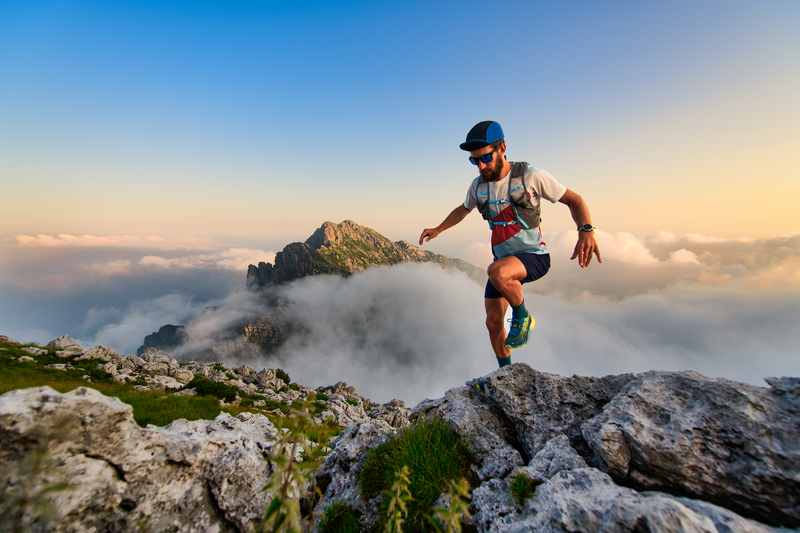
94% of researchers rate our articles as excellent or good
Learn more about the work of our research integrity team to safeguard the quality of each article we publish.
Find out more
REVIEW article
Front. Toxicol. , 13 June 2024
Sec. In Vitro Toxicology
Volume 6 - 2024 | https://doi.org/10.3389/ftox.2024.1376118
This article is part of the Research Topic Advances in inhalation safety assessment View all 9 articles
A correction has been applied to this article in:
Applying new approach methodologies to assess next-generation tobacco and nicotine products
In vitro toxicology research has accelerated with the use of in silico, computational approaches and human in vitro tissue systems, facilitating major improvements evaluating the safety and health risks of novel consumer products. Innovation in molecular and cellular biology has shifted testing paradigms, with less reliance on low-throughput animal data and greater use of medium- and high-throughput in vitro cellular screening approaches. These new approach methodologies (NAMs) are being implemented in other industry sectors for chemical testing, screening candidate drugs and prototype consumer products, driven by the need for reliable, human-relevant approaches. Routine toxicological methods are largely unchanged since development over 50 years ago, using high-doses and often employing in vivo testing. Several disadvantages are encountered conducting or extrapolating data from animal studies due to differences in metabolism or exposure. The last decade saw considerable advancement in the development of in vitro tools and capabilities, and the challenges of the next decade will be integrating these platforms into applied product testing and acceptance by regulatory bodies. Governmental and validation agencies have launched and applied frameworks and “roadmaps” to support agile validation and acceptance of NAMs. Next-generation tobacco and nicotine products (NGPs) have the potential to offer reduced risks to smokers compared to cigarettes. These include heated tobacco products (HTPs) that heat but do not burn tobacco; vapor products also termed electronic nicotine delivery systems (ENDS), that heat an e-liquid to produce an inhalable aerosol; oral smokeless tobacco products (e.g., Swedish-style snus) and tobacco-free oral nicotine pouches. With the increased availability of NGPs and the requirement of scientific studies to support regulatory approval, NAMs approaches can supplement the assessment of NGPs. This review explores how NAMs can be applied to assess NGPs, highlighting key considerations, including the use of appropriate in vitro model systems, deploying screening approaches for hazard identification, and the importance of test article characterization. The importance and opportunity for fit-for-purpose testing and method standardization are discussed, highlighting the value of industry and cross-industry collaborations. Supporting the development of methods that are accepted by regulatory bodies could lead to the implementation of NAMs for tobacco and nicotine NGP testing.
Toxicological risk assessment methods have remained largely unchanged for half a century, with the traditional default approach using high doses administered in animal studies, human exposure estimates, and the use of conservative assessment (uncertainty) factors or linear extrapolations to establish whether a given chemical exposure is deemed “safe” or “unsafe” based on human exposure as well as estimating levels of potential risk. Despite the implementation of some changes to animal testing protocols over the years, the results from new in vitro approaches are still judged against this process of extrapolating the adverse effects of high doses in animals to low-dose exposures in humans. Refinements to animal studies have come in the form of reducing numbers of animals or ultimately waiving certain in vivo tests completely (e.g., acute in vivo toxicity “6-pack” testing for oral, dermal, and inhalation acute lethality; eye and skin irritation; and skin sensitization) (Mansouri et al., 2021; Lee et al., 2022). This is consistent to the guiding principles (3Rs) for ethical use of animals in product testing and scientific research that has been introduced by Russel and Burch (Russell and Burch, 1959).
The 3Rs are:
• Replacement that seeks to use methods to avoid or replace the use of animals;
• Reduction using methods that allow researchers to obtain information using fewer numbers of animals in scientific studies; and
• Refinement using methods to reduce potential pain, suffering or distress, and enhance animal welfare.
These broadly accepted ethical principles are now embedded in the conduct of animal-based science in many countries. In 2004, the UK government funded the creation of the National Centre for Reduction Refinement and Replacement of Animals in Research (NC3Rs) with the goal that research trends do not lead to increased animal usage or suffering (Burden et al., 2015). In the U.S., the Toxic Substances Control Act (TSCA), as amended by the Chemical Safety for the 21st Century Act, directs the U.S. Environmental Protection Agency (EPA) to “reduce and replace, to the extent practicable and scientifically justified, the use of vertebrate animals in the testing of chemical substances or mixtures; and promote the development and timely incorporation of alternative test methods or strategies that do not require new vertebrate animal testing” (U.S. Environmental Protection Agency, 2023).
The challenges to use of animal models have arisen from several different applications of toxicology. Issues with animal models have been reported, including questioning the usefulness of current mouse models due to irreproducibility and poor recapitulation of human conditions and highlighting the fact that almost no animal models are validated (Justice and Dhillon, 2016; Perlman, 2016). There is also difficulty in extrapolating high doses in animal studies to low doses in humans, with 22% of all the chemicals tested in high-dose in vivo carcinogenicity studies being positive for cancer (Ennever and Lave, 2003). During development, 30% of drugs in Phase 1 (first use in humans) fail due to unexpected side effects or lack of efficacy, but the overall failure rate is ∼90% for drugs in Phase 1 clinical trials due to other causes such as low efficacy (Sun et al., 2022). In light of this issue, the U.S. National Academies of Science, Engineering, and Medicine (NASEM) were asked to radically rethink traditional toxicological testing methodology, based on the large numbers of chemicals already released into the environment (>10,000) that had no associated toxicological data and the potential time and cost required to implement animal tests. The primary goals for the report were: to provide as wide a coverage of chemicals, outcomes and life stages as possible; reduce the costs and time for testing; use fewer animals with less suffering; and develop more robust methods for environmental chemical assessment. It was further recommended that assays chosen should also reflect the large gains in science that have been made in the last decades such as the use of the omics technologies. The U.S. National Research Council (NRC) released a 2007 report entitled “Toxicity Testing in the 21st Century: A Vision and a Strategy” (TT21C) (National Research Council, 2007) that proposed an alternative assessment testing paradigm where virtually all routine toxicity testing would be conducted in vitro (in human cells or cell lines). The underlying concept was that high-throughput toxicity pathway assays could evaluate disruption in key cellular processes. Toxicological risk assessment based on results from such assays would help avoid significant perturbations of known key cellular pathways in exposed human populations. Instead, dose-response modeling of altered pathway functions could be organized based on computational systems biology models of the networks underlying each toxicity pathway (Andersen and Krewski, 2009). This concept of pathway-based approaches to risk assessment was expanded by the description of “Adverse Outcome Pathways” (AOPs). Now the challenges are translating the AOP/TT21C vision into practical tools that will be useful to those making safety decisions and determining how to provide new mechanistic data not normally reviewed by risk assessors.
Following the release of the 2007 NRC report, consortia, collaborations, and initiatives were adopted to apply TTC21 in vitro toxicological approaches. In the US, Toxicology in the 21st Century (Tox21) was formed as part of a federal agency consortium, bringing together the EPA, the National Toxicology Program at the National Institute of Environmental Health Science, National Institutes of Health’s National Center for Advancing Translational Sciences, and the Food and Drug Administration (Thomas et al., 2019). The goal of this program set out to develop assays to measure the pathways that lead to adverse effects in humans and develop models that can predict toxicity by using robotic technology to screen tens of thousands of environmental chemicals. Phase 1 of Tox21 involved testing 2,800 chemicals in 50 in vitro assays, with Phase 2 covering a further 10,000 chemicals (Attene-Ramos et al., 2013). European initiatives were developed following the ban on testing of cosmetic ingredients that came in to force in Europe 2013 (76/768EEC) (Silva and Tamburic, 2022) and continued as part of the European Union’s Horizon 2020 project (Vinken, 2020). Similar to U.S. approaches, they have goals of looking for alternative testing methods via the Safety Evaluation Ultimately Replacing Animal Testing (SEURAT) (Daston et al., 2015). More recently in 2018, the Interagency Coordinating Committee on the Validation of Alternative Methods (ICCVAM, 2018) released its roadmap for the evaluation and implementation of new approach methodologies (NAMs) to support agile validation of scientific data from TT21C-based methods to be accepted by regulatory agencies without going through full Organisation for Economic Cooperation and Development (OECD)-like validation that could take 20 years or more. At the same time, utilization of NAM approaches including extrapolating in vitro data to in vivo exposures in humans would require computational and pharmacokinetic models to predict human blood and tissue concentrations under specific exposure conditions. Unfortunately, the scientific tools needed to make these changes in toxicological risk assessment practices are still in various stages of development and qualification (ICCVAM, 2023).
Realizing this vision for the future of toxicity testing will require wide-ranging scientific discussion among stakeholders and regulators, with a potential education program to motivate a shift from animal-based toxicological tests toward an appropriate approach more firmly based on human biology. This review focuses on how such a paradigm could be applied for the evaluation of alternative next-generation tobacco and nicotine products (NGPs). The review explores how NAMs approaches can be used to assess NGPs, and will highlight key considerations, such as the use of appropriate in vitro model systems, the use of screening approaches for hazard identification, and test article characterization. Furthermore other considerations such as AOPs, acute verses repeated-exposures, and in vitro to in vivo extrapolation will be explored. The value of industry and cross-industry collaborations are discussed outlining the importance and opportunity for fit-for-purpose testing and method standardization. With the increased requirement of scientific studies to support regulatory approval of NGPs, use of NAMs approaches should be considered for the assessment of NGPs as part of a testing strategy.
Next-generation tobacco and nicotine products (NGPs) have evolved significantly over the last decade as adults who smoke seek less-harmful alternatives to conventional cigarettes. Increased global consumer uptake has driven innovation and development, which has led to greater product complexity. Toxicological risk assessment and the development of technical ingredient and product standards have enabled the development and maintenance of product quality standards and product material and ingredient quality for responsible manufacturers (Simms et al., 2019). Inclusion and adaptation of in vitro testing strategies can play a critical role in supporting NGP assessment, especially of inhalable products in filling the data gap in potential inhalation toxicity. NGPs not only offer the consumer an alternative choice to smoking, but these products have been reported to typically contain fewer toxicants and in lower levels compared to cigarette smoke (Margham et al., 2016; Schaller et al., 2016; Forster et al., 2018; Lu et al., 2021), offering a significant opportunity to potentially reduce the health impact of cigarette smoking on a global scale (McNeill et al., 2018; McNeil et al., 2022). This paper primarily discusses the in vitro testing of inhalable NGPs such as heated tobacco products (HTPs) and vapor products (also known as electronic nicotine delivery system (ENDS) or e-cigarettes (e-cigs)). While other oral products (e.g., Swedish-style smokeless tobacco products (snus) and oral nicotine pouches (ONPs) (Back et al., 2023) are increasingly available, the focus of this review is on inhalable products, so ONPs are out of scope.
HTPs utilize a specifically designed tobacco rod for use in a corresponding device that consists of a heating element, a battery, and a microprocessor controller. For HTPs to yield emissions with drastically reduced levels of harmful and potentially harmful constituents (HPHCs) as compared to cigarette smoke, the heating element should only reach temperatures below those leading to combustion of the tobacco rod (Baker, 1981; Malt et al., 2021; Lang et al., 2023; Sussman et al., 2023). The chemical composition of the resulting aerosol is typically significantly simpler than traditional cigarette smoke, with on average 90%–95% reductions in HPHC levels (Schaller et al., 2016; Forster et al., 2018). ENDS consist of a battery that powers an atomizer (microprocessor and a heating system/coil) to aerosolize an e-liquid (typically containing vegetable glycerin, propylene glycol, to United States Pharmacopoeia (USP) or European Pharmacopoeia (EP) specifications, and food-grade flavors, with or without pharmaceutical-grade nicotine). Compared to cigarette smoke, ENDS aerosols are simpler, with studied ENDS manufactured to a high-quality standard reported to yield emissions with on average 95%–99% reductions in selected HPHCs depending on the analyte assessed (Margham et al., 2016; Lu et al., 2021).
With the increased availability of NGPs and the requirement for scientific studies to support regulatory approval, NAMs may be more suitable for the assessment of such products with less complex emissions than combustible cigarettes. Recently, a number of in vitro toxicological approaches evaluating NGPs compared to cigarette smoke have been applied and reported (see Table 1). The use of a wide variety of different NAMs assays indicating the reduced bioactivity of both HTP and ENDs aerosols indicates the reduced harm potential of these NGPs when compared to cigarettes. However, in order to be able to compare these products against cigarettes, the test materials must be consistently generated and characterized, allowing the resulting in vitro exposures to be translated to human-relevant exposures.
Table 1. Summary of alternative nicotine and tobacco product characteristics and in vitro approaches–Examples from the literature.
The laboratory assessment of NGPs involves multiple test matrices evolved from classical cigarette smoke testing using Health Canada methods to capture the various smoke fractions (Health Canada, 2024). While the test articles should most appropriately mimic the mechanism by which humans are exposed, this is not always technically feasible. The following test articles have been utilized for the assessment of NGPs: 1) aerosol captured mass (ACM), which is equivalent to classical total particulate matter (TPM) capture approaches; 2) gas vapor phase (GVP), which involves filtering the particulate material from the test article, leaving predominately the vapor phase constituents; 3) aqueous trapping approaches, where the aqueous soluble components of the aerosol are captured in an aqueous trap; 4) ACM + GVP, a combination designed to be a proxy for whole aerosol approaches by individually capturing the various phases and recombining into a single test article; and 5) whole aerosol exposure, which often requires specialized equipment to expose cells to freshly generated aerosol and maintain them at an exposure interface (Moore et al., 2023).
Whole aerosol approaches are designed to more appropriately capture the chemical-to-chemical interactions in the various phases of the aerosol and human exposures. When combined with complex co-culture or 3D human constructs, it represents the most physiologically advanced system achievable in vitro (Lacroix et al., 2018). However, not all assays are compatible with aerosol-generating systems (Thorne et al., 2020), and not all laboratories have the capacity to conduct aerosol-based studies. Comprehensive reviews have been published detailing the use of in vitro aerosolization systems and their applications (Thorne and Adamson, 2013; Klus et al., 2016; Li, 2016; Rudd et al., 2020; Cao et al., 2021; Thorne et al., 2021).
Capturing the particulate phase is a traditional, well-documented approach for cigarette smoke in vitro assessment (Baker et al., 2004) and has been used to assess the ACM of HTPs and ENDS (see Table 2), (Misra et al., 2014; Schaller et al., 2016; Takahashi et al., 2018; Thorne et al., 2018; Thorne et al., 2019a; Thorne et al.2019b; Iskandar et al., 2019; Miller-Holt et al., 2023). Aqueous extracts were previously used, but typically for mechanistic studies. These are also well characterized and utilized, but they are limited to the soluble fraction of the aerosol and may preferentially filter for the vapor phase constituents rather than particulates based on solubility (Bozhilova et al., 2020; Taylor et al., 2020). More recently, TPM + GVP has been used as a whole aerosol “proxy” for those occasions where the assay is not compatible with whole-aerosol methodologies or where aerosols are not standardized or available. Such approaches can capture both fractions in a 1:1 ratio and deliver more than just the particulate fraction to the cell cultures (Crooks et al., 2022). More information is available in more detail in a recent aerosol collection methods review (Smart and Phillips, 2021).
A major challenge in evaluating inhalation toxicity is accurate determination of the delivered dose. In humans, breathing is a complex physical process (inhalation-pause-exhalation), and the complex anatomy of the respiratory tract makes it challenging to estimate the delivered doses to the cell surface (Alexander et al., 2008). After a substance is inhaled and deposited in the lung, particles can dissolve and absorb into the systemic/pulmonary circulation. Others are cleared from the lung by pulmonary metabolism or alveolar macrophages, and those deposited higher up in the respiratory tract are removed by mucociliary clearance (Clara et al., 2023).
The exact application of dosimetry measurements also largely depends on the exposure system being used, which should be selected based on the deposition and interaction of particles and vapors at the cell surface. Characterization of the exposure system is key to understanding the delivery of smoke/aerosol to the cell surface (Miller-Holt et al., 2023; Wieczorek et al., 2023). Ideally, dosimetry is the measure of the internal dose, or even the concentration at the molecular target (biologically effective dose) within the target cells for the chemicals of interest (Paustenbach, 2000; Escher and Hermens, 2004; Proença et al., 2021). However, directly measuring cellular dose in submerged cultures poses a significant obstacle to the application of target tissue dosimetry. For example, for nanoparticles and microparticle toxicity assessment, particularly for in vitro systems, due to nanoparticle agglomeration in liquids, which can alter the density of the nano particles has the ability to alter the particle transport and deposition, ultimately altering the dose response relationship (Hinderliter et al., 2010; Watson et al., 2016; Deloid et al., 2017; Thomas et al., 2018). As a consequence, the target tissue paradigm for dosimetry and hazard assessment for nanoparticles has largely been ignored in favor of using alternative indirect methods of potential or surrogate exposure such as μg particle/mL culture medium, particle surface area/mL, or particle number/mL for submerged cultures (Hinderliter et al., 2010).
Air-liquid interface (ALI) exposure is the most physiologically relevant approach to accurately determine both the dose deposited on the cell surface and the dose ultimately available to be absorbed by the cells. The most common methods are deposition of mass onto quartz crystal microbalance and the use of particle counters, photometers, or specialized gas analyzers. For a recent review of recommendations for conducting dosimetry studies in inhalable tobacco products please see the review by Miller-Holt and colleagues (Miller-Holt et al., 2023).
Animal experiments are being strongly scrutinized or entirely replaced (e.g., in the case of the cosmetics industry in certain countries) to respect the guiding principles of the 3Rs to “Replace, Reduce, Refine” in animal testing (Russell and Burch, 1959; van Meer et al., 2015). It is important that 21st Century Toxicity testing provide sufficient data that can be used for read-across purposes and ultimately to reduce animal experiments (Settivari et al., 2015). Traditional in vitro toxicity testing is often based on simple, single endpoints that quantify the global impact of toxicants on cells by determining increased cell permeability or reduced cell viability. However, these endpoints do not provide information on the underlying toxicological mechanism. Technological advances have facilitated the development of a series of high-content screening (HCS) in vitro technologies that offer a wide range of toxicological endpoints, thus increasing predictive value and complementary readouts to current regulatory toxicity testing. The goal of HCS is to provide more mechanistic information faster than traditional approaches, giving more flexibility to assess the growing diverse product landscape in a time and cost-effective manner. In general, the approaches listed below are in line with Tox21 goals (National Research Council, 2007), offering key advantages over traditional toxicity testing. Some of these NAMs have tradeoffs, but all have increased throughput, added mechanistic value, greater human and/or biological relevance, and multiplexing opportunities to maximize tissue and increase information gained. Furthermore, they provide mechanistic insights could also be used to inform AOPs (Wheeldon et al., 2020). Additionally, prior to selecting the appropriate in vitro models, there are a series of question(s) to be first addressed–for example, Figure 1 lists key questions in designing inhalation in vitro testing (Lee et al., 2022; Sharma et al., 2023).
Figure 1. Main considerations when selecting an in vitro model. Reproduced with permission from Lee et al. (2022), Sharma et al. (2023). Note: 1) Test chemical and its physicochemical properties, 2) In vitro exposure system and aerosol characterization, 3) In vitro 2D/3D systems including 2D/3D, 4) cellular types and relevant tissues, and 5) assay endpoints and clinical relevance.
The following summary highlights the strengths and weaknesses of in vitro alternatives to animal testing for inhaled toxicants relevant to tobacco and nicotine products. In particular the focus will be on screening approaches such as HCS, ToxTracker™ and MultiFlow® and respiratory in vitro models including 2D and 3D approaches, use of ex vivo models and organs-on-a-chip (OoC).
HCS enables investigative toxicity testing in vitro to provide knowledge about the affected biologic processes and functions. It generally refers to automated (high-throughput) microscopy, multi-parameter image processing, and visualization to extract quantitative data from cells growing in multi-well cell culture plates. HCS typically uses fluorescent imaging to trace the effect of chemicals on different toxicity pathways including oxidative stress, apoptotic cell death, DNA damage, and mitochondrial health and can be performed in a multiplexed fashion (Gonzalez-Suarez et al., 2016; Taylor et al., 2018; Czekala et al., 2019). In addition, HCS allows morphometric analysis for evaluating toxicant-induced effects on the morphology or size of cells and organelles. All these analyses can be performed with fixed cells or in a time-resolved mode by using live-cell imaging. HCS is also commonly used to monitor spatial (re)distribution of target molecules inside cells, which is pertinent to transcriptional activation following cell stress and inflammatory challenges (Czekala et al., 2019). Similar to flow cytometry, HCS enables information to be collected for multiple endpoints at an individual cell level or from an entire cell population, allowing analysis of dynamic ranges across treated cells in culture.
HCS methodologies are continuously expanding; they are now being applied to two-dimensional (2D) culture systems and three-dimensional (3D) organotypic tissue culture models (Booij et al., 2019). Artificial intelligence adds another level to HCS by allowing predictive in vitro toxicology analysis of new chemicals (Su et al., 2016; Lee et al., 2018).
HCS is generally perceived as a powerful in vitro screening technology for assessing the toxicity and efficacy of chemicals. There are also several associated limitations, especially as the technology is routinely used for screening cells growing in a 2D format:
• Lack of standardization
• Unavailability of specific tracer molecules or antibodies to stain and quantify targets of interest
• Increased data storage requirements
• Lower sensitivity than other quantitative methods (e.g., quantitative polymerase chain reaction)
• Limited applications for 3D image analysis
• Autofluorescence of test items (e.g., TPM) from cigarette smoke
• HCS of 3D organotypic ALI cultures is technically more demanding than for standard 2D culture systems
• Limited markers (e.g., cytotoxicity and H2AX for genotoxicity) have been demonstrated with whole aerosol approaches, but focusing and imaging the ALI can be problematic
Several groups have employed HCS to assess the biological impact of NGP aerosol fractions (Gonzalez-Suarez et al., 2016; Taylor et al., 2018; Czekala et al., 2019). All of these studies demonstrated the utility of HCS as a tool for NGP product assessment. They also provided in vitro evidence for reduced biological impacts of fractions generated from HTPs and other nicotine-containing products compared to those of cigarette smoke fractions. Moreover, complementary test methods, such as those sensitive for oxidative stress toxicity pathways (gene-expression analysis or reporter-gene assays), confirmed the trends observed by HCS. Once qualified, HCS may be applied as a standard platform for modern toxicologic analysis of NGPs.
ToxTracker™ (Toxys, Oegstgeest, the Netherlands) is a high-content assay that employs a series of mouse embryonic stem cell reporter gene cell-lines (Czekala et al., 2021). It consists of six green fluorescent protein (GFP) reporter gene lines plus a control wild-type line, providing readouts on biomarkers for oxidative stress (Srnx1 Nrf2 dependent and Blvrb Nrf2 independent), DNA damage (Bscl2 and Rtkn), cell stress (Btg2), and protein damage/misfolding (Ddit3). ToxTracker has been qualified against >450 known compounds and possesses ≥95% sensitivity and selectivity for both the Ames and in vivo micronucleus assays for mutagenicity and genotoxicity, respectively (Hendriks et al., 2016). ToxTracker could prove particularly valuable as it exhibits good/excellent concordance with classical genotoxicity testing and also offers mechanistic data on mode of action. Accordingly, ToxTracker can be a potential screening tool and/or a follow-up assay to identify mode of action in a positive in vitro response.
Several caveats exist for the application of ToxTracker:
• Although excellent concordance has been shown with classical toxicological approaches, the assay utilizes a mouse (not human) embryonic cell line
• As the assay is based on GFP expression, there are autofluorescence issues when using TPM from cigarette smoke (Johnson et al., 2009)
• It has not yet been combined or demonstrated to be applicable to whole aerosol approaches
• The assay is currently undergoing OECD validation, and there are few data on its use with NGPs
• Limited information exists on the assays ability to deal with complex mixtures where multiple direct and indirect acting chemicals are at play
The in vitro MultiFlow® (Litron Laboratories, Rochester, NY, USA) genotoxicity flow cytometric assay multiplexes several biomarkers that are responsive to diverse forms of DNA damage. The multiplexed biomarkers include: 1) phosphorylation of H2AX at serine 139 to detect double-strand DNA breaks, 2) phosphorylation of histone H3 at serine 10 to identify mitotic cells, 3) nuclear p53 content as an indicator of p53 activation, 4) frequency of 8n + cells to monitor polyploidization, and 5) relative nuclei counts to provide information about treatment-related cytotoxicity (Bryce et al., 2016; Bryce et al., 2017; Dertinger et al., 2019). Some multiplexing adaptations to the methodology have been described for a more integrated genotoxicological approach, such as the combination of the MultiFlow® with a flow-based in vitro micronucleus assays (Smart et al., 2020). The MultiFlow assay has also undergone significant inter-laboratory comparisons (Bryce et al., 2017). Eighty-four chemicals split between aneugen, clastogen, and nongenotoxin groups were collectively compared and cross-analyzed to determine inter-laboratory assay variability. Compared to historical mode of actions for the three class of chemicals, the MultiFlow assay demonstrated ≥92% sensitivity, specificity, and concordance. As a result, an excellent “training” list and established data are available to cross-reference results (Bryce et al., 2017).
Some limitations of MultiFlow include:
• The variability observed in the biomarker endpoints could confound result interpretation in less experienced laboratories. Analyzing multiplexed data requires careful interpretation and consideration.
• It has not been combined or demonstrated to be applicable to whole aerosol approaches
• Limited information exists on the assays ability to deal with mixtures where multiple direct and indirect acting chemicals are at play.
Common in vitro models for studying the biological impact of inhaled toxicants on human airway epithelial cells are based on 2D cell culture systems. Tumor cell lines and immortalized primary epithelial cells (Calu-3, BEAS-2B, 16HBE14o-, NCI-H292, NCI-H441, RERF-LC-AI, and A549) grown in submerged (2D) culture conditions have been frequently used; however, they do not accurately recapitulate the native airway epithelia (Gordon et al., 2015). Although most of them can also be grown at the ALI (Haswell et al., 2010), drawbacks of using these epithelial cell lines for in vitro toxicity studies include their limited metabolic competency that might affect their responsiveness to toxic stimuli (Garcia-Canton et al., 2013), the absence or reduced formation of tight junctions, and their refractoriness to differentiation (Stewart et al., 2012). Non-differentiated primary epithelial cells grown in submerged conditions do not fully reflect native airway epithelia because they either lack the polarized morphology and expression of markers (ion channels) normally found in airway epithelia (Kunzelmann et al., 1996) or show altered responsiveness to solid-particle exposure relative to differentiated primary epithelial cells (Ghio et al., 2013). Due to technical convenience (propagation and possibility for higher throughput), 2D culture systems based on cell lines are most suited for screening purposes to investigate the toxicity of a greater number of test items. Transformation techniques based on hTERT/Cdk4 allowed the generation of immortalized cell lines from primary lung epithelial cells that can differentiate into mucin-producing and ciliated airway models that more closely resemble the lung epithelium (Vaughan et al., 2006).
Reconstituted 3D organotypic culture systems from primary epithelial cells grown at the ALI (Nichols et al., 2013) also replicate the cellular complexity of pseudostratified epithelium found in human airways, containing ciliated/non-ciliated epithelial cells as well as basal (progenitor) cells (Gray et al., 1996; Prytherch et al., 2011). Furthermore, they closely mimic epithelial functionality by means of cilia beating, apical mucus secretion, ion channel expression, and the presence of tight junctions (BeruBe et al., 2009). These models also match the metabolic competency of the native tissue (Iskandar et al., 2013) and are amenable to whole aerosol exposure. The last characteristic can make an important difference regarding the potential underestimation of the biological impact of complex mixtures such as cigarette smoke, because frequently used smoke extracts such as TPM or GVP do not contain the totality of cigarette smoke constituents.
Three-dimensional epithelial cells from different zones of the human aerodigestive tract are commercially available as ready-to-use ALI cultures under brand names such as EpiAirway™ (MatTek Corporation, Ashland, MA, USA) MucilAir™, and SmallAir™ (both from Epithelix Sàrl, Geneva, Switzerland). They can be cultured for weeks to months and may be used for repeated (chronic) exposure (Czekala et al., 2021). The primary alveolar test model systems is increasing in use and commercial availability, with models currently available from suppliers such as Epithelix, ImmuONE (Hatfield, UK), and Invitorlize (Belvaux, Luxembourg). The use of these models is relatively new and beyond the scope of this paper.
Even though 3D organotypic cell cultures closely resemble the native tissue, they usually lack the cellular components of the immune system. More complex model systems have been evaluated as potential candidates for routine aerosol testing (Lehmann et al., 2011; Klein et al., 2013; Marescotti et al., 2019). They use ALI conditions and combine immune cells from the innate immune system or fibroblasts with epithelial cell layers. Attempts to produce functional airway epithelial model systems originating from pluripotent stem cells are also promising and might provide additional mechanistic understanding of aerosol-induced toxicity while also allowing the generation of in vitro models from specific patient populations (Wong et al., 2015). No single in vitro model can answer every study question, so a pragmatic approach should be tailored depending upon the exact hypotheses.
The use of precision-cut lung slices (Behrsing et al., 2013; Sewald and Braun, 2013) and lung-on-chip model systems (Huh et al., 2010; Stucki et al., 2015) resembling additional aspects of the human respiratory tract provide opportunities for characterizing aerosol-induced toxicity in vitro, with an even greater relevance to native tissue and the possibility to emulate pharmacokinetics. Other approaches include using co-cultures of various organ cell types as a cell-based disease screening model (BioMap®) to screen drugs and NGPs for potential adverse effects (Simms et al., 2021).
In vitro organ models have received considerable attention in the attempt to reduce animal experiments and decrease the rate of preclinical failure associated with drug development. Two major advances in the in vitro research field are helping address the weaknesses of the simple models used in the past, rendering them more physiologically relevant and predictive for the effects of compounds in humans: 1) simple 2D cell culture systems are increasingly replaced by organotypic culture systems with 3D architecture, and 2) static culture systems have been switched to dynamic exposure models. The merging of these two developments has culminated in OoC technology, which combines 3D organotypic culture systems (organs) with microfluidic devices (Alepee et al., 2014; Bovard et al., 2017).
Microfluidics enable interconnection of different organ models on the same chip platform by circulating culture medium from a common reservoir. These multi-organ-on-a-chip (MOC) devices permit organ-to-organ crosstalk, which allows compounds to be tested in a more in vivo-like environment (Rogal et al., 2017). To date, long-term, MOC-based co-cultures of different organ combinations have been established for liver spheroids with human 3D lungs (Bovard et al., 2018) or intestine (Maschmeyer et al., 2015a), neuronal (Materne et al., 2015) and pancreatic islet (Bauer et al., 2017) tissue models, as well as a skin–intestine–liver kidney chip (Maschmeyer et al., 2015b). At least two reported OoC systems have been designed for whole aerosol exposure of 3D lung models (Benam et al., 2016; Stucki et al., 2018). However, these platforms are not compatible with smoking machines or the in vitro exposure instruments commonly used in the tobacco industry.
Current OoC or MOC models have potentially improved the human relevance of in vitro assessment of chemicals (see Table 3 for systems strengths and challenges). While there are demonstrated examples in drug candidate profiling and investigative toxicology, their application for NGPs is currently in its infancy.
In summary, there are a number of established alternative in vitro models to animal testing that are more sophisticated than simple cell lines and suitable for investigating the biological impact of inhaled toxicants in an environment more relevant to human exposure. However, model validation remains important for ensuring acceptance by regulatory bodies. Additionally, inter-donor differences are difficult to capture in in vitro aerosol exposure studies, particularly with 3D organotypic ALI exposure, which is usually not sufficiently scalable to test a variety of donors (Mori et al., 2022). The development of a relevant human lung tissue model is of ultimate importance for method standardization; the in vitro model must have high reproducibility and clinical relevance for inhalation toxicity assessment. However, constructing an in vitro lung tissue model derived from human cells currently remains challenging due to the inherent complexity of the human lung and the number of different cell types involved (Petpiroon et al., 2023).
An AOP is defined as “an analytical construct that describes a sequential chain of causally linked events at different levels of biological organization that leads to an adverse health or ecotoxicological effect” as defined by the OECD (OECD, 2023). AOPs are used to help to organize the available mechanistic information relating to an adverse outcome into the key events (KEs) that are required for the adverse outcome, spanning all organizational levels of a biological system(s) (Lowe et al., 2017; Luettich et al., 2017). Key event relationships (KERs) define the relationship between a pair of KEs by showing which is up-/downstream and are supported by both biological plausibility and empirical evidence (Villeneuve et al., 2014). The use of AOPs to organize information into AOP networks has the potential to improve the use of mechanistic data and lead to improved regulatory decision making (Villeneuve et al., 2014). In this way, the use of AOPs can greatly improve the biological understanding of a particular disease process through a simplified series of events. The critical element is causality as the AOP moves from 1 KE to another (i.e., KE 1 always occurs before KE 2, etc.). AOPs can also help link biological exposures to the eventual toxic effects at the population level.
In terms of regulatory context, knowledge of disease mechanisms can guide the design of testing strategies using in vitro methods that can measure or predict KEs relevant to the biological effect of interest. AOPs are not chemical specific, but they link the molecular initiating event (MIE) for a chemical to the apical end point, which is the observable outcome in the whole organism and typically a clinical sign or pathological state (Burden et al., 2015). The well-considered use of AOPs could drive positive changes in toxicology testing, moving toward less reliance on making predictions based on animal models and focusing on the measurement of apical toxicity endpoints (Burden et al., 2015).
The vast majority of in vitro toxicity testing is restricted to one-time (acute) treatment of cells with a test item for a relatively short exposure duration (hours to a few days) before endpoint measurement. Such tests include escalation of the dose to a point where toxic (adverse) effects are either visible at the morphological level or quantifiable by standard cytotoxicity (viability) tests. While dose–response analyses in cell-based assays to determine acute effects on cell cultures are commonly used to rank the toxicity of different chemicals, they have several limitations: 1) the doses for inducing adverse effects are often selected based on potential toxicity hazard that are not relevant for human exposure levels (environmental or use levels); 2) therefore, the toxicity mechanisms observed in vitro, especially after high dosing for eliciting acute toxicity in cell-based assays also may not reflect relevant mechanisms in humans; and 3) they cannot fully predict the effects of subtoxic concentrations of a chemical when administered repeatedly (Ito et al., 2020; Czekala et al., 2021; Chapman et al., 2023). The latter is pertinent, as smoking-related diseases such as chronic obstructive pulmonary disease or cardiovascular diseases only manifest after chronic exposure to cigarette smoke (Yoshida and Tuder, 2007; Luettich et al., 2021; Farcher et al., 2023). Chronic exposure may also result in distinct adverse effects not seen after acute exposure or cause adaptive responses that are not detectable following a single treatment. Adaptations often invoke compensatory repair mechanisms elicited upon chronic stress. These considerations are also relevant for testing inhalable toxicants in vitro and are reflected in a number of published studies on assessing NGPs through repeated exposure of cells, as discussed below.
Long-term (repeated) exposure to subtoxic concentrations of cigarette smoke condensate (CSC) or total particulate matter (TPM) from 3R4F reference cigarettes for up to 12 weeks has been reported to induce an epithelial to mesenchymal transition-like phenotype in BEAS-2B cells, along with anchorage-independent growth of the cells and transient effects on both oxidative stress and DNA damage (Veljkovic et al., 2011; van der Toorn et al., 2018). These effects are only visible when cells are exposed to a much higher concentration of ACM from an NGP. Another study evaluating the short- and long-term effects of TPM on mitochondrial function in BEAS-2B cells (Malinska et al., 2018) revealed a short-term decrease in mitochondrial respiration rate after 1 week of repeated exposure, accompanied by an increase in oxidative stress markers upon 3R4F TPM treatment and cellular adaptation to stress after repeated exposure for 12 weeks. In case of ACM from a NGP, the concentrations required to elicit these effects were again much higher than those derived from reference cigarettes. A similar study on the effects of prolonged exposure to 3R4F cigarette smoke extract (CSE) on BEAS-2B cells reported increased mitochondrial capacity (Hoffmann et al., 2013). These contradictory results may be explained by the different smoke extract used, as well as the applied dose and overall duration of exposure. In a different study, the long-term effects of 1–16 weeks of exposure to nicotine or CSE from 3R4F has been analyzed in BEAS-2B cells (Stabile et al., 2018). In this repeated exposure study, distinct effects of CSE versus pure nicotine were observed when analyzing cell viability and other cellular parameters, including nerve growth factor/receptor gene expression.
Repeated exposure experiments were also extended to differentiated primary human bronchial epithelial organotypic cell cultures, which showed cumulative effects on inflammatory responses and tissue morphology after 1-month repeated exposure to 3R4F TPM (Ito et al., 2018). Interestingly, repeated exposure of bronchial epithelial organotypic cells to 3R4F CSE or TPM during the differentiating phase of the culture had profound effects on cell composition when measured after 28 days; although the number of ciliated cells decreased, those of Clara and goblet cells increased (Haswell et al., 2010; Schamberger et al., 2015).
Several studies evaluated the short-to-long-term effects of subtoxic concentrations of whole cigarette smoke on 3D organotypic ALI tissue cultures reconstituted from human primary epithelial cells, which were tested as either mono- or co-cultures with fibroblasts. The authors reported transition of normal bronchial epithelia towards a metaplastic phenotype and fewer cilia-bearing cells after repeated exposure (4–13 times) to mainstream 3R4F smoke (Aufderheide et al., 2015; Aufderheide et al., 2017). This suggests that the effect on cilia is caused by volatile organic constituents present in mainstream smoke. Similarly, reductions of ciliated, mucus-producing, and club cells were observed when differentiated immortalized primary normal human bronchial epithelial cells were repeatedly exposed to cigarette smoke or e-cigarette vapor (Aufderheide and Emura, 2017). In that study, metaplastic areas positively stained for the basal cell marker cytokeratin-13 were also identified after exposure to both cigarette smoke and e-cigarette vapor. In a co-culture model with ‘omics’ analysis using human 3D bronchial tissue cultures combined with human fibroblasts, the central carbon metabolism in relation to oxidative stress response was perturbed after 21 days of repeated exposure to 3R4F whole smoke; additionally, the epidermal growth factor receptor was identified as a key regulator of perturbed processes (Ishikawa et al., 2019). Repeated exposure (21 days) of differentiating bronchial epithelial 3D organotypic cultures to 3R4F whole smoke, in contrast to repeated exposure to CSE, did not result in an increase in goblet cells (Ishikawa and Ito, 2017).
While in vitro experiments have many advantages over in vivo testing in terms of increased human relevance as presented above, using in vitro data for the purpose of risk assessment (e.g., quantifying the margin of safety) has many challenges, including uncertainty around how to interpret and link exposure or dose. Quantitative in vitro-to-in vivo extrapolation (IVIVE) is an emerging NAM-based computational tool to facilitate this process, generally referred as an extrapolation of (human tissue-derived) cellular (in vitro) data to predict the exposures or outcomes in humans (in vivo). IVIVE implies certain quantitative extrapolations of “dose (or exposure)” and “response” to enable in vitro-based toxicological risk assessment (Zhang et al., 2021; Hines et al., 2022; Zhang and Wright, 2022). Dose extrapolation is necessary to estimate the human exposure or intake level (e.g., daily human equivalent dose) of individual toxicants, commonly by computational (e.g., physiologically-based pharmacokinetic [PBPK]) models and in silico and/or human-based in vitro data. The human equivalent dose can be extrapolated back to the corresponding exposure levels (commonly called “reverse” dosimetry) and compared to measured (environmental) exposures to evaluate the safety margin or guide regulatory safety decision-making. Therefore, one may first define and characterize the in silico or in vitro-based dose–response relationship, and the relevance of the MIEs or KEs can be selected based on the AOPs of interest. Then, using the in vitro dosimetry and disease-relevant response data and with the help of computational modeling, IVIVE enables “human-relevant” safety assessment, moving away from a need for conventional animal toxicity data.
While the concept of IVIVE is gaining interest in scientific communities (Bell et al., 2018; Chang et al., 2021; Hines et al., 2022), it is still an emerging field with limited application to regulatory risk assessment, especially for tobacco and nicotine products. The early successes—mostly from pharmaceutical and environmental safety assessment—typically involve single chemicals, although concerted effort is being made to apply IVIVE to mixtures (Chang et al., 2021; Zhang et al., 2021; Zhang and Wright, 2022). Many of the challenges in using IVIVE for tobacco or nicotine products are similar, including uncertainty in dose selection and quantification (which individual toxicants should be modeled as is or as mixtures in the complex and dynamically unstable aerosol mixtures) and the target test systems (which cellular/tissue systems should be used to reflect what clinically relevant outcomes at cellular levels). Additionally, there is a lack of chemical or mixture-related in vitro data that can be used for IVIVE model development and the independent verification of modeling outcomes.
NAMs are gaining traction as chemical toxicity and biologically relevant assessment tools based on in vitro and in silico (computational) methodologies that support 3R in vivo animal testing traditionally necessary for risk assessment. Significant progress has been made toward the adoption of NAMs for human health and environmental toxicity assessment, although they are not yet fully embraced for routine use in regulatory decision making. This review summarized the current NAMs in use by major tobacco and nicotine product manufacturers. The NAMs were chosen in line with the concepts/criteria displayed in Figure 2, with a focus on the human relevance of NAMs and their potential ability to model and predict key elements of human disease processes.
Figure 2. Key considerations in developing and qualifying NAM tools. Reproduced with permission from van der Zalm et al. (2022). Note: the figures illustrates the five inter-connected elements that are essential in establishing scientific confidence in NAM applications.
As part of the effort to promote NAM applications for use in tobacco and nicotine products, two symposiums were held during the annual Cooperation Centre for Scientific Research Relative to Tobacco (CORESTA) Smoke Science and Product Technology conferences in 2021 and 2023 to introduce the concepts and potential application of NAMs for evaluating NGPs (Lee et al., 2022; Lee et al., 2023). Many of the promises are tangible based on successful case examples demonstrating that NAMs can be a pragmatic and effective approach in terms of cost, time, and resources, in addition to offering enhanced sensitivity for predicting human-relevant health impacts. At the same time, there are ample opportunities to increase confidence in NAM context of use and standardization. Finally, clarity on the degree of validation/qualification by regulatory bodies is required. This last point is essential before NAM-based risk assessments achieve full legitimacy for regulatory risk assessment.
DT: Conceptualization, Writing–original draft, Writing–review and editing. DM: Conceptualization, Writing–review and editing. LS: Conceptualization, Writing–review and editing. KL: Conceptualization, Writing–review and editing. HF: Writing–review and editing. SM: Conceptualization, Writing–review and editing. MG: Conceptualization, Writing–original draft, Writing–review and editing.
The author(s) declare that financial support was received for the research, authorship, and/or publication of this article. All authors were employees of the respective companies in the affiliations and received a salary while working on this manuscript.
The authors would like to thank Stefan Frentzel and Gaddamnugu L Prasad for their inputs to an earlier draft version of the manuscript, Lindsay Reese who supported an editorial review, and the CORESTA Scientific Committee for their support and review.
Authors DT and MG were employed by BAT (Investments) Ltd. Author LS was employed by Imperial Brands. Author KL was employed by Altria Client Services LLC. Author HF was employed by Japan Tobacco Inc., R&D Group. Author DM was employed by Philip Morris International. Author SM was employed by Swedish Match.
All claims expressed in this article are solely those of the authors and do not necessarily represent those of their affiliated organizations, or those of the publisher, the editors and the reviewers. Any product that may be evaluated in this article, or claim that may be made by its manufacturer, is not guaranteed or endorsed by the publisher.
Alepee, N., Bahinski, A., Daneshian, M., De Wever, B., Fritsche, E., Goldberg, A., et al. (2014). State-of-the-art of 3D cultures (organs-on-a-chip) in safety testing and pathophysiology. ALTEX 31, 441–477. doi:10.14573/altex.1406111
Alexander, D. J., Collins, C. J., Coombs, D. W., Gilkison, I. S., Hardy, C. J., Healey, G., et al. (2008). Association of Inhalation Toxicologists (AIT) working party recommendation for standard delivered dose calculation and expression in non-clinical aerosol inhalation toxicology studies with pharmaceuticals. Inhal. Toxicol. 20, 1179–1189. doi:10.1080/08958370802207318
Andersen, M. E., and Krewski, D. (2009). Toxicity testing in the 21st century: bringing the vision to life. Toxicol. Sci. 107, 324–330. doi:10.1093/toxsci/kfn255
Attene-Ramos, M. S., Miller, N., Huang, R., Michael, S., Itkin, M., Kavlock, R. J., et al. (2013). The Tox21 robotic platform for the assessment of environmental chemicals--from vision to reality. Drug Discov. Today 18, 716–723. doi:10.1016/j.drudis.2013.05.015
Aufderheide, M., and Emura, M. (2017). Phenotypical changes in a differentiating immortalized bronchial epithelial cell line after exposure to mainstream cigarette smoke and e-cigarette vapor. Exp. Toxicol. Pathol. 69, 393–401. doi:10.1016/j.etp.2017.03.004
Aufderheide, M., Ito, S., Ishikawa, S., and Emura, M. (2017). Metaplastic phenotype in human primary bronchiolar epithelial cells after repeated exposure to native mainstream smoke at the air-liquid interface. Exp. Toxicol. Pathol. 69, 307–315. doi:10.1016/j.etp.2017.01.015
Aufderheide, M., Scheffler, S., Ito, S., Ishikawa, S., and Emura, M. (2015). Ciliatoxicity in human primary bronchiolar epithelial cells after repeated exposure at the air-liquid interface with native mainstream smoke of K3R4F cigarettes with and without charcoal filter. Exp. Toxicol. Pathol. 67, 407–411. doi:10.1016/j.etp.2015.04.006
Back, S., Masser, A. E., Rutqvist, L. E., and Lindholm, J. (2023). Harmful and potentially harmful constituents (HPHCs) in two novel nicotine pouch products in comparison with regular smokeless tobacco products and pharmaceutical nicotine replacement therapy products (NRTs). BMC Chem. 17, 9. doi:10.1186/s13065-023-00918-1
Baker, R. R. (1981). Product formation mechanisms inside a burning cigarette. Prog. Energy Combust. Sci. 7, 135–153. doi:10.1016/0360-1285(81)90008-3
Baker, R. R., Massey, E. D., and Smith, G. (2004). An overview of the effects of tobacco ingredients on smoke chemistry and toxicity. Food Chem. Toxicol. 42, 53–83. doi:10.1016/j.fct.2004.01.001
Bauer, S., Wennberg Huldt, C., Kanebratt, K. P., Durieux, I., Gunne, D., Andersson, S., et al. (2017). Functional coupling of human pancreatic islets and liver spheroids on-a-chip: towards a novel human ex vivo type 2 diabetes model. Sci. Rep. 7, 14620. doi:10.1038/s41598-017-14815-w
Behrsing, H. P., Furniss, M. J., Davis, M., Tomaszewski, J. E., and Parchment, R. E. (2013). In vitro exposure of precision-cut lung slices to 2-(4-amino-3-methylphenyl)-5-fluorobenzothiazole lysylamide dihydrochloride (NSC 710305, Phortress) increases inflammatory cytokine content and tissue damage. Toxicol. Sci. 131, 470–479. doi:10.1093/toxsci/kfs319
Bell, S. M., Chang, X., Wambaugh, J. F., Allen, D. G., Bartels, M., Brouwer, K. L. R., et al. (2018). In vitro to in vivo extrapolation for high throughput prioritization and decision making. Toxicol. Vitro 47, 213–227. doi:10.1016/j.tiv.2017.11.016
Benam, K. H., Villenave, R., Lucchesi, C., Varone, A., Hubeau, C., Lee, H. H., et al. (2016). Small airway-on-a-chip enables analysis of human lung inflammation and drug responses in vitro. Nat. Methods 13, 151–157. doi:10.1038/nmeth.3697
Berube, K., Aufderheide, M., Breheny, D., Clothier, R., Combes, R., Duffin, R., et al. (2009). In vitro models of inhalation toxicity and disease. The report of a FRAME workshop. Altern. Lab. Anim. 37, 89–141.
Bishop, E., Breheny, D., Hewitt, K., Taylor, M., Jaunky, T., Camacho, O. M., et al. (2020). Evaluation of a high-throughput in vitro endothelial cell migration assay for the assessment of nicotine and tobacco delivery products. Toxicol. Lett. 334, 110–116. doi:10.1016/j.toxlet.2020.07.011
Bishop, E., East, N., Miazzi, F., Fiebelkorn, S., Breheny, D., Gaca, M., et al. (2023). A contextualised e-cigarette testing strategy shows flavourings do not impact lung toxicity in vitro. Toxicol. Lett. 380, 1–11. doi:10.1016/j.toxlet.2023.03.006
Booij, T. H., Price, L. S., and Danen, E. H. J. (2019). 3D cell-based assays for drug screens: challenges in imaging, image analysis, and high-content analysis. SLAS Discov. 24, 615–627. doi:10.1177/2472555219830087
Bovard, D., Iskandar, A., Luettich, K., Hoeng, J., and Peitsch, M. (2017). Organs-on-a-chip: new in vitro tools multi-organ-on-a-chip challenges, limitations, and future prospects for fluidic devices. Toxicol. Res. Appl. 1, 1–16. doi:10.1177/2397847317726351
Bovard, D., Sandoz, A., Luettich, K., Frentzel, S., Iskandar, A., Marescotti, D., et al. (2018). A lung/liver-on-a-chip platform for acute and chronic toxicity studies. Lab. Chip 18, 3814–3829. doi:10.1039/c8lc01029c
Bozhilova, S., Baxter, A., Bishop, E., Breheny, D., Thorne, D., Hodges, P., et al. (2020). Optimization of aqueous aerosol extract (AqE) generation from e-cigarettes and tobacco heating products for in vitro cytotoxicity testing. Toxicol. Lett. 335, 51–63. doi:10.1016/j.toxlet.2020.10.005
Bryce, S. M., Bernacki, D. T., Bemis, J. C., and Dertinger, S. D. (2016). Genotoxic mode of action predictions from a multiplexed flow cytometric assay and a machine learning approach. Environ. Mol. Mutagen. 57, 171–189. doi:10.1002/em.21996
Bryce, S. M., Bernacki, D. T., Bemis, J. C., Spellman, R. A., Engel, M. E., Schuler, M., et al. (2017). Interlaboratory evaluation of a multiplexed high information content in vitro genotoxicity assay. Environ. Mol. Mutagen. 58, 146–161. doi:10.1002/em.22083
Buchanan, C. F., Verbridge, S. S., Vlachos, P. P., and Rylander, M. N. (2014). Flow shear stress regulates endothelial barrier function and expression of angiogenic factors in a 3D microfluidic tumor vascular model. Cell. adh. Migr. 8, 517–524. doi:10.4161/19336918.2014.970001
Burden, N., Sewell, F., Andersen, M. E., Boobis, A., Chipman, J. K., Cronin, M. T., et al. (2015). Adverse Outcome Pathways can drive non-animal approaches for safety assessment. J. Appl. Toxicol. 35, 971–975. doi:10.1002/jat.3165
Cao, X., Coyle, J. P., Xiong, R., Wang, Y., Heflich, R. H., Ren, B., et al. (2021). Invited review: human air-liquid-interface organotypic airway tissue models derived from primary tracheobronchial epithelial cells—overview and perspectives. Vitro Cell. Dev. Biol. Anim. 57, 104–132. doi:10.1007/s11626-020-00517-7
Caruso, M., Distefano, A., Emma, R., Zuccarello, P., Copat, C., Ferrante, M., et al. (2023). In vitro cytoxicity profile of e-cigarette liquid samples on primary human bronchial epithelial cells. Drug Test. Anal. 15, 1145–1155. doi:10.1002/dta.3275
Chang, X., Abedini, J., Bell, S., and Lee, K. M. (2021). Exploring in vitro to in vivo extrapolation for exposure and health impacts of e-cigarette flavor mixtures. Toxicol. Vitro 72, 105090. doi:10.1016/j.tiv.2021.105090
Chapman, F., Pour, S. J., Wieczorek, R., Trelles Sticken, E., Budde, J., Rower, K., et al. (2023). Twenty-eight day repeated exposure of human 3D bronchial epithelial model to heated tobacco aerosols indicates decreased toxicological responses compared to cigarette smoke. Front. Toxicol. 5, 1076752. doi:10.3389/ftox.2023.1076752
Clara, P. C., Jerez, F. R., Ramirez, J. B., and Gonzalez, C. M. (2023). Deposition and clinical impact of inhaled particles in the lung. Arch. Bronconeumol. 59, 377–382. doi:10.1016/j.arbres.2023.01.016
Crooks, I., Hollings, M., Leverette, R., Jordan, K., Breheny, D., Moore, M. M., et al. (2022). A comparison of cigarette smoke test matrices and their responsiveness in the mouse lymphoma assay: a case study. Mutat. Res. Genet. Toxicol. Environ. Mutagen. 879-880, 503502. doi:10.1016/j.mrgentox.2022.503502
Crooks, I., Neilson, L., Scott, K., Reynolds, L., Oke, T., Forster, M., et al. (2018). Evaluation of flavourings potentially used in a heated tobacco product: chemical analysis, in vitro mutagenicity, genotoxicity, cytotoxicity and in vitro tumour promoting activity. Food Chem. Toxicol. 118, 940–952. doi:10.1016/j.fct.2018.05.058
Czekala, L., Simms, L., Stevenson, M., Trelles-Sticken, E., Walker, P., and Walele, T. (2019). High Content Screening in NHBE cells shows significantly reduced biological activity of flavoured e-liquids, when compared to cigarette smoke condensate. Toxicol. Vitro 58, 86–96. doi:10.1016/j.tiv.2019.03.018
Czekala, L., Wieczorek, R., Simms, L., Yu, F., Budde, J., Trelles Sticken, E., et al. (2021). Multi-endpoint analysis of human 3D airway epithelium following repeated exposure to whole electronic vapor product aerosol or cigarette smoke. Curr. Res. Toxicol. 2, 99–115. doi:10.1016/j.crtox.2021.02.004
Daston, G., Knight, D. J., Schwarz, M., Gocht, T., Thomas, R. S., Mahony, C., et al. (2015). SEURAT: safety evaluation ultimately replacing animal testing--recommendations for future research in the field of predictive toxicology. Arch. Toxicol. 89, 15–23. doi:10.1007/s00204-014-1421-5
DeLoid, G., Cohen, J., Pyrgiotakis, G., and Demokritou, P. (2017). Preparation, characterization, and in vitro dosimetry of dispersed, engineered nanomaterials. Nat. Protoc. 12, 355–371. doi:10.1038/nprot.2016.172
Dertinger, S. D., Kraynak, A. R., Wheeldon, R. P., Bernacki, D. T., Bryce, S. M., Hall, N., et al. (2019). Predictions of genotoxic potential, mode of action, molecular targets, and potency via a tiered multiflow® assay data analysis strategy. Environ. Mol. Mutagen. 60, 513–533. doi:10.1002/em.22274
Ennever, F. K., and Lave, L. B. (2003). Implications of the lack of accuracy of the lifetime rodent bioassay for predicting human carcinogenicity. Regul. Toxicol. Pharmacol. 38, 52–57. doi:10.1016/s0273-2300(03)00068-0
Escher, B. I., and Hermens, J. L. (2004). Internal exposure: linking bioavailability to effects. Environ. Sci. Technol. 38, 455A–462A. doi:10.1021/es0406740
Farcher, R., Syleouni, M. E., Vinci, L., and Mattli, R. (2023). Burden of smoking on disease-specific mortality, DALYs, costs: the case of a high-income European country. BMC Public Health 23, 698. doi:10.1186/s12889-023-15535-9
Forster, M., Fiebelkorn, S., Yurteri, C., Mariner, D., Liu, C., Wright, C., et al. (2018). Assessment of novel tobacco heating product THP1.0. Part 3: comprehensive chemical characterisation of harmful and potentially harmful aerosol emissions. Regul. Toxicol. Pharmacol. 93, 14–33. doi:10.1016/j.yrtph.2017.10.006
Garcia-Canton, C., Minet, E., Anadon, A., and Meredith, C. (2013). Metabolic characterization of cell systems used in in vitro toxicology testing: lung cell system BEAS-2B as a working example. Toxicol. Vitro 27, 1719–1727. doi:10.1016/j.tiv.2013.05.001
Ghio, A. J., Dailey, L. A., Soukup, J. M., Stonehuerner, J., Richards, J. H., and Devlin, R. B. (2013). Growth of human bronchial epithelial cells at an air-liquid interface alters the response to particle exposure. Part. Fibre Toxicol. 10, 25. doi:10.1186/1743-8977-10-25
Gonzalez-Suarez, I., Martin, F., Marescotti, D., Guedj, E., Acali, S., Johne, S., et al. (2016). In vitro systems toxicology assessment of a candidate modified risk tobacco product shows reduced toxicity compared to that of a conventional cigarette. Chem. Res. Toxicol. 29, 3–18. doi:10.1021/acs.chemrestox.5b00321
Gordon, S., Daneshian, M., Bouwstra, J., Caloni, F., Constant, S., Davies, D. E., et al. (2015). Non-animal models of epithelial barriers (skin, intestine and lung) in research, industrial applications and regulatory toxicology. ALTEX 32, 327–378. doi:10.14573/altex.1510051
Gray, T. E., Guzman, K., Davis, C. W., Abdullah, L. H., and Nettesheim, P. (1996). Mucociliary differentiation of serially passaged normal human tracheobronchial epithelial cells. Am. J. Respir. Cell Mol. Biol. 14, 104–112. doi:10.1165/ajrcmb.14.1.8534481
Hashizume, T., Ishikawa, S., Matsumura, K., Ito, S., and Fukushima, T. (2023). Chemical and in vitro toxicological comparison of emissions from a heated tobacco product and the 1R6F reference cigarette. Toxicol. Rep. 10, 281–292. doi:10.1016/j.toxrep.2023.02.005
Haswell, L. E., Hewitt, K., Thorne, D., Richter, A., and Gaca, M. D. (2010). Cigarette smoke total particulate matter increases mucous secreting cell numbers in vitro: a potential model of goblet cell hyperplasia. Toxicol. Vitro 24, 981–987. doi:10.1016/j.tiv.2009.12.019
Health Canada (2024). Health Canada. Health Canada official methods for the testing of tobacco products (mainstream smoke). Available at: https://open.canada.ca/data/en/dataset/71394df3-aee8-4117-92cb-a035360972a2 (Accessed January 2, 2024).
Hendriks, G., Derr, R. S., Misovic, B., Morolli, B., Calleja, F. M., and Vrieling, H. (2016). The extended ToxTracker assay discriminates between induction of DNA damage, oxidative stress, and protein misfolding. Toxicol. Sci. 150, 190–203. doi:10.1093/toxsci/kfv323
Hinderliter, P. M., Minard, K. R., Orr, G., Chrisler, W. B., Thrall, B. D., Pounds, J. G., et al. (2010). ISDD: a computational model of particle sedimentation, diffusion and target cell dosimetry for in vitro toxicity studies. Part. Fibre Toxicol. 7, 36–20. doi:10.1186/1743-8977-7-36
Hines, D. E., Bell, S., Chang, X., Mansouri, K., Allen, D., and Kleinstreuer, N. (2022). Application of an accessible interface for pharmacokinetic modeling and in vitro to in vivo extrapolation. Front. Pharmacol. 13, 864742. doi:10.3389/fphar.2022.864742
Hoffmann, R. F., Zarrintan, S., Brandenburg, S. M., Kol, A., De Bruin, H. G., Jafari, S., et al. (2013). Prolonged cigarette smoke exposure alters mitochondrial structure and function in airway epithelial cells. Respir. Res. 14, 97. doi:10.1186/1465-9921-14-97
Huh, D., Matthews, B. D., Mammoto, A., Montoya-Zavala, M., Hsin, H. Y., and Ingber, D. E. (2010). Reconstituting organ-level lung functions on a chip. Science 328, 1662–1668. doi:10.1126/science.1188302
Interagency Coordinating Committee on the Validation of Alternative Methods (ICCVAM) (2023). Validation, qualification, and regulatory acceptance of new approach methodologies. Available at: https://ntp.niehs.nih.gov/sites/default/files/2023-08/VWG%20Report%20Draft_for%20public%20comment_08Aug2023.pdf (Accessed December 12, 2023).
Interagency Coordinating Committee on the Validation of Alternative Methods (ICVAM) (2018). A strategic roadmap for establishing new approaches to evaluate the safety of chemicals and medical products in the United States, Available at: https://ntp.niehs.nih.gov/iccvam/docs/roadmap/iccvam_strategicroadmap_january2018_document_508.pdf [Accessed December 12, 2023].
Ishikawa, S., and Ito, S. (2017). Repeated whole cigarette smoke exposure alters cell differentiation and augments secretion of inflammatory mediators in air-liquid interface three-dimensional co-culture model of human bronchial tissue. Toxicol. Vitro 38, 170–178. doi:10.1016/j.tiv.2016.09.004
Ishikawa, S., Matsumura, K., Kitamura, N., Takanami, Y., and Ito, S. (2019). Multi-omics analysis: repeated exposure of a 3D bronchial tissue culture to whole-cigarette smoke. Toxicol. Vitro 54, 251–262. doi:10.1016/j.tiv.2018.10.001
Iskandar, A. R., Martin, F., Talikka, M., Schlage, W. K., Kostadinova, R., Mathis, C., et al. (2013). Systems approaches evaluating the perturbation of xenobiotic metabolism in response to cigarette smoke exposure in nasal and bronchial tissues. Biomed. Res. Int. 2013, 512086. doi:10.1155/2013/512086
Iskandar, A. R., Zanetti, F., Marescotti, D., Titz, B., Sewer, A., Kondylis, A., et al. (2019). Application of a multi-layer systems toxicology framework for in vitro assessment of the biological effects of Classic Tobacco e-liquid and its corresponding aerosol using an e-cigarette device with MESH technology. Arch. Toxicol. 93, 3229–3247. doi:10.1007/s00204-019-02565-9
Ito, S., Ishimori, K., and Ishikawa, S. (2018). Effects of repeated cigarette smoke extract exposure over one month on human bronchial epithelial organotypic culture. Toxicol. Rep. 5, 864–870. doi:10.1016/j.toxrep.2018.08.015
Ito, S., Matsumura, K., Ishimori, K., and Ishikawa, S. (2020). In vitro long-term repeated exposure and exposure switching of a novel tobacco vapor product in a human organotypic culture of bronchial epithelial cells. J. Appl. Toxicol. 40, 1248–1258. doi:10.1002/jat.3982
Ito, S., Taylor, M., Mori, S., Thorne, D., Nishino, T., Breheny, D., et al. (2019). An inter-laboratory in vitro assessment of cigarettes and next generation nicotine delivery products. Toxicol. Lett. 315, 14–22. doi:10.1016/j.toxlet.2019.08.004
Jaunky, T., Adamson, J., Santopietro, S., Terry, A., Thorne, D., Breheny, D., et al. (2018). Assessment of tobacco heating product THP1.0. Part 5: in vitro dosimetric and cytotoxic assessment. Regul. Toxicol. Pharmacol. 93, 52–61. doi:10.1016/j.yrtph.2017.09.016
Johnson, M. D., Schilz, J., Djordjevic, M. V., Rice, J. R., and Shields, P. G. (2009). Evaluation of in vitro assays for assessing the toxicity of cigarette smoke and smokeless tobacco. Cancer Epidemiol. Biomarkers Prev. 18, 3263–3304. doi:10.1158/1055-9965.EPI-09-0965
Justice, M. J., and Dhillon, P. (2016). Using the mouse to model human disease: increasing validity and reproducibility. Dis. Model Mech. 9, 101–103. doi:10.1242/dmm.024547
Klein, S. G., Serchi, T., Hoffmann, L., Blomeke, B., and Gutleb, A. C. (2013). An improved 3D tetraculture system mimicking the cellular organisation at the alveolar barrier to study the potential toxic effects of particles on the lung. Part. Fibre Toxicol. 10, 31. doi:10.1186/1743-8977-10-31
Klus, H., Boenke-Nimphius, B., and Müller, L. (2016). Cigarette mainstream smoke: the evolution of methods and devices for generation, exposure and collection. Contrib. Tob. Nicotine Res. 27, 137–274. doi:10.1515/cttr-2016-0015
Kunzelmann, K., Kathofer, S., Hipper, A., Gruenert, D. C., and Gregner, R. (1996). Culture-dependent expression of Na+ conductances in airway epithelial cells. Pflugers Arch. 431, 578–586. doi:10.1007/BF02191906
Lacroix, G., Koch, W., Ritter, D., Gutleb, A., Larsen, S. T., Loret, T., et al. (2018). Air–liquid interface in vitro models for respiratory toxicology research: consensus workshop and recommendations. Appl. Vitro Toxicol. 4, 91–106. doi:10.1089/aivt.2017.0034
Lang, G., Henao, C., Almstetter, M., Arndt, D., Goujon, C., and Maeder, S. (2023). Non-targeted analytical comparison of a heated tobacco product aerosol against mainstream cigarette smoke: does heating tobacco produce an inherently different set of aerosol constituents? Anal. Bioanal. Chem. 416, 1349–1361. doi:10.1007/s00216-024-05126-x
Lee, J. J., Miller, J. A., Basu, S., Kee, T. V., and Loo, L. H. (2018). Building predictive in vitro pulmonary toxicity assays using high-throughput imaging and artificial intelligence. Arch. Toxicol. 92, 2055–2075. doi:10.1007/s00204-018-2213-0
Lee, K. M., Corley, R., Jarabek, A. M., Kleinstreuer, N., Paini, A., Stucki, A. O., et al. (2022). Advancing new approach methodologies (NAMs) for tobacco harm reduction: synopsis from the 2021 CORESTA SSPT-NAMs symposium. Toxics 10, 760. doi:10.3390/toxics10120760
Lee, K. M., Pour, S. J., Zhang, J., Keyser, B., Iskandar, A., Ito, S., et al. (2023). New approach methods (NAMs) symposium-II: applications in tobacco regulatory sciences, the 2023 CORESTA SSPT symposium. Available at: https://www.coresta.org/sites/default/files/events/CORESTA_SSPT2023-NAM-Symposium_20231013_final.pdf (Accessed January 22, 2024).
Lehmann, A. D., Daum, N., Bur, M., Lehr, C. M., Gehr, P., and Rothen-Rutishauser, B. M. (2011). An in vitro triple cell co-culture model with primary cells mimicking the human alveolar epithelial barrier. Eur. J. Pharm. Biopharm. 77, 398–406. doi:10.1016/j.ejpb.2010.10.014
Li, X. (2016). In vitro toxicity testing of cigarette smoke based on the air-liquid interface exposure: a review. Toxicol. Vitro 36, 105–113. doi:10.1016/j.tiv.2016.07.019
Lowe, F. J., Luettich, K., Talikka, M., Hoang, V., Haswell, L. E., Hoeng, J., et al. (2017). Development of an adverse outcome pathway for the onset of hypertension by oxidative stress-mediated perturbation of endothelial nitric oxide bioavailability. Appl. Vitro Toxicol. 3, 131–148. doi:10.1089/aivt.2016.0031
Lu, F., Yu, M., Chen, C., Liu, L., Zhao, P., Shen, B., et al. (2021). The emission of VOCs and CO from heated tobacco products, electronic cigarettes, and conventional cigarettes, and their health risk. Toxics 10, 8. doi:10.3390/toxics10010008
Luettich, K., Sharma, M., Yepiskoposyan, H., Breheny, D., and Lowe, F. J. (2021). An adverse outcome pathway for decreased lung function focusing on mechanisms of impaired mucociliary clearance following inhalation exposure. Front. Toxicol. 3, 750254. doi:10.3389/ftox.2021.750254
Luettich, K., Talikka, M., Lowe, F. J., Haswell, L. E., Park, J., Gaca, M. D., et al. (2017). The adverse outcome pathway for oxidative stress-mediated EGFR activation leading to decreased lung function. Appl. Vitro Toxicol. 3, 99–109. doi:10.1089/aivt.2016.0032
Malinska, D., Szymanski, J., Patalas-Krawczyk, P., Michalska, B., Wojtala, A., Prill, M., et al. (2018). Assessment of mitochondrial function following short- and long-term exposure of human bronchial epithelial cells to total particulate matter from a candidate modified-risk tobacco product and reference cigarettes. Food Chem. Toxicol. 115, 1–12. doi:10.1016/j.fct.2018.02.013
Malt, L., Thompson, K., Mason, E., Walele, T., Nahde, T., and O'Connell, G. (2022). The product science of electrically heated tobacco products: a narrative review of the scientific literature. F1000Research 11, 121. doi:10.12688/f1000research.74718.1
Mansouri, K., Karmaus, A. L., Fitzpatrick, J., Patlewicz, G., Pradeep, P., Alberga, D., et al. (2021). CATMoS: collaborative acute toxicity modeling suite. Environ. Health Perspect. 129 (4), 47013. doi:10.1289/EHP8495
Marescotti, D., Serchi, T., Luettich, K., Xiang, Y., Moschini, E., Talikka, M., et al. (2019). How complex should an in vitro model be? Evaluation of complex 3D alveolar model with transcriptomic data and computational biological network models. ALTEX 36, 388–402. doi:10.14573/altex.1811221
Margham, J., Mcadam, K., Forster, M., Liu, C., Wright, C., Mariner, D., et al. (2016). Chemical composition of aerosol from an E-cigarette: a quantitative comparison with cigarette smoke. Chem. Res. Toxicol. 29, 1662–1678. doi:10.1021/acs.chemrestox.6b00188
Maschmeyer, I., Hasenberg, T., Jaenicke, A., Lindner, M., Lorenz, A. K., Zech, J., et al. (2015a). Chip-based human liver-intestine and liver-skin co-cultures--A first step toward systemic repeated dose substance testing in vitro. Eur. J. Pharm. Biopharm. 95, 77–87. doi:10.1016/j.ejpb.2015.03.002
Maschmeyer, I., Lorenz, A. K., Schimek, K., Hasenberg, T., Ramme, A. P., Hubner, J., et al. (2015b). A four-organ-chip for interconnected long-term co-culture of human intestine, liver, skin and kidney equivalents. Lab. Chip 15, 2688–2699. doi:10.1039/c5lc00392j
Materne, E. M., Ramme, A. P., Terrasso, A. P., Serra, M., Alves, P. M., Brito, C., et al. (2015). A multi-organ chip co-culture of neurospheres and liver equivalents for long-term substance testing. J. Biotechnol. 205, 36–46. doi:10.1016/j.jbiotec.2015.02.002
McNeil, A., Simonavičius, E., Brose, L. S., Taylor, E., East, K., Zuikova, E., et al. (2022) Nicotine vaping in England: an evidence update including health risks and perceptions, September 2022. A report commissioned by the Office for Health Improvement and Disparities. London: Office for Health Improvement and Disparities.
McNeill, A., Brose, L. S., Calder, R., and Bauld, L. (2018) Evidence review of e-cigarettes and heated tobacco products 2018. A report commissioned by Public Health England. London: Public Health England.
Miller-Holt, J., Behrsing, H., Crooks, I., Curren, R., Demir, K., Gafner, J., et al. (2023). Key challenges for in vitro testing of tobacco products for regulatory applications: recommendations for dosimetry. Drug Test. Anal. 15, 1175–1188. doi:10.1002/dta.3344
Misra, M., Leverette, R. D., Cooper, B. T., Bennett, M. B., and Brown, S. E. (2014). Comparative in vitro toxicity profile of electronic and tobacco cigarettes, smokeless tobacco and nicotine replacement therapy products: E-liquids, extracts and collected aerosols. Int. J. Environ. Res. Public Health. 11, 11325–11347. doi:10.3390/ijerph111111325
Moore, M. M., Abraham, I., Ballantyne, M., Behrsing, H., Cao, X., Clements, J., et al. (2023). Key challenges and recommendations for in vitro testing of tobacco products for regulatory applications: consideration of test materials and exposure parameters. Altern. Lab. Anim. 51, 55–79. doi:10.1177/02611929221146536
Mori, S., Ishimori, K., Matsumura, K., Ishikawa, S., and Ito, S. (2022). Donor-to-donor variability of a human three-dimensional bronchial epithelial model: a case study of cigarette smoke exposure. Toxicol. Vitro 82, 105391. doi:10.1016/j.tiv.2022.105391
National Research Council (NRC) (2007) Toxicity testing in the 21st century: a vision and a strategy. Available at: https://nap.nationalacademies.org/catalog/11970/toxicity-testing-in-the-21st-century-a-vision-and-a (Accessed December 12, 2023).
Nichols, J. E., Niles, J. A., Vega, S. P., and Cortiella, J. (2013). Novel in vitro respiratory models to study lung development, physiology, pathology and toxicology. Stem Cell Res. Ther. 4 (1), S7. doi:10.1186/scrt368
Ohashi, K., Hayashida, A., Nozawa, A., Matsumura, K., and Ito, S. (2023). Human vasculature-on-a-chip with macrophage-mediated endothelial activation: the biological effect of aerosol from heated tobacco products on monocyte adhesion. Toxicol. Vitro 89, 105582. doi:10.1016/j.tiv.2023.105582
Organisation for Economic Co-operation and Development (OECD) (2023). Welcome to the collaborative adverse outcome pathway wiki (AOP-Wiki). Available at: https://aopwiki.org/(Accessed December 12, 2023).
Paustenbach, D. J. (2000). The practice of exposure assessment: a state-of-the-art review. J. Toxicol. Environ. Health B Crit. Rev. 3, 179–291. doi:10.1080/10937400050045264
Perlman, R. L. (2016). Mouse models of human disease: an evolutionary perspective. Evol. Med. Public Health 2016, 170–176. doi:10.1093/emph/eow014
Petpiroon, N., Netkueakul, W., Sukrak, K., Wang, C., Liang, Y., Wang, M., et al. (2023). Development of lung tissue models and their applications. Life Sci. 334, 122208. doi:10.1016/j.lfs.2023.122208
Poussin, C., Laurent, A., Peitsch, M. C., Hoeng, J., and De Leon, H. (2016). Systems toxicology-based assessment of the candidate modified risk tobacco product THS2.2 for the adhesion of monocytic cells to human coronary arterial endothelial cells. Toxicology 339, 73–86. doi:10.1016/j.tox.2015.11.007
Proença, S., Escher, B. I., Fischer, F. C., Fisher, C., Grégoire, S., Hewitt, N. J., et al. (2021). Effective exposure of chemicals in in vitro cell systems: a review of chemical distribution models. Toxicol. Vitro 73, 105133. doi:10.1016/j.tiv.2021.105133
Prytherch, Z., Job, C., Marshall, H., Oreffo, V., Foster, M., and Berube, K. (2011). Tissue-Specific stem cell differentiation in an in vitro airway model. Macromol. Biosci. 11, 1467–1477. doi:10.1002/mabi.201100181
Rogal, J., Probst, C., and Loskill, P. (2017). Integration concepts for multi-organ chips: how to maintain flexibility? Future Sci. OA 3, FSO180. doi:10.4155/fsoa-2016-0092
Rudd, K., Stevenson, M., Wieczorek, R., Pani, J., Trelles-Sticken, E., Dethloff, O., et al. (2020). Chemical composition and in vitro toxicity profile of a pod-based E-cigarette aerosol compared to cigarette smoke. Appl. Vitro Toxicol. 6, 11–41. doi:10.1089/aivt.2019.0015
Russell, W. M. S., and Burch, R. L. (1959) The principles of humane experimental technique. Massachusetts: Methuen.
Schaller, J. P., Keller, D., Poget, L., Pratte, P., Kaelin, E., Mchugh, D., et al. (2016). Evaluation of the Tobacco Heating System 2.2. Part 2: chemical composition, genotoxicity, cytotoxicity, and physical properties of the aerosol. Regul. Toxicol. Pharmacol. 81 (2), S27–S47. doi:10.1016/j.yrtph.2016.10.001
Schamberger, A. C., Staab-Weijnitz, C. A., Mise-Racek, N., and Eickelberg, O. (2015). Cigarette smoke alters primary human bronchial epithelial cell differentiation at the air-liquid interface. Sci. Rep. 5, 8163. doi:10.1038/srep08163
Settivari, R. S., Ball, N., Murphy, L., Rasoulpour, R., Boverhof, D. R., and Carney, E. W. (2015). Predicting the future: opportunities and challenges for the chemical industry to apply 21st-century toxicity testing. J. Am. Assoc. Lab. Anim. Sci. 54, 214–223.
Sewald, K., and Braun, A. (2013). Assessment of immunotoxicity using precision-cut tissue slices. Xenobiotica 43, 84–97. doi:10.3109/00498254.2012.731543
Sharma, M., Stucki, A. O., Verstraelen, S., Stedeford, T. J., Jacobs, A., Maes, F., et al. (2023). Human cell-based in vitro systems to assess respiratory toxicity: a case study using silanes. Toxicol. Sci. 195 (2), 213–230. doi:10.1093/toxsci/kfad074
Silva, R. J., and Tamburic, S. (2022). A state-of-the-art review on the alternatives to animal testing for the safety assessment of cosmetics. Cosmetics 9, 90. doi:10.3390/cosmetics9050090
Simms, L., Clarke, A., Paschke, T., Manson, A., Murphy, J., Stabbert, R., et al. (2019). Assessment of priority tobacco additives per the requirements of the EU Tobacco Products Directive (2014/40/EU): Part 1: background, approach, and summary of findings. Regul. Toxicol. Pharmacol. 104, 84–97. doi:10.1016/j.yrtph.2019.02.011
Simms, L., Mason, E., Berg, E. L., Yu, F., Rudd, K., Czekala, L., et al. (2021). Use of a rapid human primary cell-based disease screening model, to compare next generation products to combustible cigarettes. Curr. Res. Toxicol. 2, 309–321. doi:10.1016/j.crtox.2021.08.003
Smart, D. J., Helbling, F. R., Verardo, M., Huber, A., Mchugh, D., and Vanscheeuwijck, P. (2020). Development of an integrated assay in human TK6 cells to permit comprehensive genotoxicity analysis in vitro. Mutat. Res. Genet. Toxicol. Environ. Mutagen. 849, 503129. doi:10.1016/j.mrgentox.2019.503129
Smart, D. J., and Phillips, G. (2021). Collecting e-cigarette aerosols for in vitro applications: a survey of the biomedical literature and opportunities to increase the value of submerged cell culture-based assessments. J. Appl. Toxicol. 41, 161–174. doi:10.1002/jat.4064
Stabile, A. M., Marinucci, L., Balloni, S., Giuliani, A., Pistilli, A., Bodo, M., et al. (2018). Long term effects of cigarette smoke extract or nicotine on nerve growth factor and its receptors in a bronchial epithelial cell line. Toxicol. Vitro 53, 29–36. doi:10.1016/j.tiv.2018.07.020
Stewart, C. E., Torr, E. E., Mohd Jamili, N. H., Bosquillon, C., and Sayers, I. (2012). Evaluation of differentiated human bronchial epithelial cell culture systems for asthma research. J. Allergy (Cairo) 2012, 943982. doi:10.1155/2012/943982
Stucki, A. O., Stucki, J. D., Hall, S. R., Felder, M., Mermoud, Y., Schmid, R. A., et al. (2015). A lung-on-a-chip array with an integrated bio-inspired respiration mechanism. Lab. Chip 15, 1302–1310. doi:10.1039/c4lc01252f
Stucki, J. D., Hobi, N., Galimov, A., Stucki, A. O., Schneider-Daum, N., Lehr, C. M., et al. (2018). Medium throughput breathing human primary cell alveolus-on-chip model. Sci. Rep. 8, 14359. doi:10.1038/s41598-018-32523-x
Su, R., Xiong, S., Zink, D., and Loo, L. H. (2016). High-throughput imaging-based nephrotoxicity prediction for xenobiotics with diverse chemical structures. Arch. Toxicol. 90, 2793–2808. doi:10.1007/s00204-015-1638-y
Sun, D., Gao, W., Hu, H., and Zhou, S. (2022). Why 90% of clinical drug development fails and how to improve it? Acta. Pharm. Sin. B 12, 3049–3062. doi:10.1016/j.apsb.2022.02.002
Sussman, R. A., Sipala, F., Emma, R., and Ronsisvalle, S. (2023). Aerosol emissions from heated tobacco products: a review focusing on carbonyls, analytical methods, and experimental quality. Toxics 11 (12), 947. doi:10.3390/toxics11120947
Takahashi, Y., Kanemaru, Y., Fukushima, T., Eguchi, K., Yoshida, S., Miller-Holt, J., et al. (2018). Chemical analysis and in vitro toxicological evaluation of aerosol from a novel tobacco vapor product: a comparison with cigarette smoke. Regul. Toxicol. Pharmacol. 92, 94–103. doi:10.1016/j.yrtph.2017.11.009
Taylor, M., Jaunky, T., Hewitt, K., Breheny, D., Lowe, F., Fearon, I. M., et al. (2017). A comparative assessment of e-cigarette aerosols and cigarette smoke on in vitro endothelial cell migration. Toxicol. Lett. 277, 123–128. doi:10.1016/j.toxlet.2017.06.001
Taylor, M., Santopietro, S., Baxter, A., East, N., Breheny, D., Thorne, D., et al. (2020). In vitro biological assessment of the stability of cigarette smoke aqueous aerosol extracts. BMC Res. Notes 13, 492. doi:10.1186/s13104-020-05337-2
Taylor, M., Thorne, D., Carr, T., Breheny, D., Walker, P., Proctor, C., et al. (2018). Assessment of novel tobacco heating product THP1.0. Part 6: a comparative in vitro study using contemporary screening approaches. Regul. Toxicol. Pharmacol. 93, 62–70. doi:10.1016/j.yrtph.2017.08.016
Thomas, D. G., Smith, J. N., Thrall, B. D., Baer, D. R., Jolley, H., Munusamy, P., et al. (2018). ISD3: a particokinetic model for predicting the combined effects of particle sedimentation, diffusion and dissolution on cellular dosimetry for in vitro systems. Part Fibre Toxicol. 15, 6. doi:10.1186/s12989-018-0243-7
Thomas, R. S., Bahadori, T., Buckley, T. J., Cowden, J., Deisenroth, C., Dionisio, K. L., et al. (2019). The next generation blueprint of computational toxicology at the US Environmental Protection Agency. Toxicol. Sci. 169, 317–332. doi:10.1093/toxsci/kfz058
Thorne, D., and Adamson, J. (2013). A review of in vitro cigarette smoke exposure systems. Exp. Toxicol. Pathol. 65, 1183–1193. doi:10.1016/j.etp.2013.06.001
Thorne, D., Breheny, D., Proctor, C., and Gaca, M. (2018). Assessment of novel tobacco heating product THP1.0. Part 7: comparative in vitro toxicological evaluation. Regul. Toxicol. Pharmacol. 93, 71–83. doi:10.1016/j.yrtph.2017.08.017
Thorne, D., Crooks, I., Hollings, M., Seymour, A., Meredith, C., and Gaca, M. (2016). The mutagenic assessment of an electronic-cigarette and reference cigarette smoke using the Ames assay in strains TA98 and TA100. Mutat. Res. Genet. Toxicol. Environ. Mutagen. 812, 29–38. doi:10.1016/j.mrgentox.2016.10.005
Thorne, D., Hollings, M., Kilford, J., Clements, J., Payne, R., Ballantyne, M., et al. (2020). An experimental aerosol air–agar interface mouse lymphoma assay methodology. Mutat. Res. Genet. Toxicol. Environ. Mutagen. 856-857, 503230. doi:10.1016/j.mrgentox.2020.503230
Thorne, D., Leverette, R., Breheny, D., Lloyd, M., Mcenaney, S., Whitwell, J., et al. (2019a). Genotoxicity evaluation of tobacco and nicotine delivery products: Part One. Mouse lymphoma assay. Food Chem. Toxicol. 132, 110584. doi:10.1016/j.fct.2019.110584
Thorne, D., Leverette, R., Breheny, D., Lloyd, M., Mcenaney, S., Whitwell, J., et al. (2019b). Genotoxicity evaluation of tobacco and nicotine delivery products: Part Two. in vitro micronucleus assay. Food Chem. Toxicol. 132, 110546. doi:10.1016/j.fct.2019.05.054
Thorne, D., Wieczorek, R., Fukushima, T., Shin, H.-J., Leverette, R., Ballantyne, M., et al. (2021). A survey of aerosol exposure systems relative to the analysis of cytotoxicity: a Cooperation Centre for Scientific Research Relative to Tobacco (CORESTA) perspective. Toxicol. Res. Appl. 5, 239784732110222. doi:10.1177/23978473211022267
U.S. Environmental Protection Agency (2023). Alternative test methods and strategies to reduce vertebrate animal testing. Available at: https://www.epa.gov/assessing-and-managing-chemicals-under-tsca/alternative-test-methods-and-strategies-reduce (Accessed December 6, 2023).
Van Der Toorn, M., Sewer, A., Marescotti, D., Johne, S., Baumer, K., Bornand, D., et al. (2018). The biological effects of long-term exposure of human bronchial epithelial cells to total particulate matter from a candidate modified-risk tobacco product. Toxicol. Vitro 50, 95–108. doi:10.1016/j.tiv.2018.02.019
Van Der Zalm, A. J., Barroso, J., Browne, P., Casey, W., Gordon, J., Henry, T. R., et al. (2022). A framework for establishing scientific confidence in new approach methodologies. Arch. Toxicol. 96, 2865–2879. doi:10.1007/s00204-022-03365-4
Van Meer, P. J., Graham, M. L., and Schuurman, H. J. (2015). The safety, efficacy and regulatory triangle in drug development: impact for animal models and the use of animals. Eur. J. Pharmacol. 759, 3–13. doi:10.1016/j.ejphar.2015.02.055
Vaughan, M. B., Ramirez, R. D., Wright, W. E., Minna, J. D., and Shay, J. W. (2006). A three-dimensional model of differentiation of immortalized human bronchial epithelial cells. Differentiation 74, 141–148. doi:10.1111/j.1432-0436.2006.00069.x
Veljkovic, E., Jiricny, J., Menigatti, M., Rehrauer, H., and Han, W. (2011). Chronic exposure to cigarette smoke condensate in vitro induces epithelial to mesenchymal transition-like changes in human bronchial epithelial cells, BEAS-2B. Toxicol. Vitro 25, 446–453. doi:10.1016/j.tiv.2010.11.011
Villeneuve, D. L., Crump, D., Garcia-Reyero, N., Hecker, M., Hutchinson, T. H., Lalone, C. A., et al. (2014). Adverse outcome pathway (AOP) development I: strategies and principles. Toxicol. Sci. 142, 312–320. doi:10.1093/toxsci/kfu199
Vinken, M. (2020). 3Rs toxicity testing and disease modeling projects in the European Horizon 2020 research and innovation program. EXCLI J. 19, 775–784. doi:10.17179/excli2020-1463
Watson, C. Y., DeLoid, G. M., Pal, A., and Demokritou, P. (2016). Buoyant nanoparticles: implications for nano-biointeractions in cellular studies. Small 12 (23), 3172–3180. doi:10.1002/smll.201600314
Wheeldon, R. P., Bernacki, D. T., Dertinger, S. D., Bryce, S. M., Bemis, J. C., and Johnson, G. E. (2020). Benchmark dose analysis of DNA damage biomarker responses provides compound potency and adverse outcome pathway information for the topoisomerase II inhibitor class of compounds. Environ. Mol. Mutagen. 61, 396–407. doi:10.1002/em.22360
Wieczorek, R., Trelles Sticken, E., Pour, S. J., Chapman, F., Röwer, K., Otte, S., et al. (2023). Characterisation of a smoke/aerosol exposure in vitro system (SAEIVS) for delivery of complex mixtures directly to cells at the air-liquid interface. J. Appl. Toxicol. 43, 1050–1063. doi:10.1002/jat.4442
Wong, A. P., Chin, S., Xia, S., Garner, J., Bear, C. E., and Rossant, J. (2015). Efficient generation of functional CFTR-expressing airway epithelial cells from human pluripotent stem cells. Nat. Protoc. 10, 363–381. doi:10.1038/nprot.2015.021
Yoshida, T., and Tuder, R. M. (2007). Pathobiology of cigarette smoke-induced chronic obstructive pulmonary disease. Physiol. Rev. 87, 1047–1082. doi:10.1152/physrev.00048.2006
Zhang, J., Chang, X., Holland, T. L., Hines, D. E., Karmaus, A. L., Bell, S., et al. (2021). Evaluation of inhalation exposures and potential health impacts of ingredient mixtures using in vitro to in vivo extrapolation. Front. Toxicol. 3, 787756. doi:10.3389/ftox.2021.787756
Keywords: new approach methodologies (NAM), organs on a chip (OoC), human 3D tissues, next-generation products (NGP), airway models, high-content analysis, adverse outcome pathway (AOP), dosimetry
Citation: Thorne D, McHugh D, Simms L, Lee KM, Fujimoto H, Moses S and Gaca M (2024) Applying new approach methodologies to assess next-generation tobacco and nicotine products. Front. Toxicol. 6:1376118. doi: 10.3389/ftox.2024.1376118
Received: 25 January 2024; Accepted: 30 April 2024;
Published: 13 June 2024.
Edited by:
Victoria Hutter, University of Hertfordshire, United KingdomReviewed by:
Michael Oldham, University of California, Irvine, United StatesCopyright © 2024 Thorne, McHugh, Simms, Lee, Fujimoto, Moses and Gaca. This is an open-access article distributed under the terms of the Creative Commons Attribution License (CC BY). The use, distribution or reproduction in other forums is permitted, provided the original author(s) and the copyright owner(s) are credited and that the original publication in this journal is cited, in accordance with accepted academic practice. No use, distribution or reproduction is permitted which does not comply with these terms.
*Correspondence: Marianna Gaca, bWFyaWFubmFfZ2FjYUBiYXQuY29t
Disclaimer: All claims expressed in this article are solely those of the authors and do not necessarily represent those of their affiliated organizations, or those of the publisher, the editors and the reviewers. Any product that may be evaluated in this article or claim that may be made by its manufacturer is not guaranteed or endorsed by the publisher.
Research integrity at Frontiers
Learn more about the work of our research integrity team to safeguard the quality of each article we publish.