- 1Helmholtz Centre for Environmental Research – UFZ, Chemicals in the Environment Research Section, Leipzig, Germany
- 2University of Leipzig, Medical Faculty, Leipzig, Germany
- 3Norwegian Institute of Public Health – NIPH, Department of Chemical Toxicology, Oslo, Norway
- 4IUF – Leibniz Research Institute for Environmental Medicine, Düsseldorf, Germany
- 5DNTOX GmbH, Düsseldorf, Germany
- 6Swiss Centre for Applied Human Toxicology, University of Basel, Basel, Switzerland
- 7Uppsala University, Department of Organismal Biology, Uppsala, Sweden
- 8European Chemicals Agency (ECHA), Helsinki, Finland
- 9German Federal Institute for Risk Assessment (BfR), Berlin, Germany
- 10Université de Bordeaux, Institut National de la Santé et de la Recherche Médicale (INSERM), Maladies Rares: Génétique et Métabolisme (MRGM), Pessac, France
- 11University of Oslo, Section for Pharmacology and Pharmaceutical Biosciences, Department of Pharmacy, Olso, Norway
- 12Dutch Nation Institute for Public Health and the Environment (RIVM), Centre for Health Protection, Bilthoven, Netherlands
- 13University of Birmingham, Centre for Environmental Research and Justice, Birmingham, UK
- 14Norwegian University of Life Sciences (NMBU), Faculty of Veterinary Medicine, Ås, Norway
- 15University of Gdansk, Laboratory of Environmental Chemoinformatics, Gdansk, Poland
- 16University of Veterinary Medicine Hannover, Foundation, Institute for Food Quality and Food Safety, Hannover, Germany
- 17University of Konstanz, In Vitro Toxicology and Biomedicine/CAAT-Europe, Konstanz, Germany
- 18AIT Austrian Institute of Technology GmbH, Competence Unit Molecular Diagnostics, Center Health and Bioresources, Vienna, Austria
- 19Danube Private University, Faculty of Dentistry and Medicine, Department of Medicine, Krems, Austria
- 20Uppsala University and Science for Life Laboratory, Department of Pharmaceutical Biosciences, Uppsala, Sweden
- 21Instituto de Salud Carlos III (ISCIII), Centro Nacional de Sanidad Ambiental (CNSA), Environmental Toxicology Unit, Majadahonda, Spain
In the European regulatory context, rodent in vivo studies are the predominant source of neurotoxicity information. Although they form a cornerstone of neurotoxicological assessments, they are costly and the topic of ethical debate. While the public expects chemicals and products to be safe for the developing and mature nervous systems, considerable numbers of chemicals in commerce have not, or only to a limited extent, been assessed for their potential to cause neurotoxicity. As such, there is a societal push toward the replacement of animal models with in vitro or alternative methods. New approach methods (NAMs) can contribute to the regulatory knowledge base, increase chemical safety, and modernize chemical hazard and risk assessment. Provided they reach an acceptable level of regulatory relevance and reliability, NAMs may be considered as replacements for specific in vivo studies. The European Partnership for the Assessment of Risks from Chemicals (PARC) addresses challenges to the development and implementation of NAMs in chemical risk assessment. In collaboration with regulatory agencies, Project 5.2.1e (Neurotoxicity) aims to develop and evaluate NAMs for developmental neurotoxicity (DNT) and adult neurotoxicity (ANT) and to understand the applicability domain of specific NAMs for the detection of endocrine disruption and epigenetic perturbation. To speed up assay time and reduce costs, we identify early indicators of later-onset effects. Ultimately, we will assemble second-generation developmental neurotoxicity and first-generation adult neurotoxicity test batteries, both of which aim to provide regulatory hazard and risk assessors and industry stakeholders with robust, speedy, lower-cost, and informative next-generation hazard and risk assessment tools.
1 The European Partnership for the Assessment of Risks from chemicals (PARC)
The European Partnership for the Assessment of Risks from Chemicals (PARC) aims to develop next-generation chemical hazard and risk assessment tools to better protect human health and the environment (Marx-Stoelting et al., 2023). A major ambition of the project is to develop new approach methods (NAMs) for human health hazard assessment that covers developmental neurotoxicity (DNT), adult neurotoxicity (ANT), thyroid hormone disruption, immunotoxicity, and non-genotoxic carcinogens. Work package 5.2.1e aims to refine existing NAMs, develop new ones, and generate first-generation ANT and second-generation DNT test batteries. The NAMs that will be developed will be based on Key Events (KE) as identified in the Adverse Outcome Pathway (AOP) framework (Ankley et al., 2010; Leist et al., 2017; Spînu et al., 2021). The work is carried out by a consortium of over 25 experts from 10 EU research institutions and two partner institutions in non-EU countries.
2 Exposure to chemicals may pose a risk to the developing and mature nervous systems
Exposure to chemicals can adversely impact nervous system development and function across all stages of life (Costa et al., 2008; Giordano and Costa, 2012; P; Grandjean and Landrigan, 2006). Adverse chemical-dependent effects stemming from exposure of the developing offspring (including in utero and postnatal) to the time of sexual maturation may affect the developing nervous system (Costa et al., 2004). Such “developmental neurotoxicity” (DNT) can be long-lasting, extending far beyond the exposure period, and can vary across lifespan (Eriksson et al., 1998; Spalding et al., 2013). Note that any type of neurotoxic effect during development is of regulatory concern and relevant for developmental hazard identification. In contrast, when the mature nervous system is exposed to neurotoxic chemicals, adult neurotoxicity (ANT) effects can be immediate or they may be gradually developing and long-lasting. Depending on the type of ANT effect, it may also be reversible (Spencer and Lein, 2014). Significant and/or severe neurotoxicity, being reversible or irreversible, immediate or delayed, is of regulatory concern.
Due to the sensitivity of the developing nervous system, exposure to low concentrations of certain chemicals may lead to structural and functional disruptions (Rice and Barone, 2000; Grandjean and Landrigan, 2014; Bennett et al., 2016). Neurodevelopmental disorders including autism spectrum disorder, intellectual disability, attention deficit/hyperactivity disorder (ADHD), neurodevelopmental motor disorders (including tic disorders), and specific learning disorders can have lifelong socioeconomic consequences, including diminished economic productivity or an increased need for learning support in schools (P. Grandjean and Landrigan, 2006). Whilst estimates were acknowledged to be uncertain, in the EU, ∼30,000 disability adjusted life years (DALYs) related to neurodevelopmental disease may be the result of chemical exposure (and irrespective of a person’s genetic predisposition/sensitivity), with ∼250,000 DALYs when chemical exposure was combined with underlying genetic predisposition (EC 2019). This estimate was based on a ‘top down’ assessment of impacts of pervasive neurodevelopmental disorders from the World Health Organization (WHO) and an estimate that 3% is due to environmental exposure to legacy compounds such as lead and other environmental pollutants (EC 2019). Notable socioeconomical benefits are therefore predicted via the identification of substances which are known or presumed to cause DNT and subsequent prevention of exposure (Bellanger et al., 2013; Bellanger et al., 2015).
After the developmental period, acute and/or chronic exposure to environmental chemicals may elicit toxic responses in the peripheral and/or central nervous systems and it has been suggested that exposure to specific chemical agents may increase the probability of developing neurodegenerative disorders such as Parkinson’s and Alzheimer’s Diseases, or dementia (Landrigan et al., 2005; Tanner et al., 2014; Ockleford et al.). Moreover, exposure to certain chemicals has been suspected to be linked to adolescent and adult depression, anxiety, and other psychiatric disorders in a number of academic publications (Dickerson et al., 2020; Hollander et al., 2020; Jacobson et al., 2022; Rokoff et al., 2022; Aung et al., 2023). In a study of 22 chemical inventories from 19 countries and regions, over 350,000 chemicals and mixtures of chemicals were identified as registered for production and potentially in use (Wang et al., 2020). Despite knowledge concerning the potentially harmful impacts of environmental chemicals on the developing and mature nervous systems (P. Grandjean and Landrigan, 2006), it is understood that only a limited number of unique substances has been tested for DNT using OECD Test Guideline (TG) studies. (OECD, 2008a; Makris et al., 2009; Sachana et al., 2019; Crofton and Mundy, 2021).
3 Policy and regulatory landscapes
The EU Green Deal describes health impacts in the Zero-Pollution Action Plan, and the European Commission recently highlighted their interest in increased efforts to protect against the most harmful chemicals, by further exploring the risk management possibilities of neurotoxic and endocrine disrupting (which has been linked to DNT) substances (European Commission, 2020). In the EU, several relevant regulations are in force. For example, before entering the market or gaining approval as a biocidal or pesticidal active substance, the minimum data requirements described in the relevant EU Regulation must be fulfilled (among other conditions). EU regulations on plant protection products (Reg EC 1107; European Parliament and Council, 2009) and biocides (Reg EC 528; European Parliament and Council, 2012) can require DNT/ANT testing as part of the data requirements. Under the EU Biocides Product Regulation (Reg EC 528; European Parliament and Council, 2012), specific DNT testing, for example, OECD TG 426, recently became a mandatory information requirement for the approval process of active biocidal substances. Under REACH (European Parliament and Council, 2006), the European Regulation created to protect human health and the environment from harmful chemicals, the level of information required to identify potential neurotoxic (DNT/ANT) properties currently depends on the tonnage and identification of specific concerns that may trigger DNT or ANT tests. The available information is used to apply appropriate hazard classifications, as per the criteria specified in the CLP regulation (Reg EC 1272; European Parliament and Council, 2008), to inform on the hazardous properties of chemicals. Classification in accordance with CLP then serves to trigger or inform remedial actions in other legislation to control the hazard. The CLP regulation (Articles seven and 8) does not require DNT or ANT TGs directly but rather makes use of all available data generated in the context of relevant legislation and/or otherwise available in the public domain. In cases where such data is not available to inform on a given hazard, testing may be conducted under certain conditions including the condition that tests on animals are to be carried out only where no other alternatives, which provide adequate reliability and quality of data, are possible. This implies support and presents an opportunity for the development, validation, and implementation of NAMs.
Within the CLP Regulation, substances with DNT are addressed under the reproductive (developmental) toxicity hazard class and ANT effects are addressed under Specific Target Organ Toxicity (STOT), either single exposure (SE) or repeated exposure (RE), depending on whether the effects are caused by single or repeated exposures, respectively. The recent revision of the CLP regulation includes a new hazard class for endocrine disruption that includes endocrine activity mediated adverse effects on the developing (and mature) nervous system (Reg EC 1272; (European Parliament and Council, 2023). According to the new criteria, classification as ED Category one shall be largely based on evidence from at least one of the following: human data; animal data; non-animal data providing an equivalent predictive capacity as human data or animal data (Reg EC 1272; European Parliament and Council, 2023). Thus, the new hazard class allows for NAMs to be directly used for the purpose of this specific classification when the criteria are met.
3.1 DNT/ANT in current chemical regulations
The information needed to fulfill the data requirements under REACH and BPR is typically provided by the in vivo OECD TG studies defined in the relevant section of the applicable regulation, but there are also specific possibilities for adaptation (more specifically data waiving). Such adaptation possibilities can include non-animal approaches and/or the use of existing information stemming from similar substances via a read-across approach (European Parliament and Council, 2006). However, where data on human health and environmental properties are derived via adaptations to data requirements, certain conditions apply. The conditions for adaptations using in vitro data under REACH are specified in Annex XI, section 1.4. In the context of a read across adaptation (REACH Annex XI, section 1.5), again certain restrictive conditions apply with regard to the data that directly informs on the hazard. However, for the extrapolation of such data to other substances, there is a clear opportunity to utilize NAMs as supportive information to demonstrate similarity in the properties of the substances concerned.
It should also to be noted that, depending on the applicable regulation, new tests may not be necessary if the available data is already sufficient for the regulatory purpose as given in the specific regulation. For example, the DNT study shall not be conducted under the BPR if the available data already indicate that the substance causes developmental toxicity and meets the criteria to be classified as toxic for reproduction category 1A or 1B: May damage the unborn child (H360D), and these available data are adequate to support a robust risk assessment (European Parliament and Council, 2012).
A range of OECD TG studies, including single-dose studies (e.g., OECD TG 402, 403, 420, 423, 425) and/or repeated dose toxicity studies (e.g., OECD TG 407, 408, 421, 422, 414, 443 in the absence of DNT cohorts) may inform on ANT or DNT based on clinical signs such as paralysis, convulsions, lack of coordination, or ataxia or neurohistopathology and/or alterations in brain weight (Table 1). DNT can be evaluated more comprehensively using dedicated tests such as OECD TG 426 or in the DNT cohort (cohorts 2A and 2B) of the Extended One-Generation Reproduction Toxicity Study (EOGRTS, OECD TG 443). OECD TGs dedicated to studying ANT include OECD TG 424, 418 and 419. Under REACH, ANT or specific mechanisms/modes of action with an association to (developmental) neurotoxicity can be used to trigger specific DNT studies. Substances in food intended for infants can also prompt investigations to assess potential DNT (EFSA Scientific Committee et al., 2017).
As the development of the nervous system starts prenatally and continues to develop through adolescence, reaching adult levels of neurotransmitters, synaptic plasticity, myelination and grey matter at around age of 20 in humans and around PND60 in rats (Semple et al., 2013), it is key to implement exposure throughout the whole developmental period for improving the chances to identify developmental neurotoxicants. In an OECD TG 426, the offspring are exposed as a minimum from the time of implantation (starting on gestation day (GD) 6) throughout lactation (until postnatal day (PND) 21). In cohort 2A of an EOGRTS, the offspring are exposed via the mother in utero, through lactation and directly at least after weaning until termination on ∼ PND 66–77. The assessed DNT parameters in specific DNT studies include as a minimum (depending on the OECD TG) motor activity, motor and sensory function, associative learning and memory (only in OECD TG 426 as standard testing), brain weight, and central and peripheral nervous system histopathology (Tsuji and Crofton, 2012). As dedicated DNT studies are often complex studies using rodents, they are resource-intensive regarding time, costs, and number of animals (Crofton et al., 2012; Smirnova et al., 2014) and only a limited number of chemicals have been tested for DNT using OECD TG DNT studies (OECD, 2008a; Makris et al., 2009; Sachana et al., 2019; Crofton and Mundy, 2021). In addition, variability in rodent neurotoxicity tests has been documented (Tsuji and Crofton, 2012; Terron and Bennekou, 2018; Sachana et al., 2019; Paparella et al., 2020; Harry et al., 2022) which indicates a need to specify, optimize, and harmonize the individual test methods used as part of the OECD TG. It also underscores the need for new tests that lack the excess variability associated with in vivo guideline studies for the assessment of DNT.
The risk posed by unidentified (developmental) neurotoxic agents and the limited number of timely and cost-efficient test systems (i.e., NAMs) serve as the basis for this PARC project where the ambition is the generation of an improved in vitro and alternative DNT test battery and a first-generation in vitro ANT test battery. The need to develop NAM-based next-generation hazard and risk assessment for DNT and ANT has found international support from academic scientists, industry, certain regulatory authorities, and other interest groups (Smirnova et al., 2014; Ockleford et al., 2017; Fritsche et al., 2018; Kavlock et al., 2018; Craig et al., 2019; Paparella et al., 2020; Vinken et al., 2021; Pallocca et al., 2022; Stucki et al., 2022). ECHA recently published Key Areas of Regulatory Challenge (ECHA, 2023), which highlights several of the known scientific and regulatory challenges that NAMs face. It further underlines the need for additional research in the field of ANT and DNT NAMs (ECHA, 2023).
NAMs have been advocated to be implemented into the regulatory hazard assessment stage of chemicals risk assessment (Stucki et al., 2022; Schmeisser et al., 2023). Currently, in vitro data may be used in Weight of Evidence assessment for classification and labelling (e.g., for developmental toxicity), or to trigger further DNT tests at REACH Annex IX and X, or to support grouping and read across from similar substances. High-throughput in vitro assays have also great potential as screening tools to prioritize chemicals and specific modes of action (MoA) for further testing (Escher et al., 2023). While such high throughput screening (HTS) tools have not yet been implemented for DNT and ANT in large scale regulatory practice, the introduction of more sophisticated in vitro tests and the validation of all HTS assays for DNT and ANT appear vital to improve their regulatory usefulness. It has been suggested that new in vitro methods should be mechanistically associated with adverse (developmental) neurotoxic outcomes (Pitzer, Shafer, and Herr, 2023). This is important to establish the toxicological relevance of endpoints measured in NAMs and/or to allow for the selection of the most informative follow-up studies to produce new information to elicit regulatory action (Smirnova et al., 2014; Ockleford et al., 2017; Fritsche et al., 2018; Kavlock et al., 2018; Craig et al., 2019; Paparella et al., 2020; Vinken et al., 2021; Pallocca et al., 2022; Stucki et al., 2022).
4 Building the DNT-IVB v2.0
One of the purposes of this PARC project is to deliver a guidance document containing a framework to facilitate the regulatory use of data derived from a DNT in vitro NAM-based test battery. A basic DNT in vitro test battery (IVB) has already been developed (i.e., DNT-IVB v1.0). It covers several cellular neurodevelopmental processes vital for normal brain development (Bal-Price et al., 2018; Masjosthusmann et al., 2020; Crofton and Mundy, 2021; Blum et al., 2022; Koch et al., 2022; OECD, 2023). The DNT-IVB v1.0 (Figure 1) measures effects of chemicals on human neural progenitor cell (hNPC) proliferation (Baumann et al., 2014; Baumann et al., 2015; Harrill et al., 2018; Nimtz et al., 2019; Masjosthusmann et al., 2020; Koch et al., 2022) and apoptosis (Druwe et al., 2015; Harrill et al., 2018), cell migration (Baumann et al., 2015; Baumann et al., 2016; Nyffeler et al., 2017; Schmuck et al., 2017; Masjosthusmann et al., 2020; Koch et al., 2022), hNPC-neuronal differentiation (Baumann et al., 2015; Schmuck et al., 2017; Masjosthusmann et al., 2020; Koch et al., 2022), oligodendrocyte differentiation (Dach et al., 2017; Schmuck et al., 2017; Masjosthusmann et al., 2020; Klose et al., 2021; Koch et al., 2022), neurite outgrowth (human: Harrill et al., 2010; Harrill et al., 2018; Krug et al., 2013; Hoelting et al., 2016; Masjosthusmann et al., 2020; Koch et al., 2022; rat; Harrill et al., 2013; Harrill et al., 2018), and synaptogenesis and neuronal network formation (rat: Harrill et al., 2011; Harrill et al., 2018; Brown et al., 2016; Frank et al., 2017; Shafer, 2019).
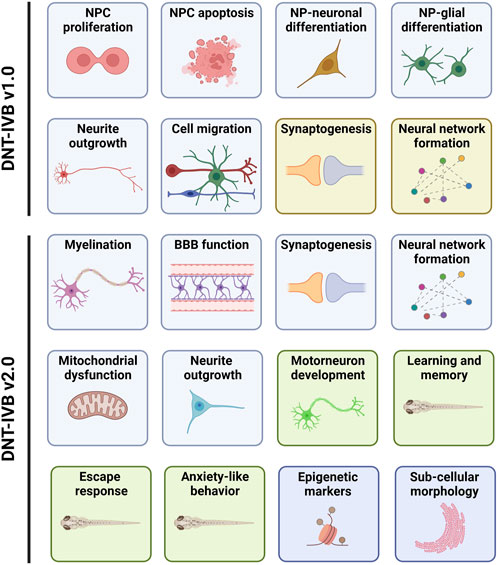
Figure 1. The DNT-IVB. Version 1.0 of the DNT-IVB (Masjosthusmann et al., 2020; Blum et al., 2022; OECD, 2023) contains endpoints for human neural progenitor cell (hNPC) proliferation and apoptosis, cell (neuronal, radial glia, oligodendrocytes) migration, hNPC-neuronal and oligodendrocyte differentiation, neurite outgrowth, neuronal maturation and synaptogenesis, and neuronal network formation (top). While most assays are conducted in human cells (blue boxes), two are performed in rat primary cells (yellow boxes). PARC 5.2.1e builds and evaluates NAMs for key battery gaps including myelination, blood-brain barrier (BBB) formation, mitochondrial function, and a suite of automated behavior-based NAMs in early life-stage zebrafish (green boxes). In addition, replacement of rodent-based NAMs with human-based test systems is also underway. Lastly, early epigenetic or sub-cellular morphological indicators of later DNT-related effects are being generated and evaluated for potential use in the DNT-IVB v2.0. Adapted from Crofton and Mundy, 2021.
Gap analysis of the DNT-IVB v1.0 revealed a requisite for supplementary cell assays (e.g., microglia) and functions (e.g., human neuronal network formation, astrocyte function, behavior, learning, and memory) to augment coverage and increase the ability to detect potential (developmental) neurotoxicants (Crofton and Mundy, 2021). Coverage of additional targets for neurotoxicants (e.g., signaling pathways and processes) is necessary, as exemplified by nicotine, a compound identified as a false negative in the DNT-IVB v1.0 (Masjosthusmann et al., 2020; Crofton and Mundy, 2021; Blum et al., 2022). This indicates the inability to detect (developmental) neurotoxic compounds that target nicotinic receptors in these test systems (e.g., neonicotinoid insecticides) (Sheets et al., 2016; Loser et al., 2021; Blum et al., 2022). To address some of the identified gaps, there are four key areas that this PARC project aims to improve during the development of the DNT-IVB v2.0 (Figure 1). This includes refinement of existing assays, generation of new NAMs to cover essential gaps, determination of the applicability domain for relevant available NAMs, and increased cost efficiency.
4.1 Refine existing assays
The current synaptogenesis and neural network formation assays were based in primary rat cortical cells differentiated in 2D on multielectrode arrays (MEA; Brown et al., 2016; Frank et al., 2017) (Figure 1). While there is also a recently established human neural network formation (hNNF) assay (Bartmann et al., 2023), it requires commercially available human-induced pluripotent stem cells (hiPSCs), which are used to derive excitatory and inhibitory neurons and primary human astrocytes that can be plated on MEAs for a functional assessment of network formation. This assay has recently been used to evaluate the effects of pesticides on human neural network formation (Bartmann et al., 2023). To decrease the costs of the hNNF assay, the PARC consortium will re-establish and refine the protocol using non-commercially generated hiPSC-derived excitatory and inhibitory neurons, together with human astrocytes derived from hNPCs (Koch et al., 2022). Synapse assembly is a critical feature of neurodevelopment. The DNT-IVB v1.0 assay for synaptogenesis is currently based on primary rat cortical cells (Harrill et al., 2011; Harrill et al., 2018). Human iPSC-derived NPCs can be differentiated into different types of postmitotic neurons and astrocytes (Davidsen et al., 2021; Lauvås et al., 2022). Therefore, a test system, comprised of a 2D mixed culture of neurons and astrocytes undergoing differentiation, will be developed and refined using high-content imaging. To enable comparison to data generated in the rat synaptogenesis assay, a chemical test set will be evaluated (described below).
Another identified gap within the DNT-IVB v1.0 is a lack of assays that describe mitochondrial toxicity events in susceptible cell types. AOP3 (“inhibition of the mitochondrial complex I of nigro-striatal neurons leads to parkinsonian motor deficits”) describes a link between inhibition of complex I of the mitochondrial respiratory chain and motor deficits associated with parkinsonian disorders (https://aopwiki.org/aops/3). The current DNT-IVB v1.0 assays are not particularly sensitive or fail to detect known mitochondrial toxicants (Masjosthusmann et al., 2020; Crofton and Mundy, 2021). To increase the sensitivity of battery assays to this class of neurotoxicants, several DNT-IVB v1.0 assays will be modified to allow for increased detection of mitochondrial toxicants. This step includes the assessment of neurite area and cell viability in human dopaminergic neurons and human immature peripheral neurons. While these endpoints are covered in the DNT-IVB v1.0, where the NAMs are performed in glucose-containing medium, in DNT-IVB v2.0, the assay will be performed in glucose-free, galactose-containing medium, which makes cells more reliant on their mitochondria and increases their sensitivity to mitochondrial toxicants (Hoelting et al., 2016; Delp et al., 2019; Delp et al., 2021).
4.2 Build new NAMs to cover essential gaps
4.2.1 Cellular gaps
In a key analysis, 29 neurotoxicity MoAs were characterized for 248 individual compounds representing 23 compound classes and 212 natural neurotoxins (Masjosthusmann et al., 2018). More comprehensive assessment of the potential for chemicals to harm the developing nervous system likely requires NAMs that cover the identified MoAs. One MoA not covered in the DNT-IVB v1.0 is the formation of a functional blood-brain barrier (BBB). The BBB determines the ability of some environmental chemicals to reach the central nervous system (Banks, 2009). Chemical exposure can affect the BBB integrity to cause DNT effects (Saili et al., 2017). Here, we will develop and use an hiPSC-based BBB NAM to test whether chemical exposure increases permeation of chemicals across the barrier, resulting in higher concentration reaching the central nervous system. According to established differentiation protocols (Appelt-Menzel et al., 2017), chemicals will be applied during cellular differentiation and transendothelial electrical resistance will be used as a readout of barrier function. One MoA considered relevant for DNT and not yet covered by the DNT-IVB v1.0 is the contribution of inflammatory reactions of glial cells. The key cell populations producing inflammatory mediators in the brain are astrocytes and microglia (Carson et al., 2006). These cells can be generated from human stem cells (Brüll et al., 2020; Spreng et al., 2022) and then either tested as pure populations, as mixed glial populations or together with various neuronal cultures (Gutbier et al., 2018; Klima et al., 2021).
4.2.2 Functional gaps
OECD TG 426 (OECD, 2007) assesses neurobehavioral endpoints which include measures of cognition (including associative learning and memory) in rodents exposed to chemicals during the developmental period. Cellular NAMs may provide information on cellular events that may eventually cause adverse effects on cognitive functions or other neurobehavioral functions but fail to provide equivalent information to neurobehavioral tests. In addition, when considering the complex integration of intracellular, intercellular, interregional, and systemic interactions that occur in development-stage and regional specific manners, in vitro NAMs do not cover all relevant cell types and processes, inherent within whole organisms, that are necessary to develop and maintain a functional nervous system. In this project, the alternative (i.e., relative to rodent models) early life zebrafish model will be used to generate a range of behavior-based assays that complement the in vitro approaches described above.
Zebrafish (Danio rerio) are a 3Rs-compliant (Hooijmans et al., 2010), non-protected vertebrate model up to 5 days post fertilization (dpf) (Strähle et al., 2012; Kalueff et al., 2013). The zebrafish embryo model may represent a powerful translational system for human hazard and risk assessment as zebrafish possess orthologs for 70% of human genes (Howe et al., 2013), 80% of human disease-related genes (Howe et al., 2013), and 86% of general human drug targets (Gunnarsson et al., 2008). Zebrafish are increasingly being utilized as a model system to investigate the function of the growing list of risk genes associated with neurodevelopment disorders (Sakai et al., 2018), including motor neuron diseases (Babin et al., 2014). Zebrafish neurodevelopment starts at 24 h post-fertilization and primary neurogenesis is complete by roughly 72 h post-fertilization (depending on rearing temperature). Resulting neuroanatomy (Gupta et al., 2018), nervous system transcriptomic lineages (Raj et al., 2018), and brain asymmetry (Duboc et al., 2015) are suggested to be comparable to humans. In addition, neurotransmitter systems, including glutaminergic, cholinergic, serotonergic, dopaminergic, adrenergic, GABAergic, and histaminergic (Babin et al., 2014; Faria et al., 2015; Horzmann and Freeman, 2016) are similar to those found in humans and associated with sensory-motor outcomes. This rapid establishment of neural structures during neurodevelopment and their link to quantifiable behavioral parameters is a major asset for PARC WP5.2.1e.
Relative to in vitro systems, metabolically competent zebrafish embryos may address potential toxicokinetics that can affect toxicity outcomes (Chu and Sadler, 2009). Regarding neurotoxicity, the assessment of neurobehavioral effects caused by xenobiotic exposure is advantageous because these perturbations are sensitive (i.e., they occur at sub-morphological concentrations) (Noyes et al., 2015; Bruni et al., 2016; Gaballah et al., 2020; Jarema et al., 2022). Locomotor activity can function as an automated and generalized readout of neurodevelopment. A major advantage of the early life zebrafish system as compared to in vitro systems is that it represents an alternative whole organism animal system that is emendable to genome-wide differential expression data collection throughout early neurodevelopment (Kettleborough et al., 2013) and are expected to address comparatively more MIEs and KEs related to DNT in a single assay (e.g., neurotransmitter signaling pathways, functional BBB, myelinated axons, functional synapses, neuronal networks, and neural circuits), as compared to individual in vitro test systems performed in single cell types or limited co-culture systems. Moreover, zebrafish development has been mapped at the resolution of single-cell transcriptomics, allowing the detection of cell-type specific changes associated with chemical induced adversity affecting neural and non-neural components of the developing brain (Farrell et al., 2018).
In compliance with EU directive (2010/63/EU; European Parliament and Council, 2010), the majority of work will be conducted in embryos up to 5 dpf. Another key advantage of early life stage zebrafish NAMs is that they can be screened in DNT and acute modes by varying the length and timing of chemical exposure. The DNT mode captures structural and functional deficits that alters locomotor activity in response to various stimuli. The acute mode identifies rapid, receptor-mediated changes in neuroactivity that can potentially be used as a complement to cellular ANT assays which aim to identify perturbations in signaling pathways (e.g., dopaminergic signaling) linked to ANT AOPs.
All DNT NAMs performed in early life-stage zebrafish described will be used following developmental exposure to PARC test chemicals and removed prior to behavior testing. This increases the likelihood of detecting functional or structural effects that arise from developmental perturbations in underlying behavior circuits after chemical exposure has ceased. Later, in the development of the ANT-IVB 1.0, the same assays will be applied post-neurogenesis (after three dpf) to detect the acute neuroactivity potential of test chemicals with a focus on the detection of perturbations in receptor-based neurotransmitter systems that are associated with DNT and/or ANT (e.g., dopaminergic, gabaergic, glutamatergic perturbations).
Another important functional topic is the impact of chemical exposure on associative learning and memory, which is assessed as a standard part of rodent-based OECD TG 426 for DNT (OECD, 2007), and may be included as an add on to TG 424 (OECD, 1997) for ANT and in TG 443 for DNT (OECD, 2018b). In vitro test systems are unable to account for these complex behavioral and cognitive aspects. Members of this consortium are developing a NAM that detects chemical-dependent disruption of non-associative learning and memory retention in early life stage zebrafish (Figure 2). An escape response NAM that identifies chemicals that specifically disrupt the motor system via the activation of reticulospinal neurons and independently of sensory processing (Dubrana et al., 2021; Knoll-Gellida et al., 2021) will be further developed and applied to screen a common set of chemicals described below. A NAM for chemical-induced developmental motor neuron toxicity will also be developed using the transgenic line Tg (nrp1a:gfp)js12 with labeled motor neurons (Sato-Maeda et al., 2006). A NAM that detects anxiety-like behavior via the detection of thigmotaxis, or the time spent along the outer edge of a well, is also under development. Finally, for a subset of test chemicals, the persistence of behavioral effects, post-exposure, will be evaluated to substantiate the detection of DNT effects in 14 dpf old larvae.
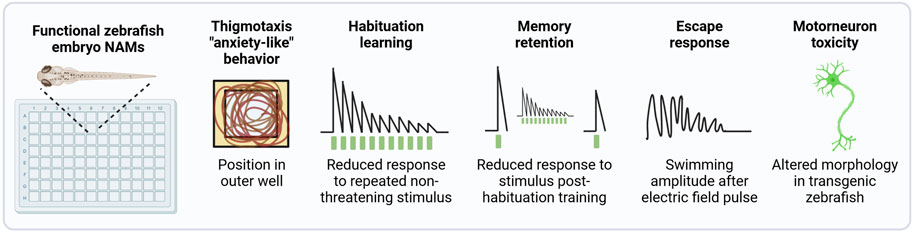
Figure 2. Functional NAMs performed in early life stage zebrafish. A suite of automated zebrafish-embryo behavior-based NAMs are under development for potential inclusion in the DNT-IVB v2.0. All NAMs are performed using automated tracking. Most assays will be performed in 96 square well plates for a comparable throughput to in vitro assays. Based on the exposure paradigm used, these assays can be performed with protocols that predict DNT and/or ANT. For the detection of DNT, chemical exposure occurs during development and is removed prior to behavior assessments. In contrast, if exposure takes place after neurogenesis is complete, any adverse effect related to the specific signalling pathways that have reached maturation may be indicative of acute ANT. However, as the organism’s nervous system is still developing, it cannot be excluded that the nature of the effect may be considered within a DNT assessment framework.
4.2.3 Determine applicability domain
The applicability domain describes the physicochemical or other properties of the chemicals for which a NAM is applicable for use (OECD, 2005). The applicability domain is generally determined using a range of reference chemicals linked to an adverse effect (OECD, 2005). Within this project, a test set of 96 reference chemicals, based on previously published work (Masjosthusmann et al., 2020; Blum et al., 2022), will be evaluated. With the exception of the zebrafish NAMs, all other DNT NAMs will be performed in human cellular assays. As these models contain a limited number of cell types and other gaps, efforts will be made to assess the applicability domain to determine whether, and to what extent, the NAMs cover established DNT AOPs, including endocrine disruption (ED) or proposed DNT AOPs such as epigenetic perturbation. Specifically, in the 2D synaptogenesis model, characterization of differentiation up to 28 days will be performed to understand the abundance and distribution of ED-relevant receptors including retinoic acid receptor, estrogen receptor, androgen receptor, thyroid hormone receptor, glucocorticoid receptor and liver X receptor. Coverage of ED and epigenetic modes of action will also be carried out for certain cellular ANT NAMs (see below).
4.2.4 Increase molecular and cellular coverage, reliability, and cost efficiency
Mammalian TG studies for DNT testing are costly and time-consuming (Crofton et al., 2012; Smirnova et al., 2014). At the same time, mammals contain a complete nervous system with all functional components throughout the whole developmental period, and their communication with other relevant organs and systems, (e.g., gut, liver, endocrine, and immune systems) that can collectively influence neurotoxicity outcomes. Next-generation DNT and ANT testing seeks to eventually replace mammalian tests with a battery of in vitro and alternative test systems. Some of these next-generation test systems are lengthy and can take up to 35 days, which increases the overall cost of the potential test battery. To complement cellular and alternative assays potentially included in the DNT-IVB v2.0 and to provide a low-cost screening strategy, four approaches will be explored.
The first strategy seeks to identify early markers of later DNT-associated KEs with a focus on epigenomic or sub-cellular morphological alterations. Epigenomic processes drive cell differentiation, and chemically induced alteration in epigenetic patterns can lead to long-term changes in gene function (Baccarelli and Bollati, 2009). In this context, we will assess whether epigenetic re-arrangements precede morphological changes observed in differentiation-related assays, with the potential to shorten and/or strengthen such assays. This step will be addressed by performing and comparing different genome-wide epigenetic (i.e., DNA methylation) analyses at specific time points in cellular differentiation assays where shortening could be of interest. Cell painting is a high-throughput microscopy technique that allows researchers to simultaneously label and visualize multiple organelles in a cell (Bray et al., 2016). It uses a cocktail of fluorescent stains and high-content imaging to obtain parallel morphological measurements on a single cell level. This project will establish and evaluate an automated cell profiling methodology for DNT assays that is cheap, fully automated, data-rich, and can operate on 2D cell monolayers, and work is ongoing to expand to 3D spheroids. Images from Cell Painting are data-rich and highly applicable for analysis with artificial intelligence methods, for example, for MoA prediction (Tian et al., 2023), and assessment of combination effects of environmental compounds (Rietdijk et al., 2022). Morphological changes will be assessed as potential early indicators of DNT or ANT outcomes in short- and longer-term cellular assays.
The second strategy employs transcriptomics to refine the search for early markers of later DNT-associated KEs with a focus on genome-wide expression patterns observed at a single cell resolution of intact developing embryo nervous systems. This single-cell transcriptome data is complemented by building transcriptomics and metabolomics technologies into an existing OECD TG for embryotoxicity (TG 236) to measure (neuro)developmental toxicity endpoints. While it was developed as one of the potential alternatives to the acute test on fish (OECD TG 203) for ecotoxicological hazard assessment, it has been reported that compared to OECD TG 203, OECD TG 236 may underestimate acute toxicity for certain types of chemicals, in particular neurotoxicants (Klüver et al., 2016; Glaberman et al., 2017; Sobanska et al., 2018). Nevertheless, OECD TG 236 has recently attracted considerable attention for its potential expansion to human health endpoints, particularly developmental (neuro) toxicity (Braunbeck et al., 2014; Krzykwa et al., 2019).
The third strategy uses a rapid, low-cost cellular neurite outgrowth assay to screen a much larger chemical test set, including human-relevant mixtures (J. Lee et al., 2022a; J; Lee et al., 2022b). For this assay, SH-SY5Y cells, sub-cloned from a neuroblastoma cell line, are used in 384 well plate format (J. Lee et al., 2022b; J; Lee et al., 2022b). The specificity and sensitivity of this assay will be compared to non-transformed, hiPSC-derived DNT models.
The fourth strategy will build in silico models to predict the probability of inducing DNT effects using Quantitative Structure-Activity Relationships (QSAR) models to link chemical structural properties with measured neurotoxicity effects (Khelfaoui et al., 2021; Grillberger et al., 2023). Molecular docking combined with observed molecular dynamics will be employed to reflect interactions of organophosphates with cellular targets (e.g., membranes, proteins) identified as MIEs according to DNT-relevant AOPs (Gadaleta et al., 2022). Serine esterases and calcium transporters are currently under consideration (Knoll-Gellida et al., 2021). Method evaluation will be performed by comparing simulations with experimentally generated data. Binding affinity for the set of targets will then be predicted by using machine learning-based models, and structural alerts for pathway perturbation will be identified.
5 Demonstration of added value and identification of a minimum assay battery for DNT-IVB v2.0
A fundamental requirement for the regulatory acceptance of NAMs involves the development of test methods characterized by a high degree of robustness, performance, and readiness (Bal-Price et al., 2018), including acceptable levels of variability (Harry et al., 2022). If they are intended for future use in the context of hazard assessment, NAMs should ideally meet or exceed the sensitivity, specificity, accuracy, and reliability of the respective OECD TG, to ensure a continued level of acceptable chemical safety (Bal-Price et al., 2018). This approach ensures the use of data with a significant level of confidence. In the case of the DNT-IVB v1.0, elevated standards of readiness and robustness have already been demonstrated (Bal-Price et al., 2018; Krebs et al., 2019; Blum et al., 2022). To assess DNT-IVBv1.0 performance, a set of 45 reference (i.e., performance) compounds, consisting of 28 substances that were considered DNT positive and 17 substances that were considered DNT negative by the assay developers were used. Using these substances, an assay sensitivity of 68%, specificity of 100%, and accuracy of 80% was observed (Blum et al., 2022).
To substantiate the added value of new and refined assays within the DNT-IVB v2.0, a 96-member reference set will be used, which encompasses 45 performance compounds from the DNT-IVB v1.0 (Blum et al., 2022) and will be augmented with known modifiers targeting pathways specific to DNT (Fritsche, 2017) (e.g., mTOR (Lee, 2015), PDGFR-PLCγ1 (Kang et al., 2016), Notch (Imayoshi et al., 2013), and thyroid hormones (TH; López-Espíndola et al., 2014; Bernal et al., 2015)). Centralized chemical procurement and distribution will occur via a collaborative effort involving PARC 5.2.1e scientists and experts from the EU Joint Research Centre (EU-JRC). This structured approach facilitates standardized and comparable assessment of substances across partner laboratories, effectively minimizing uncertainties associated with substance purity, solubilization, and concentration. By testing the DNT-IVB v1.0 performance compounds at reasonable concentrations in each newly developed test method, we will gain insight into the chemical applicability domain of each assay to understand the potential added value of DNT-IVB v2.0 NAMs. If DNT-IVB v2.0 NAMs can appropriately identify DNT-IVB v1.0 false negatives as positives, this will increase the sensitivity of the resulting v2.0 test battery. In addition, the evaluation of specific pathway agonists and antagonists will reveal the applicability domain of each assay and the whole DNT-IVB v2.0.
6 Build ANT-IVB v1.0
In contrast to DNT, there is no comparable set of NAMs with a high readiness for ANT testing that covers important MoA. ANT can be elicited through a variety of mechanisms involving neurotransmitter receptors and ion transporters which influence the transmission and processing of signals in the human brain and other parts of the nervous system (Fritsche and Hogberg, 2020; Masjosthusmann et al., 2018). Recently, a neurotoxicity MoA analysis was performed for 248 individual compounds, representing 23 compound classes and 212 natural neurotoxins (Masjosthusmann et al., 2018). The identified MoA were grouped according to ANT common key events including cholinergic, GABAergic, glycinergic, glutamatergic, adrenergic, serotonergic, and dopaminergic neurotransmission, ion channels/receptors (e.g., sodium channels, potassium channels, calcium channels, chloride channels), and a range of cellular endpoints such as mitochondrial dysfunction, oxidative stress, apoptosis, redox cycling, altered calcium signaling, cytoskeletal alterations, neuroinflammation, axonopathies, myelin toxicity, delayed neuropathy, and enzyme inhibition (Masjosthusmann et al., 2018). To enable a thorough assessment of chemicals’ ANT potential, it is necessary to compile the ANT-IVB v1.0 that includes the entirety of the identified MoA, a challenge that will be addressed in our project.
To date, several assays have been established and published that cover critical KEs for neurotoxicity (Schmidt et al., 2017) but they still need refinement to meet certain criteria for regulatory acceptance of alternative methods including assessment of the domain of applicability, assay robustness and relevance, and demonstrated predictivity for adult neurotoxicity (Bal-Price et al., 2018). For the assessment of direct activation of ion channels and receptors and altered function of channels and receptors of (nociceptive) sensory neurons, several NAMs used in the DNT-IVB v2.0 can be repurposed for acute testing in mature 2D or 3D culture systems or in early life-stage zebrafish post neurogenesis. Specifically, DNT-IVB v2.0 test methods, including the myelin and BBB NAMs, cell painting in human BrainSpheres (Hartmann et al., 2023), and zebrafish learning and memory, motor system toxicity, and anxiety-like NAMs will be performed in mature cellular cultures or zebrafish embryos at time points which occur after primary neurogenesis and differentiation has occurred (R. Schmidt et al., 2013).
In addition to repurposed DNT-IVB v2.0 NAMs, several novel NAMs are being developed and applied to the ANT-IVB v1.0. One is a recently developed NAM based on hiPSC-derived nociceptor-enriched, mature sensory neurons (Holzer et al., 2022). Using this NAM after 23 days of differentiation, acute exposure to ANT reference chemicals will be carried out to evaluate the biological applicability domain of the assay. Another is the human multi-neurotransmitter assay (hMNR), which is based on hiPSC-derived mixed neuron-glia 3D BrainSpheres. The hMNR NAM assesses neuronal subtype-specific acute neurotoxicity using micro-electrode arrays (MEA) for the recording of spontaneous electrical activity (Hartmann et al., 2023). By sorting detected signals (spikes) based on their waveform, this assay allows the distinction between glutamatergic, GABAergic, dopaminergic, serotonergic, and cholinergic responses of the mixed-neuronal co-culture, allowing for an in vitro MoA-based assessment of ANT (Hartmann et al., 2023). In a third NAM, Ca2+ signaling will be assessed at the single cell level in mature central dopaminergic neurons (LUHMES cells), which extends the coverage of the KEs to another cell type and a set of functional receptors (e.g., P2X3 receptors (Apicella and Fabbretti, 2012)). A fourth NAM under development, the Peripheral Myelin Toxicity Assay (PeriMyelinTox), assesses myelin toxicity impacting peripheral sensory and motor function, and therefore addresses the key battery gap “myelination” for peripheral ANT in human cells. In this NAM, sensory or motor neurons, along with Schwann cells, will be differentiated from hiPSCs and cultivated in co-culture (Muller et al., 2018; Schenke et al., 2020; Louit et al., 2023). A novel 3D sphere format will be developed and compared to a conventional 2D format. Myelin formation will be evaluated by quantifying myelin basic protein (MBP) or myelin protein zero (MPZ) against the pan-neuronal marker β3-tubulin (TUJ1) through immunofluorescence staining and RT-qPCR (Chesnut et al., 2021). The assay will be optimized for automated high-throughput quantification of myelin post-exposure to a training set of potential myelin toxicants. The added value of both assays in assessing sensory and motor neuron myelin toxicity will be further evaluated.
To clearly define the applicability domains and assay-specific limitations, this project aims to develop a set of ANT reference chemicals that are known to affect the human brain, as well as negative compounds. This approach will ensure the unified characterization of the applicability domain of each assay and coverage of important human-relevant ANT MoA.
7 Outcomes and future perspectives
PARC was designed to address challenges inherent in moving from animal-based test methods to (batteries of) in vitro and alternative NAMs to speed up and modernize hazard identification and chemical risk assessment. Project 5.2.1e aims to improve the hazard prediction paradigm via the establishment and refinement of NAMs for DNT and ANT testing and the assembling of high performing, reproducible NAMs that provide added value into DNT and ANT test batteries. Our strategy encompasses the refinement of existing assays, generation of innovative NAMs to address identified gaps, determination of the applicability domain, and increased cost-effectiveness of lengthier assays via the demonstration of early indicators of later effects. Importantly, the development of new assays and the refinement of existing ones includes a strong focus on assay and data reliability. One critical ambition is to introduce quality control measures as described in Good Cell Culture Practices 2.0 (Pamies et al., 2022) or the GIVIMP document of the OECD (OECD, 2018). The PARC 5.2.1e consortium therefore contributes to an improved readiness, sensitivity, and overall performance of DNT NAMs to promote an increased acceptance of DNT in vitro and alternative assays for wider regulatory use. By the end of this project, a guidance document will be delivered that will introduce a novel framework aiming to facilitate the regulatory use of data derived from the DNT-IVB v2.0. Such work will include considerations on how DNT-IVB data may be used in the context of an IATA or weight of evidence for hazard and risk characterization. This links seamlessly to other PARC work packages that provide information on physiologically-based kinetic (PBK) modelling to convert IVB concentrations to predicted in vivo doses, and to risk assessment specialists that need to consider how the predicted doses can be used to set safe exposure thresholds by, for example, considering modulatory factors in AOP or by considering variabilities and specific sensitivities in exposed populations (Schmeisser et al., 2023; Suciu et al., 2023).
In contrast to DNT, there is currently no comparable battery of NAMs for ANT testing. Therefore, the consortium will create a first-generation ANT-IVB v1.0, covering major MoAs involved in human brain functioning. Looking ahead, and to respond to PARC regulatory colleagues’ requests for data on specific compound classes, 5.2.1e NAMs will be used to evaluate the potential toxicity of natural toxins, bisphenols, and per- and polyfluoroalkyl substances (PFAS). Overall, this consortium aims to offer an unprecedented opportunity to fill a longstanding gap for the commonplace assessment of neurotoxicity potential of commercial chemicals via the generation of MoA-based, robust, reproducible, fast, and inexpensive consolidated DNT and ANT testing strategies. As all of this work is conducted under the guidance of colleagues from a regulatory field and potential end users, our ambition is to revolutionize the hazard and risk assessment of DNT and ANT in Europe.
Data availability statement
The original contributions presented in the study are included in the article/Supplementary material, further inquiries can be directed to the corresponding authors.
Author contributions
TT: Conceptualization, Funding acquisition, Project administration, Writing-original draft, Writing-review and editing. OM: Writing-review and editing, Funding acquisition, Project administration. EF: Writing-review and editing, Funding acquisition. JR: Writing-review and editing, Funding acquisition. KC: Writing-review and editing. KAH: Writing-review and editing. CA: Writing-review and editing. PB: Writing-review and editing, Funding acquisition. BE: Writing-review and editing, Funding acquisition. HD: Writing-review and editing, Funding acquisition. KH: Writing-review and editing. KD: Writing-review and editing. YH: Writing-review and editing. SH: Writing-review and editing, Funding acquisition. KJ: Writing-review and editing. BJ: Writing-review and editing. NK: Writing-review and editing. AK-G: Writing-review and editing. BK: Writing-review and editing. MLe: Writing-review and editing, Funding acquisition. MLi: Writing-review and editing. JL: Writing-review and editing, Funding acquisition. FM: Writing-review and editing, Funding acquisition. JC: Writing-review and editing, Funding acquisition. WN: Writing-review and editing, Funding acquisition. GP: Writing-review and editing. BS: Writing-review and editing, Funding acquisition. IS: Writing-review and editing. SS: Writing-review and editing, Funding acquisition. OL: Writing-review and editing, Funding acquisition. MT-R: Writing-review and editing, Funding acquisition. KB: Conceptualization, Project administration, Writing-original draft, Writing-review and editing.
Funding
The author(s) declare that financial support was received for the research, authorship, and/or publication of this article. This work was carried out in the framework of the European Partnership for the Assessment of Risks from Chemicals (PARC) and has received funding from the European Union’s Horizon Europe research and innovation programme under Grant Agreement No 101057014. Views and opinions expressed are however those of the author(s) only and do not necessarily reflect those of the European Union or the Health and Digital Executive Agency. Neither the European Union nor the granting authority can be held responsible for them. This work was also supported by a Helmholtz Association W2 award to T. Tal. The views expressed in this document are those of the authors and are not necessarily those of the EU agencies. Any statement made regarding EU regulations is solely intended for information purposes and does not represent the official opinion of the European Chemicals Agency (ECHA). ECHA is not responsible for any use that may be made of the information contained in this document. Statements made or information contained in this publication are without prejudice to any future work that ECHA may initiate. Funding performed in close connection to the European Union’s Horizon 2020 Research and Innovation Programme under Grant Agreements No. 964518 (ToxFree), No. 964537 (RISK-HUNT3R), No. 965406 (PrecisionTox) and No. 963845 (ONTOX) in the ASPIS Cluster. Figures were generated using biorender.com.
Acknowledgments
We thank Shoko Furuno (ECHA) for her expert input.
Conflict of interest
EF and KB are shareholders of the DNTOX GmbH offering neurotoxicity testing services.
The remaining authors declare that the research was conducted in the absence of any commercial or financial relationships that could be construed as a potential conflict of interest.
Publisher’s note
All claims expressed in this article are solely those of the authors and do not necessarily represent those of their affiliated organizations, or those of the publisher, the editors and the reviewers. Any product that may be evaluated in this article, or claim that may be made by its manufacturer, is not guaranteed or endorsed by the publisher.
References
Ankley, G. T., Bennett, R. S., Erickson, R. J., Hoff, D. J., Hornung, M. W., Johnson, R. D., et al. (2010). Adverse outcome pathways: a conceptual framework to support ecotoxicology research and risk assessment. Environ. Toxicol. Chem. 29 (3), 730–741. doi:10.1002/ETC.34
Apicella, L., and Fabbretti, E. (2012). P2X3 receptor expression by HEK cells conditions their survival. Purinergic Signal. 8 (2), 295–300. doi:10.1007/S11302-011-9285-0
Appelt-Menzel, A., Cubukova, A., Günther, K., Edenhofer, F., Piontek, J., Krause, G., et al. (2017). Establishment of a human blood-brain barrier Co-culture model mimicking the neurovascular unit using induced pluri-and multipotent stem cells. Stem Cell Rep. 8, 894–906. doi:10.1016/j.stemcr.2017.02.021
Aung, M. T., Eick, S. M., Padula, A. M., Smith, S., Park, J. S., DeMicco, E., et al. (2023). Maternal per- and poly-fluoroalkyl substances exposures associated with higher depressive symptom scores among immigrant women in the chemicals in our bodies cohort in san francisco. Environ. Int. 172 (February), 107758. doi:10.1016/J.ENVINT.2023.107758
Babin, P. J., Goizet, C., and Raldúa, D. (2014). Zebrafish models of human motor neuron diseases: advantages and limitations. Prog. Neurobiol. 118, 36–58. doi:10.1016/J.PNEUROBIO.2014.03.001
Baccarelli, A., and Bollati, V. (2009). Epigenetics and environmental chemicals. Curr. Opin. Pediatr. 21 (2), 243–251. doi:10.1097/MOP.0B013E32832925CC
Bal-Price, A., Hogberg, H. T., Crofton, K. M., Daneshian, M., FitzGerald, R. E., Fritsche, E., et al. (2018). Recommendation on test readiness criteria for new approach methods in Toxicology: exemplified for developmental neurotoxicity. Altex 35 (3), 306–352. doi:10.14573/altex.1712081
Banks, W. A. (2009). Characteristics of compounds that cross the blood-brain barrier. BMC Neurol. 9 (Suppl. 1), S3–S9. doi:10.1186/1471-2377-9-S1-S3
Bartmann, K., Bendt, F., Dönmez, A., Haag, D., Eike Keßel, H., Masjosthusmann, S., et al. (2023). A human IPSC-based in vitro neural network formation assay to investigate neurodevelopmental toxicity of pesticides. ALTEX - Altern. Animal Exp. 40, 452–470. doi:10.14573/ALTEX.2206031
Baumann, J., Barenys, M., Gassmann, K., and Fritsche, E. (2014). Comparative human and rat ‘neurosphere assay’ for developmental neurotoxicity testing. Curr. Protoc. Toxicol. 1 (Suppl. 59), 12–24. doi:10.1002/0471140856.TX1221S59
Baumann, J., Dach, K., Barenys, M., Giersiefer, S., Goniwiecha, J., Lein, P. J., et al. (2015). “Application of the neurosphere assay for DNT hazard assessment: challenges and limitations,” in Methods in pharmacology and Toxicology (Totowa, NJ: Humana Press), 29. doi:10.1007/7653_2015_49
Baumann, J., Gassmann, K., Masjosthusmann, S., DeBoer, D., Bendt, F., Giersiefer, S., et al. (2016). Comparative human and rat neurospheres reveal species differences in chemical effects on neurodevelopmental key events. Archives Toxicol. 90 (6), 1415–1427. doi:10.1007/S00204-015-1568-8
Bellanger, M., Demeneix, B., Grandjean, P., Thomas Zoeller, R., and Trasande, L. (2015). Neurobehavioral deficits, diseases, and associated costs of exposure to endocrine-disrupting chemicals in the European union. J. Clin. Endocrinol. Metabolism 100 (4), 1256–1266. doi:10.1210/JC.2014-4323
Bellanger, M., Pichery, C., Aerts, D., Berglund, M., Castaño, A., Čejchanová, M., et al. (2013). Economic benefits of methylmercury exposure control in Europe: monetary value of neurotoxicity prevention. Environ. Health A Glob. Access Sci. Source 12 (1), 3–10. doi:10.1186/1476-069x-12-3
Bennett, D., Bellinger, D. C., Birnbaum, L. S., Bradman, A., Chen, A., Cory-Slechta, D. A., et al. (2016). Project TENDR: targeting environmental neuro-developmental risks the TENDR consensus statement. Environ. Health Perspect. 124 (7), A118–A122. doi:10.1289/EHP358
Bernal, J., Guadaño-Ferraz, A., and Morte, B. (2015). Thyroid hormone transporters--functions and clinical implications. Nat. Rev. Endocrinol. 11 (7), 690–717. doi:10.1038/nrendo.2015.186
Blum, J., Masjosthusmann, S., Bartmann, K., Bendt, F., Dolde, X., Dönmez, A., et al. (2022). Establishment of a human cell-based in vitro battery to assess developmental neurotoxicity hazard of chemicals. Chemosphere 311 (Pt 2), 137035. doi:10.1016/J.CHEMOSPHERE.2022.137035
Braunbeck, T., Kais, B., Lammer, E., Otte, J., Schneider, K., Stengel, D., et al. (2014). The fish embryo test (FET): origin, applications, and future. Environ. Sci. Pollut. Res. 22 (21), 16247–16261. doi:10.1007/S11356-014-3814-7
Bray, M. A., Singh, S., Han, H., Davis, C. T., Kost-Alimova, M., Gustafsdottir, S. M., et al. (2016). Cell painting, a high-content image-based assay for morphological profiling using multiplexed fluorescent dyes. Nat. Protoc. 11 (9), 1757–1774. doi:10.1038/NPROT.2016.105
Brown, J. P., Hall, D., Frank, C. L., Wallace, K., Mundy, W. R., and Shafer, T. J. (2016). Editor's highlight: evaluation of a microelectrode array-based assay for neural network ontogeny using training set chemicals. Toxicol. Sci. 154 (1), 126–139. doi:10.1093/toxsci/kfw147
Brüll, M., Spreng, A.-S., Gutbier, S., Loser, D., Krebs, A., Reich, M., et al. (2020). Incorporation of stem cell-derived astrocytes into neuronal organoids to allow neuro-glial interactions in toxicological studies. ALTEX - Altern. Animal Exp. 37 (3), 409–428. doi:10.14573/ALTEX.1911111
Bruni, G., Rennekamp, A. J., Velenich, A., McCarroll, M., Gendelev, L., Fertsch, E., et al. (2016). Zebrafish behavioral profiling identifies multitarget antipsychotic-like compounds. Nat. Chem. Biol. 12 (7), 559–566. doi:10.1038/nchembio.2097
Carson, M. J., Cameron Thrash, J., and Walter, B. (2006). The cellular response in neuroinflammation: the role of leukocytes, microglia and astrocytes in neuronal death and survival. Clin. Neurosci. Res. 6 (5), 237–245. doi:10.1016/J.CNR.2006.09.004
Chesnut, M., Paschoud, H., Repond, C., Smirnova, L., Hartung, T., Zurich, M.-G., et al. (2021). Human IPSC-derived model to study myelin disruption. Int. J. Mol. Sci. 22 (17), 9473. doi:10.3390/IJMS22179473
Chu, J., and Sadler, K. C. (2009). New school in liver development: lessons from zebrafish. Hepatology 50 (5), 1656–1663. doi:10.1002/HEP.23157
Costa, L. G., Aschner, M., Vitalone, A., Syversen, T., and Soldin, O. P. (2004). Developmental neuropathology of environmental agents. Annu. Rev. Pharmacol. Toxicol. 44, 87–110. doi:10.1146/annurev.pharmtox.44.101802.121424
Costa, L. G., Giordano, G., Guizzetti, M., and Vitalone, A. (2008). Neurotoxicity of pesticides: a brief review. Front. Biosci. 13 (4), 1240–1249. doi:10.2741/2758
Craig, E., Kelly, L., Akerman, G., Dawson, J., May, B., Reaves, E., et al. (2019). Reducing the need for animal testing while increasing efficiency in a pesticide regulatory setting: lessons from the EPA office of pesticide programs’ hazard and science policy Council. Regul. Toxicol. Pharmacol. RTP 108 (November), 104481. doi:10.1016/J.YRTPH.2019.104481
Crofton, K. M., and Mundy, W. R. (2021). External scientific report on the interpretation of data from the developmental neurotoxicity in vitro testing assays for use in integrated approaches for testing and assessment. EFSA Support. Publ. 18 (10). doi:10.2903/SP.EFSA.2021.EN-6924
Crofton, K. M., Mundy, W. R., and Shafer, T. J. (2012). Developmental neurotoxicity testing: a path forward. Congenit. Anomalies. Congenit. Anom. (Kyoto) 52, 140–146. doi:10.1111/j.1741-4520.2012.00377.x
Dach, K., Bendt, F., Huebenthal, U., Giersiefer, S., Lein, P. J., Heuer, H., et al. (2017). BDE-99 impairs differentiation of human and mouse NPCs into the oligodendroglial lineage by species-specific modes of action. Sci. Rep. 7 (44861), 44861. doi:10.1038/srep44861
Davidsen, N., Lauvås, A. J., Myhre, O., Ropstad, E., Carpi, D., Gyves, E. M. de, et al. (2021). Exposure to human relevant mixtures of halogenated persistent organic pollutants (POPs) alters neurodevelopmental processes in human neural stem cells undergoing differentiation. Reprod. Toxicol. 100 (April 2020), 17–34. doi:10.1016/j.reprotox.2020.12.013
Delp, J., Cediel-Ulloa, A., Suciu, I., Kranaster, P., Vugt-Lussenburg, B.Ma V., Munic Kos, V., et al. (2021). Neurotoxicity and underlying cellular changes of 21 mitochondrial respiratory chain inhibitors. Archives Toxicol. 95, 591–615. doi:10.1007/s00204-020-02970-5
Delp, J., Funke, M., Rudolf, F., Cediel, A., Bennekou, S. H., van der Stel, W., et al. (2019). Development of a neurotoxicity assay that is tuned to detect mitochondrial toxicants. Archives Toxicol. 93 (6), 1585–1608. doi:10.1007/S00204-019-02473-Y
Dickerson, A. S., Wu, A. C., Liew, Z., and Weisskopf, M. (2020). A scoping review of non-occupational exposures to environmental pollutants and adult depression, anxiety, and suicide. Curr. Environ. Health Rep. 7 (3), 256–271. doi:10.1007/s40572-020-00280-7
Druwe, I., Freudenrich, T. M., Wallace, K., Shafer, T. J., and Mundy, W. R. (2015). Sensitivity of neuroprogenitor cells to chemical-induced apoptosis using a multiplexed assay suitable for high-throughput screening. Toxicology 333 (July), 14–24. doi:10.1016/J.TOX.2015.03.011
Duboc, V., Dufourcq, P., Blader, P., and Roussigné, M. (2015). Asymmetry of the brain: development and implications. Annu. Rev. Genet. 49, 647–672. doi:10.1146/ANNUREV-GENET-112414-055322
Dubrana, L. E., Knoll-Gellida, A., Bourcier, L. M., Mercé, T., Pedemay, S., Nachon, F., et al. (2021). An antidote screening system for organophosphorus poisoning using zebrafish larvae. ACS Chem. Neurosci. 12 (15), 2865–2877. doi:10.1021/ACSCHEMNEURO.1C00251
ECHA (2023). Key areas of regulatory challenge. Eur. Chem. Agency. ECHA-23-R- (ED-07-23-212-EN-N). doi:10.2823/568850
EFSA Scientific Committee Hardy, A., Benford, D., Halldorsson, T., Jeger, M. J., Katrine Knutsen, H., More, S., et al. (2017). Guidance on the risk assessment of substances present in food intended for infants below 16 Weeks of age. EFSA J. 15 (5), e04849. doi:10.2903/J.EFSA.2017.4849
Eriksson, P. S., Perfilieva, E., Björk-Eriksson, T., Alborn, A. M., Nordborg, C., Peterson, D. A., et al. (1998). Neurogenesis in the adult human Hippocampus. Nat. Med. 4 (11), 1313–1317. doi:10.1038/3305
Escher, B. I., Altenburger, R., Blüher, M., Colbourne, J. K., Ebinghaus, R., Fantke, P., et al. (2023). Modernizing persistence-bioaccumulation-toxicity (PBT) assessment with high throughput animal-free methods. Archives Toxicol. 97 (5), 1267–1283. doi:10.1007/S00204-023-03485-5
European Commission (2020). Communication from the commission to the European parliament, the Council, the European economic and social committee and the committee of the regions. Chem. Strategy Sustain. - Towards a Toxic-Free Environ.
European Parliament and Council. 2006. “Regulation (EC) No. 1907/2006 of the European parliament and of the Council of 18 december 2006 concerning the registration, evaluation, authorisation and restriction of chemicals (REACH), establishing a European chemicals agency, amending directive 1999/45/EC and repealing Council regulation (EEC) No 793/93 and commission regulation (EC) No 1488/94 as well as Council directive 76/769/EEC and commission directives 91/155/EEC, 93/67/EEC, 93/105/EC and 2000/21/EC.”
European Parliament and Council. 2008. “Regulation (EC) No. 1272/2008 of the European parliament and the Council of 16 december 2008 on classification, labelling and packaging of substances, and mixtures, amending and repealing directives 67/548/EEC and 1999/45/EC, and amending regulation (EC) No 1907/2006.” In .
European Parliament and Council (2009). Regulation (EC) No. 1107/2009 of the European parliament and of the Council of 21 october 2009 concerning the placing of plant protection products on the market and repealing Council directives 79/117/EEC and 91/414/EEC. Paris: Brussels, OECD.
European Parliament and Council. 2010. “Directive 2010/63/EU of the European paliament and of the Council of 22 september 2010 on the protection of animals used for scientific purposes.” In .
European Parliament and Council (2012). Regulation (EU) No. 528/2012 of the European parliament and the Council of 22 may 2012 concerning the making available on the market and use of biocidal products. Available at: https://eur-lex.europa.eu/legal-content/EN/TXT/HTML/?uri=CELEX:02012R0528-20220415.
European Parliament and Council (2023). Commission delegated regulation (EU) 2023/707 of 19 december 2022 amending regulation (EC) No 1272/2008 as regards hazard classes and criteria for the classification, labelling and packaging of substances and mixtures. Available at: https://publications.europa.eu/resource/cellar/5fe7da2d-cf65-11ed-a05c-01aa75ed71a1.0006.03/DOC_1.
Faria, M., Garcia-Reyero, N., Padrós, F., Babin, P. J., Sebastián, D., Cachot, J., et al. (2015). Zebrafish models for human acute organophosphorus poisoning. Sci. Rep. 5 (October), 15591. doi:10.1038/SREP15591
Farrell, J. A., Wang, Y., Riesenfeld, S. J., Shekhar, K., Regev, A., and Schier, A. F. (2018). Single-cell reconstruction of developmental trajectories during zebrafish embryogenesis. Science 360 (6392), eaar3131. doi:10.1126/science.aar3131
Frank, C. L., Brown, J. P., Wallace, K., Mundy, W. R., and Shafer, T. J. (2017). From the cover: developmental neurotoxicants disrupt activity in cortical networks on microelectrode arrays: results of screening 86 compounds during neural network formation. Toxicol. Sci. 160 (1), 121–135. doi:10.1093/toxsci/kfx169
Fritsche, E. (2017). “Report on integrated testing strategies for the identification and evaluation of chemical hazards associated with the developmental neurotoxicity (DNT), to facilitate discussions at the Joint EFSA/OECD workshop on DNT.” ENV/JM/MONO(2017)4/ANN1.
Fritsche, E., Grandjean, P., Crofton, K. M., Aschner, M., Goldberg, A., Heinonen, T., et al. (2018). Consensus statement on the need for innovation, transition and implementation of developmental neurotoxicity (DNT) testing for regulatory purposes. Toxicol. Appl. Pharmacol. 354 (September), 3–6. doi:10.1016/J.TAAP.2018.02.004
Gaballah, S., Swank, A., Sobus, J. R., Meng Howey, X., Schmid, J., Catron, T., et al. (2020). Evaluation of developmental toxicity, developmental neurotoxicity, and tissue dose in zebrafish exposed to GenX and other PFAS. Environ. Health Perspect. 128 (4), 47005. doi:10.1289/EHP5843
Gadaleta, D., Spînu, N., Roncaglioni, A., Cronin, M. T. D., and Benfenati, E. (2022). Prediction of the neurotoxic potential of chemicals based on modelling of molecular initiating events upstream of the adverse outcome pathways of (developmental) neurotoxicity. Int. J. Mol. Sci. 23 (6), 3053. doi:10.3390/ijms23063053
Giordano, G., and Costa, L. G. (2012). Developmental neurotoxicity: some old and new issues. ISRN Toxicol. 2012 (June), 814795–814812. doi:10.5402/2012/814795
Glaberman, S., Padilla, S., and Barron, M. G. (2017). Evaluating the zebrafish embryo toxicity test for pesticide hazard screening. Environ. Toxicol. Chem. 36 (5), 1221–1226. doi:10.1002/ETC.3641
Grandjean, P., and Landrigan, P. J. (2006). Developmental neurotoxicity of industrial chemicals. Lancet 368 (9553), 2167–2178. doi:10.1016/S0140-6736(06)69665-7
Grandjean, P., and Landrigan, P. J. (2014). Neurobehavioural effects of developmental toxicity. Lancet Neurology 13 (3), 330–338. doi:10.1016/S1474-4422(13)70278-3
Grillberger, K., Cöllen, E., Immacolata Trivisani, C., Blum, J., Leist, M., and Ecker, G. F. (2023). Structural insights into neonicotinoids and N-unsubstituted metabolites on human NAChRs by molecular docking, dynamics simulations, and calcium imaging. Int. J. Mol. Sci. 24 (17), 13170. doi:10.3390/IJMS241713170
Gunnarsson, L., Jauhiainen, A., Kristiansson, E., Nerman, O., and Joakim Larsson, D. G. (2008). Evolutionary conservation of human drug targets in organisms used for environmental risk assessments. Environ. Sci. Technol. 42 (15), 5807–5813. doi:10.1021/es8005173
Gupta, T., Marquart, G. D., Horstick, E. J., Tabor, K. M., Pajevic, S., and Burgess, H. A. (2018). Morphometric analysis and neuroanatomical mapping of the zebrafish brain. Methods 150 (November), 49–62. doi:10.1016/J.YMETH.2018.06.008
Gutbier, S., Spreng, A. S., Delp, J., Schildknecht, S., Karreman, C., Suciu, I., et al. (2018). Prevention of neuronal apoptosis by astrocytes through thiol-mediated stress response modulation and accelerated recovery from proteotoxic stress. Cell Death Differ. 25 (12), 2101–2117. doi:10.1038/S41418-018-0229-X
Harrill, J. A., Freudenrich, T., Wallace, K., Ball, K., Shafer, T. J., and Mundy, W. R. (2018). Testing for developmental neurotoxicity using a battery of in vitro assays for key cellular events in neurodevelopment. Toxicol. Appl. Pharmacol. 354 (September), 24–39. doi:10.1016/J.TAAP.2018.04.001
Harrill, J. A., Freudenrich, T. M., Machacek, D. W., Stice, S. L., and Mundy, W. R. (2010). Quantitative assessment of neurite outgrowth in human embryonic stem cell-derived HN2 cells using automated high-content image analysis. Neurotoxicology 31 (3), 277–290. doi:10.1016/J.NEURO.2010.02.003
Harrill, J. A., Robinette, B. L., Freudenrich, T., and Mundy, W. R. (2013). Use of high content image analyses to detect chemical-mediated effects on neurite sub-populations in primary rat cortical neurons. NeuroToxicology 34 (1), 61–73. doi:10.1016/J.NEURO.2012.10.013
Harrill, J. A., Robinette, B. L., and Mundy, W. R. (2011). Use of high content image analysis to detect chemical-induced changes in synaptogenesis in vitro. Toxicol. Vitro 25 (1), 368–387. doi:10.1016/j.tiv.2010.10.011
Harry, G. J., McBride, S., Witchey, S. K., Mhaouty-Kodja, S., Trembleau, A., Bridge, M., et al. (2022). Roadbumps at the crossroads of integrating behavioral and in vitro approaches for neurotoxicity assessment. Front. Toxicol. 4, 812863. doi:10.3389/FTOX.2022.812863
Hartmann, J., Henschel, N., Bartmann, K., Dönmez, A., Brockerhoff, G., Koch, K., et al. (2023). Molecular and functional characterization of different BrainSphere models for use in neurotoxicity testing on microelectrode arrays. Cells 12 (9), 1270. doi:10.3390/cells12091270
Hoelting, L., Klima, S., Karreman, C., Grinberg, M., Meisig, J., Henry, M., et al. (2016). Stem cell-derived immature human dorsal root ganglia neurons to identify peripheral neurotoxicants. Stem Cells Transl. Med. 5 (4), 476–487. doi:10.5966/SCTM.2015-0108
Hollander, J. A., Cory-Slechta, D. A., Jacka, F. N., Szabo, S. T., Guilarte, T. R., Bilbo, S. D., et al. (2020). Beyond the looking glass: recent advances in understanding the impact of environmental exposures on neuropsychiatric disease. Neuropsychopharmacology 45 (7), 1086–1096. doi:10.1038/s41386-020-0648-5
Holzer, A. K., Karreman, C., Suciu, I., Furmanowsky, L. S., Wohlfarth, H., Loser, D., et al. (2022). Generation of human nociceptor-enriched sensory neurons for the study of pain-related dysfunctions. Stem Cells Transl. Med. 11 (7), 727–741. doi:10.1093/STCLTM/SZAC031
Hooijmans, C. R., Leenaars, M., and Ritskes-Hoitinga, M. (2010). A gold standard publication checklist to improve the quality of animal studies, to fully integrate the three rs, and to make systematic reviews more feasible. Altern. Lab. Anim. 38 (2), 167–182. doi:10.1177/026119291003800208
Horzmann, K. A., and Freeman, J. L. (2016). Zebrafish get connected: investigating neurotransmission targets and alterations in chemical toxicity. Toxics 4 (3), 19. doi:10.3390/TOXICS4030019
Howe, K., Clark, M. D., Torroja, C. F., Torrance, J., Berthelot, C., Muffato, M., et al. (2013). The zebrafish reference genome sequence and its relationship to the human genome. Nature 496, 498–503. doi:10.1038/nature12111
Imayoshi, I., Shimojo, H., Sakamoto, M., Ohtsuka, T., and Kageyama, R. (2013). Genetic visualization of Notch signaling in mammalian neurogenesis. Cell. Mol. Life Sci. 70, 2045–2057. doi:10.1007/s00018-012-1151-x
Jacobson, M. H., Ghassabian, A., Gore, A. C., and Trasande, L. (2022). Exposure to environmental chemicals and perinatal psychopathology. Biochem. Pharmacol. 195 (January), 114835. doi:10.1016/J.BCP.2021.114835
Jarema, K. A., Hunter, D. L., Hill, B. N., Olin, J. K., Britton, K. N., Waalkes, M. R., et al. (2022). Developmental neurotoxicity and behavioral screening in larval zebrafish with a comparison to other published results. Toxics 10 (5), 256. doi:10.3390/toxics10050256
Kalueff, A. V., Gebhardt, M., Stewart, A. M., Cachat, J. M., Brimmer, M., Chawla, J. S., et al. (2013). Towards a comprehensive catalog of zebrafish behavior 1.0 and beyond. Zebrafish 10 (1), 70–86. Https://Home.Liebertpub.Com/Zeb. doi:10.1089/ZEB.2012.0861
Kang, Du S., Yang, Y. R., Lee, C., Kim, S. B., Ryu, S.Ho, and Suh, P. G. (2016). Roles of phosphoinositide-specific phospholipase Cγ1 in brain development. Adv. Biol. Regul. 60 (January), 167–173. doi:10.1016/J.JBIOR.2015.10.002
Kavlock, R. J., Bahadori, T., Barton-Maclaren, T. S., Gwinn, M. R., Rasenberg, M., and Thomas, R. S. (2018). Accelerating the pace of chemical risk assessment. Chem. Res. Toxicol. 31 (5), 287–290. doi:10.1021/ACS.CHEMRESTOX.7B00339
Kettleborough, R. N. W., Busch-Nentwich, E. M., Harvey, S. A., Dooley, C. M., De Bruijn, E., Van Eeden, F., et al. (2013). A systematic genome-wide analysis of zebrafish protein-coding gene function. Nature 496 (7446), 494–497. doi:10.1038/NATURE11992
Khelfaoui, H., Harkati, D., and Saleh, B. A. (2021). Molecular docking, molecular dynamics simulations and reactivity, studies on approved drugs library targeting ACE2 and SARS-CoV-2 binding with ACE2. J. Biomol. Struct. Dyn. 39 (18), 7246–7262. doi:10.1080/07391102.2020.1803967
Klima, S., Brüll, M., Spreng, A. S., Suciu, I., Falt, T., Schwamborn, J. C., et al. (2021). A human stem cell-derived test system for agents modifying neuronal N-Methyl-D-Aspartate-Type glutamate receptor Ca 2+-signalling. Archives Toxicol. 95 (5), 1703–1722. doi:10.1007/S00204-021-03024-0
Klose, J., Tigges, J., Masjosthusmann, S., Schmuck, K., Bendt, F., Hübenthal, U., et al. (2021). TBBPA targets converging key events of human oligodendrocyte development resulting in two novel AOPs. Altex 38 (2), 215–234. doi:10.14573/altex.2007201
Klüver, N., Vogs, C., Altenburger, R., Escher, B. I., and Scholz, S. (2016). Development of a general baseline toxicity QSAR model for the fish embryo acute toxicity test. Chemosphere 164 (December), 164–173. doi:10.1016/J.CHEMOSPHERE.2016.08.079
Knoll-Gellida, A., Dubrana, L. E., Bourcier, L. M., eo Merc, Th, Gruel, E., Soares, M., et al. (2021). Hyperactivity and seizure induced by tricresyl phosphate are isomer specific and not linked to phenyl valerate-neuropathy target esterase activity inhibition in zebrafish. Toxicol. Sci. 180 (1), 160–174. doi:10.1093/toxsci/kfab006
Koch, K., Bartmann, K., Hartmann, J., Kapr, J., Pahl, M., Fritsche, E., et al. (2022). Scientific validation of human neurosphere assays for developmental neurotoxicity evaluation. Front. Press 0, 816370–816438. doi:10.3389/FTOX.2022.816370
Krebs, A., Waldmann, T., Wilks, M. F., van Vugt-Lussenburg, B. M. A., van der Burg, B., Terron, A., et al. (2019). Template for the description of cell-based toxicological test methods to allow evaluation and regulatory use of the data. ALTEX - Altern. Animal Exp. 36 (4), 682–699. doi:10.14573/ALTEX.1909271
Krug, A. K., Balmer, N. V., Matt, F., Schönenberger, F., Merhof, D., and Leist, M. (2013). Evaluation of a human neurite growth assay as specific screen for developmental neurotoxicants. Archives Toxicol. 87 (12), 2215–2231. doi:10.1007/s00204-013-1072-y
Krzykwa, J. C., Saeid, A., and Sellin Jeffries, M. K. (2019). Identifying sublethal endpoints for evaluating neurotoxic compounds utilizing the fish embryo toxicity test. Ecotoxicol. Environ. Saf. 170 (April), 521–529. doi:10.1016/J.ECOENV.2018.11.118
Landrigan, P. J., Sonawane, B., Butler, R. N., Trasande, L., Callan, R., and Droller, D. (2005). Early environmental origins of neurodegenerative disease in later life. Environ. Health Perspect. 113 (9), 1230–1233. doi:10.1289/EHP.7571
Lauvås, A. J., Lislien, M., Andreas Holme, J., Dirven, H., Paulsen, E., Alm, I. M., et al. (2022). Developmental neurotoxicity of acrylamide and its metabolite glycidamide in a human mixed culture of neurons and astrocytes undergoing differentiation in concentrations relevant for human exposure. Neurotoxicology 92, 33–48. doi:10.1016/j.neuro.2022.07.001
Lee, Da Y. (2015). Roles of MTOR signaling in brain development. Exp. Neurobiol. 24 (3), 177–185. doi:10.5607/EN.2015.24.3.177
Lee, J., Escher, B. I., Scholz, S., and Schlichting, R. (2022a). Inhibition of neurite outgrowth and enhanced effects compared to baseline toxicity in SH-SY5Y cells. Archives Toxicol. 96 (4), 1039–1053. doi:10.1007/s00204-022-03237-x
Lee, J., Schlichting, R., König, M., Scholz, S., Krauss, M., and Escher, B. I. (2022b). Monitoring mixture effects of neurotoxicants in surface water and wastewater treatment plant effluents with neurite outgrowth inhibition in SH-SY5Y cells. Am. Chem. Soc. 2, 523–535. doi:10.1021/acsenvironau.2c00026
Leist, M., Ahmed, G., Graepel, R., Marchan, R., Hassan, R., Hougaard Bennekou, S., et al. (2017). Adverse outcome pathways: opportunities, limitations and open questions. Archives Toxicol. 91 (11), 3477–3505. doi:10.1007/S00204-017-2045-3
López-Espíndola, D., Morales-Bastos, C., Grijota-Martínez, C., Liao, X. H., Lev, D., Sugo, E., et al. (2014). Mutations of the thyroid hormone transporter MCT8 cause prenatal brain damage and persistent hypomyelination. J. Clin. Endocrinol. Metabolism 99 (12), E2799–E2804. doi:10.1210/jc.2014-2162
Loser, D., Hinojosa, M. G., Blum, J., Schaefer, J., Brüll, M., Johansson, Y., et al. (2021). Functional alterations by a subgroup of neonicotinoid pesticides in human dopaminergic neurons. Archives Toxicol. 95 (6), 2081–2107. doi:10.1007/S00204-021-03031-1
Louit, A., Beaudet, M. J., Pépin, R., and Berthod, F. (2023). Differentiation of human induced pluripotent stem cells into mature and myelinating Schwann cells. Https//Home.Liebertpub.Com/Tec 29 (4), 134–143. doi:10.1089/TEN.TEC.2022.0186
Makris, S. L., Raffaele, K., Allen, S., Bowers, W. J., Hass, U., Alleva, E., et al. (2009). A retrospective performance assessment of the developmental neurotoxicity study in support of OECD test guideline 426. Environ. Health Perspect. 117 (1), 17–25. doi:10.1289/EHP.11447
Marx-Stoelting, P., Rivière, G., Luijten, · M., Aiello-Holden, · K., Bandow, · N., Baken, · K., et al. (2023). A walk in the PARC: developing and implementing 21st century chemical risk assessment in Europe. Arch. Toxicol. 97, 893–908. doi:10.1007/s00204-022-03435-7
Masjosthusmann, S., Barenys, M., El-Gamal, M., Geerts, L., Gerosa, L., Gorreja, A., et al. (2018). Literature review and appraisal on alternative neurotoxicity testing methods. EFSA Support. Publ. 15 (4), 1–108. doi:10.2903/sp.efsa.2018.en-1410
Masjosthusmann, S., Blum, J., Bartmann, K., Dolde, X., Holzer, A.-K., Stürzl, L.-C., et al. (2020). Establishment of an a priori protocol for the implementation and interpretation of an in-vitro testing battery for the assessment of developmental neurotoxicity. EFSA Supporting Publication. Wiley. doi:10.2903/sp.efsa.2020.en-1938
Muller, Q., Beaudet, M. J., De Serres-Bérard, T., Bellenfant, S., Vincent, F., and Berthod, F. (2018). Development of an innervated tissue-engineered skin with human sensory neurons and Schwann cells differentiated from IPS cells. Acta Biomater. 82 (December), 93–101. doi:10.1016/J.ACTBIO.2018.10.011
Nimtz, L., Klose, J., Masjosthusmann, S., Barenys, M., and Fritsche, E. (2019). The neurosphere assay as an in vitro method for developmental neurotoxicity (DNT) evaluation. Eval. Neuromethods 145, 141–168. doi:10.1007/978-1-4939-9228-7_8
Noyes, P. D., Haggard, D. E., Gonnerman, G. D., and Tanguay, R. L. (2015). Advanced morphological — behavioral test platform reveals neurodevelopmental defects in embryonic zebrafish exposed to comprehensive suite of halogenated and organophosphate flame retardants. Toxicol. Sci. 145 (1), 177–195. doi:10.1093/TOXSCI/KFV044
Nyffeler, J., Dolde, X., Krebs, A., Pinto-Gil, K., Pastor, M., Behl, M., et al. (2017). Combination of multiple neural crest migration assays to identify environmental toxicants from a proof-of-concept chemical library. Archives Toxicol. 91 (11), 3613–3632. doi:10.1007/s00204-017-1977-y
Ockleford, C., Adriaanse, P., Berny, P., Brock, T., Duquesne, S., Grilli, S., et al. (2017). Investigation into experimental toxicological properties of plant protection products having a potential link to Parkinson’s disease and childhood leukaemia. EFSA J. 15 (3), e04691. doi:10.2903/J.EFSA.2017.4691
OECD. (1995a). “Test No. 418: delayed neurotoxicity of organophosphorus substances following acute exposure.” In . OECD guidelines for the testing of chemicals, Section 4. OECD. doi:10.1787/9789264070905-en
OECD. (1995b). “Test No. 419: delayed neurotoxicity of organophosphorus substances: 28-day repeated dose study.” In . OECD guidelines for the testing of chemicals, Section 4. OECD. doi:10.1787/9789264070929-en
OECD. (1997). “Test No. 424: neurotoxicity study in rodents.” In . OECD guidelines for the testing of chemicals, Section 4. OECD. doi:10.1787/9789264071025-en
OECD. (2002a). “Test No. 420: acute oral toxicity - fixed dose procedure.” In . OECD guidelines for the testing of chemicals, Section 4. OECD. doi:10.1787/9789264070943-en
OECD. (2002b). “Test No. 423: acute oral toxicity - acute toxic class method.” In . OECD guidelines for the testing of chemicals, Section 4. OECD. doi:10.1787/9789264071001-en
OECD. (2005). “Guidance document on the validation and international acceptance of new or updated test methods for hazard assessment, series on testing and assessment No. 34.” In .
OECD. 2007. “Test No. 426: developmental neurotoxicity study.” In . OECD guidelines for the testing of chemicals, Section 4. OECD. doi:10.1787/9789264067394-en
OECD (2008a). Series on testing and assessment No. 89 - retrospective performance assessment of the test guideline 426 on developmental neurotoxicity. ENV/JM/MON (89).
OECD. 2008b. “Test No. 407: repeated dose 28-day oral toxicity study in rodents.” In . OECD guidelines for the testing of chemicals, Section 4. OECD. doi:10.1787/9789264070684-en
OECD. (2009). “Test No. 403: acute inhalation toxicity.” In . OECD guidelines for the testing of chemicals, Section 4. OECD. doi:10.1787/9789264070608-en
OECD. (2017). “Test No. 402: acute dermal toxicity.” In . OECD guidelines for the testing of chemicals, Section 4. OECD. doi:10.1787/9789264070585-en
OECD. (2018a). “Test No. 408: repeated dose 90-day oral toxicity study in rodents.” In . OECD guidelines for the testing of chemicals, Section 4. OECD. doi:10.1787/9789264070707-en
OECD. (2018b). Test No. 443: extended one-generation reproductive toxicity study. doi:10.1787/20745788
OECD. (2022). “Test No. 425: acute oral toxicity - up-and-down procedure.” In . OECD guidelines for the testing of chemicals, Section 4. OECD. doi:10.1787/9789264071049-en
OECD (2023). Initial recommendations on evaluation of data from the developmental neurotoxicity (DNT) in-vitro testing battery. Ser. Test. Assess. 377 (13). ENV/CBC/MONO(2023. Available at: https://www.oecd.org/env/ehs/testing/developmental-neurotoxicity.htm.
Pallocca, G., Moné, M. J., Kamp, H., Luijten, M., van de Water, B., and Leist, M. (2022). Next-generation risk assessment of chemicals - rolling out a human-centric testing strategy to drive 3R implementation: the RISK-hunt3r project perspective. Altex 39 (3), 419–426. doi:10.14573/ALTEX.2204051
Pamies, D., Leist, M., Coecke, S., Bowe, G., Allen, D. G., Gstraunthaler, G., et al. (2022). Guidance document on Good cell and tissue culture practice 2.0 (GCCP 2.0). ALTEX 39 (1), 30–70. doi:10.14573/ALTEX.2111011
Paparella, M., Bennekou, S. H., and Bal-Price, A. (2020). An analysis of the limitations and uncertainties of in vivo developmental neurotoxicity testing and assessment to identify the potential for alternative approaches. Reprod. Toxicol. 96 (September), 327–336. doi:10.1016/J.REPROTOX.2020.08.002
Pitzer, E. M., Shafer, T. J., and Herr, D. W. (2023). Identification of Neurotoxicology (NT)/Developmental Neurotoxicology (DNT) adverse outcome pathways and key event linkages with in vitro DNT screening assays. Neurotoxicology 99 (December), 184–194. doi:10.1016/J.NEURO.2023.10.007
Raj, B., Wagner, D. E., McKenna, A., Pandey, S., Klein, A. M., Shendure, J., et al. (2018). Simultaneous single-cell profiling of lineages and cell types in the vertebrate brain. Nat. Biotechnol. 36 (5), 442–450. doi:10.1038/nbt.4103
Rice, D., and Barone, S. (2000). Critical periods of vulnerability for the developing nervous system: evidence from humans and animal models. Environ. Health Perspect. 108 (Suppl. 3), 511–533. doi:10.1289/ehp.00108s3511
Rietdijk, J., Aggarwal, T., Georgieva, P., Lapins, M., Carreras-Puigvert, J., and Spjuth, O. (2022). Morphological profiling of environmental chemicals enables efficient and untargeted exploration of combination effects, Sci. Total Environ. 832, 155058. doi:10.1016/j.scitotenv.2022.155058
Rokoff, L. B., Shoaff, J. R., Coull, B. A., Enlow, M. B., Bellinger, D. C., and Korrick, S. A. (2022). Prenatal exposure to a mixture of organochlorines and metals and internalizing symptoms in childhood and adolescence. Environ. Res. 208 (May), 112701. doi:10.1016/J.ENVRES.2022.112701
Sachana, M., Bal-Price, A., Crofton, K. M., Bennekou, S. H., Shafer, T. J., Behl, M., et al. (2019). International regulatory and scientific effort for improved developmental neurotoxicity testing. Toxicol. Sci. 167 (1), 45–57. doi:10.1093/toxsci/kfy211
Saili, K. S., Todd, J. Z., Schwab, A. J., Silvin, A., Baker, N. C., Sidney Hunter, E., et al. (2017). Blood-brain barrier development: systems modeling and predictive Toxicology. Birth Defects Res. 109 (20), 1680–1710. doi:10.1002/BDR2.1180
Sakai, C., Ijaz, S., Hoffman, E. J., Grünblatt, E., Carl, M., and Lange, M. (2018). Zebrafish models of neurodevelopmental disorders: past, present, and future. Front. Mol. Neurosci. 11, 294. doi:10.3389/fnmol.2018.00294
Sato-Maeda, M., Tawarayama, H., Obinata, M., Kuwada, J. Y., and Shoji, W. (2006). Sema3a1 guides spinal motor axons in a cell- and stage-specific manner in zebrafish. Dev. Camb. Engl. 133 (5), 937–947. doi:10.1242/DEV.02268
Schenke, M., Schjeide, B. M., Püschel, G. P., and Seeger, B. (2020). Analysis of motor neurons differentiated from human induced pluripotent stem cells for the use in cell-based botulinum neurotoxin activity assays. Toxins 12 (5), 276. doi:10.3390/TOXINS12050276
Schmeisser, S., Miccoli, A., von Bergen, M., Berggren, E., Braeuning, A., Busch, W., et al. (2023). New approach methodologies in human regulatory Toxicology – not if, but how and when. Environ. Int. 178 (March), 108082. doi:10.1016/J.ENVINT.2023.108082
Schmidt, B. Z., Lehmann, M., Gutbier, S., Nembo, E., Noel, S., Smirnova, L., et al. (2017). In vitro acute and developmental neurotoxicity screening: an overview of cellular platforms and high-throughput technical possibilities. Archives Toxicol. 91 (1), 1–33. doi:10.1007/S00204-016-1805-9
Schmidt, R., Strähle, U., and Scholpp, S. (2013). Neurogenesis in zebrafish - from embryo to adult. Neural Dev. 8 (1), 3–13. doi:10.1186/1749-8104-8-3
Schmuck, M. R., Temme, T., Dach, K., Boer, D. de, Barenys, M., Bendt, F., et al. (2017). Omnisphero: a high-content image analysis (hca) approach for phenotypic developmental neurotoxicity (DNT) screenings of organoid neurosphere cultures in vitro. Archives Toxicol. 91 (4), 2017–2028. doi:10.1007/s00204-016-1852-2
Semple, B. D., Blomgren, K., Gimlin, K., Ferriero, D. M., and Noble-Haeusslein, L. J. (2013). Brain development in rodents and humans: identifying benchmarks of maturation and vulnerability to injury across species. Prog. Neurobiol. 106-107, 1–16. 0 (July). doi:10.1016/J.PNEUROBIO.2013.04.001
Shafer, T. J. (2019). Application of microelectrode array approaches to neurotoxicity testing and screening. Adv. Neurobiol. 22, 275–297. doi:10.1007/978-3-030-11135-9_12
Sheets, L. P., Li, A. A., Minnema, D. J., Collier, R. H., Creek, M. R., and Peffer, R. C. (2016). A critical review of neonicotinoid insecticides for developmental neurotoxicity. Crit. Rev. Toxicol. 46 (2), 153–190. doi:10.3109/10408444.2015.1090948
Smirnova, L., Hogberg, H. T., Leist, M., and Hartung, T. (2014). Food for thought.: developmental neurotoxicity - challenges in the 21st century and in vitro opportunities. Altex 31 (2), 129–156. doi:10.14573/ALTEX.1403271
Sobanska, M., Scholz, S., Nyman, A. M., Cesnaitis, R., Alonso, S. G., Klüver, N., et al. (2018). Applicability of the fish embryo acute toxicity (FET) test (OECD 236) in the regulatory context of registration, evaluation, authorisation, and restriction of chemicals (REACH). Environ. Toxicol. Chem. 37 (3), 657–670. doi:10.1002/ETC.4055
Spalding, K. L., Bergmann, O., Alkass, K., Bernard, S., Salehpour, M., Huttner, H. B., et al. (2013). Dynamics of hippocampal neurogenesis in adult humans. Cell 153 (6), 1219–1227. doi:10.1016/j.cell.2013.05.002
Spencer, P. S., and Lein, P. J. (2014). “Neurotoxicity,” in Encyclopedia of Toxicology (January, Third Edition), 489–500. doi:10.1016/B978-0-12-386454-3.00169-X
Spînu, N., Cronin, M. T. D., Lao, J., Bal-Price, A., Campia, I., Enoch, S. J., et al. (2021). Probabilistic modelling of developmental neurotoxicity based on a simplified adverse outcome pathway network. Comput. Toxicol., 100206. doi:10.1016/j.comtox.2021.100206
Spreng, A. S., Brüll, M., Leisner, H., Suciu, I., and Leist, M. (2022). Distinct and dynamic transcriptome adaptations of IPSC-generated astrocytes after cytokine stimulation. Cells 11 (17), 2644. doi:10.3390/CELLS11172644
Strähle, U., Scholz, S., Geisler, R., Greiner, P., Hollert, H., Rastegar, S., et al. (2012). Zebrafish embryos as an alternative to animal experiments—a commentary on the definition of the onset of protected life stages in animal welfare regulations. Reprod. Toxicol. 33 (2), 128–132. doi:10.1016/J.REPROTOX.2011.06.121
Stucki, A. O., Barton-Maclaren, T. S., Bhuller, Y., Henriquez, J. E., Henry, T. R., Hirn, C., et al. (2022). Use of new approach methodologies (NAMs) to meet regulatory requirements for the assessment of industrial chemicals and pesticides for effects on human health. Front. Toxicol. 4, 964553. doi:10.3389/FTOX.2022.964553
Suciu, I., Pamies, D., Peruzzo, R., Wirtz, P. H., Smirnova, L., Pallocca, G., et al. (2023). G × E interactions as a basis for toxicological uncertainty. Archives Toxicol. 97 (7), 2035–2049. doi:10.1007/S00204-023-03500-9
Tanner, C. M., Goldman, S. M., Webster Ross, G., and Grate, S. J. (2014). The disease intersection of susceptibility and exposure: chemical exposures and neurodegenerative disease risk. Alzheimer’s Dementia 10 (3 Suppl. L), S213–S225. doi:10.1016/J.JALZ.2014.04.014
Terron, A., and Bennekou, S. H. (2018). Towards a regulatory use of alternative developmental neurotoxicity testing (DNT). Toxicol. Appl. Pharmacol. 354 (December 2017), 19–23. doi:10.1016/j.taap.2018.02.002
Tian, G., Harrison, P. J., Sreenivasan, A. P., Carreras-Puigvert, J., and Spjuth, O. (2023). Combining molecular and cell painting image data for mechanism of action prediction. Artif. Intell. Life Sci. 3 (December), 100060. doi:10.1016/J.AILSCI.2023.100060
Tsuji, R., and Crofton, K. M. (2012). Developmental neurotoxicity guideline study: issues with methodology, evaluation and regulation. Congenit. Anomalies 52 (3), 122–128. doi:10.1111/J.1741-4520.2012.00374.X
Vinken, M., Benfenati, E., Busquet, F., Castell, J., Clevert, D.-A., de Kok, T. M., et al. (2021). Safer chemicals using less animals: kick-off of the European ONTOX project. Toxicology 458 (June), 152846. doi:10.1016/J.TOX.2021.152846
Keywords: new approach method (NAM), developmental neurotoxicity (DNT), adult neurotoxicity (ANT), DNT-IVB, zebrafish, applicability domain
Citation: Tal T, Myhre O, Fritsche E, Rüegg J, Craenen K, Aiello-Holden K, Agrillo C, Babin PJ, Escher BI, Dirven H, Hellsten K, Dolva K, Hessel E, Heusinkveld HJ, Hadzhiev Y, Hurem S, Jagiello K, Judzinska B, Klüver N, Knoll-Gellida A, Kühne BA, Leist M, Lislien M, Lyche JL, Müller F, Colbourne JK, Neuhaus W, Pallocca G, Seeger B, Scharkin I, Scholz S, Spjuth O, Torres-Ruiz M and Bartmann K (2024) New approach methods to assess developmental and adult neurotoxicity for regulatory use: a PARC work package 5 project. Front. Toxicol. 6:1359507. doi: 10.3389/ftox.2024.1359507
Received: 21 December 2023; Accepted: 18 March 2024;
Published: 26 April 2024.
Edited by:
Lena Smirnova, Johns Hopkins University, United StatesReviewed by:
Sandra McBride, Social and Scientific Systems, United StatesCopyright © 2024 Tal, Myhre, Fritsche, Rüegg, Craenen, Aiello-Holden, Agrillo, Babin, Escher, Dirven, Hellsten, Dolva, Hessel, Heusinkveld, Hadzhiev, Hurem, Jagiello, Judzinska, Klüver, Knoll-Gellida, Kühne, Leist, Lislien, Lyche, Müller, Colbourne, Neuhaus, Pallocca, Seeger, Scharkin, Scholz, Spjuth, Torres-Ruiz and Bartmann. This is an open-access article distributed under the terms of the Creative Commons Attribution License (CC BY). The use, distribution or reproduction in other forums is permitted, provided the original author(s) and the copyright owner(s) are credited and that the original publication in this journal is cited, in accordance with accepted academic practice. No use, distribution or reproduction is permitted which does not comply with these terms.
*Correspondence: Tamara Tal, dGFtYXJhLnRhbEB1ZnouZGU=; Kristina Bartmann, a3Jpc3RpbmEuYmFydG1hbm5AaXVmLWR1ZXNzZWxkb3JmLmRl