute immune reactions are considered beneficial for joint healing. Therefore, a complete inhibition of these reactions may not be beneficial.
In addition to the catabolic and pro-inflammatory effects, secreted inflammatory cytokines are also involved in the neuronal sensitization and transduction of pain as well as in controlling the rate of cell senescence. Human and canine joint disease progresses due to the contributing activities of pro-inflammatory and catabolic signaling pathways. Nevertheless, significance and spatiotemporal expression and activity of individual molecules still have to be elucidated.
ECM Changes
Healthy human and canine menisci contain predominantly collagen type I produced by fibrochondrocytes (27). Collagen is synthesized as pro-collagen and secreted into the ECM, where final collagen assembly takes place. Flexible collagen fibers are cross-linked by lysyl oxidases to gain additional strength. Under physiological conditions, collagen is stable (112). Menisci also contain proteoglycans (aggrecan, versican, and perlecan) in lower quantities.
Degradation and lamellar disorganization of collagen fibers occurs in human aging and contributes to likelihood of meniscal tears. Meniscal tears alter the stress distribution in the meniscus, increasing the risk for load-induced knee injuries (12). Mechanical loads also influence ECM turnover, as non-physiological loading of the meniscus can induce synthesis of catabolic and pro-inflammatory genes and reduce cell viability (12, 96). On the other hand, physiological loading promotes ECM turnover in both articular cartilage and menisci. Physiological loading regimes were shown to inhibit inflammatory and catabolic responses and activate anabolic genes in human articular chondrocytes (28) and induce production of collagens and meniscal repair in a canine model (13). It was also demonstrated that physical activity modulates the synthesis of collagens in the hyaline cartilage of aging dogs, partially preserving the cartilage structure (26). Molecular mechanisms behind responses to mechanical loading in meniscal and articular chondrocytes remain largely unexplored, due to the inhomogeneous composition and the anisotropic structure of these tissues. Intrinsic coupling of tensile, compressive, and shear stresses in vivo, as well as complex osmolarity and fluid flow environments complicate the design of experiments (12). Nevertheless, it has been shown that cells in the middle and inner human (and ovine) meniscus contain a pericellular region, with a possible role in shielding these cells from large mechanical strains and stresses. The pericellular region is formed mainly by the proteoglycan perlecan that connects cell surface molecules with ECM components (113). Interestingly, the perlecan content in menisci is reduced with age (114). However, the existence and importance of meniscal pericellular region in dogs remains unknown.
Collagen can be cleaved enzymatically by collagenases (MMPs), whereas proteoglycans are degraded by aggrecanases (ADAMTS), expressed by meniscal fibrochondrocytes and other cell types such as synovial cells and articular chondrocytes. Balanced expression of MMPs and ADAMTS ensures normal ECM turnover in a healthy joint. However, the ability of human cartilaginous tissues to maintain the ECM declines with age and with the degree of degeneration (26, 27, 107). Overexpressed collagenases and aggrecanases are released within the knee joint and transported by the SF, enhancing the local catabolic environment. Elevated MMPs were also found in the SF of canine patients with CCL ruptures and OA (63, 104), but their expression/activity in canine menisci was not yet studied. It is likely that an increased activity of canine ECM-degrading enzymes, together with age-related collagen dysfunction and mechanical overloading, can reduce exchange of solutes structure, impairing repair capacity of the meniscal ECM, and promoting degeneration. Apart from collagenases and aggrecanases, human ECM of cartilaginous tissues contains antagonists of inflammatory pathways (e.g., interleukin-1 receptor antagonist), enzyme inhibitors and activators, and other molecules diversely affecting cell fate. The importance of these molecules in canine menisci remains to be elucidated.
Cells can sense their local environment via cytoskeleton and membrane receptors (115). Ion channels on cell surfaces can be activated mechanically, by deformations of the ECM that pull or push on these receptors, or chemically, by changes in concentrations and activities of their ligands. Opening ion channels allows flux of ions and molecules through these channels, regulating various functions, such as cell adhesion, spreading, migration, proliferation, and differentiation. As an example, it has been shown that fluid flow-induced shear stress causes an increase in intracellular Ca2+ and GAG production in isolated rabbit meniscal cells (116), but the exact receptors responsible for sensing these signals and transporting the Ca2+ into the meniscal cells have yet to be identified (12). Candidates could be a family of transient receptor potential (TRP) channels that can be activated by various mechanical and chemical stimuli. TRP activation is known to increase intracellular Ca2+ and induce various cell responses (117). For example, TRPV4 activity is important for maintaining knee joint health of mice (118), and TRPV5 expression appears to be regulated by mechanical loading in articular cartilage of rats (119). However, exact mechanisms of channel-mediated communication between chondrocytes and their microenvironment are explored neither in human nor in dog. Uncovering the role of these receptors in mechanosensing of cartilaginous tissues, including meniscus, can improve our understanding of cell–ECM communication thus facilitating novel preventative and therapeutic strategies for meniscal pathology and the development of functional tissue-engineered meniscal implants.
Oxidative Damage
Oxidative damage of macromolecules is mediated by reactive oxygen species (ROS), among which superoxide anion radical , hydroxyl radical (OH⋅), and nitric oxide (NO) are most prominent. ROS formation is a consequence of aerobic metabolism, normal aging processes, and exogenous stress. Cellular responses to ROS depend on the ROS concentration and the duration of ROS exposure. Although balanced ROS at nanomolar concentrations act as signaling molecules in various physiological functions, extensive ROS formation damages both cells and ECM (120). To ensure a properly maintained redox balance, organisms require a complex, coordinated network of antioxidants that control ROS production. Cellular and plasma antioxidants, such as superoxide dismutase, catalase, and glutathione peroxidase, counteract the formation of ROS. However, their activity can decrease with age and in degenerated tissues, as shown in human (121, 122). Additional radical scavengers, namely vitamins C and E, carotenoids, and flavonoids, can be obtained through dietary sources, but the delivery of these antioxidants into avascular tissues such as meniscus can be limited (123).
In contrast to many other cell types, the long lifetime of chondrocytes and fibrochondrocytes makes them particularly susceptible to the accumulation of oxidative damage from both aging and extrinsic stress (25). As shown in human studies, oxidative damage accelerates cell senescence and causes irreversible cell cycle arrest. The resulting senescence-associated secretory phenotype is characterized by active release of pro-inflammatory cytokines and an altered microenvironment, causing tissue fibrosis and loss of function (124–126). It has been confirmed that oxidative stress induces genomic instability and dysfunction of chondrocytes in human OA cartilage explants, suggesting that ROS are involved in the development of OA (127). Oxidative stress also activates production of nociceptive prostaglandins (PG) and causes time-dependent cell death of primary canine CCL cells. As elevated oxidative stress markers can be found in damaged human menisci (86, 95), it is likely that they also occur in degenerative and damaged canine menisci and play active roles in the development of pain. Recently, an in vitro study showed that canine chondrocytes are responsive to oxidative stress inducer hydrogen peroxide and its detrimental effects can be reduced by antioxidants (128). Furthermore, one can hypothesize that oxidative stress-induced damage positively correlates with degenerative state and/or patient’s age.
Oxidative stress also accelerates accumulation of damaging advanced glycation end products (AGEs) both inside and outside the cells. AGEs are modified proteins or lipids created by non-enzymatic glycation, due to the presence of reducing sugars (e.g., glucose). AGEs form irreversible cross-links in long-lived proteins of the ECM, preventing their repair and turnover (129). Accumulation of AGEs correlates with aging and development of knee OA both in humans and dogs (93, 130). In the canine CCL transection model, AGEs cause collagen damage and release of proteoglycans from CCL, impair its repair capacity, and reduce proteoglycan synthesis (131). Apart from the extracellular effects, AGEs can increase inflammatory responses of human articular and meniscal cells isolated from OA knees, by promoting the expression of cyclooxygenase-2 (COX-2) and the release of PG E2, MMP1, MMP3, MMP13, and TNF-α (87, 132). Intracellular accumulation of AGEs also induces apoptosis of chondrocytes via endoplasmic reticulum-related pathways (133). Deleterious effects of AGEs on the progression of human OA can be attenuated by inhibition of the receptor for advanced glycation end products (RAGE) (132, 134, 135). It has been confirmed that RAGE regulates a number of physiological and pathophysiological processes and RAGE-dependent signal transduction leads to the development of several human diseases (136). As elevated protein and mRNA expressions of RAGE were found in SF of human patients with knee OA (94), AGE–RAGE signaling appears to be a promising therapeutic target (137). No studies on AGE–RAGE in degenerative stifles of canine patients are available to assess the relevance of this therapeutic target in dogs.
It has been shown that nerve damage and resulting neuropathic pain are closely linked with the increased ROS levels (138). Degenerated cartilage from human OA patients contains elevated oxidative stress markers such as inducible nitric oxide synthase (iNOS) and nitrotyrosine, when compared with healthy samples (127, 139). Both iNOS and its metabolite NO were shown to intensify the sensation in neuropathic pain (140–142). Overproduction of NO was also found in SF isolated from dogs that underwent impact injury to the femoral condyle (97). In addition, NO can be involved in inflammatory responses that follow CCL damage in canine models (143). The importance of oxidative stress contributions to knee and stifle pain have not yet been well defined. Nevertheless, it has been shown that the MAP kinase pathways, which include ERK, JNK, and p38, can be involved (25, 143, 144). Molecules that interfere with oxidative stress, either by radical scavenging or by activation of cellular defense mechanisms, can be good therapeutic candidates to prevent ROS-related damage of musculoskeletal tissues. In preclinical tests, ROS scavengers were shown to reduce PG release and prevent oxidative stress-induced death of chondrocytes, suggesting that balancing production of ROS in damaged knees can slow down the progression of OA (145).
The Role of the SF
Molecules released within degenerative or damaged knee/stifle joint spread through the SF, stimulating nerve endings and activating pain. In addition, degenerative processes influence hyaluronic acid (HA) quantity, quality, and residence time. HA, one of the main components of the SF, can be degraded by hyaluronidases, especially HYAL2, resulting in reduced joint lubrication, as shown in human studies. Expression of HYALs increases in synovium of human OA patients, while the expression of HA synthases is reduced (146). In addition to reduced joint lubrication, fragmented HA can have pro-inflammatory effects (147). Overproduction of ROS, e.g., by mechanical overloading, also accelerates HA fragmentation and consequently reduces joint lubrication (96, 148). Importantly, efficiency of HA-based treatments is decreased by ROS produced in damaged knees (149), underscoring the importance of oxidative stress in knee pathology. Fragmented HA has been found in OA knee joints both in human and canine patients and is correlated with lower load-bearing capacity (150, 151). It is likely that oxidative stress caused by meniscal pathology also negatively influences HA in dogs.
Clinical Significance of Meniscal Pathology
Although a number of dysregulated molecular pathways have been implicated in pain both in human knees and canine stifles, pain mechanisms associated with damaged menisci are largely unknown. Two types of pain can occur: (1) neuropathic pain, resulting from neural damage, and (2) nociceptive pain, caused by a neural sensitization due to a tissue damage (152). Joint capsule, synovium, ligaments, and menisci are innervated by sensory nerves, which can be sensitized by various chemicals. Cytokines act as nociceptive triggers and also induce the expression of other potentially nociceptive molecules and direct pain mediators, such as NO and PG E (88). Biosynthesis of PG E2, mediated by COX-2, significantly increases in degenerative and injured human tissues, magnifying nociceptive and inflammatory responses (153). Neuropeptides such as substance P and nerve growth factor (NGF) are located in the sensory nerve endings, and their expression and release are associated with OA in humans (82, 154). In addition, neuropeptides can be produced by chondrocytes (155). NGF has been recognized as an important mediator of chronic pain and as a marker of inflammation (156, 157). NGF binds to two different receptors on the nociceptor neurons: (1) low-affinity 75-kDa neurotrophin receptors (p75NTR), which bind all neurotrophins, and (2) tropomyosin-related tyrosin kinase A (TrkA), which binds NGF more selectively than the other neurotrophins. NGF-activated p75NTR regulates a wide range of functions, including axonal growth, apoptosis, and myelination (158). NGF signaling through TrkA mediates the nociceptive functions of the sensory neurons through enhanced expression of substance P, calcitonin gene-related peptide, and transient receptor potential vanilloid 1 (156, 159). Therefore, NGF can be involved in both, pathological nerve ingrowth and nerve sensitization in painful human joints. As enhanced concentrations of NGF were also found in canine SF and correlated with chronic lameness, similar processes are likely involved in painful OA of both species (83). However, it is unclear whether the damaged meniscus plays an active role in NGF release.
Apart from transmission of pain signals, neuropeptides are involved in pathological neo-innervation of degenerative joints. By-products of cell metabolism, namely lactic acid, can also be noxious through dysregulation of acid sensing ion channels (160). Free radicals such as NO are involved in both inflammation and nerve damage of human cartilaginous tissues (141, 161). Despite the clinical importance of both neuropathic and nociceptive pain, mechanisms underlying pain in knee and stifle pathologies are not fully understood (3).
Current and prospective treatments for meniscal pathology are described in the Data Sheet S1 in Supplementary Material.
Conclusion
Dogs with spontaneously occurring meniscal pathology have the potential of being an excellent model for studying mechanisms of degeneration and for testing new treatment strategies for both dogs and humans. A naturally occurring model of degeneration and injury may be appropriate especially for biologic-based and tissue engineering approaches, where pre-existing inflammatory and catabolic responses largely influence treatment outcomes. Although the anatomy, physiology, and pathology of the human and canine meniscus are similar, a detailed analysis of the known signaling pathways involved in spontaneous meniscus pathology in dogs is lacking. Due to lack of knowledge, it is currently not possible to fully assess the relevance of dogs with spontaneous meniscal pathology as a translational model. To evaluate the relevance of this model, more basic research on canine meniscal biology and pathophysiology is needed in the future.
Author Contributions
All authors contributed to the manuscript, have read, and approved the final version. OK carried out the literature review, drafted the manuscript, and conceived funding. LS contributed to the manuscript draft. KW-K critically revised the manuscript. JC critically revised the manuscript. AP contributed to the manuscript draft and conceived funding.
Conflict of Interest Statement
The authors declare that the research was conducted in the absence of any commercial or financial relationships that could be construed as a potential conflict of interest.
Acknowledgments
The authors would like to thank the OPO-Stiftung (2017-0056) and Center for Applied Biotechnology and Molecular Medicine (CABMM-2017) for funding the study.
Supplementary Material
The Supplementary Material for this article can be found online at https://www.frontiersin.org/articles/10.3389/fvets.2018.00073/full#supplementary-material.
References
1. Peat G, McCarney R, Croft P. Knee pain and osteoarthritis in older adults: a review of community burden and current use of primary health care. Ann Rheum Dis (2001) 60:91–7. doi:10.1136/ard.60.2.91
2. Neogi T. The epidemiology and impact of pain in osteoarthritis. Osteoarthritis Cartilage (2013) 21:1145–53. doi:10.1016/j.joca.2013.03.018
3. Denayer T, Stöhr T, Van Roy M. Animal models in translational medicine: validation and prediction. N Horiz Transl Med (2014) 2:5–11. doi:10.1016/j.nhtm.2014.08.001
4. Gregory MH, Capito N, Kuroki K, Stoker AM, Cook JL, Sherman SL. A review of translational animal models for knee osteoarthritis. Arthritis (2012) 2012:764621. doi:10.1155/2012/764621
5. Gupte CM, Bull AM, Murray R, Amis AA. Comparative anatomy of the meniscofemoral ligament in humans and some domestic mammals. Anat Histol Embryol (2007) 36:47–52. doi:10.1111/j.1439-0264.2006.00718.x
7. Deponti D, Di Giancamillo A, Scotti C, Peretti GM, Martin I. Animal models for meniscus repair and regeneration. J Tissue Eng Regen Med (2015) 9:512–27. doi:10.1002/term.1760
8. Makris EA, Hadidi P, Athanasiou KA. The knee meniscus: structure-function, pathophysiology, current repair techniques, and prospects for regeneration. Biomaterials (2011) 32:7411–31. doi:10.1016/j.biomaterials.2011.06.037
9. Chevrier A, Nelea M, Hurtig MB, Hoemann CD, Buschmann MD. Meniscus structure in human, sheep, and rabbit for animal models of meniscus repair. J Orthop Res (2009) 27:1197–203. doi:10.1002/jor.20869
10. Kambic HE, McDevitt CA. Spatial organization of types I and II collagen in the canine meniscus. J Orthop Res (2005) 23:142–9. doi:10.1016/j.orthres.2004.06.016
11. Valiyaveettil M, Mort JS, McDevitt CA. The concentration, gene expression, and spatial distribution of aggrecan in canine articular cartilage, meniscus, and anterior and posterior cruciate ligaments: a new molecular distinction between hyaline cartilage and fibrocartilage in the knee joint. Connect Tissue Res (2005) 46:83–91. doi:10.1080/03008200590954113
12. McNulty AL, Guilak F. Mechanobiology of the meniscus. J Biomech (2015) 48:1469–78. doi:10.1016/j.jbiomech.2015.02.008
13. Dowdy PA, Miniaci A, Arnoczky SP, Fowler PJ, Boughner DR. The effect of cast immobilization on meniscal healing – an experimental-study in the dog. Am J Sport Med (1995) 23:721–8. doi:10.1177/036354659502300615
14. Nemery E, Gabriel A, Grulke S, Piret J, Toppets V, Antoine N. Mechanoreceptors in the anterior horn of the equine medial meniscus: an immunohistochemical approach. Anat Histol Embryol (2016) 45:131–9. doi:10.1111/ahe.12181
15. Han WM, Heo SJ, Driscoll TP, Boggs ME, Duncan RL, Mauck RL, et al. Impact of cellular microenvironment and mechanical perturbation on calcium signalling in meniscus fibrochondrocytes. Eur Cell Mater (2014) 27:321–31. doi:10.22203/eCM.v027a23
16. Driscoll C, Chanalaris A, Knights C, Ismail H, Sacitharan PK, Gentry C, et al. Nociceptive sensitizers are regulated in damaged joint tissues, including articular cartilage, when osteoarthritic mice display pain behavior. Arthritis Rheumatol (2016) 68:857–67. doi:10.1002/art.39523
17. Comerford EJ, Smith K, Hayashi K. Update on the aetiopathogenesis of canine cranial cruciate ligament disease. Vet Comp Orthop Traumatol (2011) 24:91–8. doi:10.3415/VCOT-10-04-0055
18. Williams J, Tomlinson J, Constantinescu GM. Diagnosing and treating meniscal injuries in the dog. Vet Med (1994) 89:42–7.
19. Arnoczky SP, Warren RF. Winner of the 1982 Odonoghue award – the microvasculature of the meniscus and its response to injury – an experimental-study in the dog. Am J Sport Med (1983) 11:131–41. doi:10.1177/036354658301100305
20. Day B, Mackenzie WG, Shim SS, Leung G. The vascular and nerve supply of the human meniscus. Arthroscopy (1985) 1:58–62. doi:10.1016/S0749-8063(85)80080-3
21. Salata MJ, Gibbs AE, Sekiya JK. A systematic review of clinical outcomes in patients undergoing meniscectomy. Am J Sports Med (2010) 38:1907–16. doi:10.1177/0363546510370196
22. Englund M, Guermazi A, Lohmander SL. The role of the meniscus in knee osteoarthritis: a cause or consequence? Radiol Clin North Am (2009) 47:703–12. doi:10.1016/j.rcl.2009.03.003
23. Lohmander LS, Englund PM, Dahl LL, Roos EM. The long-term consequence of anterior cruciate ligament and meniscus injuries: osteoarthritis. Am J Sports Med (2007) 35:1756–69. doi:10.1177/0363546507307396
24. Cook JL, Kuroki K, Stoker AM, Monibi FA, Roller BL. Meniscal biology in health and disease. Connect Tissue Res (2017) 58:225–37. doi:10.1080/03008207.2016.1243670
25. Loeser RF. Aging and osteoarthritis: the role of chondrocyte senescence and aging changes in the cartilage matrix. Osteoarthritis Cartilage (2009) 17:971–9. doi:10.1016/j.joca.2009.03.002
26. Francuski JV, Radovanović A, Andrić N, Krstić V, Bogdanović D, Hadzić V, et al. Age-related changes in the articular cartilage of the stifle joint in non-working and working German shepherd dogs. J Comp Pathol (2014) 151:363–74. doi:10.1016/j.jcpa.2014.09.002
27. Pauli C, Grogan SP, Patil S, Otsuki S, Hasegawa A, Koziol J, et al. Macroscopic and histopathologic analysis of human knee menisci in aging and osteoarthritis. Osteoarthritis Cartilage (2011) 19:1132–41. doi:10.1016/j.joca.2011.05.008
28. Varady NH, Grodzinsky AJ. Osteoarthritis year in review 2015: mechanics. Osteoarthritis Cartilage (2016) 24:27–35. doi:10.1016/j.joca.2015.08.018
29. Loeser RF. Aging processes and the development of osteoarthritis. Curr Opin Rheumatol (2013) 25:108–13. doi:10.1097/BOR.0b013e32835a9428
30. Ionescu LC, Lee GC, Garcia GH, Zachry TL, Shah RP, Sennett BJ, et al. Maturation state-dependent alterations in meniscus integration: implications for scaffold design and tissue engineering. Tissue Eng Part A (2011) 17:193–204. doi:10.1089/ten.TEA.2010.0272
31. Majewski M, Susanne H, Klaus S. Epidemiology of athletic knee injuries: a 10-year study. Knee (2006) 13:184–8. doi:10.1016/j.knee.2006.01.005
32. Simon D, Mascarenhas R, Saltzman BM, Rollins M, Bach BR Jr, MacDonald P, et al. The relationship between anterior cruciate ligament injury and osteoarthritis of the knee. Adv Orthop (2015). doi:10.1155/2015/928301
33. Barrett JG, Hao Z, Graf BK, Kaplan LD, Heiner JP, Muir P. Inflammatory changes in ruptured canine cranial and human anterior cruciate ligaments. Am J Vet Res (2005) 66:2073–80. doi:10.2460/ajvr.2005.66.2073
34. Canapp SO Jr. The canine stifle. Clin Tech Small Anim Pract (2007) 22:195–205. doi:10.1053/j.ctsap.2007.09.008
35. Lawrence RC, Felson DT, Helmick CG, Arnold LM, Choi H, Deyo RA, et al. Estimates of the prevalence of arthritis and other rheumatic conditions in the United States. Arthritis Rheum (2008) 58:26–35. doi:10.1002/art.23176
36. Mapp PI, Walsh DA. Mechanisms and targets of angiogenesis and nerve growth in osteoarthritis. Nat Rev Rheumatol (2012) 8:390–8. doi:10.1038/nrrheum.2012.80
37. Brennan S, O’Farrell D. Familial predisposition for injury to the anterior cruciate ligament. Irish J Med Sci (2014) 183:S64–64. doi:10.1016/j.asmart.2014.02.002
38. Deng ZH, Sun MH, Li YS, Luo W, Zhang FJ, Tian J, et al. Single nucleotide polymorphisms in the CD40 gene associate with the disease susceptibility and severity in knee osteoarthritis in the Chinese Han population: a case-control study. BMC Musculoskelet Disord (2017) 18:115. doi:10.1186/s12891-017-1466-8
39. Ge W, Mu J, Huang C. The GDF5 SNP is associated with meniscus injury and function recovery in male Chinese soldiers. Int J Sports Med (2014) 35:625–8. doi:10.1055/s-0033-1355417
40. Muir P, Oldenhoff WE, Hudson AP, Manley PA, Schaefer SL, Markel MD, et al. Detection of DNA from a range of bacterial species in the knee joints of dogs with inflammatory knee arthritis and associated degenerative anterior cruciate ligament rupture. Microb Pathog (2007) 42:47–55. doi:10.1016/j.micpath.2006.10.002
41. Schwartz Z, Zitzer NC, Racette MA, Manley PA, Schaefer SL, Markel MD, et al. Are bacterial load and synovitis related in dogs with inflammatory stifle arthritis? Vet Microbiol (2011) 148:308–16. doi:10.1016/j.vetmic.2010.09.011
42. Janusz MJ, Bendele AM, Brown KK, Taiwo YO, Hsieh L, Heitmeyer SA. Induction of osteoarthritis in the rat by surgical tear of the meniscus: inhibition of joint damage by a matrix metalloproteinase inhibitor. Osteoarthritis Cartilage (2002) 10:785–91. doi:10.1053/joca.2002.0823
43. Arnoczky SP, Cook JL, Carter T, Turner AS. Translational models for studying meniscal repair and replacement: what they can and cannot tell us. Tissue Eng Part B Rev (2010) 16:31–9. doi:10.1089/ten.TEB.2009.0428
44. Takroni T, Laouar L, Adesida A, Elliott JA, Jomha NM. Anatomical study: comparing the human, sheep and pig knee meniscus. J Exp Orthop (2016) 3:35. doi:10.1186/s40634-016-0071-3
45. Sweigart MA, Zhu CF, Burt DM, DeHoll PD, Agrawal CM, Clanton TO, et al. Intraspecies and interspecies comparison of the compressive properties of the medial meniscus. Ann Biomed Eng (2004) 32:1569–79. doi:10.1114/B:ABME.0000049040.70767.5c
46. Kuyinu EL, Narayanan G, Nair LS, Laurencin CT. Animal models of osteoarthritis: classification, update, and measurement of outcomes. J Orthop Surg Res (2016) 11:19. doi:10.1186/s13018-016-0346-5
47. Frost-Christensen LN, Mastbergen SC, Vianen ME, Hartog A, DeGroot J, Voorhout G, et al. Degeneration, inflammation, regeneration, and pain/disability in dogs following destabilization or articular cartilage grooving of the stifle joint. Osteoarthritis Cartilage (2008) 16:1327–35. doi:10.1016/j.joca.2008.03.013
48. Cook JL, Kuroki K, Visco D, Pelletier JP, Schulz L, Lafeber FP. The OARSI histopathology initiative – recommendations for histological assessments of osteoarthritis in the dog. Osteoarthritis Cartilage (2010) 18(Suppl 3):S66–79. doi:10.1016/j.joca.2010.04.017
49. Johnston SA. Osteoarthritis. Joint anatomy, physiology, and pathobiology. Vet Clin North Am Small Anim Pract (1997) 27:699–723. doi:10.1016/S0195-5616(97)50076-3
50. Brandt KD, Myers SL, Burr D, Albrecht M. Osteoarthritic changes in canine articular cartilage, subchondral bone, and synovium fifty-four months after transection of the anterior cruciate ligament. Arthritis Rheum (1991) 34:1560–70. doi:10.1002/art.1780341214
51. Pozzi A, Kowaleski MP, Apelt D, Meadows C, Andrews CM, Johnson KA. Effect of medial meniscal release on tibial translation after tibial plateau leveling osteotomy. Vet Surg (2006) 35:486–94. doi:10.1111/j.1532-950X.2006.00180.x
52. Luther JK, Cook CR, Cook JL. Meniscal release in cruciate ligament intact stifles causes lameness and medial compartment cartilage pathology in dogs 12 weeks postoperatively. Vet Surg (2009) 38:520–9. doi:10.1111/j.1532-950X.2009.00520.x
53. Cook JL, Smith PA, Bozynski CC, Kuroki K, Cook CR, Stoker AM, et al. Multiple injections of leukoreduced platelet rich plasma reduce pain and functional impairment in a canine model of ACL and meniscal deficiency. J Orthop Res (2016) 34:607–15. doi:10.1002/jor.23054
54. Berjon JJ, Munuera L, Calvo M. Meniscal repair following meniscectomy: mechanism and protective effect. Experimental study in the dog. Skeletal Radiol (1990) 19:567–74.
55. Lawrence D, Bao S, Canfield PJ, Allanson M, Husband AJ. Elevation of immunoglobulin deposition in the synovial membrane of dogs with cranial cruciate ligament rupture. Vet Immunol Immunopathol (1998) 65:89–96. doi:10.1016/S0165-2427(98)00173-1
56. Scavelli TD, Schrader SC, Matthiesen DT, Skorup DE. Partial rupture of the cranial cruciate ligament of the stifle in dogs: 25 cases (1982–1988). J Am Vet Med Assoc (1990) 196:1135–8.
57. Franklin SP, Cook JL, Cook CR, Shaikh LS, Clarke KM, Holmes SP. Comparison of ultrasonography and magnetic resonance imaging to arthroscopy for diagnosing medial meniscal lesions in dogs with cranial cruciate ligament deficiency. J Am Vet Med Assoc (2017) 251:71–9. doi:10.2460/javma.251.1.71
58. Bennett D, Eckersall PD, Waterston M, Marchetti V, Rota A, McCulloch E, et al. The effect of robenacoxib on the concentration of C-reactive protein in synovial fluid from dogs with osteoarthritis. BMC Vet Res (2013) 9:42. doi:10.1186/1746-6148-9-42
59. Piel MJ, Kroin JS, van Wijnen AJ, Kc R, Im HJ. Pain assessment in animal models of osteoarthritis. Gene (2014) 537:184–8. doi:10.1016/j.gene.2013.11.091
60. Garner BC, Stoker AM, Kuroki K, Evans R, Cook CR, Cook JL. Using animal models in osteoarthritis biomarker research. J Knee Surg (2011) 24:251–64. doi:10.1055/s-0031-1297361
61. Kulkarni P, Deshpande S, Koppikar S, Patil S, Ingale D, Harsulkar A. Glycosaminoglycan measured from synovial fluid serves as a useful indicator for progression of osteoarthritis and complements Kellgren-Lawrence score. BBA Clin (2016) 6:1–4. doi:10.1016/j.bbacli.2016.05.002
62. Adams ME, Billingham MEJ, Muir H. The glycosaminoglycans in menisci in experimental and natural osteoarthritis. Arthritis Rheum (1983) 26:69–76. doi:10.1002/art.1780260111
63. Fujita Y, Hara Y, Nezu Y, Schulz KS, Tagawa M. Proinflammatory cytokine activities, matrix metalloproteinase-3 activity, and sulfated glycosaminoglycan content in synovial fluid of dogs with naturally acquired cranial cruciate ligament rupture. Vet Surg (2006) 35:369–76. doi:10.1111/j.1532-950X.2006.00159.x
64. Innes JF, Little CB, Hughes CE, Caterson B. Products resulting from cleavage of the interglobular domain of aggrecan in samples of synovial fluid collected from dogs with early- and late-stage osteoarthritis. Am J Vet Res (2005) 66:1679–85. doi:10.2460/ajvr.2005.66.1679
65. Fox AJ, Bedi A, Rodeo SA. The basic science of human knee menisci: structure, composition, and function. Sports Health (2012) 4:340–51. doi:10.1177/1941738111429419
66. Catterall JB, Stabler TV, Flannery CR, Kraus VB. Changes in serum and synovial fluid biomarkers after acute injury (NCT00332254). Arthritis Res Ther (2010) 12:R229. doi:10.1186/ar3216
67. Stone AV, Loeser RF, Vanderman KS, Long DL, Clark SC, Ferguson CM. Pro-inflammatory stimulation of meniscus cells increases production of matrix metalloproteinases and additional catabolic factors involved in osteoarthritis pathogenesis. Osteoarthritis Cartilage (2014) 22:264–74. doi:10.1016/j.joca.2013.11.002
68. Zhang E, Yan X, Zhang M, Chang X, Bai Z, He Y, et al. Aggrecanases in the human synovial fluid at different stages of osteoarthritis. Clin Rheumatol (2013) 32:797–803. doi:10.1007/s10067-013-2171-0
69. Roberts S, Evans H, Wright K, van Niekerk L, Caterson B, Richardson JB, et al. ADAMTS-4 activity in synovial fluid as a biomarker of inflammation and effusion. Osteoarthritis Cartilage (2015) 23:1622–6. doi:10.1016/j.joca.2015.05.006
70. Tchetverikov I, Lohmander LS, Verzijl N, Huizinga TW, TeKoppele JM, Hanemaaijer R, et al. MMP protein and activity levels in synovial fluid from patients with joint injury, inflammatory arthritis, and osteoarthritis. Ann Rheum Dis (2005) 64:694–8. doi:10.1136/ard.2004.022434
71. Salinardi BJ, Roush JK, Schermerhorn T, Mitchell KE. Matrix metalloproteinase and tissue inhibitor of metalloproteinase in serum and synovial fluid of osteoarthritic dogs. Vet Comp Orthop Traumatol (2006) 19:49–55. doi:10.1055/s-0038-1632973
72. Heard BJ, Martin L, Rattner JB, Frank CB, Hart DA, Krawetz R. Matrix metalloproteinase protein expression profiles cannot distinguish between normal and early osteoarthritic synovial fluid. BMC Musculoskelet Disord (2012) 13:126. doi:10.1186/1471-2474-13-126
73. Boland L, Danger R, Cabon Q, Rabillard M, Brouard S, Bouvy B, et al. MMP-2 as an early synovial biomarker for cranial cruciate ligament disease in dogs. Vet Comp Orthop Traumatol (2014) 27:210–5. doi:10.3415/VCOT-13-06-0082
74. Rabillard M, Danger R, Doran IP, Niebauer GW, Brouard S, Gauthier O. Matrix metalloproteinase activity in stifle synovial fluid of cranial cruciate ligament deficient dogs and effect of postoperative doxycycline treatment. Vet J (2012) 193:271–3. doi:10.1016/j.tvjl.2011.10.028
75. Brophy RH, Rai MF, Zhang Z, Torgomyan A, Sandell LJ. Molecular analysis of age and sex-related gene expression in meniscal tears with and without a concomitant anterior cruciate ligament tear. J Bone Joint Surg Am (2012) 94:385–93. doi:10.2106/JBJS.K.00919
76. Özler K, Aktaş E, Atay Ç, Yılmaz B, Arıkan M, Güngör Ş. Serum and knee synovial fluid matrix metalloproteinase-13 and tumor necrosis factor-alpha levels in patients with late-stage osteoarthritis. Acta Orthop Traumatol Turc (2016) 50:356–61. doi:10.3944/AOTT.2015.15.0115
77. Mabey T, Honsawek S. Cytokines as biochemical markers for knee osteoarthritis. World J Orthop (2015) 6:95–105. doi:10.5312/wjo.v6.i1.95
78. Venn G, Nietfeld JJ, Duits AJ, Brennan FM, Arner E, Covington M, et al. Elevated synovial-fluid levels of interleukin-6 and tumor-necrosis-factor associated with early experimental canine osteoarthritis. Arthritis Rheum (1993) 36:819–26. doi:10.1002/art.1780360613
79. de Bruin T, de Rooster H, van Bree H, Cox E. Interleukin-8 mRNA expression in synovial fluid of canine stifle joints with osteoarthritis. Vet Immunol Immunopathol (2005) 108:387–97. doi:10.1016/j.vetimm.2005.06.013
80. Kilani RT, Maksymowych WP, Aitken A, Boire G, St-Pierre Y, Li Y, et al. Detection of high levels of 2 specific isoforms of 14-3-3 proteins in synovial fluid from patients with joint inflammation. J Rheumatol (2007) 34:1650–7.
81. Sardari K, Chavez-Munoz C, Kilani RT, Schiller T, Ghahary A. Increased levels of the 14-3-3 eta and gamma proteins in the synovial fluid of dogs with unilateral cranial cruciate ligament rupture. Can J Vet Res (2011) 75:271–7.
82. Halliday DA, Zettler C, Rush RA, Scicchitano R, McNeil JD. Elevated nerve growth factor levels in the synovial fluid of patients with inflammatory joint disease. Neurochem Res (1998) 23:919–22. doi:10.1023/A:1022475432077
83. Isola M, Ferrari V, Miolo A, Stabile F, Bernardini D, Carnier P, et al. Nerve growth factor concentrations in the synovial fluid from healthy dogs and dogs with secondary osteoarthritis. Vet Comp Orthop Traumatol (2011) 24:279–84. doi:10.3415/VCOT-10-04-0051
84. Kumahashi N, Naitou K, Nishi H, Oae K, Watanabe Y, Kuwata S, et al. Correlation of changes in pain intensity with synovial fluid adenosine triphosphate levels after treatment of patients with osteoarthritis of the knee with high-molecular-weight hyaluronic acid. Knee (2011) 18:160–4. doi:10.1016/j.knee.2010.04.013
85. Torres BT, Jimenez DA, Budsberg SC. Elevated synovial fluid concentration of adenosine triphosphate in dogs with osteoarthritis or sodium urate-induced synovitis of the stifle. Vet Comp Orthop Traumatol (2016) 29:344–6. doi:10.3415/VCOT-15-06-0111
86. Hennerbichler A, Fermor B, Hennerbichler D, Weinberg JB, Guilak F. Regional differences in prostaglandin E2 and nitric oxide production in the knee meniscus in response to dynamic compression. Biochem Biophys Res Commun (2007) 358:1047–53. doi:10.1016/j.bbrc.2007.05.026
87. Hiraiwa H, Sakai T, Mitsuyama H, Hamada T, Yamamoto R, Omachi T, et al. Inflammatory effect of advanced glycation end products on human meniscal cells from osteoarthritic knees. Inflamm Res (2011) 60:1039–48. doi:10.1007/s00011-011-0365-y
88. Liu B, Goode AP, Carter TE, Utturkar GM, Huebner JL, Taylor DC, et al. Matrix metalloproteinase activity and prostaglandin E2 are elevated in the synovial fluid of meniscus tear patients. Connect Tissue Res (2016):305–16. doi:10.1080/03008207.2016.1256391
89. Mastbergen SC, Marijnissen AC, Vianen ME, Zoer B, van Roermund PM, Bijlsma JW, et al. Inhibition of COX-2 by celecoxib in the canine groove model of osteoarthritis. Rheumatology (2006) 45:405–13. doi:10.1093/rheumatology/kei187
90. Inoue H, Shimoyama Y, Hirabayashi K, Kajigaya H, Yamamoto S, Oda H, et al. Production of neuropeptide substance P by synovial fibroblasts from patients with rheumatoid arthritis and osteoarthritis. Neurosci Lett (2001) 303:149–52. doi:10.1016/S0304-3940(01)01713-X
91. Ashraf S, Wibberley H, Mapp PI, Hill R, Wilson D, Walsh DA. Increased vascular penetration and nerve growth in the meniscus: a potential source of pain in osteoarthritis. Ann Rheum Dis (2011) 70:523–9. doi:10.1136/ard.2010.137844
92. Dong T, Chang H, Zhang F, Chen W, Zhu Y, Wu T, et al. Calcitonin gene-related peptide can be selected as a predictive biomarker on progression and prognosis of knee osteoarthritis. Int Orthop (2015) 39:1237–43. doi:10.1007/s00264-015-2744-4
93. Senolt L, Braun M, Olejárová M, Forejtová S, Gatterová J, Pavelka K. Increased pentosidine, an advanced glycation end product, in serum and synovial fluid from patients with knee osteoarthritis and its relation with cartilage oligomeric matrix protein. Ann Rheum Dis (2005) 64:886–90. doi:10.1136/ard.2004.029140
94. Sun XH, Liu Y, Han Y, Wang J. Expression and significance of high-mobility group protein B1 (HMGB1) and the receptor for advanced glycation end-product (RAGE) in knee osteoarthritis. Med Sci Monit (2016) 22:2105–12. doi:10.12659/MSM.895689
95. Hashimoto S, Takahashi K, Ochs RL, Coutts RD, Amiel D, Lotz M. Nitric oxide production and apoptosis in cells of the meniscus during experimental osteoarthritis. Arthritis Rheum (1999) 42:2123–31. doi:10.1002/1529-0131(199910)42:10<2123::AID-ANR12>3.0.CO;2-G
96. Weinberg JB, Fermor B, Guilak F. Nitric oxide synthase and cyclooxygenase interactions in cartilage and meniscus: relationships to joint physiology, arthritis, and tissue repair. Subcell Biochem (2007) 42:31–62. doi:10.1007/1-4020-5688-5_2
97. Green DM, Noble PC, Bocell JR Jr, Ahuero JS, Poteet BA, Birdsall HH. Effect of early full weight-bearing after joint injury on inflammation and cartilage degradation. J Bone Joint Surg Am (2006) 88a:2201–9. doi:10.2106/00004623-200610000-00012
98. Schett G, Elewaut D, McInnes IB, Dayer JM, Neurath MF. Toward a cytokine-based disease taxonomy. Nat Med (2013) 19:822–4. doi:10.1038/nm.3260
99. Berenbaum F. Osteoarthritis as an inflammatory disease (osteoarthritis is not osteoarthrosis!). Osteoarthritis Cartilage (2013) 21:16–21. doi:10.1016/j.joca.2012.11.012
100. McNulty AL, Weinberg JB, Guilak F. Inhibition of matrix metalloproteinases enhances in vitro repair of the meniscus. Clin Orthop Relat Res (2009) 467:1557–67. doi:10.1007/s11999-008-0596-6
101. Wilusz RE, Weinberg JB, Guilak F, McNulty AL. Inhibition of integrative repair of the meniscus following acute exposure to interleukin-1 in vitro. J Orthop Res (2008) 26:504–12. doi:10.1002/jor.20538
102. Fuller ES, Smith MM, Little CB, Melrose J. Zonal differences in meniscus matrix turnover and cytokine response. Osteoarthritis Cartilage (2012) 20:49–59. doi:10.1016/j.joca.2011.10.002
103. El-Hadi M, Charavaryamath C, Aebischer A, Smith CW, Shmon C, Singh B. Expression of interleukin-8 and intercellular cell adhesion molecule-1 in the synovial membrane and cranial cruciate ligament of dogs after rupture of the ligament. Can J Vet Res (2012) 76:8–15.
104. Muir P, Schaefer SL, Manley PA, Svaren JP, Oldenhoff WE, Hao Z. Expression of immune response genes in the stifle joint of dogs with oligoarthritis and degenerative cranial cruciate ligament rupture. Vet Immunol Immunopathol (2007) 119:214–21. doi:10.1016/j.vetimm.2007.05.016
106. Maccoux LJ, Salway F, Day PJ, Clements DN. Expression profiling of select cytokines in canine osteoarthritis tissues. Vet Immunol Immunopathol (2007) 118:59–67. doi:10.1016/j.vetimm.2007.04.006
107. Yin JH, Xia Y. Proteoglycan concentrations in healthy and diseased articular cartilage by Fourier transform infrared imaging and principal component regression. Spectrochim Acta A (2014) 133:825–30. doi:10.1016/j.saa.2014.05.092
108. Scanzello CR, McKeon B, Swaim BH, DiCarlo E, Asomugha EU, Kanda V, et al. Synovial inflammation in patients undergoing arthroscopic meniscectomy molecular characterization and relationship to symptoms. Arthritis Rheum (2011) 63:391–400. doi:10.1002/art.30137
109. de Lange-Brokaar BJE, Ioan-Facsinay A, van Osch GJ, Zuurmond AM, Schoones J, Toes RE, et al. Synovial inflammation, immune cells and their cytokines in osteoarthritis: a review. Osteoarthritis Cartilage (2012) 20:1484–99. doi:10.1016/j.joca.2012.08.027
110. Little JP, Bleedorn JA, Sutherland BJ, Sullivan R, Kalscheur VL, Ramaker MA, et al. Arthroscopic assessment of stifle synovitis in dogs with cranial cruciate ligament rupture. PLoS One (2014) 9:e97329. doi:10.1371/journal.pone.0097329
111. Niebauer GW, Wolf B, Bashey RI, Newton CD. Antibodies to canine collagen types I and II in dogs with spontaneous cruciate ligament rupture and osteoarthritis. Arthritis Rheum (1987) 30:319–27. doi:10.1002/art.1780300311
112. Cramer GD, Darby S. Basic and clinical anatomy of the spine, spinal cord, and ANS. J Manipulative Physiol Ther (1997) 20:294–294.
113. Melrose J, Roughley P, Knox S, Smith S, Lord M, Whitelock J. The structure, location, and function of perlecan, a prominent pericellular proteoglycan of fetal, postnatal, and mature hyaline cartilages. J Biol Chem (2006) 281:36905–14. doi:10.1074/jbc.M608462200
114. Melrose J, Smith S, Cake M, Read R, Whitelock J. Comparative spatial and temporal localisation of perlecan, aggrecan and type I, II and IV collagen in the ovine meniscus: an ageing study. Histochem Cell Biol (2005) 124:225–35. doi:10.1007/s00418-005-0005-0
115. Fletcher DA, Mullins D. Cell mechanics and the cytoskeleton. Nature (2010) 463:485–92. doi:10.1038/nature08908
116. Eifler RL, Blough ER, Dehlin JM, Haut Donahue TL. Oscillatory fluid flow regulates glycosaminoglycan production via an intracellular calcium pathway in meniscal cells. J Orthop Res (2006) 24:375–84. doi:10.1002/jor.20028
117. Wu LJ, Sweet TB, Clapham DE. International union of basic and clinical pharmacology. LXXVI. Current progress in the mammalian TRP ion channel family. Pharmacol Rev (2010) 62:381–404. doi:10.1124/pr.110.002725
118. Clark AL, Votta BJ, Kumar S, Liedtke W, Guilak F. Chondroprotective role of the osmotically sensitive ion channel transient receptor potential vanilloid 4: age- and sex-dependent progression of osteoarthritis in Trpv4-deficient mice. Arthritis Rheum (2010) 62:2973–83. doi:10.1002/art.27624
119. Zhou X, Wang W, Miao J, Bai L. Expression and significance of transient receptor potential cation channel V5 in articular cartilage cells under exercise loads. Biomed Rep (2014) 2:813–7. doi:10.3892/br.2014.333
120. Sies H. Role of metabolic H2O2 generation: redox signaling and oxidative stress. J Biol Chem (2014) 289:8735–41. doi:10.1074/jbc.R113.544635
121. Espinoza SE, Guo H, Fedarko N, DeZern A, Fried LP, Xue QL, et al. Glutathione peroxidase enzyme activity in aging. J Gerontol A Biol Sci Med Sci (2008) 63:505–9. doi:10.1093/gerona/63.5.505
122. Suantawee T, Tantavisut S, Adisakwattana S, Tanavalee A, Yuktanandana P, Anomasiri W, et al. Oxidative stress, vitamin E, and antioxidant capacity in knee osteoarthritis. J Clin Diagn Res (2013) 7:1855–9. doi:10.7860/JCDR/2013/5802.3333
123. Smith VH. Vitamin C deficiency is an under-diagnosed contributor to degenerative disc disease in the elderly. Med Hypotheses (2010) 74:695–7. doi:10.1016/j.mehy.2009.10.041
124. Hayflick L, Moorhead PS. The serial cultivation of human diploid cell strains. Exp Cell Res (1961) 25:585–621. doi:10.1016/0014-4827(61)90192-6
125. Munoz-Espin D, Serrano M. Cellular senescence: from physiology to pathology. Nat Rev Mol Cell Biol (2014) 15:482. doi:10.1038/nrm3823
126. van Deursen JM. The role of senescent cells in ageing. Nature (2014) 509:439–46. doi:10.1038/nature13193
127. Yudoh K, Nguyen VT, Nakamura H, Hongo-Masuko K, Kato T, Nishioka K. Potential involvement of oxidative stress in cartilage senescence and development of osteoarthritis: oxidative stress induces chondrocyte telomere instability and downregulation of chondrocyte function. Arthritis Res Ther (2005) 7:R380–91. doi:10.1186/ar1765
128. Dycus DL, Au AY, Grzanna MW, Wardlaw JL, Frondoza CG. Modulation of inflammation and oxidative stress in canine chondrocytes. Am J Vet Res (2013) 74:983–9. doi:10.2460/ajvr.74.7.983
129. Verzijl N, DeGroot J, Ben Zaken C, Brau-Benjamin O, Maroudas A, Bank RA, et al. Crosslinking by advanced glycation end products increases the stiffness of the collagen network in human articular cartilage – a possible mechanism through which age is a risk factor for osteoarthritis. Arthritis Rheum (2002) 46:114–23. doi:10.1002/1529-0131(200201)46:1<114::AID-ART10025>3.0.CO;2-P
130. DeGroot J, Bank R, Bijlsma J, TeKoppele J, Verzijl N, Lafeber F. Advanced glycation endproducts in the development of osteoarthritis. Arthritis Res Therv (2004) 6:78. doi:10.1186/ar1414
131. DeGroot J, Verzijl N, Wenting-van Wijk MJ, Jacobs KM, Van El B, Van Roermund PM, et al. Accumulation of advanced glycation end products as a molecular mechanism for aging as a risk factor in osteoarthritis. Arthritis Rheum (2004) 50:1207–15. doi:10.1002/art.20170
132. Nah SS, Choi IY, Yoo B, Kim YG, Moon HB, Lee CK. Advanced glycation end products increases matrix metalloproteinase-1, -3, and -13, and TNF-alpha in human osteoarthritic chondrocytes. FEBS Lett (2007) 581:1928–32. doi:10.1016/j.febslet.2007.03.090
133. Yamabe S, Hirose J, Uehara Y, Okada T, Okamoto N, Oka K, et al. Intracellular accumulation of advanced glycation end products induces apoptosis via endoplasmic reticulum stress in chondrocytes. FEBS J (2013) 280:1617–29. doi:10.1111/febs.12170
134. Larkin DJ, Kartchner JZ, Doxey AS, Hollis WR, Rees JL, Wilhelm SK, et al. Inflammatory markers associated with osteoarthritis after destabilization surgery in young mice with and without receptor for advanced glycation end-products (RAGE). Front Physiol (2013) 4:121. doi:10.3389/fphys.2013.00121
135. Wilhelm S, Mecham D, Matias EC, Kartchner J, Crepeau P. Blocking the receptor for advanced glycation end-products but not toll-like receptor 4 attenuates the progression of OA. FASEB J (2014) 28.
136. Xie JL, Mendez JD, Mendez-Valenzuela V, Aguilar-Hernández MM. Cellular signalling of the receptor for advanced glycation end products (RAGE). Cell Signal (2013) 25:2185–97. doi:10.1016/j.cellsig.2013.06.013
137. Garg S, Syngle A, Vohra K. Efficacy and tolerability of advanced glycation end-products inhibitor in osteoarthritis a randomized, double-blind, placebo-controlled study. Clin J Pain (2013) 29:717–24. doi:10.1097/AJP.0b013e318272ebec
138. Areti A, Yerra VG, Naidu V, Kumar A. Oxidative stress and nerve damage: role in chemotherapy induced peripheral neuropathy. Redox Biol (2014) 2:289–95. doi:10.1016/j.redox.2014.01.006
139. Melchiorri C, Meliconi R, Frizziero L, Silvestri T, Pulsatelli L, Mazzetti I, et al. Enhanced and coordinated in vivo expression of inflammatory cytokines and nitric oxide synthase by chondrocytes from patients with osteoarthritis. Arthritis Rheum (1998) 41:2165–74. doi:10.1002/1529-0131(199812)41:12<2165::AID-ART11>3.0.CO;2-O
140. Cízková D, Lukácová N, Marsala M, Marsala J. Neuropathic pain is associated with alterations of nitric oxide synthase immunoreactivity and catalytic activity in dorsal root ganglia and spinal dorsal horn. Brain Res Bull (2002) 58:161–71. doi:10.1016/S0361-9230(02)00761-X
141. Levy D, Zochodne DW. NO pain: potential roles of nitric oxide in neuropathic pain. Pain Pract (2004) 4:11–8. doi:10.1111/j.1533-2500.2004.04002.x
142. Floyd RA. Antioxidants, oxidative stress, and degenerative neurological disorders. Proc Soc Exp Biol Med (1999) 222:236–45. doi:10.1046/j.1525-1373.1999.d01-140.x
143. Boileau C, Martel-Pelletier J, Moldovan F, Jouzeau JY, Netter P, Manning PT, et al. The in situ up-regulation of chondrocyte interleukin-1-converting enzyme and interleukin-18 levels in experimental osteoarthritis is mediated by nitric oxide. Arthritis Rheum (2002) 46:2637–47. doi:10.1002/art.10518
144. Shen C, Yan J, Erkocak OF, Zheng XF, Chen XD. Nitric oxide inhibits autophagy via suppression of JNK in meniscal cells. Rheumatology (2014) 53:1022–33. doi:10.1093/rheumatology/ket471
145. Forterre S, Zurbriggen A, Spreng D. Nitric oxide induces cell death in canine cruciate ligament cells by activation of tyrosine kinase and reactive oxygen species. BMC Vet Res (2012) 8:40. doi:10.1186/1746-6148-8-40
146. Yoshida M, Sai S, Marumo K, Tanaka T, Itano N, Kimata K, et al. Expression analysis of three isoforms of hyaluronan synthase and hyaluronidase in the synovium of knees in osteoarthritis and rheumatoid arthritis by quantitative real-time reverse transcriptase polymerase chain reaction. Arthritis Res Ther (2004) 6:R514–20. doi:10.1186/ar1223
147. Quero L, Klawitter M, Schmaus A, Rothley M, Sleeman J, Tiaden AN, et al. Hyaluronic acid fragments enhance the inflammatory and catabolic response in human intervertebral disc cells through modulation of toll-like receptor 2 signalling pathways. Arthritis Res Ther (2013) 15:R94. doi:10.1186/ar4274
148. Kitamura R, Tanimoto K, Tanne Y, Kamiya T, Huang YC, Tanaka N, et al. Effects of mechanical load on the expression and activity of hyaluronidase in cultured synovial membrane cells. J Biomed Mater Res A (2010) 92a:87–93. doi:10.1002/jbm.a.32345
149. Conrozier T, Mathieu P, Rinaudo M. Mannitol preserves the viscoelastic properties of hyaluronic acid in an in vitro model of oxidative stress. Rheumatol Ther (2014) 1:45–54. doi:10.1007/s40744-014-0001-8
150. Noble PW. Hyaluronan and its catabolic products in tissue injury and repair. Matrix Biol (2002) 21:25–9. doi:10.1016/S0945-053X(01)00184-6
151. Venable RO, Stoker AM, Cook CR, Cockrell MK, Cook JL. Examination of synovial fluid hyaluronan quantity and quality in stifle joints of dogs with osteoarthritis. Am J Vet Res (2008) 69:1569–73. doi:10.2460/ajvr.69.12.1569
152. Thakur M, Dickenson AH, Baron R. Osteoarthritis pain: nociceptive or neuropathic? Nat Rev Rheumatol (2014) 10:374–80. doi:10.1038/nrrheum.2014.47
153. Ricciotti E, FitzGerald GA. Prostaglandins and inflammation. Arterioscler Thromb Vasc Biol (2011) 31:986–1000. doi:10.1161/ATVBAHA.110.207449
154. Pecchi E, Priam S, Gosset M, Pigenet A, Sudre L, Laiguillon MC, et al. Induction of nerve growth factor expression and release by mechanical and inflammatory stimuli in chondrocytes: possible involvement in osteoarthritis pain. Arthritis Res Ther (2014) 16:R16. doi:10.1186/ar4443
155. Grassel S. The role of peripheral nerve fibers and their neurotransmitters in cartilage and bone physiology and pathophysiology. Arthritis Res Ther (2014) 16:485. doi:10.1186/s13075-014-0485-1
156. Kumar V, Mahal BA. NGF – the TrkA to successful pain treatment. J Pain Res (2012) 5:279–87. doi:10.2147/JPR.S33408
157. Freund V, Pons F, Joly V, Mathieu E, Martinet N, Frossard N. Upregulation of nerve growth factor expression by human airway smooth muscle cells in inflammatory conditions. Eur Respir J (2002) 20:458–63. doi:10.1183/09031936.02.00269202
158. Kraemer BR, Yoon SO, Carter BD. The biological functions and signaling mechanisms of the p75 neurotrophin receptor. Handb Exp Pharmacol (2014) 220:121–64. doi:10.1007/978-3-642-45106-5_6
159. Skoff AM, Adler JE. Nerve growth factor regulates substance P in adult sensory neurons through both TrkA and p75 receptors. Exp Neurol (2006) 197:430–6. doi:10.1016/j.expneurol.2005.10.006
160. Sluka KA, Gregory NS. The dichotomized role for acid sensing ion channels in musculoskeletal pain and inflammation. Neuropharmacology (2015) 94:58–63. doi:10.1016/j.neuropharm.2014.12.013
Keywords: meniscus, inflammation, oxidative stress, pain, dog
Citation: Krupkova O, Smolders L, Wuertz-Kozak K, Cook J and Pozzi A (2018) The Pathobiology of the Meniscus: A Comparison Between the Human and Dog. Front. Vet. Sci. 5:73. doi: 10.3389/fvets.2018.00073
Received: 19 December 2017; Accepted: 26 March 2018;
Published: 16 April 2018
Edited by:
Christopher R. Byron, Virginia Tech, United StatesReviewed by:
David L. Dycus, Veterinary Orthopedic Sports Medicine Group, United StatesAmy S. Kapatkin, University of California, Davis, United States
Copyright: © 2018 Krupkova, Smolders, Wuertz-Kozak, Cook and Pozzi. This is an open-access article distributed under the terms of the Creative Commons Attribution License (CC BY). The use, distribution or reproduction in other forums is permitted, provided the original author(s) and the copyright owner are credited and that the original publication in this journal is cited, in accordance with accepted academic practice. No use, distribution or reproduction is permitted which does not comply with these terms.
*Correspondence: Olga Krupkova, b2tydXBrb3ZhQGV0aHouY2g=